the Creative Commons Attribution 4.0 License.
the Creative Commons Attribution 4.0 License.
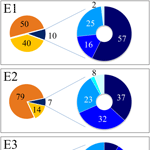
Transfer of diazotroph-derived nitrogen to the planktonic food web across gradients of N2 fixation activity and diversity in the western tropical South Pacific Ocean
Mathieu Caffin
Hugo Berthelot
Véronique Cornet-Barthaux
Aude Barani
Sophie Bonnet
Biological dinitrogen (N2) fixation provides the major source of new nitrogen (N) to the open ocean, contributing more than atmospheric deposition and riverine inputs to the N supply. Yet the fate of the diazotroph-derived N (DDN) in the planktonic food web is poorly understood. The main goals of this study were (i) to quantify how much of DDN is released to the dissolved pool during N2 fixation and how much is transferred to bacteria, phytoplankton and zooplankton, and (ii) to compare the DDN release and transfer efficiencies under contrasting N2 fixation activity and diversity in the oligotrophic waters of the western tropical South Pacific (WTSP) Ocean. We used nanometre-scale secondary ion mass spectrometry (nanoSIMS) coupled with 15N2 isotopic labelling and flow cytometry cell sorting to track the DDN transfer to plankton, in regions where the diazotroph community was dominated by either Trichodesmium or by UCYN-B. After 48 h, ∼ 20–40 % of the N2 fixed during the experiment was released to the dissolved pool when Trichodesmium dominated, while the DDN release was not quantifiable when UCYN-B dominated; ∼ 7–15 % of the total fixed N (net N2 fixation + release) was transferred to non-diazotrophic plankton within 48 h, with higher transfer efficiencies (15 ± 3 %) when UCYN-B dominated as compared to when Trichodesmium dominated (9 ± 3 %). The pico-cyanobacteria Synechococcus and Prochlorococcus were the primary beneficiaries of the DDN transferred (∼ 65–70 %), followed by heterotrophic bacteria (∼ 23–34 %). The DDN transfer in bacteria was higher (34 ± 7 %) in the UCYN-B-dominating experiment compared to the Trichodesmium-dominating experiments (24 ± 5 %). Regarding higher trophic levels, the DDN transfer to the dominant zooplankton species was less efficient when the diazotroph community was dominated by Trichodesmium (∼ 5–9 % of the DDN transfer) than when it was dominated by UCYN-B (∼ 28 ± 13 % of the DDN transfer). To our knowledge, this study provides the first quantification of DDN release and transfer to phytoplankton, bacteria and zooplankton communities in open ocean waters. It reveals that despite UCYN-B fix N2 at lower rates compared to Trichodesmium in the WTSP, the DDN from UCYN-B is much more available and efficiently transferred to the planktonic food web than the DDN originating from Trichodesmium.
- Article
(2361 KB) - Full-text XML
-
Supplement
(758 KB) - BibTeX
- EndNote
Nitrogen (N) is one of the basic building blocks of life, though much of the global ocean surface (∼ 70 %) is oligotrophic and characterized by low N availability, which limits primary productivity and phytoplankton growth (Falkowski, 1997; Moore et al., 2013). In these N-depleted areas of the tropical and subtropical ocean, biological dinitrogen (N2) fixation (the reduction of atmospheric N2 into bioavailable ammonia) sustains the major part of new production and organic matter export (Bonnet et al., 2009; Caffin et al., 2018; Capone et al., 2005; Karl et al., 2012). At the global scale, N2 fixation is the major source of new N to the ocean, before atmospheric deposition and riverine inputs (100–150 Tg N yr−1: Duce et al., 2008; Gruber, 2008). N2 fixation is performed by prokaryotic organisms termed diazotrophs, which include the non-heterocystous filamentous cyanobacterium Trichodesmium (Capone et al., 1997; Carpenter, 1983), heterocystous cyanobacteria living in symbiosis with diatoms (or diatom–diazotroph associations, termed DDAs; Villareal, 1994), unicellular cyanobacteria termed UCYN (subdivided into groups A, B and C based on the nifH gene sequence, (Zehr et al., 1998, 2008; Zehr and Turner, 2001), diverse non-cyanobacterial bacteria (Bombar et al., 2015; Moisander et al., 2014; Riemann et al., 2010), and archaea (Loescher et al., 2014). Although considerable efforts have been made over the past decades to quantify N2 fixation, identify the major players, and assess their biogeographical distribution in relation to environmental drivers, the fate of new N provided by N2 fixation in the ocean and its role in the planktonic food web structure and large-scale biogeochemical fluxes are still poorly understood.
Early studies have reported high dissolved organic N (DON) and ammonia (NH) concentrations during and following Trichodesmium blooms in the Indian Ocean (Devassy et al., 1978, 1979; Glibert and O'Neil, 1999), suggesting that Trichodesmium release part of the recently fixed N2 (hereafter referred to as diazotroph-derived N, DDN) to the dissolved pool, which could subsequently be consumed by the surrounding plankton communities. The first direct release measurements were performed in the early 1990s and showed that Trichodesmium colonies isolated from the tropical Atlantic Ocean release ∼ 50 % of the recently fixed N2 (Glibert and Bronk, 1994). Accumulations of DON and NH have subsequently been confirmed near Trichodesmium blooms in the Pacific (Karl et al., 1992, 1997) and Atlantic (Lenes et al., 2001) oceans, although not systematically (Bonnet et al., 2016a; Hansell and Carlson, 2001), and in senescent Trichodesmium cultures (Mulholland and Capone, 2000), possibly related to the Trichodesmium programmed cell death (PCD, Berman-Frank et al., 2004). This DDN release has been attributed (i) to endogenous processes such as the dissipation of excess electrons linked to an excess of light (Wannicke et al., 2009) or to a means for the filamentous diazotrophs to transfer fixed N from N2-fixing cells to vegetative cells (Mulholland and Capone, 2000) and (ii) to exogenous processes such as viral lysis (Hewson et al., 2004; Ohki, 1999) or “sloppy feeding” by copepods (O'Neil, 1999).
Numerous studies performed in culture (Karl et al., 1992, 1997; Hutchins et al., 2007) and in the field (Benavides et al., 2013b; Konno et al., 2010; Mulholland and Bernhardt, 2005) focused on quantifying this release, and most of them were performed on Trichodesmium and were based on the difference between the measurement of gross N2 fixation (through the acetylene reduction method, Capone, 1993) and net N2 fixation rates (through the 15N2 isotope labelling method, Montoya et al., 1996) (Mulholland et al., 2004a). It was thus shown that the DDN released to the dissolved pool averages ∼ 50 % (10 to 80 %) of the total N2 fixation. The estimates based on this approach were then questioned since the discovery of the methodological underestimation of net 15N2 rates when the 15N2 tracer is injected as a bubble in the incubation bottles (Mohr et al., 2010), leading to a potential overestimation of the DDN release. An alternative approach based on the direct measurement of the 15N enrichment of both the particulate and dissolved pools (Glibert and Bronk, 1994; Slawyk and Raimbault, 1995) after incubation with 15N2 presents the advantage of providing a ratio of particulate N2 fixation vs. DDN release, without being affected by potential underestimation issues. The studies based on this approach reveal that the proportion of DDN released to the dissolved pool ranges from 10 to > 80 % of total N2 fixation measured in the field (Benavides et al., 2013b; Berthelot et al., 2017; Glibert and Bronk, 1994; Konno et al., 2010). The release appears to be higher when Trichodesmium dominates the diazotroph community (Berthelot et al., 2017; Bonnet et al., 2016a; Glibert and Bronk, 1994) than when UCYN dominate (Benavides et al., 2013b; Bonnet et al., 2016b). The DDN release is generally much lower (< 5 %) in monospecific cultures (Benavides et al., 2013a; Berthelot et al., 2015) than in field experiments, suggesting that external factors such as sloppy feeding and viral lysis have a strong influence on the DDN release by diazotrophs in the field.
The DDN released in the surface ocean is potentially available for surrounding planktonic communities, but its fate in the planktonic food web has been poorly quantified, mainly due to methodological locks. Devassy et al. (1979), who reported high DON and NH concentrations near Trichodesmium blooms, were also the first to observe that during the decline of the Trichodesmium blooms, diatom abundances increased, followed by a succession of cladocerans, dinoflagellates, green algae and finally copepods. In the tropical Atlantic Ocean, high abundances of non-diazotrophic phytoplankton have also been observed following Trichodesmium blooms (Mulholland et al., 2004b), and more recently, Chen et al. (2011) showed a positive correlation between abundances of Trichodesmium and diatoms in the Kuroshio. These studies suggest a link between diazotroph blooms and non-diazotrophic organisms. Studies based on size fractionation carried out during a Trichodesmium bloom incubated in the presence of 15N2 report that ∼ 10 % of the fixed N2 by Trichodesmium (recovered in the size fraction > 30 µm) was rapidly transferred to non-diazotrophic organisms (recovered in the < 30 µm fraction; Bryceson and Fay, 1981). Using similar methods, other studies suggested that during Trichodesmium blooms (Mulholland et al., 2004b; Garcia et al., 2007) and Nodularia and Aphanizomenon blooms (Ohlendieck et al., 2000), 5 to 10 % of the DDN is transferred to the picoplankton compartment. However, the methods based on size fractionation do not discriminate the DDN transfer towards the non-diazotroph picoplankton to the potentially active N2 fixation within picoplankton, in particular the UCYN-A, one of the most abundant diazotrophs in our ocean (Luo et al., 2012). This method therefore potentially overestimates the DDN transfer and is not applicable to study the DDN transfer associated with UCYN. Moreover, it is not possible with size fractionation methods to determine which populations (e.g. autotrophic vs. heterotrophic plankton, small vs. large plankton) have benefited the most from this source of new N. The recent use of high-resolution nanometre-scale secondary ion mass spectrometry (nanoSIMS) coupled with 15N2 isotopic labelling and flow cytometry cell sorting (Bonnet et al., 2016a, b; Berthelot et al., 2016) has proven its efficiency in the quantification of the DDN transfer to specific groups of phytoplankton and bacteria. Applying this method during Trichodesmium blooms in the coastal western tropical South Pacific (WTSP), Bonnet et al. (2016a) revealed that after 48 h 13 ± 2 to 48 ± 5 % of the fixed N2 was released to the dissolved pool and 6 ± 1 to 8 ± 2 % of this DDN was transferred to non-diazotrophic plankton, mainly diatoms (45 ± 4 to 61 ± 38 %) and bacteria (22 ± 27 to 38 ± 12 %). A mesocosm experiment performed in the New Caledonian lagoon during a UCYN-C bloom (Bonnet et al., 2016b) revealed that after 48 h 16 ± 6 % of the fixed N2 was released to the dissolved pool and 21 ± 4 % of this DDN was transferred to non-diazotrophic plankton, mainly picoplankton (18 ± 4 %) and diatoms (3 ± 2 %). Finally, a comparative study between Trichodesmium vs. UCYN blooms simulated thanks to culture isolates (Berthelot et al., 2016) revealed that the DDN transfer to non-diazotrophic plankton is twice as high with Trichodesmium as with UCYN. The differences of DDN release and transfer rates observed between the different field experiments and the different diazotrophs suggest that these processes strongly depend on the physiological state of diazotrophs and the environment. However, the transfer of DDN to different groups of plankton from different diazotrophs (Trichodesmium vs. UCYN) in the open ocean, where most global marine N2 fixation takes place, has never been investigated.
Regarding higher trophic levels, the low 15N isotopic signature (δ15N) of zooplankton reveals that N2 fixation can significantly contribute to zooplankton N requirements in high N2 fixation areas (Aberle et al., 2010; Landrum et al., 2011; Loick-Wilde et al., 2012; Mompeán et al., 2013; Montoya et al., 2002; Sommer et al., 2006; Wannicke et al., 2013; Hunt et al., 2016). Few studies report active grazing of Trichodesmium by some specific copepods (Micro- and Macro-setella sp., O'Neil et al., 1996; O'Neil and Roman, 1992). However, Trichodesmium has been shown to be toxic for most of the grazers (Guo and Tester, 1994; Hawser et al., 1992; Hawser and Codd, 1992), and the low δ15N signature found in zooplankton (indicative of DDN consumption) where Trichodesmium thrive most likely originates from indirect transfer mediated by recycling processes (Capone et al., 1994; Capone and Montoya, 2001; Letelier and Karl, 1996) rather than from direct grazing. A recent study based on 15N2 labelling in the coastal WTSP (Hunt et al., 2016) reveals that the DDN is less efficiently transferred to zooplankton when Trichodesmium and DDA dominate the diazotroph community than when UCYN-C dominate, suggesting that the DDN transfer efficiency to zooplankton strongly depends on the diazotroph involved in the N2 fixation. To our knowledge, this has never been investigated so far in the open ocean.
The WTSP Ocean has recently been identified as a hotspot of N2 fixation (Bonnet et al., 2017) and is characterized by trophic and N2 fixation gradients (Moutin et al., 2017), with oligotrophic waters characterized by high N2 fixation rates (631 ± 286 µmol N m−2 d−1) mainly associated with Trichodesmium in the western part, and ultra-oligotrophic waters characterized by low N2 fixation rates (85 ± 79 µmol N m−2 d−1) mainly associated with UCYN in the eastern part (Bonnet et al., 2018; Stenegren et al., 2018). We performed a series of experiments under contrasting situations (when either Trichodesmium or UCYN was dominating the diazotroph community) to study the fate of DDN in the planktonic food web, with the following specific goals: (1) quantify the proportion of DDN released to the dissolved pool relative to total N2 fixation, (2) quantify the DDN transfer to the non-diazotrophic phytoplankton and bacteria, and (3) quantify the DDN transfer to zooplankton.
2.1 Experimental set-up for DDN transfer experiments in phytoplankton and heterotrophic bacteria
This study was carried out during the OUTPACE (Oligotrophic to UlTra oligotrophic PACific Experiment) cruise (https://doi.org/10.17600/15000900), which took place in February–March 2015 (austral summer) onboard R/V L'Atalante. Samples were collected along a ∼ 4000 km west-to-east zonal transect along ∼ 19∘ S starting in New Caledonia and ending in French Polynesia, crossing Melanesian archipelago waters (hereafter referred to as MA waters) around New Caledonia, Vanuatu, and Fiji up to Tonga and South Pacific Gyre waters located at the western boundary of the South Pacific Gyre (hereafter referred to as GY waters) (see Moutin et al., 2017, for details). Three experiments are reported here (hereafter named E1, E2 and E3). Two were performed at stations located in MA waters: station LD A, 19∘12.8′ S–164∘41.3′ E, and station LD B, 18∘14.4′ S–170∘51.5′ W, where Trichodesmium accounted for 95 and 100 % of the total diazotroph community quantified by quantitative PCR (Stenegren et al., 2018). The third experiment was performed in GY waters: station LD C, 18∘25.2′ S–165∘56.4′ W, where UCYN accounted for 82 % of the total diazotroph community (Stenegren et al., 2018).
The experiments were designed according to Bonnet et al. (2016a, b) and Berthelot et al. (2016). For experiments E1 and E2, seawater was collected by the surface underway pumping system at 6 m in depth. For E3, seawater was collected at 55 m in depth using Niskin bottles mounted on a CTD rosette. For all experiments, seawater was collected into eight HCl-washed-sample rinsed (three times) 4.5 L polycarbonate bottles equipped with septum caps; 5 mL of 15N2 gas (98.9 at. % 15N2, Cambridge isotopes) were injected into all bottles using a gas-tight syringe. The purity of the 15N2 Cambridge isotope stocks was previously checked by Dabundo et al. (2014) and more recently by Benavides et al. (2015) and Bonnet et al. (2016b), who concluded that the purity is satisfying (2 × 10−8 mol : mol N of 15N2) and therefore does not alter the results presented below. The bottles were shaken 30 times to facilitate the 15N2 dissolution and, except for the T0 set of bottles (see below), were incubated for 48 h in on-deck incubators covered with blue screening (50 % surface irradiance for E1 and E2 and 15 % surface irradiance for E3) and cooled with circulating surface seawater. At T0 and after incubation, a set of four bottles was collected and subsampled for the following measurements (see below for methods): N2 fixation rates, DDN release, quantification of diazotrophs, heterotrophic bacteria and pico-, nano- and micro-phytoplankton enumeration, organic and inorganic nutrient concentrations, and 15N enrichment on diazotrophic and non-diazotrophic plankton. Unless otherwise stated, each parameter reported below was measured in triplicate.
2.2 Net N2 fixation rates and DDN released to the dissolved pool
N2 fixation rates were measured using the 15N2 isotopic tracer technique (adapted from Montoya et al., 1996), as described in Bonnet et al. (2018).
The DDN released to the dissolved pool in the form of NH and DON during the N2 fixation process was quantified using the three-step diffusion method extensively described in Berthelot et al. (2015) and derived from Slawyk and Raimbault (1995). As the NO pool was negligible, the total dissolved N (TDN) pool was defined as the sum of DON and NH pools (TDN = DON + NH). After incubation with the 15N2 tracer, 300 mL of the filtrate passed through pre-combusted Whatman GF/F filters was collected in 500 mL Duran Schott borosilicate flasks, poisoned with HgCl2 (300 µL, final concentration 20 mg L−1) and stored at 4 ∘C in the dark until analysis. At the end of each step, NH and DON fraction were recovered on acidified pre-combusted Whatman GF/F filters, dried 24 h at 60 ∘C and stored in pre-combusted glass tubes until analysis on an elemental analyser coupled to an isotope ratio mass spectrometer (EA-IRMS, Integra2 Sercon Ltd) as described in Berthelot et al. (2015).
2.3 Inorganic and organic nutrient analyses
NH concentrations were measured fluorimetrically according to Holmes et al. (1999) on a FP-2020 fluorimeter (Jasco, detection limit = 3 nM). NO and nitrite (NO) concentrations were measured using standard colorimetric procedures (Aminot and Kérouel, 2007) on a AA3 AutoAnalyzer (Seal Analytical). DON concentrations were measured by the wet oxidation method according to Pujo-Pay and Raimbault (1994).
2.4 Plankton abundance determination
The abundance of Trichodesmium and UCYN-B was determined microscopically: 2.2 L of each triplicate 15N2-amended 4.5 L bottle was gently filtered onto 2 µm pore size, 25 mm diameter Millipore polycarbonate filters and fixed with paraformaldehyde (2 % final concentration) for 1 h. Trichodesmium were enumerated on the entire surface of the filter at a ×100 magnification with a Zeiss Axio Observer epifluorescence microscope fitted with a green (510–560 nm) excitation filter. The number of cells per trichome was counted on 20 trichomes for each experiment; we counted an average of 85 and 115 cells trichome−1 for E1 and E2, respectively. UCYN-B were counted on 40 fields (1.3 mm2 fields; 0-2800 UCYN-B per field) scanned and analysed with the ImageJ1 software.
Samples for micro-phytoplankton identification and enumeration were collected in each of the triplicate 4.5 L incubated bottles (except for E1, where only one replicate was available) in five 50 mL sterile polypropylene tubes and preserved in an acidic Lugol solution (0.5 % final concentration). Diatoms were enumerated from a 250 mL subsample following the Utermöhl method (Hasle, 1978), using a Nikon TE2000 inverted microscope equipped with phase contrast and a long-distance condenser. Diatoms were identified to the lowest possible taxonomic level in one of the three replicates.
Pico-phytoplankton, nano-phytoplankton and heterotrophic bacteria abundances were determined by flow cytometry. After incubation, 1.8 mL was subsampled from triplicate 4.5 L bottles into cryotubes, fixed with paraformaldehyde (200 µL, 4 % final concentration) for 5 min at room temperature, flash-frozen in liquid N2, and stored at −80 ∘C until analysis on a FACSCalibur (BD Biosciences, San Jose, CA) according to Marie et al. (1999), at the PRECYM flow cytometry platform (https://precym.mio.univ-amu.fr/, last access: 31 December 2017). Phytoplankton communities were clustered as Prochlorococcus spp. cell like, Synechococcus spp. cell like, nano-eukaryotes cell like, pico-eukaryotes cell like, and UCYN-B cell like. Trucount TM (BD Biosciences) beads were used to determine the volume analysed.
2.5 Cell sorting and sampling for nanoSIMS analyses
For flow cytometry cell sorting and subsequent analysis using nanoSIMS, 1 L of one of the 4.5 L bottles was filtered onto 0.2 µm pore size 47 mm polycarbonate filters to preconcentrate the cells and facilitate cell sorting. Filters were placed in a 5 mL cryotube filled with 0.2 µm filtered seawater and PFA (2 % final concentration), and incubated for 1 h at room temperature in the dark. The cryovials were vortexed for ∼ 20 s to detach the cells from the filter and were stored at −80 ∘C until analysis. Cell sorting was performed using a BD InfluxTM Mariner (BD Biosciences) high-speed cell sorter at the PRECYM platform, as described in Bonnet et al. (2016a, Supplement). Planktonic groups (pico-phytoplankton, nano-phytoplankton, bacteria and UCYN-B (for E3 only)) were separated using the same clusters as for the phytoplankton abundance determination. At the issue of the cell sorter, the cells were directly dropped onto a 0.2 µm pore size, 13 mm diameter polycarbonate filter (Millipore) connected to a low-pressure pump in order to concentrate them on a surface as tightly as possible. The filters were stored at −80 ∘C until nanoSIMS analyses.
To recover large phytoplanktonic cells (Trichodesmium and diatoms), 1 L of the same 4.5 L bottle was filtered on 10 µm pore size 25 mm polycarbonate filters. The cells were fixed with PFA (2 % final concentration), incubated 1 h at ambient temperature and stored at −20 ∘C until nanoSIMS analyses.
2.6 NanoSIMS analyses
Just before nanoSIMS analyses, filters were thawed at ambient temperature and sputtered with gold and palladium to ensure conductivity. Analyses were performed on a NanoSIMS N50 (Cameca, Gennevilliers, France) at the French National Ion MicroProbe Facility as previously described in Bonnet et al. (2016a, b) and Berthelot et al. (2016). Briefly, high-density cell areas were retrieved using the nanoSIMS optical camera (Fig. 1f). Samples were pre-sputtered prior to analyses for at least 2 min to remove surface contaminants and increase conductivity with a ∼ 22 pA caesium primary beam. For the analysis, a ∼ 1.2 pA caesium (16 KeV) primary beam focused onto a ∼ 100 nm spot diameter was scanned across a 256 × 256 or 512 × 512 pixel raster (depending on the image size, which ranged from 20 µm × 20 µm to 40 µm × 40 µm) with a counting time of 1 ms per pixel. Negative secondary ions (12C−, 13C−, 12C14N−, 12C15N− and 28Si−) were collected by electron multiplier detectors, and secondary electrons were imaged simultaneously. A total of 20 serial quantitative secondary ion images were generated to create the final image. Individual cells were easily identified in nanoSIMS secondary electron, 12C−, 12C14N− and 28Si− images that were used to define regions of interest (ROIs) around individual cells. A total of ∼ 1500 ROIs were analysed. For each ROI, the 15N enrichment was calculated; ∼ 80 cells on average were analysed for each plankton group and for each experiment (see Table S1 in the Supplement).
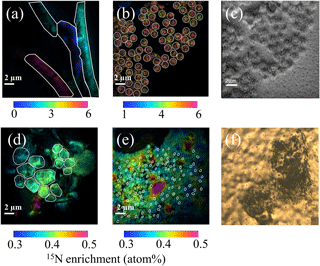
Figure 1NanoSIMS images showing the 15N enrichment (a, b, d, e) after 48 h of incubation in the presence of 15N2 for Trichodesmium (a), UCYN-B (b), nano-eukaryotes (d) and Synechococcus (e). The ROIs are represented with white lines. NanoSIMS images showing the secondary electron channel of UCYN (c) and optical camera image of Prochlorococcus spotted on the filter before NanoSIMS analyses (f).
2.7 Cell-specific N content and DDN transfer calculations
To determine cell-specific N contents, cell sizes of Trichodesmium and dominant diatoms were directly measured on each sample collected for microscopy (see Sect. 2.4). For Trichodesmium, cell length and width were measured on 25 to 50 cells per sample at ×400 magnification with a Zeiss Axio Observer epifluorescence microscope. For diatoms, the cell cross section and apical and transapical dimensions were measured on at least 20 cells using a Nikon TE2000 inverted microscope equipped with phase contrast and a long-distance condenser. For UCYN-B, cell diameters were directly measured on the nanoSIMS images and further confirmed on microscopic images. The biovolumes (BVs) of Trichodesmium, UCYN-B and diatoms were estimated following the geometric model of each cell type (Sun and Liu, 2003). The cellular carbon (C) contents were determined by using the relation between BV and C content according to Verity et al. (1992) for Trichodesmium and UCYN-B, and according to Eppley et al. (1970) and Smayda (1978) for diatoms. The N content was calculated based on C : N ratios of 6 for Trichodesmium (Carpenter et al., 2004) and 5 for UCYN-B (Dekaezemacker and Bonnet, 2011; Knapp et al., 2012), and a typical Redfield ratio of 6.6 for diatoms.
For Synechococcus and Prochlorococcus we used the C content reported in Buitenhuis et al. (2012) (255 and 36 fg C cell−1, respectively). For nano-eukaryotes we used the C content reported in Grégori et al. (2001), and converted it into N content according to the C : N Redfield ratio of 6.6 (leading to 3.2, 0.45 and 219 fmol N cell−1 for Synechococcus, Prochlorococcus and nano-eukaryotes). For bacteria, an average N content of 2.1 fg N cell−1 (Fukuda et al., 1998) was used. For the pico-eukaryotes, the cellular N content of 9.2 ± 2.9 fmol N cell−1 was used as reported in Grégori et al. (2001).
The cell-specific N2 fixation rates and DDN transfer rates (in nmol N L−1 48 h−1) to non-diazotrophic phytoplankton were calculated for each plankton group analysed as follows:
where 15Nex (atom %) is the excess 15N enrichment of the individual cells measured by nanoSIMS after 48 h of incubation relative to the time zero value, Nsr (atom %) is the excess 15N enrichment of the source pool (N2) in the experimental bottles determined by MIMS, Ncon is the cellular N content (in nmol N cell−1) of each cell and A is the abundance of the specific plankton group (in cell L−1). Incertitude was estimated on each variable and the final incertitude was estimated using the propagation error rule.
2.8 Experimental set-up for DDN transfer experiments in zooplankton
The DDN transfer to zooplankton was measured in four experiments performed at the same stations where the E1, E2 and E3 experiments were performed (hereafter named Zoo-1, Zoo-3 and Zoo-4) plus an additional station (Zoo-2) located between LDA and LDB (SD9, 20∘57′ S–178∘39′ E). Trichodesmium dominated the diazotroph community in the Zoo-1, Zoo-2, and Zoo-3 experiments, and UCYN-B dominated the Zoo-4 experiment. The experiments consisted in incubations of freshly collected zooplankton in the presence of the natural planktonic assemblage pre-labelled with 15N2 as a food source. In parallel to the experiments described above, six additional HCl-washed-sample rinsed (three times) 1 L polycarbonate bottles equipped with septum caps were collected by the underway pumping system at 6 m in depth for Zoo-1, Zoo-2 and Zoo-3 and with Niskin bottles at 55 m in depth for Zoo-4. All bottles were amended with 1 mL of 15N2 (98.9 at. % 15N, Cambridge isotopes). The bottles were shaken 30 times to facilitate the 15N2 dissolution and incubated in on-deck incubators for 24–36 h as described above.
The incubation was stopped by filtering the bottles on 0.2 µm pore size 47 mm membrane filters in such a way that the 15N enrichment of the food source provided to zooplankton (hereafter referred to as 15N pre-labelled plankton) does not increase during the course of the experiment. For each experiment, the initial 15N enrichment of the 15N pre-labelled plankton was analysed in triplicate by EA-IRMS. Plankton was then re-suspended in six 1 L bottles filled with 0.2 µm filtered surface seawater collected at the same station. Meanwhile, zooplankton was collected using repeated net tows (120 mesh size) before dawn. Animals were recovered on a 120 µm sieve and placed into 4.5 L polycarbonate bottles filled with 0.2 µm filtered surface seawater in the dark for at least 6 h in order to allow them to empty their guts. Living animals were visually identified and the individuals belonging to the genus Clausocalanus, which largely dominated the zooplankton community at all stations (Carlotti et al., 2018), were handpicked and 12 animals were dispatched into each of the three 1 L bottles containing the 15N pre-labelled plankton before being incubated in on-deck incubators for 24 h as described above. The three other bottles were immediately filtered after the introduction of animals, firstly through a 120 µm mesh in order to recover the animals and secondly through precombusted (4 h, 450 ∘C) GF/F filters, which were used to quantify the isotopic signature of the 15N pre-labelled plankton at the beginning of the experiment, together with the initial NH concentrations in the incubation bottles. After 24 h, the triplicate bottles containing the mixture of 15N pre-labelled plankton and zooplankton were filtered in the same way. In addition, the filtrate was recovered as described in Sect. 2.2 in order the measure the NH concentration and the 15N enrichment in the dissolved pool by using the two-step diffusion method. The recovered animals were placed on GF/F filters, which were analysed by EA-IRMS as described above in Sect. 2.2.
Dupouy et al. (2018)Bock et al. (2018)Stenegren et al. (2018)3.1 Description of the biogeochemical context at the studied stations
Regarding the chlorophyll a and nutrient concentrations, the four studied stations were divided into two main sub-regions (Table 1): (i) stations of E1/Zoo-1, Zoo-2 and E2/Zoo3 were located in the oligotrophic MA waters characterized by surface chlorophyll a concentrations of 0.159–0.377 µg L−1 and NO and PO concentrations below 50 nmol L−1, (ii) station E3/Zoo-4 located in the ultra-oligotrophic GY waters presenting lower chlorophyll a concentrations than in MA waters (0.053 µg L−1), NO concentration below 50 nmol L−1, and PO concentration ∼ 110 nmol L−1.
Trichodesmium dominated the diazotroph community (95–100 % of total nifH gene copies detected by qPCR, Stenegren et al., 2018) at 6 m in depth in the MA waters (E1/Zoo-1, Zoo-2 and E2/Zoo3), while UCYN-B dominated (82 % of nifH gene copies) at 55 m in depth in the GY waters (E3/Zoo-4 experiment). MA waters were characterized by a higher abundance of pico-phytoplankton (Synechococcus and Prochlorococcus) and bacteria abundances (125–200 and 271–424 × 1011 cells m−2, respectively) than GY waters (∼ 110 and ∼ 290 × 1011 cells m−2, respectively; Bock et al., 2018).
3.2 N2 fixation and DDN released to the dissolved pool
Net N2 fixation rates were 20.1 ± 13.4, 49.9 ± 2.4 and 3.2 ± 0.3 nmol N L−1 48 h−1 for the E1, E2 and E3 experiments, respectively (Fig. 2). The DDN released to the dissolved pool (the sum of DON and NH) was 14 ± 9 and 8 ± 2 nmol N L−1 48 h−1 in E1 and E2, and was below quantification limits in E3. Considering gross N2 fixation as the sum of net N2 fixation and DDN release rates (Mulholland et al., 2004a), the DDN released to the dissolved pool accounted for 40 ± 27 and 14 ± 4 % of gross N2 fixation in E1 and E2, respectively (Fig. 4). DON accounted for the major part of the 15N released and accounted for ∼ 93 and ∼ 96 % of the total N release in E1 and E2, respectively.
3.3 Cell-specific 15N enrichment and DDN transfer to non-diazotrophic plankton
NanoSIMS analyses performed on individual trichomes (E1 and E2) and UCYN-B cells (E3) revealed significant (p< 0.05) 15N enrichment after 48 h of incubation (Fig. 1a, b) compared to T0 samples (0.371 ± 0.005 at. %, n=5), indicating active N2 fixation during the experiments: 1.946 ± 0.837 at. % (n=32) in E1, 1.523 ± 0.477 at. % (n=25) in E2 and 4.707 ± 0.210 at. % (n=192) in E3 (Fig. 3). Cell-specific N2 fixation rates of Trichodesmium were 252.7 ± 50.5 fmol N cell−1 48 h−1 in E1 and 341.5 ± 68.3 fmol N cell−1 48 h−1 in E2, and cell-specific rates of UCYN-B were 18.6 ± 3.8 fmol N cell-1 48 h−1 in E3.
NanoSIMS analyses performed on non-diazotrophic plankton (diatoms and cell-sorted Synechococcus (Fig. 1d), Prochlorococcus, bacteria, and pico- and nano-eukaryotes (Fig. 1e)) also revealed significant 15N enrichment as compared to T0 values (p< 0.05) (Fig. 3). The 15N enrichment of the non-diazotrophic plankton (all groups pooled together) was not statistically different (p> 0.05) between E1 and E2. However, it was significantly lower (p< 0.05) in E3 compared to E1 and E2.
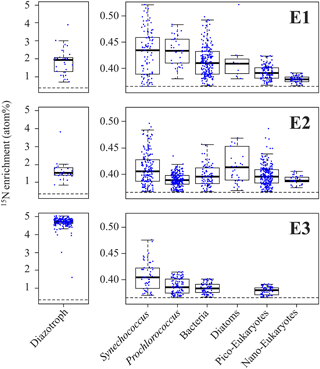
Figure 3Left panels: boxplot of the 15N enrichment measured in diazotrophs (Trichodesmium for E1 and E2, and UCYN-B for E3). Right panels: 15N enrichment measured in non-diazotrophic plankton: Synechococcus, Prochlorococcus, bacteria, diatoms, pico-eukaryotes and nano-eukaryotes for each experiment. The black dotted line indicates the natural isotopic enrichment.
Over the 48 h of the experiment, 10 ± 2 % of the total DDN was transferred to non-diazotrophic plankton in E1, 7 ± 1 % in E2, and 15 ± 3 % in E3 (Fig. 4). DDN was mainly transferred to pico-cyanobacteria (Synechococcus and Prochlorococcus), accounting for 73 ± 15, 68 ± 14 and 65 ± 13 % of the total transfer into non-diazotrophs in E1, E2 and E3, respectively (Fig. 4). The transfer into heterotrophic bacteria accounted for 25 ± 5, 23 ± 5 and 34 ± 7 % of the total transfer, in E1, E2 and E3, respectively. Lastly, 50 ± 40, 79 ± 4 and 85 ± 9 % of the newly fixed 15N2 remained in the pool of diazotrophs, for E1, E2 and E3, respectively. This pool takes into account the major group of diazotrophs detected and analysed by nanoSIMS at each station (Trichodesmium or UCYN-B), other potential diazotrophs that have not been targeted by qPCR (such as non-cyanobacterial diazotrophs), and other groups of non-diazotrophic plankton to which 15N2 was transferred but that were not analysed by nanoSIMS (due to their very low abundance).
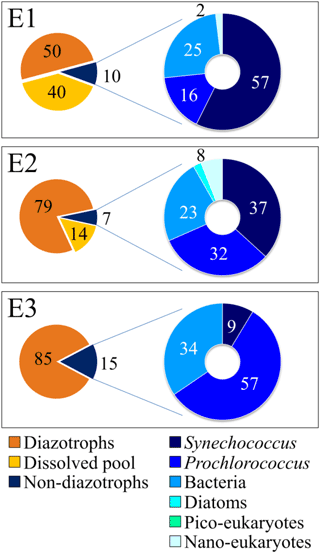
Figure 4DDN fate after 48 h for each experiment. Left pie charts: orange: DDN remained in diazotrophs (orange); yellow: DDN released to the dissolved pool; dark blue: DDN transferred to non-diazotrophic plankton. Right pie charts, from dark blue to light blue: relative DDN transferred to Synechococcus, Prochlorococcus, bacteria, diatoms, pico-eukaryotes and nano-eukaryotes in E1 (top), E2 (middle) and E3 (bottom pie chart).
3.4 DDN transfer to zooplankton
Before incubation with zooplankton, the isotopic enrichment of the 15N pre-labelled plankton was not significantly different in experiments Zoo-1, Zoo-2 and Zoo-3 (dominated by Trichodesmium) averaging 1.035 ± 0.091 at. % (n=9). The isotopic enrichment was lower in the Zoo-4 experiment (dominated by UCYN-B), averaging 0.385 ± 0.005 at. % (n=3). After 24 h of incubation with zooplankton, the 15N enrichment of the 15N pre-labelled plankton decreased to 0.431 ± 0.014 at. % on average in Zoo-1, Zoo-2 and Zoo-3, and to 0.372 ± 0.010 at. % in Zoo-4. Meanwhile, the 15N enrichment of zooplankton increased as compared to T0 values (0.383 at. % on average) and reached 0.482, 0.376, 0.513 and 0.368 at. % on average in Zoo-1, Zoo-2, Zoo-3 and Zoo-4, respectively. As the 15N enrichment of the initial source food (15N pre-labelled plankton) was different between the four stations/experiments, and in order to compare the results obtained among experiments, we normalized the values as the percentage of initial amount of 15N atoms in excess in the 15N pre-labelled plankton transferred to the different compartments (i.e. conserved in the 15N pre-labelled plankton pool, transferred to the zooplankton pool and to the NH pool) at the end of the incubation. In the experiments where Trichodesmium dominated the diazotroph community (Zoo-1, Zoo-2, and Zoo-3), 19 ± 7 to 48 ± 21 % of the initial DD15N remained in the phytoplankton pool, 5 ± 5 to 9 ± 13 % was transferred to the zooplankton and 0.4 ± 0.3 to 7 ± 3 % was transferred to the NH pool (Fig. 5). In Zoo-4 (where UCYN dominated the diazotroph community, Table 1), a greater proportion of DD15N was conserved in the phytoplankton (76 ± 34 %), but a greater transfer to the zooplankton was also observed (28 ± 8 %, Fig. 5). The recovery of the initial DD15N was comprised between 29 % and 100 %, suggesting that the remaining fraction was released to the DON pool. Interestingly, the recovery of the DD15N was in surplus in the Zoo-4 experiment (112.5 ± 8.5 %), suggesting that the DD15N transfer in the DON pool is close to zero.
4.1 DDN release to the dissolved pool
The quantity and quality of N released by diazotrophs to the dissolved pool during N2 fixation potentially play a key role in shaping the planktonic and microbial food webs. In this study, Trichodesmium released 14 ± 4 to 40 ± 57 % of the newly fixed N into the dissolved pool, which is in agreement with values reported in the literature for field studies (Mulholland, 2007; Bonnet et al., 2016a). DON accounted for ∼ 95 % of the DDN released by Trichodesmium (Fig. 2), according with contributions measured in culture (80–90 %; Berthelot et al., 2015) and in the field (Berthelot et al., 2016). The low contribution of NH to the DDN release does not mean that it was not released, but is likely the result of immediate consumption by surrounding plankton, which shows a great affinity for NH. Similarly, part of the DON released by Trichodesmium was probably taken up by heterotrophic and mixotrophic plankton (Bronk et al., 2007), but a significant fraction was likely refractory (not easily available for organisms), leading to the observed accumulation in the dissolved pool. If not refractory, the DON would likely have been immediately assimilated as the regions where these experiments were performed are strongly limited by N availability (Van Wambeke et al., 2008, 2018; Bonnet et al., 2008).
In the E1 experiment, we noticed a large variability of N2 fixation and DDN release rates among the three replicates, which explains the high standard deviations (Fig. 2): two replicates exhibited net N2 fixation rates ∼ 25–30 nmol N L−1 48 h−1 and DDN release rates ∼ 7–10 nmol N L−1 48 h−1, whereas in the third replicate, the DDN release (∼ 24 nmol N L−1 48 h−1) exceeded net N2 fixation rates (5 nmol N L−1 48 h−1). This can be attributed to the decline of Trichodesmium in this replicate as we counted much more degraded trichomes in the third replicate. This suggests that decaying Trichodesmium release DDN more efficiently than healthy Trichodesmium, which has already been observed by Bonnet et al. (2016a). This may also explain why the DDN transfer to non-diazotrophic plankton was slightly higher in E1 (10 ± 2 %) than in E2 (7 ± 1 %), despite both stations being dominated by Trichodesmium.
Conversely to E1 and E2, the DDN released by UCYN-B (E3) was not quantifiable in our study. However, significant DDN transfer into non-diazotrophic plankton was detected (15 ± 3 % of the total fixed N, Fig. 4), suggesting that the DDN released to the dissolved pool is likely immediately transferred to surrounding communities. To our knowledge, this is the first report of DDN release in the field in the presence of a diazotroph community dominated by UCYN-B. Bonnet et al. (2016b) report low release from UCYN-C in coastal waters of the WTSP (16 ± 6 % of total N2 fixation) compared to Trichodesmium (13 ± 2 to 48 ± 5 %; Bonnet et al., 2016b). This seems to indicate that the DDN from UCYN is generally lower than the DDN from Trichodesmium. Several hypotheses may explain the differences observed between Trichodesmium and UCYN. DDN compounds released by UCYN may be more bio-available than the DDN released by Trichodesmium, limiting its accumulation. The lack of accumulation in E3 could also be due to the more severe N limitation of planktonic communities in the ultra-oligotrophic waters as compared to MA waters (Van Wambeke et al., 2018), and to the nature of the resident community. Prochlorococcus was dominating the planktonic community at LD C (E3) and is known to have a high affinity with its small surface to volume ratio (Partensky et al., 1999). PCD causing Trichodesmium bloom demise can also be involved in the relatively high DDN release and accumulation during Trichodesmium-dominated experiments (Bar-Zeev et al., 2013). Exogenous factors, such as viral lyses (Fuhrman, 1999) and sloppy feeding (O'Neil and Roman, 1992; Vincent et al., 2007), are also suspected to enhance the DDN release. These factors were found to exert a higher pressure in the MA waters where Trichodesmium dominated compared to ultra-oligotrophic waters (Bock et al., 2018), where UCYN-B dominated. Finally, part of the discrepancy might be due to a methodological artefact: different sampling procedures between E1 and E2 (pump) and E3 (Niskin bottles) as the pump is suspected to induce mechanical stress to the cells which may have potentially affected the DDN release.
The DDN release plays a key role in the transfer of N from diazotrophs to the surrounding non-diazotrophs, only it is not a good indicator of the DDN transfer efficiency as we observed that DDN transfer to non-diazotrophs was higher when the release was low (E3) than when it was high (E1 and E2). This has already been observed in coastal waters of the WTSP by Berthelot et al. (2016).
4.2 DDN transfer efficiency and pathways in the WTSP
Here we report for the first time data on the transfer of DDN to the planktonic food web under contrasting diazotroph community composition in the open ocean. We reveal that 7 ± 1 to 15 ± 3 % of the DDN is transferred to the non-diazotrophic plankton (Fig. 4) at short timescales (48 h), which is of the same order of magnitude as the transfer (∼ 10 %) reported in coastal waters of the WTSP (Bonnet et al., 2016a; Berthelot et al., 2016). In terms of efficiency, despite UCYN-B fix at lower rates compared to Trichodesmium, the DDN originating from UCYN-B is more efficiently transferred to non-diazotrophic plankton (15 ± 3 % of total fixed N in the E3 experiment, Fig. 4) compared to the DDN originating from Trichodesmium (10 ± 2 and 7 ± 1 % in the E1 and E2 experiments, respectively). This results is in accordance with the fact that we did not detect any accumulation of 15N-labelled N forms in the dissolved pool (see section above).
Several studies have proven that a fraction of the DDN release is transferred to surrounding non-diazotrophic plankton, and one of them (Bonnet et al., 2016a) concludes that diatoms are the major beneficiaries of the DDN originating from Trichodesmium and develop extensively during/after Trichodesmium blooms in the coastal WTSP ocean. Despite Trichodesmium being rarely recovered in sediment traps (Chen et al., 2003; Walsby, 1992), these authors hypothesize a tight coupling between Trichodesmium blooms and export of organic matter as diatoms are efficient exporters of organic carbon to depth (Nelson et al., 1995). In contrast, in the present study, > 90 % of the DDN was transferred to picoplankton (Synechococcus, Prochlorococcus and bacteria), whatever the station studied (Fig. 4). The cyanobacteria Synechococcus and Prochlorococcus were the primary beneficiaries (73 ± 15, 68 ± 14 and 65 ± 13 % of the DDN transfer, Fig. 4), which is consistent with Bonnet et al. (2018), who observed a positive correlation between N2 fixation rates and the abundance of Synechococcus and Prochlorococcus in the WTSP. We attributed this difference between the present study and the Bonnet et al. (2016a) study to the phytoplanktonic populations present in ambient waters at the time of the experiments. In the Bonnet et al. (2016a) study, diatoms were accounting for ∼ 30 % of the non-diazotrophic phytoplankton biomass at T0, whereas diatoms were scarce (1 % of the non-diazotrophic phytoplankton biomass) in our offshore experiments, i.e. too low to show a significant increase in 48 h, even if they benefited from the DDN. However, in E2 (LD B), the diatom abundances were the highest of the three experiments (Leblanc et al., 2018) and the bloom at this station was mainly composed of diatoms and Trichodesmium, suggesting that Trichodesmium contributed to sustain this bloom.
In the E1 and E2 experiments, where Trichodesmium was the dominant diazotroph (Stenegren et al., 2018), the DDN was preferentially transferred to Synechococcus, while it was preferentially transferred to Prochlorococcus in E3 where UCYN-B was the dominant diazotroph (Stenegren et al., 2018). This suggests a possible coupling between Synechococcus and Trichodesmium as ever mentioned by Campbell et al. (2005), who report higher Synechococcus abundances inside Trichodesmium blooms compared to surrounding waters, while it is not the case for other plankton groups. This difference may also be linked with the communities present at the time of the experiments: Prochlorococcus accounted for ∼ 65 % of pico-phytoplankton in E1 and E2, while it accounted for ∼ 80 % in GY in E3. While the transfer of DDN to Prochlorococcus and Synechococcus together was roughly equivalent for E1 and E2 (∼ 70 %, Fig. 4), Synechococcus abundances increased by 150 % in E1, and Prochlorococcus increased by 12 % during the time course of the experiment, while none of the populations increased in abundance in E2 (Fig. S1 in the Supplement). This result is in agreement with Bock et al. (2018), who report an increase of the grazing pressure with the decrease of the oligotrophic degree as E1 was performed in more oligotrophic waters than E2.
Besides Prochlorococcus and Synechococcus, heterotrophic bacteria were the second beneficiaries of the DDN, especially when the diazotroph community was dominated by UCYN-B (34 ± 7 % of the DDN transfer, Fig. 4). In this experiment, bacteria abundances increased by 70 % on average (Fig. S1), which is in agreement with Bonnet et al. (2016a, b) and Berthelot et al. (2016), who reported similar bacterial increases in the coastal WTSP. When Trichodesmium was the dominant diazotroph, 23–25 % was transferred to bacteria, whose abundance increased by 135 and 15 % in E1 and E2, consistent with Sheridan et al. (2002), who reported higher bacterial abundances in Trichodesmium blooms than in surrounding waters. The significant DDN transfer from Trichodesmium to bacteria concurs with Trichodesmium and bacteria association that has been largely highlighted in the last decades (Hmelo et al., 2012; Paerl et al., 1989; Sheridan et al., 2002). That is in accordance with Van Wambeke et al. (2018), who report that N2 fixation fuels 3–35 % of bacterial production in MA waters. Then, we could not discriminate the DDN transfer to pico- and nano-eukaryotes, but for diatoms, the DDN transfer represented a low contribution to the overall transfer into non-diazotrophs in this region of the open ocean.
4.3 Transfer of DDN to zooplankton
Regarding higher trophic levels, the experiments performed here show that the DDN transfer to the major group of zooplankton present in this ecosystem (the copepod Clausocalanus) was less efficient (Fig. 5) when the diazotroph community was dominated by Trichodesmium (∼ 5–9 %) than when it was dominated by UCYN-B (∼ 28 %). This result is consistent with a previous study based on analogous 15N2 labelling method in coastal waters of the WTSP (Hunt et al., 2016), which also report a higher DDN transfer efficiency in the presence of UCYN. Regarding the DDN transfer from UCYN-B, although the transfer experiments to phytoplankton and bacteria (E3) and zooplankton (Zoo-4) were not performed in the same incubation bottles, they consistently report lower 15N enrichments in all the studied pools as compared to the experiments performed when Trichodesmium dominated, but in fine, the DDN transfer efficiency was more important in the presence of UCYN. We observed that a larger fraction of DDN was conserved in the UCYN-B pool than in the Trichodesmium pool, and a larger part of the DDN was missing (likely associated with the DON pool) with Trichodesmium than with UCYN-B (Fig. 5). These observations are consistent with the transfer experiments E1, E2, and E3 which show that Trichodesmium released more DDN in the dissolve pool (DON + NH) than UCYN-B. The DDN released in the NH pool did not presented significant differences between Trichodesmium (Zoo-1, Zoo-2 and Zoo-3) and UCYN-B (Zoo-4), and in all experiments, the DDN contribution was low in the NH pool, as it was immediately assimilated by surrounding organisms as explained in Sect. 4.1. We suggest that the DDN transfer was higher with UCYN than with Trichodesmium since the UCYN-B can be directly grazed due to their small size (2–3 µm) as mentioned in Hunt et al. (2016), who revealed high UCYN abundances in the copepod guts based on qPCR data, while less Trichodesmium was measured. This pleads for a direct transfer of DDN from UCYN-B to zooplankton and an indirect transfer from Trichodesmium through non-diazotrophs. At the ecosystem level, even if the DDN transfer efficiency (∼ 15 %) to zooplankton from UCYN-B is higher than the one of Trichodesmium, the ultimate quantity of DDN transferred to secondary producers is higher when Trichodesmium dominates, as cell-specific N2 fixation rates of Trichodesmium (∼ 250–340 fmol N cell−1 48 h−1) are far higher than those of UCYN-B (∼ 19 fmol N cell−1 48 h−1). This result is in agreement with Carlotti et al. (2018) results based on 15N isotopic data showing that ∼ 50–95 and ∼ 10–40 % of the zooplankton N content originates from N2 fixation in MA and GY waters, respectively. Finally, the DDN transferred to zooplankton, either directly or indirectly, may be released in the dissolved pool as NH, providing additional NH from DDN in the environment that is likely assimilated by organisms in N-depleted waters. Thus, zooplankton N release appears as another DDN transfer pathway to the microbial communities in the WTSP. Zooplankton can contribute to organic matter export by production of sinking fecal pellets, active transport to depth and carcass export. These processes are increasingly recognized as important vectors of organic matter export, and the magnitudes of their contributions to organic matter export are highly dependent on regionally variable plankton community structure (Steinberg and Landry, 2017). In the WTSP, where N2 fixation sustains most of the new primary production (Caffin et al., 2018) and an important fraction of the DDN is transferred to zooplankton, it might play a key role in the export production and hence the CO2 sink which is the WTSP.
N2 fixation acts as a natural N fertilizer in the ocean, releasing DDN in the dissolved pool, which is available for surrounding marine organisms. To our knowledge, this study provides the first quantification of DDN transfer to phytoplankton, bacteria and zooplankton communities in open ocean waters. The main interest of this study was to compare DDN transfer and release under contrasting N2 fixation activity and diversity.
Here, we reveal that Trichodesmium released more DDN than UCYN-B, but a significant part of the DDN released by Trichodesmium accumulated in the dissolved pool, while the DDN released by UCYN-B immediately assimilated by the surrounding plankton communities. The DDN transfer efficiency to non-diazotrophic plankton was higher when UCYN-B dominated the diazotroph community than when Trichodesmium dominated. In the open ocean, most N2 fixation is performed by Trichodesmium (Capone et al., 1997; Luo et al., 2012); thus, on a global scale most of the DDN transfer can be attributed to Trichodesmium. The regions where UCYN are the dominant diazotrophs generally present lower N2 fixation rates than the ones where Trichodesmium dominates, but UCYN provide a continuous source of DDN to surrounding plankton communities. The DDN was transferred to pico-plankton, which dominated the WTSP, suggesting that N2 fixation fuelled the growth of biomass in the N-depleted environment. This is consistent with Caffin et al. (2018), who revealed that N2 fixation provides > 90 % of the new N to the photic layer of the WTSP. On a larger-scale view, the simulation performed by Dutheil et al. (2018) predicts that diazotrophs will support a large part of primary production (∼ 15 %) in low nutrient low chlorophyll regions of the Pacific Ocean, comprising the WTSP.
Overall, this study indicates that N2 fixation plays a key role in the marine biomass production, the structure of the planktonic food web, and finally the export of organic matter towards the deep ocean. The DDN can be exported to the deep ocean by different pathways: (i) the direct of export of diazotrophs, (ii) the export of non-diazotrophs which benefited from the DDN, and (iii) the export of zooplankton which benefited from the DDN. The direct export of diazotrophs accounted for ∼ 30 % of total C export at LD A (E1), 5 % at LD B (E2) and < 0.1 % at LD C (E3) (Caffin et al., 2018). Using a δ15N budget, Knapp et al. (2018) found that 50–80 % of exported material was sustained by N2 fixation (this includes both direct and indirect export of DDN). Thus, N2 fixation has ineluctably a key role in the biological carbon pump, as mentioned in Moutin et al. (2018), who reveal a significant biological “soft tissue” carbon pump in the MA waters almost exclusively sustained by N2 fixation, and acting as a net sink for of atmospheric CO2 in the WTSP.
All data and metadata are available at the French INSU/CNRS LEFE CYBER database (scientific coordinator: Hervé Claustre; data manager and webmaster: Catherine Schmechtig) at the following web address: http://www.obs-vlfr.fr/proof/php/outpace/outpace.php (INSU/CNRS LEFE CYBER, 2017).
The supplement related to this article is available online at: https://doi.org/10.5194/bg-15-3795-2018-supplement.
The authors declare that they have no conflict of interest.
This article is part of the special issue “Interactions between planktonic organisms and biogeochemical cycles across trophic and N2 fixation gradients in the western tropical South Pacific Ocean: a multidisciplinary approach (OUTPACE experiment)”. It is not associated with a conference.
This is a contribution of the OUTPACE (Oligotrophy from Ultra-oligoTrophy
PACific Experiment) project (https://outpace.mio.univ-amu.fr/, last access: 31 December 2017) funded by the
French research national agency (ANR-14-CE01-0007-01), the LEFE-CyBER program
(CNRS-INSU), IRD, the GOPS program and the CNES (BC T23, ZBC 4500048836). The
OUTPACE cruise (https://doi.org/10.17600/15000900) was managed by the MIO
(OSU Institut Pytheas, AMU) from Marseille (France) and received funding from
European FEDER Fund under project 1166-39417. The authors thank the crew of
the R/V L'Atalante for outstanding shipboard operation. Gilles Rougier and
Marc Picheral are warmly thanked for their efficient help in CTD rosette
management and data processing, as is Catherine Schmechtig for the LEFE CYBER
database management. The satellite-derived data of sea surface temperature,
chl a concentration and current have been provided by CLS in the framework of
the CNES funding; we warmly thank Marie Isabelle Pujol and Gillaume Taburet
for their support in providing these data. We acknowledge NOAA, and in
particular Rick Lumpkin, for providing the SVP drifter.
Edited by: Douglas G. Capone
Reviewed by: Carolin Löscher and one anonymous referee
Aberle, N., Hansen, T., Boettger-Schnack, R., Burmeister, A., Post, A. F., and Sommer, U.: Differential routing of “new” nitrogen toward higher trophic levels within the marine food web of the Gulf of Aqaba, Northern Red Sea, Mar. Biol., 157, 157–169, https://doi.org/10.1007/s00227-009-1306-y, 2010. a
Aminot, A. and Kérouel, R.: Dosage automatique des nutriments dans les eaux marines: méthodes en flux continu, Editions Quae, 2007. a
Bar-Zeev, E., Avishay, I., Bidle, K. D., and Berman-Frank, I.: Programmed cell death in the marine cyanobacterium Trichodesmium mediates carbon and nitrogen export, ISME J., 7, 2340–2348, https://doi.org/10.1038/ismej.2013.121, 2013. a
Benavides, M., Agawin, N., Arístegui, J., Peene, J., and Stal, L.: Dissolved organic nitrogen and carbon release by a marine unicellular diazotrophic cyanobacterium, Aquat. Microb. Ecol., 69, 69–80, https://doi.org/10.3354/ame01621, 2013a. a
Benavides, M., Bronk, D. A., Agawin, N. S. R., Pérez-Hernández, M. D., Hernández-Guerra, A., and Arístegui, J.: Longitudinal variability of size-fractionated N2 fixation and DON release rates along 24.5∘N in the subtropical North Atlantic, J. Geophys. Res., 118, 3406–3415, 2013b. a, b, c
Benavides, M., Moisander, P. H., Berthelot, H., Dittmar, T., and Grosso, O.: Mesopelagic N2 fixation related to organic matter composition in the Solomon and Bismarck Seas (Southwest Pacific), PLoS One, 10, e0143775, https://doi.org/10.1371/journal.pone.0143775, 2015. a
Berman-Frank, I., Bidle, K. D., Haramaty, L., and Falkowski, P. G.: The demise of the marine cyanobacterium, Trichodemsium spp., via an autocatalyzed cell death pathway, Limnol. Oceanogr., 49, 997–1005, 2004. a
Berthelot, H., Bonnet, S., Camps, M., Grosso, O., and Moutin, T.: Assessment of the dinitrogen released as ammonium and dissolved organic nitrogen by unicellular and filamentous marine diazotrophic cyanobacteria grown in culture, Front. Mar. Sci., 2, 1–14, https://doi.org/10.3389/fmars.2015.00080, 2015. a, b, c, d
Berthelot, H., Bonnet, S., Grosso, O., Cornet, V., and Barani, A.: Transfer of diazotroph-derived nitrogen towards non-diazotrophic planktonic communities: a comparative study between Trichodesmium erythraeum, Crocosphaera watsonii and Cyanothece sp., Biogeosciences, 13, 4005–4021, https://doi.org/10.5194/bg-13-4005-2016, 2016. a, b, c, d, e, f, g, h
Berthelot, H., Benavides, M., Moisander, P. H., Grosso, O., and Bonnet, S.: High-nitrogen fixation rates in the particulate and dissolved pools in the Western Tropical Pacific (Solomon and Bismarck Seas), Geophys. Res. Lett., 2, 1–10, https://doi.org/10.1002/2017GL073856, 2017. a, b
Bock, N., Van Wambeke, F., Dion, M., and Duhamel, S.: Microbial community structure in the Western Tropical South Pacific, Biogeosciences Discuss., https://doi.org/10.5194/bg-2017-562, in review, 2018. a, b, c, d
Bombar, D., Taylor, C. D., Wilson, S. T., Robidart, J. C., Rabines, A., Turk-Kubo, K. A., Kemp, J. N., Karl, D. M., and Zehr, J. P.: Measurements of nitrogen fixation in the oligotrophic North Pacific Subtropical Gyre using a free-drifting submersible incubation device, J. Plankton Res., 37, 727–739, https://doi.org/10.1093/plankt/fbv049, 2015. a
Bonnet, S., Guieu, C., Bruyant, F., Prášil, O., Van Wambeke, F., Raimbault, P., Moutin, T., Grob, C., Gorbunov, M. Y., Zehr, J. P., Masquelier, S. M., Garczarek, L., and Claustre, H.: Nutrient limitation of primary productivity in the Southeast Pacific (BIOSOPE cruise), Biogeosciences, 5, 215–225, https://doi.org/10.5194/bg-5-215-2008, 2008. a
Bonnet, S., Biegala, I. C., Dutrieux, P., Slemons, L. O., and Capone, D. G.: Nitrogen fixation in the western equatorial Pacific: Rates, diazotrophic cyanobacterial size class distribution, and biogeochemical significance, Global Biogeochem. Cy., 23, 1–13, https://doi.org/10.1029/2008gb003439, 2009. a
Bonnet, S., Berthelot, H., Turk-Kubo, K. A., Cornet-Barthaux, V., Fawcett, S., Berman-Frank, I., Barani, A., Grégori, G., Dekaezemacker, J., Benavides, M., and Capone, D. G.: Diazotroph derived nitrogen supports diatom growth in the South West Pacific: A quantitative study using nanoSIMS, Limnol. Oceanogr., 61, 1549–1562, https://doi.org/10.1002/lno.10300, 2016a. a, b, c, d, e, f, g, h, i, j, k, l, m, n
Bonnet, S., Berthelot, H., Turk-Kubo, K. A., Fawcett, S., Rahav, E., L'Helguen, S., and Berman-Frank, I.: Dynamics of N2 fixation and fate of diazotroph-derived nitrogen in a low-nutrient, low-chlorophyll ecosystem: Results from the VAHINE mesocosm experiment (New Caledonia), Biogeosciences, 13, 2653–2673, https://doi.org/10.5194/bg-13-2653-2016, 2016b. a, b, c, d, e, f, g, h, i
Bonnet, S., Caffin, M., Berthelot, H., and Moutin, T.: Hot spot of N2 fixation in the western tropical South Pacific pleads for a spatial decoupling between N2 fixation and denitrification, P. Natl. Acad. Sci. USA, 114, E2800–E2801, https://doi.org/10.1073/pnas.1619514114, 2017. a
Bonnet, S., Caffin, M., Berthelot, H., Grosso, O., Benavides, M., Helias-Nunige, S., Guieu, C., Stenegren, M., and Foster, R. A.: In depth characterization of diazotroph activity across the Western Tropical South Pacific hot spot of N2 fixation, Biogeosciences Discuss., https://doi.org/10.5194/bg-2017-567, in review, 2018. a, b, c
Bronk, D. A., See, J. H., Bradley, P., and Killberg, L.: DON as a source of bioavailable nitrogen for phytoplankton, Biogeosciences, 4, 283–296, https://doi.org/10.5194/bg-4-283-2007, 2007. a
Bryceson, I. and Fay, P.: Nitrogen fixation in Oscillatoria (Trichodesmium) erythraea in relation to bundle formation and trichome differentiation, Mar. Biol., 61, 159–166, 1981. a
Buitenhuis, E. T., Li, W. K. W., Vaulot, D., Lomas, M. W., Landry, M. R., Partensky, F., Karl, D. M., Ulloa, O., Campbell, L., Jacquet, S., Lantoine, F., Chavez, F., Macias, D., Gosselin, M., and McManus, G. B.: Picophytoplankton biomass distribution in the global ocean, Earth Syst. Sci. Data, 4, 37–46, https://doi.org/10.5194/essd-4-37-2012, 2012. a
Caffin, M., Moutin, T., Foster, R. A., Bouruet-Aubertot, P., Doglioli, A. M., Berthelot, H., Guieu, C., Grosso, O., Helias-Nunige, S., Leblond, N., Gimenez, A., Petrenko, A. A., de Verneil, A., and Bonnet, S.: N2 fixation as a dominant new N source in the western tropical South Pacific Ocean (OUTPACE cruise), Biogeosciences, 15, 2565–2585, https://doi.org/10.5194/bg-15-2565-2018, 2018. a, b, c, d
Campbell, L., Carpenter, E. J., Montoya, J. P., Kustka, A. B., and Capone, D. G.: Picoplankton community structure within and outside a Trichodesmium bloom in the southwestern Pacific Ocean, Vie Milieu, 55, 185–195, 2005. a
Capone, D. G.: Determination of nitrogenase activity in aquatic samples using the acetylene reduction procedure, in: Handbook of Methods in Aquat. Microb. Ecol., edited by: Cole, J. J., Lewis Press, Boca Raton, 621–31, 1993. a
Capone, D. G. and Montoya, J. P.: Nitrogen fixation and denitrification, Method. Microbiol., 30, 501–515, 2001. a
Capone, D. G., Ferrier, M. D., and Carpenter, E. J.: Amino acid cycling in colonies of the planktonic marine cyanobacterium Trichodesmium thiebautii, Appl. Environ. Microb., 60, 3989–3995, 1994. a
Capone, D. G., Zehr, J. P., Paerl, H. W., Bergman, B., and Carpenter, E. J.: Trichodesmium, a globally significant marine cyanobacterium, Science, 276, 1221–1229, 1997. a, b
Capone, D. G., Burns, J. A., Montoya, J. P., Michaels, A. F., Subramaniam, A., and Carpenter, E. J.: New nitrogen input to the tropical North Atlantic Ocean by nitrogen fixation by the cyanobacterium, Trichodesmium spp, Global Biogeochem. Cy., 19, 1–17, https://doi.org/10.1029/2004GB002331, 2005. a
Carlotti, F., Pagano, M., Guilloux, L., Donoso, K., Valdés, V., and Hunt, B. P. V.: Mesozooplankton structure and functioning in the western tropical South Pacific along the 20∘ parallel south during the OUTPACE survey (February–April 2015), Biogeosciences Discuss., https://doi.org/10.5194/bg-2017-573, in review, 2018. a, b
Carpenter, E. J.: Physiology and ecology of marine planktonic Oscillatoria (Trichodesmium), Mar. Biol. Lett., 4, 69–85, 1983. a
Carpenter, E. J., Subramaniam, A., and Capone, D. G.: Biomass and primary productivity of the cyanobacterium Trichodesmium spp. in the tropical N Atlantic ocean, Deep-Sea Res. Pt. I, 51, 173–203, 2004. a
Chen, Y. L., Tuo, S., and Chen, H. Y.: Co-occurrence and transfer of fixed nitrogen from Trichodesmium spp. to diatoms in the low-latitude Kuroshio Current in the North West Pacific, Mar. Ecol.-Prog. Ser., 421, 25–38, 2011. a
Chen, Y. L. L., Chen, H. Y., and Lin, Y. H.: Distribution and downward flux of Trichodesmium in the South China Sea as influenced by the transport from the Kuroshio Current, Mar. Ecol.-Prog. Ser., 259, 47–57, https://doi.org/10.3354/meps259047, 2003. a
Dabundo, R., Lehmann, M. F., Treibergs, L., Tobias, C. R., Altabet, M. A., Moisander, A. M., and Granger, J.: The contamination of commercial 15N2 gas stocks with 15N-labeled nitrate and ammonium and consequences for nitrogen fixation measurements, PLoS One, 9, e110335, https://doi.org/10.1371/journal.pone.0110335, 2014. a
Dekaezemacker, J. and Bonnet, S.: Sensitivity of N2 fixation to combined nitrogen forms (NO and NH) in two strains of the marine diazotroph Crocosphaera watsonii (Cyanobacteria), Mar. Ecol.-Prog. Ser., 438, 33–46, https://doi.org/10.3354/meps09297, 2011. a
Devassy, V. P., Bhattathiri, P. M. A., and Qasim, S. Z.: Trichodesmium phenomenon, Indian J. Mar. Sci., 7, 168–186, 1978. a
Devassy, V. P., Bhattathiri, P. M. A., and Qasim, S. Z.: Succession of organisms following Trichodesmium phenomenon, Indian J. Mar. Sci., 8, 89–93, 1979. a, b
Duce, R., LaRoche, J., Altieri, K., Arrigo, K., Baker, A., Capone, D., Cornell, S., Dentener, F., Galloway, J., Ganeshram, R., Geider, R. J., Jickells, T., Kuypers, M. M., Langlois, R., Liss, P. S., Liu, S. M., Middelburg, J. J., Moore, C. M., Nickovic, S., Oschlies, A., Pedersen, T., Prospero, J., Schlitzer, R., Seitzinger, S., Sorensen, L. L., Uematsu, M., Ulloa, O., Voss, M., Ward, B., and Zamora, L.: Impacts of atmospheric anthropogenic nitrogen on the open ocean, Science, 320, 893–897, 2008. a
Dupouy, C., Frouin, R., Tedetti, M., Maillard, M., Rodier, M., Lombard, F., Guidi, L., Picheral, M., Duhamel, S., Charrière, B., and Sempéré, R.: Diazotrophic Trichodesmium influence on ocean color and pigment composition in the South West tropical Pacific, Biogeosciences Discuss., https://doi.org/10.5194/bg-2017-570, in review, 2018. a
Dutheil, C., Aumont, O., Gorguès, T., Lorrain, A., Bonnet, S., Rodier, M., Dupouy, C., Shiozaki, T., and Menkes, C.: Modelling the processes driving Trichodesmium sp. spatial distribution and biogeochemical impact in the tropical Pacific Ocean, Biogeosciences Discuss., https://doi.org/10.5194/bg-2017-559, in review, 2018. a
Eppley, R. W., Reid, F. M. H., and Strickland, J. D. H.: Estimates of phytoplankton crop size, growth rate, and primary production, Bull. Scripps Inst. Oceanogr., 17, 33–42, 1970. a
Falkowski, P. G.: Evolution of the nitrogen cycle and its influence on the biological sequestration of CO2 in the ocean, Nature, 387, 272–275, https://doi.org/10.1038/387272a0, 1997. a
Fuhrman, J. A.: Marine viruses: biogeochemical and ecological effects, Nature, 399, 541–548, 1999. a
Fukuda, R., Ogawa, H., Nagata, T., and Koike, I.: Direct determination of carbon and nitrogen contents of natural bacterial assemblages in marine environments nitrogen contents of natural bacterial assemblages in marine environments, Appl. Environ. Microb., 64, 3352–3358, 1998. a
Garcia, N., Raimbault, P., and Sandroni, V.: Seasonal nitrogen fixation and primary production in the Southwest Pacific: nanoplankton diazotrophy and transfer of nitrogen to picoplankton organisms, Mar. Ecol.-Prog. Ser., 343, 25–33, https://doi.org/10.3354/Meps06882, 2007. a
Glibert, P. M. and Bronk, D.: Release of dissolved organic nitrogen by marine diazotrophic cyanobacteria, Trichodesmium spp., Appl. Environ. Microb., 60, 3996–4000, 1994. a, b, c, d
Glibert, P. M. and O'Neil, J. M.: Dissolved organic nitrogen release and amino-acid oxidase activity by Trichodesmium, in: Marine cyanobacteria, edited by: ORSTOM, Bulletin de l'Institut Océanographoque, edited by: Charpy, L. and Larkum, T., Paris, 265–271, 1999. a
Grégori, G., Colosimo, A., and Denis, M.: Phytoplankton group dynamics in the Bay of Marseilles during a 2-year survey based on analytical flow cytometry, Cytometry, 44, 247–256, 2001. a, b
Gruber, N.: The Marine Nitrogen Cycle: Overview and Challenges, Nitrogen in the Marine Environment, 1–50, https://doi.org/10.1016/B978-0-12-372522-6.00001-3, 2008. a
Guo, C. and Tester, P. A.: Toxic effect of the bloom-forming Trichodesmium sp. (cyanophyta) to the copepod Acartia tonsa, Nat. Toxins, 2, 222–227, https://doi.org/10.1002/nt.2620020411, 1994. a
Hansell, D. A. and Carlson, C. A.: Biogeochemistry of total organic carbon and nitrogen in the Sargasso Sea: control by convective overturn, Deep-Sea Res. Pt. II, 48, 1649–1667, 2001. a
Hasle, G. R.: The inverted microscope, in: Phytoplankton Manual, edited by Sournia, A., vol. 6, UNESCO Monographs on oceanographic methodology, Paris, 1978. a
Hawser, S. P. and Codd, G. A.: The toxicity of Trichodesmium blooms from Caribbean waters, in: Marine Pelagic Cyanobacteria: Trichodesmium and other Diazotrophs, edited by: Carpenter, E. J., Capone, D. G., and Rueter, J. G., Springer Netherlands, Dordrecht, 319–329, 1992. a
Hawser, S. P., O'Neil, J. M., Roman, M. R., and Codd, G. A.: Toxicity of blooms of the cyanobacterium Trichodesmium to zooplankton, J. Appl. Phycol., 4, 79–86, https://doi.org/10.1007/BF00003963, 1992. a
Hewson, I., Govil, S. R., Capone, D. G., Carpenter, E. J., and Fuhrman, J. A.: Evidence of Trichodesmium viral lysis and potential significance for biogeochemical cycling in the oligotrophic ocean, Aquat. Microb. Ecol., 36, 1–8, https://doi.org/10.3354/ame036001, 2004. a
Hmelo, L. R., Van Mooy, B. A. S., and Mincer, T. J.: Characterization of bacterial epibionts on the cyanobacterium Trichodesmium, Aquat. Microb. Ecol., 67, 1–14, 2012. a
Holmes, R. M., Aminot, A., Kerouel, R., Hooker, B. A., and Peterson, B. J.: A simple and precise method for measuring ammonium in marine and freshwater ecosystems, Can. J. Fish. Aquat. Sci., 56, 1801–1808, 1999. a
Hunt, B. P. V., Bonnet, S., Berthelot, H., Conroy, B. J., Foster, R. A., and Pagano, M.: Contribution and pathways of diazotroph-derived nitrogen to zooplankton during the VAHINE mesocosm experiment in the oligotrophic New Caledonia lagoon, Biogeosciences, 13, 3131–3145, https://doi.org/10.5194/bg-13-3131-2016, 2016. a, b, c, d
Hutchins, D. A., Fu, F. X., Zhang, Y., Warner, M. E., Feng, Y., Portune, K., Bernhardt, P. W., and Mulholland, M. R.: CO2 control of Trichodesmium N2 fixation, photosynthesis, growth rates, and elemental ratios: Implications for past, present, and future ocean biogeochemistry, Limnol. Oceanogr., 52, 1293–1304, 2007. a
Karl, D. M., Letelier, R., Hebel, D. V., Bird, D. F., and Winn, C. D.: Trichodesmium blooms and new nitrogen in the North Pacific gyre, Springer, 1992. a, b
Karl, D. M., Letelier, R. M., Tupas, L. M., Dore, J. E., Christian, J., and Hebel, D. V.: The role of nitrogen fixation in biogeochemical cycling in the subtropical North Pacific Ocean, Nature, 388, 533–538, https://doi.org/10.1038/41474, 1997. a, b
Karl, D. M., Church, M. J., Dore, J. E., Letelier, R. M., and Mahaffey, C.: Predictable and efficient carbon sequestration in the North Pacific Ocean supported by symbiotic nitrogen fixation, P. Natl. Acad. Sci. USA, 109, 1842–1849, https://doi.org/10.1073/pnas.1120312109, 2012. a
Knapp, A. N., Dekaezemacker, J., Bonnet, S., Sohm, J. A., and Capone, D. G.: Sensitivity of Trichodesmium erythraeum and Crocosphaera watsonii abundance and N2 fixation rates to varying NO and PO concentrations in batch cultures, Aquat. Microb. Ecol., 66, 223–236, 2012. a
Knapp, A. N., McCabe, K. M., Grosso, O., Leblond, N., Moutin, T., and Bonnet, S.: Distribution and rates of nitrogen fixation in the western tropical South Pacific Ocean constrained by nitrogen isotope budgets, Biogeosciences, 15, 2619–2628, https://doi.org/10.5194/bg-15-2619-2018, 2018. a
Konno, U., Tsunogai, U., Komatsu, D. D., Daita, S., Nakagawa, F., Tsuda, A., Matsui, T., Eum, Y. J., and Suzuki, K.: Determination of total N2 fixation rates in the ocean taking into account both the particulate and filtrate fractions, Biogeosciences, 7, 2369–2377, https://doi.org/10.5194/bg-7-2369-2010, 2010. a, b
Landrum, J. P., Altabet, M. A., and Montoya, J. P.: Basin-scale distributions of stable nitrogen isotopes in the subtropical North Atlantic Ocean: Contribution of diazotroph nitrogen to particulate organic matter and mesozooplankton, Deep-Sea Res. Pt. I, 58, 615–625, 2011. a
Leblanc, K., Cornet, V., Rimmelin-Maury, P., Grosso, O., Hélias-Nunige, S., Brunet, C., Claustre, H., Ras, J., Leblond, N., and Quéguiner, B.: Silicon cycle in the Tropical South Pacific: evidence for an active pico-sized siliceous plankton, Biogeosciences Discuss., https://doi.org/10.5194/bg-2018-149, in review, 2018. a
Lenes, J. M., Darrow, B. P., Catrall, C., Heil, C. A., Callahan, L., Vargo, G. A., Byrne, R. H., Prospero, J. M., Bates, D. E., Fanning, K. A., and Walsh, J. J.: Iron fertilization and the Trichodesmium response on the West Florida shelf, Limnol. Oceanogr., 46, 1261–1277, 2001. a
Letelier, R. M. and Karl, D. M.: Role of Trichodesmium spp. in the productivity of the subtropical north Pacific Ocean, Mar. Ecol.-Prog. Ser., 133, 263–273, 1996. a
Loescher, C. R., Großkopf, T., Desai, F. D., Gill, D., Schunck, H., Croot, P. L., Schlosser, C., Neulinger, S. C., Pinnow, N., Lavik, G., Kuypers, M. M., LaRoche, J., and Schmitz, R. A.: Facets of diazotrophy in the oxygen minimum zone waters off Peru, ISME J., 8, 2180–2192, https://doi.org/10.1038/ismej.2014.71, 2014. a
Loick-Wilde, N., Dutz, J., Miltner, A., Gehre, M., Montoya, J. P., and Voss, M.: Incorporation of nitrogen from N2 fixation into amino acids of zooplankton, Limnol. Oceanogr., 57, 199–210, https://doi.org/10.4319/lo.2012.57.1.0199, 2012. a
Luo, Y. W., Doney, S. C., Anderson, L. A., Benavides, M., Bode, A., Bonnet, S., Boström, K. H., Böttjer, D., Capone, D. G., Carpenter, E. J., Chen, Y. L., Church, M. J., Dore, J. E., Falcón, L. I., Fernández, A., Foster, R. A., Furuya, K., Gómez, F., Gundersen, K., Hynes, A. M., Karl, D. M., Kitajima, S., Langlois, R. J., LaRoche, J., Letelier, R. M., Maranón, E., McGillicuddy Jr., D. J., Moisander, P. H., Moore, C. M., Mourino-Carballido, B., Mulholland, M. R., Needoba, J. A., Orcutt, K. M., Poulton, A. J., Raimbault, P., Rees, A. P., Riemann, L., Shiozaki, T., Subramaniam, A., Tyrrell, T., Turk-Kubo, K. A., Varela, M., Villareal, T. A., Webb, E. A., White, A. E., Wu, J., and Zehr, J. P.: Database of diazotrophs in global ocean: abundance, biomass and nitrogen fixation rates, Earth Syst. Sci. Data, 4, 47–73, https://doi.org/10.5194/essd-4-47-2012, 2012. a, b
Marie, D., Partensky, F., Vaulot, D., and Brussaard, C.: Enumeration of phytoplankton, bacteria and viruses in marine samples, in: Current Protocols in Cytometry, edited by: Robinson, J. P., John Wiley & Sons, Inc., New York, 11–15, 1999. a
Mohr, W., Großkopf, T., Wallace, D. W. R., and LaRoche, J.: Methodological underestimation of oceanic nitrogen fixation rates, PLoS ONE, 5, 1–7, https://doi.org/10.1371/journal.pone.0012583, 2010. a
Moisander, A. M., Serros, T., Pearl, R. W., Beinart, A., and Zehr, J. P.: Gammaproteobacterial diazotrophs and nifH gene expression in surface waters of the South Pacific Ocean, ISME J., 1–12, https://doi.org/10.1038/ismej.2014.49, 2014. a
Mompeán, C., Bode, A., Benítez-Barrios, V. M., Domínguez-Yanes, J. F., Escánez, J., and Fraile-Nuez, E.: Spatial patterns of plankton biomass and stable isotopes reflect the influence of the nitrogen-fixer Trichodesmium along the subtropical North Atlantic, J. Plankton Res., 35, 513–525, https://doi.org/10.1093/plankt/fbt011, 2013. a
Montoya, J. P., Voss, M., Kahler, P., and Capone, D. G.: A simple, high-precision, high-sensitivity tracer assay for N2 fixation, Appl. Environ. Microb., 62, 986–993, 1996. a, b
Montoya, J. P., Carpenter, E. J., and Capone, D. G.: Nitrogen fixation and nitrogen isotope abundances in zooplankton of the oligotrophic North Atlantic Ocean, Limnol. Oceanogr., 47, 1617–1628, 2002. a
Moore, C. M., Mills, M. M., Arrigo, K. R., Berman-Frank, I., Bopp, L., Boyd, P. W., Galbraith, E. D., Geider, R. J., Guieu, C., Jaccard, S. L., Jickells, T. D., La Roche, J., Lenton, T. M., Mahowald, N. M., Marañón, E., Marinov, I., Moore, J. K., Nakatsuka, T., Oschlies, A., Saito, M. A., Thingstad, T. F., Tsuda, A., and Ulloa, O.: Processes and patterns of oceanic nutrient limitation, Nat. Geosci., 6, 701–710, https://doi.org/10.1038/ngeo1765, 2013. a
Moutin, T., Doglioli, A. M., de Verneil, A., and Bonnet, S.: Preface: The Oligotrophy to the UlTra-oligotrophy PACific Experiment (OUTPACE cruise, 18 February to 3 April 2015), Biogeosciences, 14, 3207–3220, https://doi.org/10.5194/bg-14-3207-2017, 2017. a, b
Moutin, T., Wagener, T., Caffin, M., Fumenia, A., Gimenez, A., Baklouti, M., Bouruet-Aubertot, P., Pujo-Pay, M., Leblanc, K., Lefevre, D., Helias Nunige, S., Leblond, N., Grosso, O., and de Verneil, A.: Nutrient availability and the ultimate control of the biological carbon pump in the western tropical South Pacific Ocean, Biogeosciences, 15, 2961–2989, https://doi.org/10.5194/bg-15-2961-2018, 2018. a
Mulholland, M. R.: The fate of nitrogen fixed by diazotrophs in the ocean, Biogeosciences, 4, 37–51, https://doi.org/10.5194/bg-4-37-2007, 2007. a
Mulholland, M. R. and Bernhardt, P. W.: The effect of growth rate, phosphorus concentration, and temperature on N2 fixation, carbon fixation, and nitrogen release in continuous cultures of Trichodesmium IMS101, Limnol. Oceanogr., 50, 839–849, 2005. a
Mulholland, M. R. and Capone, D. G.: The nitrogen physiology of the marine N2-fixing cyanobacteria Trichodesmium spp., Trends Plant Sci., 5, 148–153, 2000. a, b
Mulholland, M. R., Bronk, D. A., and Capone, D. G.: Dinitrogen fixation and release of ammonium and dissolved organic nitrogen by Trichodesmium IMS101, Aquat. Microb. Ecol., 37, 85–94, 2004a. a, b
Mulholland, M. R., Heil, C. A., Bronk, D. A., O'Neil, J. M., and Bernhardt, P. W.: Does nitrogen regeneration from the N2 fixing cyanobacteria, Trichodesmium spp. fuel Karenia brevis blooms in the Gulf of Mexico?, Harmful Algae 2002, 47–49, 2004b. a, b
Nelson, D. M., Tréguer, P., Brzezinski, M. A., Leynaert, A., and Quéguiner, B.: Production and dissolution of biogenic silica in the ocean: Revised global estimates, comparison with regional data and relationship to biogenic sedimentation, Global Biogeochem. Cy., 9, 359–372, https://doi.org/10.1029/95GB01070, 1995. a
Ohki, K.: A possible role of temperate phage in the regulation of Trichodesmium, Bull. Inst. Oceanogr. Monaco, 19, 235–256, 1999. a
Ohlendieck, U., Stuhr, A., and Siegmund, H.: Nitrogen fixation by diazotrophic cyanobacteria in the Baltic Sea and transfer of the newly fixed nitrogen to picoplankton organisms, J. Marine Syst., 25, 213–219, 2000. a
O'Neil, J. and Roman, M. R.: Grazers and Associated Organisms of Trichodesmium, in: Marine Pelagic Cyanobacteria: Trichodesmium and other Diazotrophs, edited by: Carpenter, E. J., Capone, D. G., and Rueter, J. G., NATO ASI Series, Springer Netherlands, 1992. a, b
O'Neil, J. M.: Grazer interactions with nitrogen-fixing marine Cyanobacteria: adaptation for N-acquisition?, Bull. Inst. Oceanogr. Monaco, 19, 293–317, 1999. a
O'Neil, J. M., Metzler, P., and Glibert, P. M.: Ingestion of 15N2-labelled Trichodesmium, and ammonium regeneration by the pelagic harpacticoid copepod Macrosetella gracilis, Mar. Biol., 125, 89–96, 1996. a
Paerl, H. W., Priscu, J. C., and Brawner, D. L.: Immunochemical localization of nitrogenase in marine Trichodesmium aggregates: Relationship to N2 fixation potential, Appl. Environ. Microb., 55, 2965–2975, 1989. a
Partensky, F., Hess, W. R., and Vaulot, D.: Prochlorococcus, a marine photosynthetic prokaryote of global significance, Microbiol. Mol. Biol. R., 63, 106–127, 1999. a
Pujo-Pay, M. and Raimbault, P.: Improvement of the wet-oxidation procedure for simultaneous determination of particulate organic nitrogen and phosphorus collected on filters, Mar. Ecol.-Prog. Ser., 105, 203–207, https://doi.org/10.3354/meps105203, 1994. a
Riemann, L., Farnelid, H., and Steward, G. F.: Nitrogenase genes in non-cyanobacterial plankton: prevalence, diversity and regulation in marine waters, Aquat. Microb. Ecol., 61, 235–247, https://doi.org/10.3354/ame01431, 2010. a
Sheridan, C. C., Steinberg, D. K., and Kling, G. W.: The microbial and metazoan community associated with colonies of Trichodesmium spp.: a quantitative survey, J. Plankton Res., 24, 913–922, https://doi.org/10.1093/plankt/24.9.913, 2002. a, b
Slawyk, G. and Raimbault, P.: Simple procedure for simultaneous recovery of dissolved inorganic and organic nitrogen in 15N-tracer experiments and improving the isotopic mass balance, Mar. Ecol.-Prog. Ser., 124, 289–299, https://doi.org/10.3354/meps124289, 1995. a, b
Smayda, T.: What to count, Phytoplankton manual, UNESCO, Paris, 165–166, 1978. a
Sommer, F., Hansen, T., and Sommer, U.: Transfer of diazotrophic nitrogen to mesozooplankton in Kiel Fjord, Western Baltic Sea: a mesocosm study, Mar. Ecol.-Prog. Ser., 324, 105–112, 2006. a
Steinberg, D. K. and Landry, M. R.: Zooplankton and the ocean carbon cycle, Annu. Rev. Mar. Sci., 9, 413–444, 2017. a
Stenegren, M., Caputo, A., Berg, C., Bonnet, S., and Foster, R. A.: Distribution and drivers of symbiotic and free-living diazotrophic cyanobacteria in the western tropical South Pacific, Biogeosciences, 15, 1559–1578, https://doi.org/10.5194/bg-15-1559-2018, 2018. a, b, c, d, e, f, g
Sun, J. and Liu, D.: Geometric models for calculating cell biovolume and surface area for phytoplankton, J. Plankton Res., 25, 1331–1346, https://doi.org/10.1093/plankt/fbg096, 2003. a
Van Wambeke, F., Bonnet, S., Moutin, T., Raimbault, P., Alarcón, G., and Guieu, C.: Factors limiting heterotrophic bacterial production in the southern Pacific Ocean, Biogeosciences, 5, 833–845, https://doi.org/10.5194/bg-5-833-2008, 2008. a
Van Wambeke, F., Gimenez, A., Duhamel, S., Dupouy, C., Lefevre, D., Pujo-Pay, M., and Moutin, T.: Dynamics and controls of heterotrophic prokaryotic production in the western tropical South Pacific Ocean: links with diazotrophic and photosynthetic activity, Biogeosciences, 15, 2669–2689, https://doi.org/10.5194/bg-15-2669-2018, 2018. a, b, c
Verity, P. G., Robertson, C. Y., Tronzo, C. R., Andrews, M. G., Nelson, J. R., and Sieracki, M. E.: Relationships between cell volume and the carbon and nitrogen content of marine photosynthetic nanoplankton, Limnol. Oceanogr., 37, 1434–1446, https://doi.org/10.4319/lo.1992.37.7.1434, 1992. a
Villareal, T. A.: Widespread occurrence of the Hemiaulus-cyanobacteria symbiosis in the southwest North Atlantic Ocean, B. Mar. Sci., 54, 1–7, 1994. a
Vincent, D., Slawyk, G., L'Helguen, S., Sarthou, G., Gallinari, M., Seuront, L., Sautour, B., and Ragueneau, O.: Net and gross incorporation of nitrogen by marine copepods fed on 15N-labelled diatoms: Methodology and trophic studies, J. Exp. Mar. Biol. Ecol., 352, 295–305, 2007. a
Walsby, A. E.: The gas vesicles and buoyancy of Trichodesmium, in: Marine pelagic cyanobacteria: Trichodesmium and other diazotrophs, Springer Netherlands, 141–161, 1992. a
Wannicke, N., Koch, B. P., and Voss, M.: Release of fixed N2 and C as dissolved compounds by Trichodesmium erythreum and Nodularia spumigena under the influence of high light and high nutrient (P), Aquat. Microb. Ecol., 57, 175–189, 2009. a
Wannicke, N., Korth, F., Liskow, I., and Voss, M.: Incorporation of diazotrophic fixed N2 by mesozooplankton – Case studies in the southern Baltic Sea, J. Marine Syst., 117–118, 1–13, 2013. a
Zehr, J. P. and Turner, P. J.: Nitrogen fixation: Nitrogenase genes and gene expression, in: Methods in Marine Microbiology, Academic Press, New York, 2001. a
Zehr, J. P., Mellon, M. T., and Zani, S.: New nitrogen-fixing microorganisms detected in oligotrophic oceans by amplification of nitrogenase (nifH) genes, Appl. Environ. Microb., 64, 3444–3450, 1998. a
Zehr, J. P., Bench, S. R., Carter, B. J., Hewson, I., Niazi, F., Shi, T., Tripp, H. J., and Affourtit, J. P.: Globally Distributed Uncultivated Oceanic N2-Fixing Cyanobacteria Lack Oxygenic Photosystem II, Science, 322, 1110–1112, https://doi.org/10.1126/science.1165340, 2008. a