the Creative Commons Attribution 4.0 License.
the Creative Commons Attribution 4.0 License.
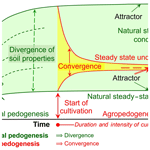
Reviews and syntheses: Agropedogenesis – humankind as the sixth soil-forming factor and attractors of agricultural soil degradation
Kazem Zamanian
Agricultural land covers 5.1×109 ha (ca. 50 % of potentially suitable land area), and agriculture has immense effects on soil formation and degradation. Although we have an advanced mechanistic understanding of individual degradation processes of soils under agricultural use, general concepts of agropedogenesis are absent. A unifying theory of soil development under agricultural practices, of agropedogenesis, is urgently needed. We introduce a theory of anthropedogenesis – soil development under the main factor “humankind” – the sixth factor of soil formation, and deepen it to encompass agropedogenesis as the most important direction of anthropedogenesis. The developed theory of agropedogenesis consists of (1) broadening the classical concept of along with their feedbacks to the processes, (2) a new concept of attractors of soil degradation, (3) selection and analysis of master soil properties, (4) analysis of phase diagrams of master soil properties to identify thresholds and stages of soil degradation, and, finally, (5) a definition of the multidimensional attractor space of agropedogenesis. The main feature of anthropedogenesis is the narrowing of soil development to only one function (e.g. crop production for agropedogenesis), and this function is becoming the main soil-forming factor. The focus on only one function and the disregard of other functions inevitably lead to soil degradation. We show that the factor humankind dominates over the effects of the five natural soil-forming factors and that agropedogenesis is therefore much faster than natural soil formation. The direction of agropedogenesis is largely opposite to that of natural soil development and is thus usually associated with soil degradation. In contrast to natural pedogenesis leading to divergence of soil properties, agropedogenesis leads to their convergence because of the efforts to optimize conditions for crop production. Agricultural practices lead soil development toward a quasi-steady state with a predefined range of measured properties – attractors (an attractor is a minimal or maximal value of a soil property toward which the property will develop via long-term intensive agricultural use from any natural state). Based on phase diagrams and expert knowledge, we define a set of “master properties” (bulk density and macroaggregates, soil organic matter content, C:N ratio, pH and electrical conductivity – EC, microbial biomass and basal respiration) as well as soil depth (A and B horizons). These master properties are especially sensitive to land use and determine the other properties during agropedogenesis. Phase diagrams of master soil properties help identify thresholds and stages of soil degradation, each of which is characterized by one dominating process. Combining individual attractors in a multidimensional attractor space enables predicting the trajectory and the final state of agrogenic soil development and developing measures to combat soil degradation. In conclusion, the suggested new theory of anthro- and agropedogenesis is a prerequisite for merging various degradation processes into a general view and for understanding the functions of humankind not only as the sixth soil-forming factor but also as an ecosystem engineer optimizing its environment to fulfil a few desired functions.
- Article
(10815 KB) - Full-text XML
-
Supplement
(1725 KB) - BibTeX
- EndNote
- Included in Encyclopedia of Geosciences
1.1 Soil degradation by agricultural land use
Soils (S) as natural bodies are formed via interactions of soil-forming factors, i.e. climate (cl), organisms (o), relief (r) and parent material (p) over time (t) (Dokuchaev, 1883; Glinka, 1927; Jenny, 1941; Zakharov, 1927): (see the copy–paste history of the equation in the Supplement).
The processes of additions, losses, transfers and translocation, and transformations of matter and energy over centuries and millennia produce a medium – soil (Simonson, 1959), which supports plant roots and fulfils many other ecosystem functions (Lal, 2008; Nannipieri et al., 2003; Paul, 2014). These functions commonly decrease due to human activities, in particular through agricultural practices because of accelerated soil erosion, nutrient loss (despite intensive fertilization), aggregate destruction, compaction, acidification, alkalization and salinization (Homburg and Sandor, 2011; Sandor and Homburg, 2017). Accordingly, the factor humankind has nearly always been considered to be a soil-degrading entity that, by converting natural forests and grasslands to arable lands, changes the natural cycles of energy and matter. Except in very rare cases that lead to the formation of fertile soils such as Terra Preta in the Amazon Basin (Glaser et al., 2001), Plaggen in northern Europe (Pape, 1970) and Hortisols (Burghardt et al., 2018), soil degradation is the most common outcome of agricultural practices (DeLong et al., 2015; Homburg and Sandor, 2011). Soil degradation begins immediately after conversion of natural soil and involves the degradation in all physical, chemical and biological properties (Table 1). The result is a decline in ecosystem functions.
Table 1Processes and mechanisms of soil degradation by agricultural land use.
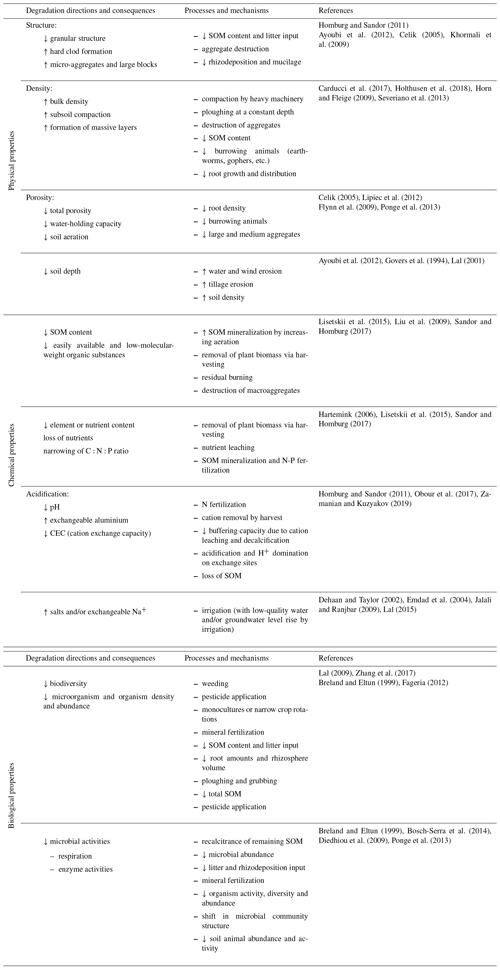
↑ and ↓ mean increase or decrease, respectively.
Soil degradation gains importance with the rapid increase in the human population (Carozza et al., 2007) and technological progress. Increasing food demand requires either larger areas for croplands and/or intensification of crop production per area of already-cultivated land. Because the land resources suitable for agriculture are limited, most increases in food production depend on the second option: intensification (Lal, 2005). While prohibiting or reducing degradation is essential in achieving sustainable food production (Lal, 2009), many studies have addressed individual mechanisms and specific drivers of soil degradation (Table 1). Nonetheless, there is still no standard and comprehensive measure to determine soil degradation intensity and to differentiate between degradation stages.
Agricultural soils (croplands plus grasslands) cover 5.1×109 ha, corresponding to about 34 % of the global land area. Huge areas are located in very cold regions that are continuously covered by ice (1.5×109 ha); in hot deserts, mountainous areas or barren regions (2.8×109 ha); and sealed in urban and industrial regions and roads (ca. 0.2×109 ha). Accordingly, agricultural lands cover about 50 % of the area potentially suitable for agriculture (https://ourworldindata.org/yields-and-land-use-in-agriculture, last access: May 2019). Even though huge areas of land are occupied by agriculture, and humans have modified natural soils over the last 10–12 thousand years, a theory of soil formation as affected by humankind – anthropedogenesis and its subcategory agropedogenesis – is absent. This paper therefore presents for the first time a unifying theory of anthropedogenesis – soil development under the main factor humankind – the sixth factor of soil formation. Moreover, we expand it to encompass agropedogenesis as a key aspect of general anthropedogenesis.
1.2 Humans as the main soil-forming factor
Humans began to modify natural soils at the onset of agriculture ca. 10–12 thousand years ago (Diamond, 2002; Richter, 2007), resulting in soil degradation. Examples of soil degradation leading to civilization collapses are well known, starting at least with Mesopotamia (18th to 6th centuries BCE; Diamond, 2002; Weiss et al., 1993). Notwithstanding all the negative impacts humans have on soils, the intention was always to increase fertility to boost crop production (Richter et al., 2011; Sandor and Homburg, 2017), reduce negative environmental consequences and achieve more stable agroecosystems. To attain these aims, humans have (i) modified soil physical and hydrological properties (for example, by removing stones and loosening soil by tillage, run-off irrigation, draining and terracing); (ii) altered soil chemical conditions through fertilization, liming and desalinization; and (iii) controlled biodiversity by sowing domesticated plant species and applying biocides (Richter et al., 2015; Richter, 2007). Although these manipulations commonly lead to soil degradation (Homburg and Sandor, 2011; Paz-González et al., 2000; Sandor et al., 2008), they are aimed at decreasing the most limiting factors (nutrient contents, soil acidity, water scarcity, etc.) for crop production regardless of the original environmental conditions in which the soil was formed (Guillaume et al., 2016b; Liu et al., 2009). Thus, agricultural land use always focused on removing limiting factors and providing optimal growth conditions for a few selected crops: 15 species make up 90 % of the world's food, and three of them – corn, wheat and rice – supply two-thirds of this amount (FAO, 2018). These crops (except rice) have similar water and nutrient requirements in contrast to the plants growing under natural conditions. Consequently, agricultural land use has always striven to narrow soil properties to uniform environmental conditions.
Humans can even change soil types as defined by classification systems (Fig. S1 in the Supplement) by inducing erosion, changing the thickness of horizons and their mixture, decreasing soil organic matter (SOM) content, destroying aggregates, and accumulating salts (Dazzi and Monteleone, 2007; Ellis and Newsome, 1991; Shpedt et al., 2017). A Mollisol (similar to Chernozems or Phaeozems), for example, turns into an Inceptisol (similar to Cambisols) by decreasing total SOM (Lo Papa et al., 2013; Tugel et al., 2005) and/or thinning of the mollic epipedon by tillage and erosion and destroying granular and sub-polyhedric structure (Ayoubi et al., 2012; Lo Papa et al., 2013). Accordingly, humankind can no longer be treated solely as a soil-degrading but also as a soil-forming factor (Amundson and Jenny, 1991; Dudal, 2004; Gerasimov and Fridland, 1984; Richter et al., 2015; Sandor et al., 2005). The result is the formation of anthropogenic soils (soils formed under the main factor humankind). This is well known for rice paddies, i.e. Hydragric Anthrosols (Chen et al., 2011; Cheng et al., 2009; Kölbl et al., 2014; Sedov et al., 2007), Hortic Anthrosols (long-term fertilized soils with household wastes and manure) and Irragric Anthrosols (long-term irrigated soils in dry regions; WRB, 2014). These effects have stimulated the ongoing development of soil classifications to reflect new directions of soil evolution (Bryant and Galbraith, 2003; Richter, 2007): anthropedogenesis, i.e. soil genesis under the main factor humankind, and in particular agropedogenesis, i.e. soil genesis under agricultural practices as a subcategory of anthropedogenesis.
Human impacts on soil formation have immensely accelerated in the last 50–100 years (Dudal, 2004; Gerasimov and Fridland, 1984; Richter, 2007) with the (1) introduction of heavy machinery, (2) application of high rates of mineral fertilizers, especially after discovery of N fixation by the Haber–Bosch technology, (3) application of chemical plant protection, and (4) introduction of crops with higher yield and reduced root systems. We expect that, despite various ecological measures (no-till practices, restrictions on chemical fertilizer applications and heavy machinery, etc.), the effects of humans on soil formation will increase in the Anthropocene and will be even stronger than for most other components of global change. This urgently calls for a concept and theory of soil formation under humans as the main factor.
Anthropedogenesis is the soil formation under the main factor “human” (Amundson and Jenny, 1991; Bidwell and Hole, 1965; Howard, 2017; Meuser, 2010; Richter, 2007; Yaalon and Yaron, 1966). Agropedogenesis is the dominant form of anthropedogenesis and includes soil formation under agricultural use – mainly cropland (Sandor et al., 2005). The other forms of anthropedogenesis are construction of completely new soils (Technosols, e.g. urban soils or mine soils). These other forms of anthropedogenesis are not treated here because they are not connected with agriculture.
Agropedogenesis should be clearly separated from the natural pedogenesis because of (1) strong dominance of the factor “human” over the other five factors of soil formation, (2) new processes and mechanisms that are absent under natural soil development (Table 2), (3) new directions of soil developments compared to natural processes (Table 2), (4) frequent development of processes in the reverse direction compared to natural pedogenesis, and (5) much higher intensity of many specific processes compared to natural developments and consequently faster rates of all changes.
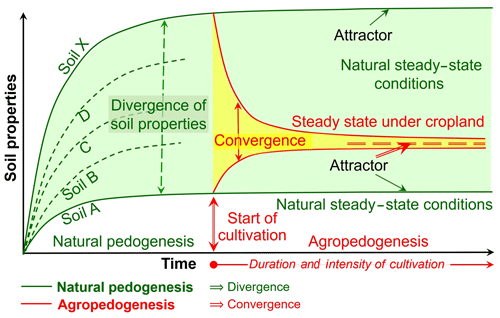
Figure 1Conceptual scheme of soil development, i.e. pedogenesis, under natural conditions (green lines) and agropedogenesis due to long-term agricultural practices (red lines). Green area: the increasing variability in natural soils during pedogenesis. Yellow area: decrease in the variability in soil properties by agricultural use. Double-line vertical arrow: the start of cultivation. x axis: time for natural soil development and duration and intensity of cultivation under agricultural use. Natural pedogenesis leads (from the initial parent material) to a wide range of steady-state values (green dashed arrow) for a given soil property over hundreds or thousands of years due to various combinations of the five soil-forming factors. Natural pedogenesis leads to divergence of soil properties. In contrast, agricultural practices and the dominance of humans as the main soil-forming factor cause each property to tend toward a very narrow field of values, i.e. attractors of that property defined by human actions, namely land management for optimizing the production of few crops. Therefore, agropedogenesis leads to convergence of soil properties.
Agropedogenesis and natural pedogenesis are partially opposite. Natural soil formation involves the development of soils from parent materials under the effects of climate, organisms, relief and time (Dokuchaev, 1883; Jenny, 1941; Zakharov, 1927; Supplement). Here, soil formation will reach the quasi-steady state typical for the combination of the five soil-forming factors (Targulian and Goryachkin, 2004) (Fig. 1). Agropedogenesis, in most cases, is a process involving the loss of soil fertility, i.e. degradation because of intensive agriculture and narrowing of soil properties. Agropedogenesis is partially the reverse of soil formation, but the final stage is not the parent material (except in a few cases of extreme erosion). Agropedogenesis also leads to a quasi-steady state of soils (Fig. 1; Eleftheriadis et al., 2018; Wei et al., 2014). The time needed to reach this quasi-steady state, however, is much shorter (in the range of a few centuries, decades or an even shorter time period) than for natural pedogenesis, which involves millennia (Tugel et al., 2005). The range of soil properties at this quasi-steady state will show the end limit of agricultural effects on soil development.
Our theory of agropedogenesis is based on five components: the (1) concept of “”, (2) concept of “attractors of soil degradation”, (3) selection and analysis of master soil properties, (4) analysis of phase diagrams between the master soil properties and identification of thresholds and stages of soil degradation, and (5) “multi-dimensional attractor space” and trajectory of pedogenesis.
2.1 Concept: factors → processes → properties → functions
The original concept of “soil factors→soil properties” was initially suggested by Dokuchaev (1883) and Zakharov (1927) and was modified by “processes”, which are dependent on the factors of soil formation and develop the properties (Gerasimov, 1984; McBratney et al., 2003). This triad, , enables understanding soil development from the initial parent materials by the effects of climate, organisms and relief, over time. This describes the visible morphological soil properties in the field and measurable parameters in the lab very well, leading to the development of various semi-genetic or genetic soil classifications (KA-5, 2005; KDPR, 2004; WRB, 2014).
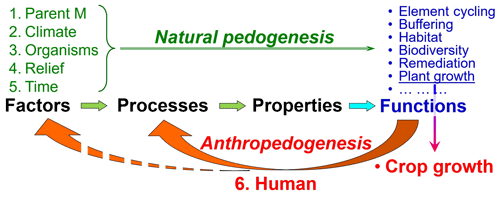
Figure 2Soil genesis based on the five natural factors of soil formation and the sixth factor: human. Natural processes are presented in green, and human processes are presented in red. The concept factors → properties was suggested by Dokuchaev (1883) and Zakharov (1927; see Supplement) and later by Jenny (1941). The theory we introduced, factors → processes → properties → functions, considers not only the functions of natural soils but also human modification of soils toward only one function of interest (here crop growth). Anthropogenic optimization of only one function involves strongly modifying processes and factors, leading to formation of a new process group: anthropedogenesis. The arrows pointing downward reflect the main specifics of anthropogenesis: one of the functions becomes a factor of pedogenesis and modifies the processes.
Considering the recent development of functional approaches and ecosystem perspectives, this triad is insufficient. We therefore introduce the concept (Fig. 2). Rather than describing here the very broad range of functions of natural soils as related to clean air and water, biodiversity, decontamination of pollutants, biofuel and waste management, etc., we refer to excellent reviews focused on soil functions (Lal, 2008; Nannipieri et al., 2003).
One function – plant growth – is crucial for agropedogenesis (Fig. 2) because humans change this natural function to an anthropogenic function – crop growth – thus adapting and modifying natural soils to maximize productivity and crop yields. As it is not possible to simultaneously maximize all functions, the functions other than “crop growth” decrease or even disappear. Accordingly, agropedogenesis is driven by processes pursuing the maximization of only one function – crop growth. The consequence is that all other soil functions are reduced. We define soil degradation as a reduction of functions. Initially, all functions will be reduced at the cost of increased crop production. As degradation advances, however, the production function decreases as well. Nearly all previous definitions of soil degradation were based on declining crop productivity. The principal difference between our concept of soil degradation and the most common other concepts is that the degradation starts with the reduction of one or more functions – before crop productivity decreases. This concept, based on multifunctionality, is much broader and considers the ecosystem functions and services of soil and the growing human demand for a healthy environment.
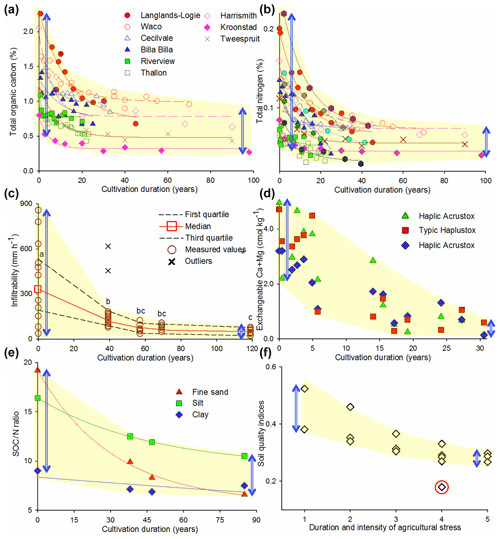
Figure 3Examples for attractors of soil properties by anthropogenic degradation: (a) soil organic carbon content, (b) total nitrogen content, (c) infiltration rates, (d) exchangeable Ca2+ and Mg2+ contents, (e) C:N ratio, and (f) overall decrease in soil quality, i.e. degradation over the cultivation period. Yellow shading area: covering all experimental points, showing a decrease in the area with cultivation duration. Blue double arrows: range of data points in natural soils (left of each panel) and strong decrease in data range due to cultivation (right of each panel). (a) Narrowing range (blue arrows) of soil organic C over cultivation periods in southern Queensland, Australia (six sites; Dalal and Mayer, 1986a), and savanna soils in South Africa (three sites; Lobe et al., 2001). The natural soils in different climatic regions have various ranges of properties, e.g. organic C from 0.8 % to 2.3 %. During cultivation, however, the organic C content strongly narrows to between 0.3 % and 1.0 %. (b) Narrowing range (blue arrows) of total soil N over cultivation periods. Sampling sites similar to (a) plus five sites (hexagons) from the Great Plains, USA (Haas et al., 1957). Before agriculture start, the Great Plains soils had a wide range of texture classes (silt loam, loam, clay loam and very fine sandy loam), an initial organic C content of 1.13 %–2.47 % and a total N content of 0.05 %–0.22 %. Nonetheless, the total N range narrowed to 0.03 %–0.07 % over 45 years of intensive agriculture. As Haas et al. (1957) anticipated, all soils may finally reach a similar value for total N (i.e. the attractor for N) by continuing the ongoing management (in line with Australian and South African soils). (c) Infiltration rates as a function of years since land-use change from forest to agriculture (Nyberg et al., 2012). Note the narrowing trend (blue arrows) from forest (t=0) toward long-term cultivation (t=39, 57, 69 and 119 years since conversion). The value at ca. 120 years is defined as the attractor of the infiltration rate, and 120 years is the time needed to reach that attractor. (d) Narrowing content (blue arrows) of exchangeable Ca2+ and Mg2+ in the first 15 cm of Oxisols during 31 years (1978–2009) of sugar cane cultivation (Morrison and Gawander, 2016). The three soils developed under various natural vegetation prior to cultivation and received different management thereafter. (e) Narrow ranges of C:N ratios in all texture classes (sand, silt and clay) over 85 years of cultivation (Eleftheriadis et al., 2018). Note that the different rates of C:N decrease in the three fractions. That ratio in the sand fraction is more susceptible to cultivation duration but is rather resistant in the clay fraction. (f) Dependence of the soil quality index on duration and intensity of soil cultivation (on the x axis: 1 – virgin land, 2 – idle land in the modern era, 3 – modern-day ploughed land, 4 – post-antique idle land – and 5 – continually ploughed land) over 220 to 800 years cultivation (Lisetskii et al., 2015). Note that soil quality became similar (blue arrows) with increasing cultivation duration and/or cultivation intensity (from 1 to 5; value in red circle is an outlier).
Agropedogenesis clearly shows that the natural sequence is changed by humans: functions are no longer the final step in this sequence because one function becomes a factor (Fig. 2). This is because humans tailor the processes of soil development for the main function of agricultural soils – crop production. Based on the example of agropedogenesis, we conclude that all types of anthropedogenesis are directed at the functions that humans desire from the soil; hence, the one function becomes the factor of soil development (Fig. 2).
2.2 Attractors of soil degradation: definitions and concept
Despite a very broad range of individual properties of natural soils, long-term intensive agricultural land use strongly narrows their range (Homburg and Sandor, 2011; Kozlovskii, 1999; Sandor et al., 2008) and ultimately brings individual properties to the so-called attractors of degradation (Kozlovskii, 1999):
-
An attractor of a soil property is a numerical value toward which the property develops from a wide variety of initial or intermediate states of pedogenesis.
-
An attractor of agricultural soil degradation is a minimal or maximal value, toward which the property tends to develop by long-term intensive agricultural use from a wide variety of initial conditions common for natural soils.
Attractors of soil properties are common for natural pedogenesis and anthropedogenesis (Fig. 1). The well-known examples of natural pedogenic attractors are the maximal SOM accumulation (C≈5 %–6 % for mineral soils), the highest increase in clay content in the Bt horizon by a ∼ 2-fold illuviation compared to the upper horizon (without lithological discontinuity), the upper depth of the Bt horizon for sheet erosion, a minimal bulk density of mineral soils of ∼0.8 g cm−3, and the maximal weathering in wet tropics by removal of all minerals until only Fe and Al oxides remain (Chadwick and Chorover, 2001).
Natural pedogenesis leads to a divergence of pedogenic properties and consequently to the broadening of the multidimensional attractor space (see below) because various soils develop to steady state from the same parent materials depending on climate, organisms and relief (Fig. 1). The time necessary for natural processes to reach these attractors is at least 1–2 orders of magnitude longer than the periods to reach the attractors of agropedogenesis (see below).
In contrast to natural pedogenesis, agropedogenesis narrows the soil properties by optimizing environmental conditions for agricultural crops with similar requirements (Lo Papa et al., 2011, 2013). Consequently, each soil property follows a trajectory from a specific natural level toward the unified agrogenic attractor (Fig. 1). Therefore, in contrast to natural pedogenesis resulting in divergence of soil properties, agropedogenesis leads to convergence of soil properties.
2.3 Examples of attractors of soil degradation
The convergence in soil properties (and thus reaching an attractor) after starting in various initial states is evident by comparing soils under long-term (e.g. decades and centuries) cultivation (Sandor and Homburg, 2017). The challenges that ancient farmers faced were fundamentally the same as today, although recent decades are characterized by a major intensification of chemical impacts (fertilization and pesticides) and heavy machinery (Dudal, 2004; Sandor and Homburg, 2017). The main difference between soil degradation in the past and in the modern era is the rates and extent but not the processes or mechanisms themselves. The dynamics of soil properties in long-term cultivations have revealed a narrowing in the measured values of a given property over time, i.e. a tendency toward the attractor of that property (Alletto and Coquet, 2009; Dalal and Mayer, 1986a, c; Haas et al., 1957; Nyberg et al., 2012; Figs. 3, 4, 5, and S2).
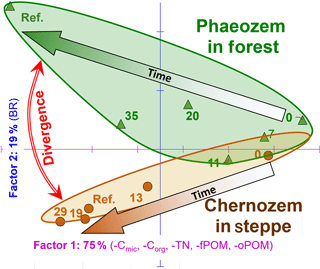
Figure 4Example of the divergence of soil properties of abandoned agriculturally used Chernozem (under steppe) and Phaeozem (under forest) after termination of cultivation (Ovsepyan et al., 2019, modified). The soil properties were analysed by principal component analysis (PCA). The soils had very similar properties due to long-term (>100 years) cropping (time point “0”). After abandonment, they started to develop to their natural analogues (Ref.: natural reference soils), leading to strong divergences of their properties. This figure reflects the divergence by natural pedogenesis, i.e. the opposite situation to agropedogenesis. Numbers close to points: duration of abandonment, 0, is agricultural soil, and Ref. is natural analogues (never cultivated under natural vegetation). The soil parameters primarily driving the divergence are on the x axis: microbial biomass C (Cmic), soil organic C (Corg), total N (TN), free particulate organic matter (fPOM) and occluded organic matter (oPOM). On the y axis is basal respiration (BR). For details, see Ovsepyan et al., 2019.
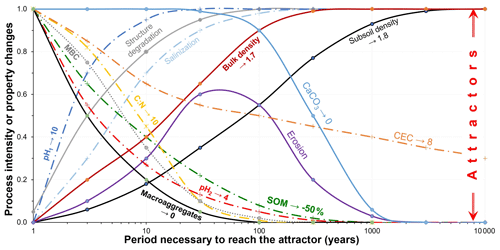
Figure 5Overview on rates of key processes of agropedogenesis and their trajectory in reaching their attractors. Curves start from 0 or 1 (relative values) at the onset of cultivation and go to 1 or 0 for the specific attractors. Each curve is labelled with the specific property. Small arrows after each parameter title show the estimated level of attractor in absolute values. After approaching its attractor, each process slows down and finally stops. The timescale is logarithmic. Curve shape, time to reach attractor and attractor levels are only estimates and require future adjustment based on experimental data. pH1 is for alkaline, and pH2 is for acidic soils. Note that not all attractors are defined yet. Properties in bold are master soil properties for agropedogenesis (see Table 3). MBC is microbial biomass carbon, SOM is soil organic matter and CEC is cation exchange capacity. Continuous lines present physical properties or processes. Dotted–dashed lines correspond to chemical properties, and dotted lines correspond to biological properties.
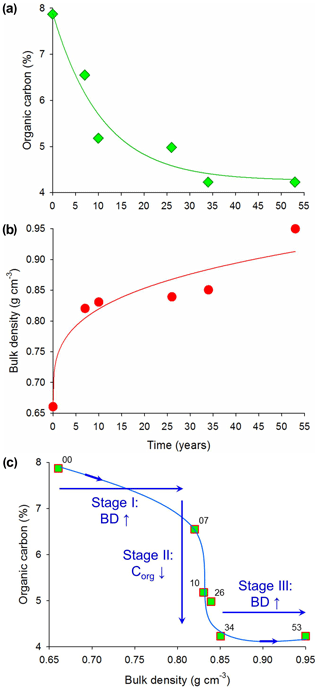
Figure 6Effects of duration of forest conversion to cropland on decreasing soil organic carbon (SOC) (a) and increasing bulk density (b) over 53 years (southern Ethiopian Highlands; Lemenih et al., 2005). (c) Phase diagram: relation between SOC and bulk density at corresponding time. Note the stepwise changes in bulk density following decreasing SOC content below the thresholds of 7.8 %, 6.5 % and 4.2 %. Numbers beside symbols refer to years after conversion.
In reaching the attractor values, however, the process rates and dynamics differ among various soil properties (Figs. 5 and 6), in various geo-climatological regions (Chen et al., 2011, p. 29 011; Guillaume et al., 2016b; Hartemink, 2006) and according to land-use intensity. For example, microbial biomass carbon (C) (Henrot and Robertson, 1994) and aggregate stability (Wei et al., 2014) respond faster than SOM and total N to cultivation. Cultivation affects total N and P content less than organic C because of N and P fertilization (Guillaume et al., 2016a), whereby a strong decrease in C input is inferred by the decreasing C:N ratio with cultivation duration (Wei et al., 2014). Whereas cultivation on deforested lands in the tropics can degrade soils within a few years, converting temperate prairies and steppes to agricultural fields supports crop production without fertilization for decades (Tiessen et al., 1994). Generally, the degradation rates (e.g. C losses) in the moist tropics are faster (e.g. about 4-fold faster) than in the dry tropics (Hall et al., 2013). Despite the differences in rates, however, the long-term cultivated soils ultimately reach similar degradation levels (Lisetskii et al., 2015; Fig. 3f).
2.4 Master soil properties
Soils and their functions are characterized by and are dependent on the full range of physical, chemical and biological properties. A few of them – the master soil properties – however, are responsible for a very broad range of functions and define other properties (Lincoln et al., 2014; Lisetskii et al., 2013; Seybold et al., 1997). We define a soil property as being a master property if it has a strong effect on a broad range of other properties and functions and if it cannot be easily assessed based on the other properties. For natural pedogenesis, such master properties – inherited partially from the parent material – are clay mineralogy and CaCO3 content, texture, nutrient content, and bulk density. The master properties that are cumulated or formed during pedogenesis are soil aggregation and structure, depth of A and B horizons, SOM stock and C:N ratio, pH, electrical conductivity (EC), etc. (Table 3). These properties largely define the other properties and soil functions under natural conditions and generally under agricultural use as well.
Table 3Soil properties suggested in the literature and in agropedogenesis theory as being master properties
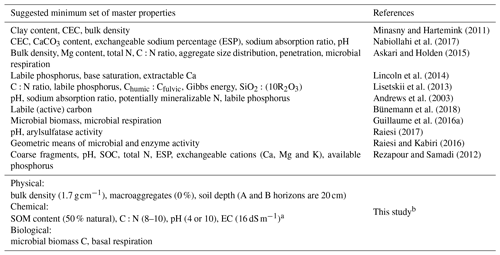
a CEC has been omitted from chemical master properties because it depends on
(i) clay content and clay mineralogy, whose properties are resistant to
agricultural practices, and (ii) SOM, which is considered a master property.
b The values in brackets are very preliminary attractors of each property
by anthropogenic soil degradation. The two pH attractors are presented for
acidic (humid climate) and alkaline (semiarid climate) soils. Note that not
all attractors can be suggested in this study. The criteria for selecting
master soil properties are described in the text.
The master properties of agropedogenesis may differ from those of natural soil development. The crucial difference is that the master properties of agropedogenesis must sensitively respond to agricultural use over the cultivation period. Accordingly, properties such as texture, clay content and mineralogy – crucial master properties of natural pedogenesis, are not relevant in agropedogenesis. Note that although these properties may change under certain circumstances (Karathanasis and Wells, 1989; Velde and Peck, 2002), they fail to qualify as master properties in agropedogenesis because they are relatively insensitive to agricultural land use and soil degradation.
Master soil properties have an additional important function: they are responsible or co-responsible for the changes in other properties. Changes in a master property over time may therefore intensify or dampen changes in other (secondary) properties. The stability of macroaggregates, for example, increases with the content and quality of SOM (Boix-Fayos et al., 2001; Celik, 2005). The infiltration rate and water-holding capacity decrease with increasing bulk density (Rasa and Horn, 2013; Raty et al., 2010), promoting erosion. These relations between soil properties, however, seem to be significant only within certain ranges, i.e. until thresholds are reached. Beyond such thresholds, new relations or new master properties may govern. For example, an increasing effect of SOM content on aggregate stability in extremely arid regions of the Mediterranean was recorded at above the 5 % SOM content threshold (Boix-Fayos et al., 2001). Increasing organic matter contents up to this 5 % threshold had no effect on aggregate stability: instead, the carbonate content was the main regulator (Boix-Fayos et al., 2001). Microbial biomass and respiration in well-drained Acrisols in Indonesia are resistant to decreasing SOM down to 2.7 % of SOM but strongly dropped beyond that value (Guillaume et al., 2016a). While the amounts of SOM and total N in sand and silt fractions may continuously decrease with cultivation duration, those values in the clay fraction remain stable (Eleftheriadis et al., 2018; Fig. 3e). Bulk density increases non-linearly with SOM decrease, and the rates depend on SOM content (Fig. 6). Phase diagrams are very useful in identifying such thresholds (see below).
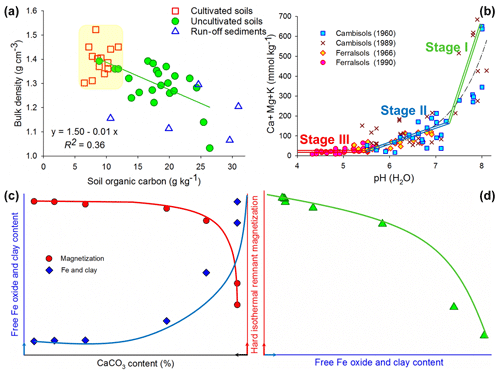
Figure 7Phase diagrams of various properties of agricultural soils. Small arrows at the start or end of the axes show the increase in the corresponding soil property. (a) Narrow range (yellow shaded area) of organic carbon and bulk density in ancient agricultural soils cultivated for 1500 years at Mimbres (New Mexico, USA) compared to uncultivated soils and run-off sediments (Sandor et al., 2008). Note that the decreasing trend of bulk density with increasing soil organic carbon content (green line with regression equation for uncultivated soils) is absent in cultivated soils (Sandor et al., 2008). (b) Changes in exchangeable base cations depending on soil pH in Cambisols and Ferralsols in coastal plains of Tanzania (Hartemink and Bridges, 1995). Ferralsols clearly decline in exchangeable cations (i.e. two separated groups in stage II and III) with decreasing pH over ca. 24 years of cultivation. The exchangeable cations in Cambisols remain in stage I. Double lines: stages of exchangeable cation decrease with decreasing soil pH. Content of exchangeable cations levels off at ∼25 (stage III). This value – which corresponds to the amount of exchangeable Ca2+ and Mg2+ shown in Fig. 3d (31 years of sugar cane cultivation on Fijian Ferralsols) – is an attractor. (c) The content of free iron oxides, clay content and hard isothermal remnant magnetization (IRMh) as a function of CaCO3 content in soil (adopted from Chen et al., 2011). (d) The relation between IRMh vs. free iron oxides and clay content.
Summarizing, we define master properties as a group of soil-fertility-related parameters that (1) are directly affected by management, i.e. are sensitive to agricultural use and soil degradation, (2) determine the state of many other (non-master) parameters and soil fertility indicators during agropedogenesis, and (3) should be orthogonal to each other, i.e. independent (or minimally dependent) of one other (Kozlovskii, 1999). Note that, in reality, all soil properties are at least partially dependent on each other. Nonetheless, the last prerequisite – orthogonality – ensures the best separation of soils in multidimensional space (see below) and reduces the redundancy of the properties.
Considering the three prerequisites and based on expert knowledge, as well as on phase diagrams (see below), we suggest soil depth (A and B horizons) and eight properties as being master properties (Table 3): density, macroaggregates, SOM, the C:N ratio, pH, EC, microbial biomass C and basal respiration. We consider these nine to be sufficient to describe the degradation state of most other parameters during agropedogenesis: water permeability, penetration resistance, erodibility, base saturation, exchangeable sodium percentage, sodium absorption ratio, N mineralization, availability of other nutrients, etc.
The combination of master properties provides a minimum dataset to determine soil development stages with cultivation duration (Andrews et al., 2002). Organic C content is the most important and universally accepted master property that directly and indirectly determines the state of many physical (soil structure, density, porosity, water-holding capacity, percolation rate and erodibility; Andrews et al., 2003; Nabiollahi et al., 2017; Seybold et al., 1997; Shpedt et al., 2017), chemical (nutrient availability, sorption capacity and pH; Lal, 2006; Minasny and Hartemink, 2011) and biological (biodiversity, microbial biomass and basal respiration; Raiesi, 2017) properties. The values of the mentioned secondary properties can be estimated with an acceptable uncertainty based on robust data on SOM content (Gharahi Ghehi et al., 2012). Finding additional soil properties beyond SOM to form the set of master properties is, however, not straightforward (Homburg et al., 2005) because it depends on the desired soil functions (Andrews et al., 2003) such as nutrient availability, water permeability and holding capacity, crop yield quantity and quality, etc. (Andrews et al., 2002). Therefore, various types of master properties, depending on geo-climatological conditions (Cannell and Hawes, 1994), have already been suggested (Table 3). Nonetheless, the dynamics, sensitivity and resistance of such properties to degradation and with cultivation duration remain unknown (Guillaume et al., 2016a).
2.5 Analysis of phase diagrams and identification of thresholds and stages of soil degradation
All the properties described above move toward their attractors over the course of soil degradation with time (Figs. 3 and 6). The duration, however, is difficult to compare between soils because the process rates depend on climatic conditions and land-use intensity. One option for understanding and analysing soil degradation independent of time is to use phase diagrams. Generally, a phase diagram is a type of chart for showing the state and simultaneous development of two or more parameters of a matter1. Phase diagrams present (and then analyse) properties against each other without the time factor (Figs. 6c and 7). Thus, various properties measured in a chronosequence of soil degradation are related to each other on 2-D or even 3-D graphs (Fig. 8), and time is excluded.
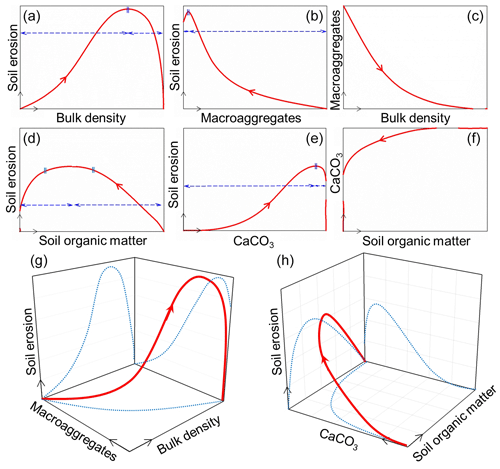
Figure 8Examples of conceptual 2-D and 3-D phase diagrams linking soil erosion intensity with (a, b, g) bulk density and macroaggregate content and (d, e, h) SOM and CaCO3 contents during agropedogenesis. The original curves were taken from Fig. 6. Small red arrows on curved lines show the direction of soil degradation and correspond to the increasing duration or intensity of agricultural use. Vertical blue double lines show the arbitrary thresholds of soil degradation, and horizontal blue dashed arrows show the degradation stages. The stages are time-lapse to reach a threshold for a given soil property. After a threshold the trend may slow down or reverse. Projections of 3-D lines (light blue) in (g, h) correspond to the individual lines on the 2-D phase diagrams in (a)–(c) and (d)–(f). Similar phase diagrams can be built in multidimensional space corresponding to the number of master soil properties (Table 3).
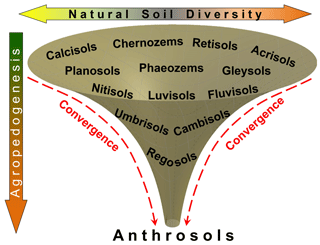
Figure 9Conceptual schema of convergence of soil properties by agropedogenesis. The very broad range of natural soils and their properties will be tailored for crop production by agricultural use, resulting in Anthrosols with a very narrow range of properties. Note that the soils within the funnel are mentioned exemplarily, and not all World Reference Base (WRB) soil groups are presented. The sequence of soils within the funnel does not reflect their transformations during agropedogenesis to Anthrosols. (The extended version of this figure, reflecting multiple pathways to Anthrosols, e.g. formed and used under completely different climate and management conditions, is presented in Fig. S3.)
Phase diagrams have two advantages: (1) they help evaluate the dependence of properties on each other – independent of time, climate or management intensity. They represent generalized connection between the properties. This greatly simplifies comparing the trajectory of soil degradation under various climatic conditions, management intensity levels and even various land uses. (2) Such diagrams enable identifying the thresholds and stages of soil development and degradation.
We define these terms as follows.
-
Thresholds of soil development and degradation are relatively abrupt changes in process rates or process directions leading to a switch in the dominating mechanism of soil degradation.
-
Stages of soil degradation are periods confined by two thresholds and characterized by one dominating degradation mechanism (Fig. 6c).
Importantly, soil degradation does not always follow a linear or exponential trajectory (Kozlovskii, 1999; Kozlovskii and Goryachkin, 1996). This means that changes (absolute for linear or relative for exponential) are not proportional to time or management intensity (Kozlovskii and Goryachkin, 1996; Matus and Egli, 2019). Soil degradation proceeds in stages of various levels of duration and intensity. The key consideration, however, is that each stage is characterized by the dominance of one (group) of degradation processes, whose prerequisites are formed in the previous phase.
We conclude that phase diagrams (1) enable tracing the trajectory of various soil properties as they reach their attractors, independent of time, land-use or management intensity, and (2) are useful for analysing not only the dependence (or at least correlation) between individual properties but also for identifying the thresholds of soil degradation. The thresholds clearly show that soil degradation proceeds in stages (Figs. 6c, 7 and 8), each of which is characterized by the dominance of one specific degradation process with its specific rates (and affecting the degradation of related soil properties).
2.6 Multi-dimensional attractor space
The phase diagrams described above were presented in 2-D or 3-D space (Figs. 7 and 8) and help in evaluating the connections between the properties and the stages of soil degradation. The suggested nine master soil properties are orthogonal, and the phase diagrams can therefore be built in multidimensional attractor space – the space defining the soil degradation trajectory based on the master soil properties (Fig. 8g and h). Therefore, development of master soil properties during long-term agricultural land use and degradation forms a multidimensional space of properties (multidimensional space) toward which the soil will develop (trajectory) during agropedogenesis and will then remain unchanged within this equilibrium field. Accordingly, the multidimensional space of attractors defines the final stage of agropedogenesis.
The degraded soil will remain within this multidimensional space even if subsequently slightly disturbed (or reclaimed). This explains why long-term agricultural fields that have been abandoned for centuries or even millennia still show evidence of soil degradation (Hall et al., 2013; Jangid et al., 2011; Kalinina et al., 2013; Lisetskii et al., 2013; Ovsepyan et al., 2019; Sandor et al., 2008). For example, abandoned soils under succession of local vegetation such as grassland and forest show similar physicochemical and biological properties as a result of similarities in their history, i.e. agricultural land use (Jangid et al., 2011; Kalinina et al., 2019; Kurganova et al., 2019; Ovsepyan et al., 2019). The flood-irrigated soils in Cave Creek, Arizona, support only the growth of the creosote bush even about 700 years after abandonment. This contrasts with the presence of seven species of shrubs and cacti in areas between such soils. The reason is substantial changes in soil texture, i.e. via siltation, thus reducing the water-holding capacity in the flood-irrigated soils and leading to a shift in the vegetation community toward more drought-resistant species, in this case the creosote bush (Hall et al., 2013). Whereas establishing a no-till system on former pasture-land leads to a decrease in SOM, changing a formerly ploughed land to no-till had no such effect (Francis and Knight, 1993). The amidase activity in Colca soils, Peru, is still high 400 years after land abandonment due to the remaining effect of applied organic amendments on microorganisms (Dick et al., 1994). We argue that during agropedogenesis the multidimensional space of master soil properties will continuously narrow when approaching the attractors. This multidimensional space resembles a funnel (Fig. 9), meaning that the broad range of all properties in initial natural soils will be narrowed and unified to a (very) small range in agricultural and subsequently degraded soils. Identifying the attractors of master properties and the relations among them in this multidimensional space yields diagnostic characteristics for identifying and classifying agrogenic soils (Gerasimov, 1984; Kozlovskii, 1999).
2.7 Changes in the attractors by specific land use or climatic conditions
Despite the principle of attractors – the convergence of a property of various soils to one value by degradation – we assume that these attractors may differ slightly depending on climate, parent material and management (Fig. S3). This means that the multidimensional attractor space can exhibit some local minima – metastable states (Kozlovskii, 1999). If the initial natural soil is close to such a minimum, or the management pushes the trajectory in such a direction, then agropedogenesis may stop at local minima. Thus, the global minimum will not be reached.
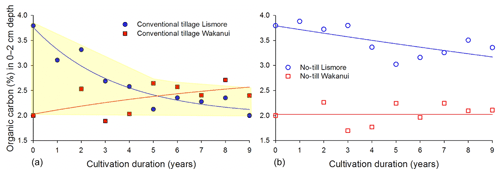
Figure 10Nine years of continuous cropping and conventional tillage (a) led to similar soil organic carbon (SOC) contents in contrast to no-till soils (b) (Francis and Knight, 1993). The Lismore no-till soil either needs longer cultivation duration to reach the C content characterizing soils under conventional tillage or the attractor of SOC has already been reached, i.e. local minima for this soil. Note that the Wakanui no-till soil was cultivated for 10 years before beginning the trial and thus shows similar values, i.e. similar attractor for SOC as under conventional tillage. Thus, changing the conventional tillage to no-till had no effect on SOC content. Lismore soil is an Umbric Dystochrept, containing 5 % stones, with rapid draining, which was cultivated for 5 years under mixed rye grass and white clover pasture. Wakanui soil is an Udic Ustochrept, with slow draining, and 10-year rotation of wheat, barley and peas.
For example, no-till farming may increase SOM in the Ap horizon (Lal, 1997) and cause SOM contents to level off at higher values compared to tillage practices (Fig. 10). However, periodically tilling the soil to simplify weed control quickly destroys the improvements in soil properties during the no-till period (Cannell and Hawes, 1994). This results in degradation stages similar to soils under conventional tillage. The ultimate effect of irrigation on soil degradation is expected to be similar to that of dry-land farming. Despite more organic C input into irrigated systems, the SOM content remains unchanged (Trost et al., 2014) due to accelerated decomposition (Denef et al., 2008). The state of soil properties in the tropics is predictable based on pedotransfer functions commonly used in temperate regions, which, even though tropical soils are usually more clayey, have a lower available water capacity and exhibit a higher bulk density. The explanation lies in the similarities in relations among soil properties under various climatic conditions (Minasny and Hartemink, 2011). This makes the concept of attractors generalizable to all cultivated soils (Kozlovskii, 1999), although geo-climatic conditions and specific management may modify the attractor values and affect the rates of soil degradation following cultivation (Tiessen et al., 1994).
3.1 Conclusions
We state that (1) human activities are stronger in intensity and rates than all other soil-forming factors (Liu et al., 2009; Richter et al., 2015). Because humans exploit mainly one soil function – crop production – they optimize all soil processes and properties toward a higher yield of a few agricultural crops. Because most crops have similar requirements, the range of measured values for any soil property becomes narrower during agropedogenesis. Therefore, human activities for crop production lead to the formation of a special group of agrogenic soils with a defined and narrow range of properties – Anthrosols. The range of properties moves toward the attractor: specific for each property but similar for various soils. (2) Analysing the properties of soils from various geo-climatological conditions and types of management in relation to cultivation periods reveals (i) the dynamics of soil properties by agropedogenesis and (ii) demonstrates the final stage of agrogenic degradation when the values of various soil properties reach the attractor.
By analysing the soil development and the properties' dynamics under agricultural use, we develop for the first time the basic theory of agropedogenesis. This theory is based on (1) the modified classical concept of and back to the processes, (2) the concept of attractors of soil degradation, (3) identifying master soil properties and analysing their dynamics by agropedogenesis, (4) analysing phase diagrams of master soil properties to identify the thresholds and stages of soil degradation, and finally (5) defining multidimensional attractor space. We defined the attractors and provided the basic prerequisites for elucidating the nine master properties responsible for the trajectory of any soil during agropedogenesis within multidimensional attractor space.
3.2 Outlook
We developed a new unifying theory of agropedogenesis based on the long observation of soil degradation under agricultural use and on experiments with agricultural soils under various land-use intensity under a broad range of climatic conditions. The presented examples of soil degradation trajectories and of attractors of soil properties clearly do not reflect the full range of situations. This theory therefore needs to be filled with more observational and experimental data. Various emerging topics can be highlighted.
-
Confirmation of master soil properties. The master properties presented here represent suggested entities. This calls for clarifying whether these are sufficient (or excessive) to describe the stages of soil degradation under agropedogenesis. The degree of orthogonality of these properties also remains to be determined. Defining the master soil properties and their multidimensional attractor space will clearly simplify the modelling of degradation trajectories.
-
Identification of attractor values. The suggested attractor values (Figs. 3, 6 and 7b; Table 3) are mainly based on a few chronosequence studies and expert knowledge. These values should be defined more precisely based on a larger database. The challenge here is that the average values are not suitable as attractors because only the maximal or minimal values – the attractors – of a variable are of interest. Therefore, specific statistical methods should be applied, e.g. the lower (or upper – depending on the property) 95 % confidence interval or envelope testing should be used instead of means to set the attractor value.
-
The determination of local minima. The determination of local minima is necessary (and is closely connected with the identification of the multidimensional attractor space). Arriving at such local minima will temporarily stop soil degradation, and knowing their values can help simplify the measures to combat degradation and accelerate soil recovery.
-
Threshold investigation. Investigating the thresholds and stages of soil degradation, along with identifying the main mechanisms dominating at each stage, should be done based on the phase diagrams of various soil properties – at least those of the master properties. These stages of agropedogenesis with their corresponding main mechanisms are crucial for understanding, modelling and combating soil degradation.
-
Model development. Only a few models of natural pedogenesis in its full complexity are available (Finke, 2012; Finke and Hutson, 2008; Keyvanshokouhi et al., 2016), and the models addressing soil degradation describe more or less individual or a selected few processes but not overall agropedogenesis. For example, various models are available for erosion (Afshar et al., 2018; Arekhi et al., 2012; Ebrahimzadeh et al., 2018; Millward and Mersey, 1999; Morgan et al., 1998; Pournader et al., 2018; Rose et al., 1983), SOM decrease (Chertov and Komarov, 1997; Davidson et al., 2012; Del Grosso et al., 2002; Grant, 1997; Liu et al., 2003; Smith et al., 1997), density increase (Hernanz et al., 2000; Jalabert et al., 2010; Makovnikova et al., 2017; Shiri et al., 2017; Taalab et al., 2013; Tranter et al., 2007) and other processes due to land use. This calls for complex theory-based models of agropedogenesis.
No data sets were used in this article.
The supplement related to this article is available online at: https://doi.org/10.5194/bg-16-4783-2019-supplement.
YK and KZ contributed equally to writing the paper.
The authors declare that they have no conflict of interest.
This paper is devoted to the 90th anniversary of Felix I. Kozlovskii – eminent pedologist and geo-ecologist, who introduced the theory of agropedogenesis more than 30 years ago and was the first to suggest the concept of attractors of soil degradation. We are very thankful to Sergey Goryachkin for very helpful critical suggestions to the first version of the concept.
This open-access publication was funded by the University of Göttingen.
This paper was edited by Jianming Xu and reviewed by Rouholla Taghizade and one anonymous referee.
Afshar, E., Yarnia, M., Bagherzadeh, A., Mirshekari, B., and Haghighi, R. S.: The Effect of Crops Cultivation on Soil Erosion Indices Based on Impelero Model in Northeast Iran, Appl. Ecol. Environ. Res., 16, 855–866, https://doi.org/10.15666/aeer/1601_855866, 2018.
Alletto, L. and Coquet, Y.: Temporal and spatial variability of soil bulk density and near-saturated hydraulic conductivity under two contrasted tillage management systems, Geoderma, 152, 85–94, https://doi.org/10.1016/j.geoderma.2009.05.023, 2009.
Amundson, R. and Jenny, H.: The Place of Humans in the State Factor Theory of Ecosystems and Their Soils, Soil Sci., 151, 99–109, 1991.
Andrews, S. S., Karlen, D. L., and Mitchell, J. P.: A comparison of soil quality indexing methods for vegetable production systems in Northern California, Agric. Ecosyst. Environ., 90, 25–45, https://doi.org/10.1016/S0167-8809(01)00174-8, 2002.
Andrews, S. S., Flora, C. B., Mitchell, J. P., and Karlen, D. L.: Growers' perceptions and acceptance of soil quality indices, Geoderma, 114, 187–213, https://doi.org/10.1016/S0016-7061(03)00041-7, 2003.
Arekhi, S., Niazi, Y., and Kalteh, A. M.: Soil erosion and sediment yield modeling using RS and GIS techniques: a case study, Iran, Arab. J. Geosci., 5, 285–296, https://doi.org/10.1007/s12517-010-0220-4, 2012.
Askari, M. S. and Holden, N. M.: Quantitative soil quality indexing of temperate arable management systems, Soil Tillage Res., 150, 57–67, https://doi.org/10.1016/j.still.2015.01.010, 2015.
Ayoubi, S., Mokhtari Karchegani, P., Mosaddeghi, M. R., and Honarjoo, N.: Soil aggregation and organic carbon as affected by topography and land use change in western Iran, Soil Tillage Res., 121, 18–26, https://doi.org/10.1016/j.still.2012.01.011, 2012.
Bidwell, O. W. and Hole, F. D.: Man as a factor of soil formation, Soil Sci., 99, 65–72, 1965.
Boix-Fayos, C., Calvo-Cases, A., Imeson, A. C., and Soriano-Soto, M. D.: Influence of soil properties on the aggregation of some Mediterranean soils and the use of aggregate size and stability as land degradation indicators, Catena, 44, 47–67, https://doi.org/10.1016/S0341-8162(00)00176-4, 2001.
Bosch-Serra, A. D., Padro, R., Boixadera-Bosch, R. R., Orobitg, J., and Yaguee, M. R.: Tillage and slurry over-fertilization affect oribatid mite communities in a semiarid Mediterranean environment, Appl. Soil Ecol., 84, 124–139, https://doi.org/10.1016/j.apsoil.2014.06.010, 2014.
Breland, T. A. and Eltun, R.: Soil microbial biomass and mineralization of carbon and nitrogen in ecological, integrated and conventional forage and arable cropping systems, Biol. Fertil. Soils, 30, 193–201, https://doi.org/10.1007/s003740050608, 1999.
Bryant, R. B. and Galbraith, J. M.: Incorporating Anthropogenic Processes in Soil Classification, in: Soil Classification: A Global Desk Reference, edited by: Eswaran, H., Ahrens, R., Rice, T. J., and Stewart, B. A., CRC Press, Boca Raton, FL, 2003.
Bünemann, E. K., Bongiorno, G., Bai, Z., Creamer, R. E., De Deyn, G., de Goede, R., Fleskens, L., Geissen, V., Kuyper, T. W., Mäder, P., Pulleman, M., Sukkel, W., van Groenigen, J. W., and Brussaard, L.: Soil quality – A critical review, Soil Biol. Biochem., 120, 105–125, https://doi.org/10.1016/j.soilbio.2018.01.030, 2018.
Burghardt, W., Heintz, D., and Hocke, N.: Soil Fertility Characteristics and Organic Carbon Stock in Soils of Vegetable Gardens Compared with Surrounding Arable Land at the Center of the Urban and Industrial Area of Ruhr, Germany, Eurasian Soil Sci., 51, 1067–1079, https://doi.org/10.1134/S106422931809003X, 2018.
Cannell, R. Q. and Hawes, J. D.: Trends in tillage practices in relation to sustainable crop production with special reference to temperate climates, Soil Tillage Res., 30, 245–282, https://doi.org/10.1016/0167-1987(94)90007-8, 1994.
Carducci, C. E., Zinn, Y. L., Rossoni, D. F., Heck, R. J., and Oliveira, G. C.: Visual analysis and X-ray computed tomography for assessing the spatial variability of soil structure in a cultivated Oxisol, Soil Tillage Res., 173, 15–23, https://doi.org/10.1016/j.still.2016.03.006, 2017.
Carozza, J.-M., Galop, D., Metailie, J.-P., Vanniere, B., Bossuet, G., Monna, F., Lopez-Saez, J. A., Arnauld, M.-C., Breuil, V., Forne, M., and Lemonnier, E.: Landuse and soil degradation in the southern Maya lowlands, from Pre-Classic to Post-Classic times: The case of La Joyanca (Petén, Guatemala), Geodin. Acta, 20, 195–207, https://doi.org/10.3166/ga.20.195-207, 2007.
Celik, I.: Land-use effects on organic matter and physical properties of soil in a southern Mediterranean highland of Turkey, Soil Tillage Res., 83, 270–277, https://doi.org/10.1016/j.still.2004.08.001, 2005.
Chadwick, O. A. and Chorover, J.: The chemistry of pedogenic thresholds, Geoderma, 100, 321–353, https://doi.org/10.1016/S0016-7061(01)00027-1, 2001.
Chen, L.-M., Zhang, G.-L., and Effland, W. R.: Soil Characteristic Response Times and Pedogenic Thresholds during the 1000-Year Evolution of a Paddy Soil Chronosequence, Soil Sci. Soc. Am. J., 75, 1807–1820, https://doi.org/10.2136/sssaj2011.0006, 2011.
Cheng, Y.-Q., Yang, L.-Z., Cao, Z.-H., Ci, E., and Yin, S.: Chronosequential changes of selected pedogenic properties in paddy soils as compared with non-paddy soils, Geoderma, 151, 31–41, https://doi.org/10.1016/j.geoderma.2009.03.016, 2009.
Chertov, O. G. and Komarov, A. S.: SOMM: A model of soil organic matter dynamics, Ecol. Model., 94, 177–189, https://doi.org/10.1016/S0304-3800(96)00017-8, 1997.
Dalal, R. and Mayer, J. R.: Long term trends in fertility of soils under continuous cultivation and cereal cropping in southern Queensland. II. Total organic carbon and its rate of loss from the soil profile, Aust. J. Soil Res., 24, 281–292, https://doi.org/10.1071/SR9860281, 1986a.
Dalal, R. and Mayer, R.: Long-term trends in fertility of soils under continuous cultivation and cereal cropping in southern Queensland. IV. Loss of organic carbon for different density functions, Aust. J. Soil Res., 24, 281–292, https://doi.org/10.1071/SR9860301, 1986b.
Dalal, R. C. and Mayer, R. J.: Long term trends in fertility of soils under continuous cultivation and cereal cropping in southern Queensland .V. Rate of loss of total nitrogen from the soil profile and changes in carbon?: nitrogen ratios, Aust. J. Soil Res., 24, 493–504, https://doi.org/10.1071/sr9860493, 1986c.
Davidson, E. A., Samanta, S., Caramori, S. S., and Savage, K.: The Dual Arrhenius and Michaelis-Menten kinetics model for decomposition of soil organic matter at hourly to seasonal time scales, Glob. Change Biol., 18, 371–384, https://doi.org/10.1111/j.1365-2486.2011.02546.x, 2012.
Dazzi, C. and Monteleone, S.: Anthropogenic processes in the evolution of a soil chronosequence on marly-limestone substrata in an Italian Mediterranean environment, Geoderma, 141, 201–209, https://doi.org/10.1016/j.geoderma.2007.05.016, 2007.
Dehaan, R. L. and Taylor, G. R.: Field-derived spectra of salinized soils and vegetation as indicators of irrigation-induced soil salinization, Remote Sens. Environ., 80, 406–417, https://doi.org/10.1016/S0034-4257(01)00321-2, 2002.
Del Grosso, S., Ojima, D., Parton, W., Mosier, A., Peterson, G., and Schimel, D.: Simulated effects of dryland cropping intensification on soil organic matter and greenhouse gas exchanges using the DAYCENT ecosystem model, Environ. Pollut., 116, S75–S83, https://doi.org/10.1016/S0269-7491(01)00260-3, 2002.
DeLong, C., Cruse, R., and Wiener, J.: The Soil Degradation Paradox: Compromising Our Resources When We Need Them the Most, Sustainability, 7, 866–879, https://doi.org/10.3390/su7010866, 2015.
Denef, K., Stewart, C. E., Brenner, J., and Paustian, K.: Does long-term center-pivot irrigation increase soil carbon stocks in semi-arid agro-ecosystems?, Geoderma, 145, 121–129, https://doi.org/10.1016/j.geoderma.2008.03.002, 2008.
Diamond, J.: Evolution, consequences and future of plant and animal domestication, Nature, 418, 700–707, https://doi.org/10.1038/nature01019, 2002.
Dick, R. P., Sandor, J. A., and Eash, N. S.: Soil enzyme activities after 1500 years of terrace agriculture in the Colca Valley, Peru, Agric. Ecosyst. Environ., 50, 123–131, https://doi.org/10.1016/0167-8809(94)90131-7, 1994.
Diedhiou, A. G., Dupouey, J.-L., Buée, M., Dambrine, E., Laüt, L., and Garbaye, J.: Response of ectomycorrhizal communities to past Roman occupation in an oak forest, Soil Biol. Biochem., 41, 2206–2213, https://doi.org/10.1016/j.soilbio.2009.08.005, 2009.
Dokuchaev, V.: Russian Chernozem, Saint Petersburg, 1883.
Dudal, R.: The sixth factor of soil formation, available at: https://www.researchgate.net/publication/228669778_The_sixth_factor_of_soil_formation (last access: 5 October 2018), 2004.
Ebrahimzadeh, S., Motagh, M., Mahboub, V., and Harijani, F. M.: An improved RUSLE/SDR model for the evaluation of soil erosion, Environ. Earth Sci., 77, 454, https://doi.org/10.1007/s12665-018-7635-8, 2018.
Eleftheriadis, A., Lafuente, F., and Turrión, M.-B.: Effect of land use, time since deforestation and management on organic C and N in soil textural fractions, Soil Tillage Res., 183, 1–7, https://doi.org/10.1016/j.still.2018.05.012, 2018.
Ellis, S. and Newsome, D.: Chalkland soil formation and erosion on the Yorkshire Wolds, northern England, Geoderma, 48, 59–72, https://doi.org/10.1016/0016-7061(91)90006-F, 1991.
Emdad, M. R., Raine, S. R., Smith, R. J., and Fardad, H.: Effect of water quality on soil structure and infiltration under furrow irrigation, Irrig. Sci., 23, 55–60, https://doi.org/10.1007/s00271-004-0093-y, 2004.
Fageria, N. K.: Role of Soil Organic Matter in Maintaining Sustainability of Cropping Systems, Commun. Soil Sci. Plant Anal., 43, 2063–2113, https://doi.org/10.1080/00103624.2012.697234, 2012.
FAO: World Food and Agriculture: Statistical Pocketbook, FAO, Rome, 2018.
Finke, P. A.: Modeling the genesis of luvisols as a function of topographic position in loess parent material, Quat. Int., 265, 3–17, https://doi.org/10.1016/j.quaint.2011.10.016, 2012.
Finke, P. A. and Hutson, J. L.: Modelling soil genesis in calcareous loess, Geoderma, 145, 462–479, https://doi.org/10.1016/j.geoderma.2008.01.017, 2008.
Flynn, D. F. B., Gogol-Prokurat, M., Nogeire, T., Molinari, N., Richers, B. T., Lin, B. B., Simpson, N., Mayfield, M. M., and DeClerck, F.: Loss of functional diversity under land use intensification across multiple taxa, Ecol. Lett., 12, 22–33, https://doi.org/10.1111/j.1461-0248.2008.01255.x, 2009.
Francis, G. S. and Knight, T. L.: Long-term effects of conventional and no-tillage on selected soil properties and crop yields in Canterbury, New Zealand, Soil Tillage Res., 26, 193–210, https://doi.org/10.1016/0167-1987(93)90044-P, 1993.
Gerasimov, I.: The System of Basic Genetic Concepts That Should Be Included in Modern Dokuchayevian Soil Science, Sov. Geogr., 25, 1–14, 1984.
Gerasimov, I. P. and Fridland, V.: Historical role and current problems of genetical soil science of Dokuchaev, Pochvovedenie, 4, 5–10, 1984.
Gharahi Ghehi, N., Nemes, A., Verdoodt, A., Van Ranst, E., Cornelis, W., and Boeckx, P.: Nonparametric techniques for predicting soil bulk density of tropical rainforest topsoils in Rwanda, Soil Sci. Soc. Am. J., 76, 1172–1183, https://doi.org/10.2136/sssaj2011.0330, 2012.
Glaser, B., Haumaier, L., Guggenberger, G., and Zech, W.: The “Terra Preta” phenomenon: a model for sustainable agriculture in the humid tropics, Naturwissenschaften, 88, 37–41, https://doi.org/10.1007/s001140000193, 2001.
Glinka, K. D.: Dokuchaiev's ideas in the development of pedology and cognate sciences, The Academy, Leningrad, 1927.
Govers, G., Vandaele, K., Desmet, P., Poesen, J., and Bunte, K.: The Role of Tillage in Soil Redistribution on Hillslopes, Eur. J. Soil Sci., 45, 469–478, https://doi.org/10.1111/j.1365-2389.1994.tb00532.x, 1994.
Grant, R. F.: Changes in soil organic matter under different tillage and rotation: Mathematical modeling in ecosys, Soil Sci. Soc. Am. J., 61, 1159–1175, https://doi.org/10.2136/sssaj1997.03615995006100040023x, 1997.
Guillaume, T., Holtkamp, A. M., Damris, M., Brummer, B., and Kuzyakov, Y.: Soil degradation in oil palm and rubber plantations under land resource scarcity, Agric. Ecosyst. Environ., 232, 110–118, https://doi.org/10.1016/j.agee.2016.07.002, 2016a.
Guillaume, T., Maranguit, D., Murtilaksono, K., and Kuzyakov, Y.: Sensitivity and resistance of soil fertility indicators to land-use changes: New concept and examples from conversion of Indonesian rainforest to plantations, Ecol. Indic., 67, 49–57, https://doi.org/10.1016/j.ecolind.2016.02.039, 2016b.
Haas, H. J., Evans, C. E., and Miles, E. F.: Nitrogen and Carbon Changes in Great Plains Soils as Influenced by Cropping and Soil Treatments, U.S. Department of Agriculture, Washington D.C., 1957.
Hall, S. J., Trujillo, J., Nakase, D., Strawhacker, C., Kruse-Peeples, M., Schaafsma, H., and Briggs, J.: Legacies of Prehistoric Agricultural Practices Within Plant and Soil Properties Across an Arid Ecosystem, Ecosystems, 16, 1273–1293, https://doi.org/10.1007/s10021-013-9681-0, 2013.
Hartemink, A. E.: Assessing Soil Fertility Decline in the Tropics Using Soil Chemical Data, Advances in Agronomy, 89, 179–225, 2006.
Hartemink, A. E. and Bridges, E. M.: The influence of parent material on soil fertility degradation in the coastal plain of Tanzania, Land Degrad. Dev., 6, 215–221, https://doi.org/10.1002/ldr.3400060403, 1995.
Henrot, J. and Robertson, G. P.: Vegetation removal in two soils of the humid tropics: Effect on microbial biomass, Soil Biol. Biochem., 26, 111–116, https://doi.org/10.1016/0038-0717(94)90202-X, 1994.
Hernanz, J. L., Peixoto, H., Cerisola, C., and Sanchez-Giron, V.: An empirical model to predict soil bulk density profiles in field conditions using penetration resistance, moisture content and soil depth, J. Terramechanics, 37, 167–184, https://doi.org/10.1016/S0022-4898(99)00020-8, 2000.
Holthusen, D., Brandt, A. A., Reichert, J. M., and Horn, R.: Soil porosity, permeability and static and dynamic strength parameters under native forest/grassland compared to no-tillage cropping, Soil Tillage Res., 177, 113–124, https://doi.org/10.1016/j.still.2017.12.003, 2018.
Homburg, J. A. and Sandor, J. A.: Anthropogenic effects on soil quality of ancient agricultural systems of the American Southwest, Catena, 85, 144–154, https://doi.org/10.1016/j.catena.2010.08.005, 2011.
Homburg, J. A., Sandor, J. A., and Norton, J. B.: Anthropogenic influences on Zuni agricultural soils, Geoarchaeology Int. J., 20, 661–693, https://doi.org/10.1002/gea.20076, 2005.
Horn, R. and Fleige, H.: Risk assessment of subsoil compaction for arable soils in Northwest Germany at farm scale, Soil Tillage Res., 102, 201–208, https://doi.org/10.1016/j.still.2008.07.015, 2009.
Howard, J.: Anthropogenic Soils, Springer International Publishing, available at: https://www.springer.com/de/book/9783319543307 (last access: 22 April 2019), 2017.
Jalabert, S. S. M., Martin, M. P., Renaud, J.-P., Boulonne, L., Jolivet, C., Montanarella, L., and Arrouays, D.: Estimating forest soil bulk density using boosted regression modelling, Soil Use Manag., 26, 516–528, https://doi.org/10.1111/j.1475-2743.2010.00305.x, 2010.
Jalali, M. and Ranjbar, F.: Effects of sodic water on soil sodicity and nutrient leaching in poultry and sheep manure amended soils, Geoderma, 153, 194–204, https://doi.org/10.1016/j.geoderma.2009.08.004, 2009.
Jangid, K., Williams, M. A., Franzluebbers, A. J., Schmidt, T. M., Coleman, D. C., and Whitman, W. B.: Land-use history has a stronger impact on soil microbial community composition than aboveground vegetation and soil properties, Soil Biol. Biochem., 43, 2184–2193, https://doi.org/10.1016/j.soilbio.2011.06.022, 2011.
Jenny, H.: Factors of soil formation: a system of quantitative pedology, McGraw-Hill, New York, 1941.
KA-5: Bodenkundliche Kartieranleitung, 5th ed., Schweizerbart'sche, E., Stuttgart, 2005.
Kalinina, O., Chertov, O., Dolgikh, A. V., Goryachkin, S. V., Lyuri, D. I., Vormstein, S., and Giani, L.: Self-restoration of post-agrogenic Albeluvisols: Soil development, carbon stocks and dynamics of carbon pools, Geoderma, 207–208, 221–233, https://doi.org/10.1016/j.geoderma.2013.05.019, 2013.
Kalinina, O., Cherkinsky, A., Chertov, O., Goryachkin, S., Kurganova, I., Lopes de Gerenyu, V., Lyuri, D., Kuzyakov, Y., and Giani, L.: Post-agricultural restoration: Implications for dynamics of soil organic matter pools, Catena, 181, 104096, https://doi.org/10.1016/j.catena.2019.104096, 2019.
Karathanasis, A. D. and Wells, K. L.: A Comparison of Mineral Weathering Trends Between Two Management Systems on a Catena of Loess-Derived Soils, Soil Sci. Soc. Am. J., 53, 582–588, https://doi.org/10.2136/sssaj1989.03615995005300020047x, 1989.
KDPR: Klassifikazia i Diagnostika Pochv Rossii (Classification and Diagnostics of Soil of Russia), edited by: Shishov, L. L., Lebedeva, I. I., Gerasimova, M. I., and Tonkongov, V. D., Smolensk: Oikumena, available at: http://infosoil.ru/index.php?pageID=clas04mode (last access: 10 September 2018), 2004.
Keyvanshokouhi, S., Cornu, S., Samouelian, A., and Finke, P.: Evaluating SoilGen2 as a tool for projecting soil evolution induced by global change, Sci. Total Environ., 571, 110–123, https://doi.org/10.1016/j.scitotenv.2016.07.119, 2016.
Khormali, F., Ajami, M., Ayoubi, S., Srinivasarao, C., and Wani, S. P.: Role of deforestation and hillslope position on soil quality attributes of loess-derived soils in Golestan province, Iran, Agric. Ecosyst. Environ., 134, 178–189, https://doi.org/10.1016/j.agee.2009.06.017, 2009.
Kölbl, A., Schad, P., Jahn, R., Amelung, W., Bannert, A., Cao, Z. H., Fiedler, S., Kalbitz, K., Lehndorff, E., Müller-Niggemann, C., Schloter, M., Schwark, L., Vogelsang, V., Wissing, L., and Kögel-Knabner, I.: Accelerated soil formation due to paddy management on marshlands (Zhejiang Province, China), Geoderma, 228–229, 67–89, https://doi.org/10.1016/j.geoderma.2013.09.005, 2014.
Kozlovskii, F. I.: The Modeling of Agropedogenesis in Plowed Soils on Mantle Loams, Eurasian Soil Sci. CC Pochvovedenie, 32, 710–720, 1999.
Kozlovskii, F. I. and Goryachkin, S. V.: Soil as a mirror of landscape and the concept on informational structure of soil cover, Eur. Soil Sci., 29, 255–263, 1996.
Kurganova, I., Merino, A., Lopes de Gerenyu, V., Barros, N., Kalinina, O., Giani, L., and Kuzyakov, Y.: Mechanisms of carbon sequestration and stabilization by restoration of arable soils after abandonment: A chronosequence study on Phaeozems and Chernozems, Geoderma, 354, 113882, https://doi.org/10.1016/j.geoderma.2019.113882, 2019.
Lal, R.: Long-term tillage and maize monoculture effects on a tropical Alfisol in western Nigeria. I. Crop yield and soil physical properties, Soil Tillage Res., 42, 145–160, https://doi.org/10.1016/S0167-1987(97)00006-8, 1997.
Lal, R.: Soil degradation by erosion, Land Degrad. Dev., 12, 519–539, https://doi.org/10.1002/ldr.472, 2001.
Lal, R.: Climate change, soil carbon dynamics and global food security, in: Climate change and global food security, edited by: Lal, R., Stewart, B. A., Uphoff, N., and Hansen, D. O., 113–143, CRC Press, Taylor & Francis, Boca Raton, FL, 2005.
Lal, R.: Enhancing crop yields in the developing countries through restoration of the soil organic carbon pool in agricultural lands, Land Degrad. Dev., 17, 197–209, https://doi.org/10.1002/ldr.696, 2006.
Lal, R.: Soils and sustainable agriculture. A review, Agron. Sustain. Dev., 28, 57–64, https://doi.org/10.1051/agro:2007025, 2008.
Lal, R.: Soil degradation as a reason for inadequate human nutrition, Food Secur., 1, 45–57, https://doi.org/10.1007/s12571-009-0009-z, 2009.
Lal, R.: Restoring Soil Quality to Mitigate Soil Degradation, Sustainability, 7, 5875–5895, https://doi.org/10.3390/su7055875, 2015.
Lemenih, M., Karltun, E., and Olsson, M.: Assessing soil chemical and physical property responses to deforestation and subsequent cultivation in smallholders farming system in Ethiopia, Agric. Ecosyst. Environ., 105, 373–386, https://doi.org/10.1016/j.agee.2004.01.046, 2005.
Lincoln, N., Chadwick, O., and Vitousek, P.: Indicators of soil fertility and opportunities for precontact agriculture in Kona, Hawai'i, Ecosphere, 5, 42, https://doi.org/10.1890/ES13-00328.1, 2014.
Lipiec, J., Horn, R., Pietrusiewicz, J., and Siczek, A.: Effects of soil compaction on root elongation and anatomy of different cereal plant species, Soil Tillage Res., 121, 74–81, https://doi.org/10.1016/j.still.2012.01.013, 2012.
Lisetskii, F., Stolba, V., Ergina, E., Rodionova, M., and Terekhin, E.: Post-agrogenic evolution of soils in ancient Greek land use areas in the Herakleian Peninsula, southwestern Crimea, The Holocene, 23, 504-514, https://doi.org/10.1177/0959683612463098, 2013.
Lisetskii, F., Stolba, V. F., and Marininà, O.: Indicators of agricultural soil genesis under varying conditions of land use, Steppe Crimea, Geoderma, 239–240, 304–316, https://doi.org/10.1016/j.geoderma.2014.11.006, 2015.
Liu, S. G., Bliss, N., Sundquist, E., and Huntington, T. G.: Modeling carbon dynamics in vegetation and soil under the impact of soil erosion and deposition, Glob. Biogeochem. Cycles, 17, 1074, https://doi.org/10.1029/2002GB002010, 2003.
Liu, X., Zhang, W., Zhang, M., Ficklin, D. L., and Wang, F.: Spatio-temporal variations of soil nutrients influenced by an altered land tenure system in China, Geoderma, 152, 23–34, https://doi.org/10.1016/j.geoderma.2009.05.022, 2009.
Lobe, I., Amelung, W., and Preez, C. C. D.: Losses of carbon and nitrogen with prolonged arable cropping from sandy soils of the South African Highveld, Eur. J. Soil Sci., 52, 93–101, https://doi.org/10.1046/j.1365-2389.2001.t01-1-00362.x, 2001.
Lo Papa, G., Palermo, V., and Dazzi, C.: Is land-use change a cause of loss of pedodiversity? The case of the Mazzarrone study area, Sicily, Geomorphology, 135, 332–342, https://doi.org/10.1016/j.geomorph.2011.02.015, 2011.
Lo Papa, G., Palermo, V., and Dazzi, C.: The “genetic erosion” of the soil ecosystem, Int. Soil Water Conserv. Res., 1, 11–18, https://doi.org/10.1016/S2095-6339(15)30045-9, 2013.
Makovnikova, J., Siran, M., Houskova, B., Palka, B., and Jones, A.: Comparison of different models for predicting soil bulk density. Case study – Slovakian agricultural soils, Int. Agrophysics, 31, 491–498, https://doi.org/10.1515/intag-2016-0079, 2017.
Matus F. and Egli M.: Mathematical–statistical problem that has a significant implication on estimation of interval-specific rates of soil-forming processes, J. Soil Sci. Plant Nutr., in press, https://doi.org/10.1007/s42729-019-00095-y, 2019.
McBratney, A. B., Mendonça Santos, M. L., and Minasny, B.: On digital soil mapping, Geoderma, 117, 3–52, 2003.
Meuser, H.: Anthropogenic Soils, in: Contaminated Urban Soils, edited by: Meuser, H., pp. 121–193, Springer Netherlands, Dordrecht, 2010.
Millward, A. A. and Mersey, J. E.: Adapting the RUSLE to model soil erosion potential in a mountainous tropical watershed, Catena, 38, 109–129, https://doi.org/10.1016/S0341-8162(99)00067-3, 1999.
Minasny, B. and Hartemink, A. E.: Predicting soil properties in the tropics, Earth-Sci. Rev., 106, 52–62, https://doi.org/10.1016/j.earscirev.2011.01.005, 2011.
Morgan, R. P. C., Quinton, J. N., Smith, R. E., Govers, G., Poesen, J. W. A., Auerswald, K., Chisci, G., Torri, D., and Styczen, M. E.: The European Soil Erosion Model (EUROSEM): A dynamic approach for predicting sediment transport from fields and small catchments, Earth Surf. Process. Landf., 23, 527–544, https://doi.org/10.1002/(SICI)1096-9837(199806)23:6<527::AID-ESP868>3.0.CO;2-5, 1998.
Morrison, R. J. and Gawander, J. S.: Changes in the properties of Fijian Oxisols over 30 years of sugarcane cultivation, Soil Res., 54, 418–429, https://doi.org/10.1071/SR15173, 2016.
Nabiollahi, K., Taghizadeh-Mehrjardi, R., Kerry, R., and Moradian, S.: Assessment of soil quality indices for salt-affected agricultural land in Kurdistan Province, Iran, Ecol. Indic., 83, 482–494, https://doi.org/10.1016/j.ecolind.2017.08.001, 2017.
Nannipieri, P., Ascher, J., Ceccherini, M. T., Landi, L., Pietramellara, G., and Renella, G.: Microbial diversity and soil functions, Eur. J. Soil Sci., 54, 655–670, https://doi.org/10.1046/j.1351-0754.2003.0556.x, 2003.
Nyberg, G., Bargués Tobella, A., Kinyangi, J., and Ilstedt, U.: Soil property changes over a 120-yr chronosequence from forest to agriculture in western Kenya, Hydrol. Earth Syst. Sci., 16, 2085–2094, https://doi.org/10.5194/hess-16-2085-2012, 2012.
Obour, A. K., Mikha, M. M., Holman, J. D., and Stahlman, P. W.: Changes in soil surface chemistry after fifty years of tillage and nitrogen fertilization, Geoderma, 308, 46–53, https://doi.org/10.1016/j.geoderma.2017.08.020, 2017.
Ovsepyan, L., Kurganova, I., de Gerenyu, V. L., and Kuzyakov, Y.: Recovery of organic matter and microbial biomass after abandonment of degraded agricultural soils: the influence of climate, Land Degrad. Dev., 30, 1861–1874, https://doi.org/10.1002/ldr.3387, 2019.
Pape, J. C.: Plaggen soils in the Netherlands, Geoderma, 4, 229–255, https://doi.org/10.1016/0016-7061(70)90005-4, 1970.
Paul, E. A.: Soil Microbiology, Ecology and Biochemistry, Academic Press, London, 2014.
Paz-González, A., Vieira, S. R., and Taboada Castro, M. T.: The effect of cultivation on the spatial variability of selected properties of an umbric horizon, Geoderma, 97, 273–292, https://doi.org/10.1016/S0016-7061(00)00066-5, 2000.
Ponge, J.-F., Peres, G., Guernion, M., Ruiz-Camacho, N., Cortet, J., Pernin, C., Villenave, C., Chaussod, R., Martin-Laurent, F., Bispo, A., and Cluzeau, D.: The impact of agricultural practices on soil biota: A regional study, Soil Biol. Biochem., 67, 271–284, https://doi.org/10.1016/j.soilbio.2013.08.026, 2013.
Pournader, M., Ahmadi, H., Feiznia, S., Karimi, H., and Peirovan, H. R.: Spatial prediction of soil erosion susceptibility: an evaluation of the maximum entropy model, Earth Sci. Inform., 11, 389–401, https://doi.org/10.1007/s12145-018-0338-6, 2018.
Raiesi, F.: A minimum data set and soil quality index to quantify the effect of land use conversion on soil quality and degradation in native rangelands of upland arid and semiarid regions, Ecol. Indic., 75, 307–320, https://doi.org/10.1016/j.ecolind.2016.12.049, 2017.
Raiesi, F. and Kabiri, V.: Identification of soil quality indicators for assessing the effect of different tillage practices through a soil quality index in a semi-arid environment, Ecol. Indic., 71, 198–207, https://doi.org/10.1016/j.ecolind.2016.06.061, 2016.
Rasa, K. and Horn, R.: Structure and hydraulic properties of the boreal clay soil under differently managed buffer zones, Soil Use Manag., 29, 410–418, https://doi.org/10.1111/sum.12043, 2013.
Raty, M., Horn, R., Rasa, K., Yli-Halla, M., and Pietola, L.: Compressive behaviour of the soil in buffer zones under different management practices in Finland, Agric. Food Sci., 19, 160–172, 2010.
Rezapour, S. and Samadi, A.: Assessment of inceptisols soil quality following long-term cropping in a calcareous environment, Environ. Monit. Assess., 184, 1311–1323, https://doi.org/10.1007/s10661-011-2042-6, 2012.
Richter, D. D. B., Bacon, A. R., Megan, L. M., Richardson, C. J., Andrews, S. S., West, L., Wills, S., Billings, S., Cambardella, C. A., Cavallaro, N., DeMeester, J. E., Franzluebbers, A. J., Grandy, A. S., Grunwald, S., Gruver, J., Hartshorn, A. S., Janzen, H., Kramer, M. G., Ladha, J. K., Lajtha, K., Liles, G. C., Markewitz, D., Megonigal, P. J., Mermut, A. R., Rasmussen, C., Robinson, D. A., Smith, P., Stiles, C. A., Tate, R. L., Thompson, A., Tugel, A. J., Es, H. V., Yaalon, D., and Zobeck, T. M.: Human-soil relations are changing rapidly: Proposals from SSSA's cross-divisional soil change working group, Soil Sci. Soc. Am. J., 75, 2079–2084, https://doi.org/10.2136/sssaj2011.0124, 2011.
Richter, D. deB. J.: Humanity's transformation of earth's soil: pedology's new frontier, Soil Sci., 172, 957, https://doi.org/10.1097/ss.0b013e3181586bb7, 2007.
Richter, D. deB., Bacon, A. R., Brecheisen, Z., and Mobley, M. L.: Soil in the Anthropocene, IOP Conf. Ser. Earth Environ. Sci., 25, 012010, https://doi.org/10.1088/1755-1315/25/1/012010, 2015.
Rose, C. W., Williams, J. R., Sander, G. C., and Barry, D. A.: A Mathematical Model of Soil Erosion and Deposition Processes: I. Theory for a Plane Land Element 1, Soil Sci. Soc. Am. J., 47, 991–995, https://doi.org/10.2136/sssaj1983.03615995004700050030x, 1983.
Sandor, J., Burras, C. L., and Thompson, M.: Factors of soil formation: human impacts, in: Encyclopedia of Soils in the Environment, edited by: Hillel, D., pp. 520–532, Elsevier Ltd., available at: https://www.elsevier.com/books/encyclopedia-of-soils-in-the-environment/9780123485304 (last access: 22 April 2019), 2005.
Sandor, J. A. and Homburg, J. A.: Anthropogenic Soil Change in Ancient and Traditional Agricultural Fields in Arid to Semiarid Regions of the Americas, J. Ethnobiol., 37, 196–217, https://doi.org/10.2993/0278-0771-37.2.196, 2017.
Sandor, J. A., Hawley, J. W., Schiowitz, R. H., and Gersper, P. L.: Soil-geomorphic setting and change in prehistoric agricultural terraces in the Mimbres area, New Mexico, in: Geology of the Gila Wilderness-Silver City area: New Mexico Geological Society Fifty-ninth Annual Field Conference, 23–25 October 2008, pp. 167–176, New Mexico Geological Society, Socorro, NM, 2008.
Sedov, S., Solleiro-Rebolledo, E., Fedick, S. L., Gama-Castro, J., Palacios-Mayorga, S., and Vallejo Gómez, E.: Soil genesis in relation to landscape evolution and ancient sustainable land use in the northeastern Yucatan Peninsula, Mexico, Atti Della Soc. Toscana Sci. Nat. Mem. Ser. A, 112, 115–126, 2007.
Severiano, E. da C., de Oliveira, G. C., Dias Junior, M. de S., Curi, N., de Pinho Costa, K. A., and Carducci, C. E.: Preconsolidation pressure, soil water retention characteristics, and texture of Latosols in the Brazilian Cerrado, Soil Res., 51, 193–202, https://doi.org/10.1071/SR12366, 2013.
Seybold, C. A., Mausbach, M. J., Karlen, D. L., and Rogers, H. H.: Quantification of soil quality, in: Soil Processes and the Carbon Cycle, edited by: Lal, R., Kimble, J. M., Follett, R. F., and Stewart, B. A., CRC Press, Boca Raton, FL, 1997.
Shiri, J., Keshavarzi, A., Kisi, O., Karimi, S., and Iturraran-Viveros, U.: Modeling soil bulk density through a complete data scanning procedure: Heuristic alternatives, J. Hydrol., 549, 592–602, https://doi.org/10.1016/j.jhydrol.2017.04.035, 2017.
Shpedt, A. A., Trubnikov, Y. N., and Zharinova, N. Y.: Agrogenic degradation of soils in Krasnoyarsk forest-steppe, Eurasian Soil Sci., 50, 1209–1216, https://doi.org/10.1134/S106422931710012X, 2017.
Simonson, R. W.: Outline of a Generalized Theory of Soil Genesis 1, Soil Sci. Soc. Am. J., 23, 152–156, https://doi.org/10.2136/sssaj1959.03615995002300020021x, 1959.
Smith, P., Smith, J. U., Powlson, D. S., McGill, W. B., Arah, J. R. M., Chertov, O. G., Coleman, K., Franko, U., Frolking, S., Jenkinson, D. S., Jensen, L. S., Kelly, R. H., Klein-Gunnewiek, H., Komarov, A. S., Li, C., Molina, J. A. E., Mueller, T., Parton, W. J., Thornley, J. H. M., and Whitmore, A. P.: A comparison of the performance of nine soil organic matter models using datasets from seven long-term experiments, Geoderma, 81, 153–225, https://doi.org/10.1016/S0016-7061(97)00087-6, 1997.
Taalab, K. P., Corstanje, R., Creamer, R., and Whelan, M. J.: Modelling soil bulk density at the landscape scale and its contributions to C stock uncertainty, Biogeosciences, 10, 4691–4704, https://doi.org/10.5194/bg-10-4691-2013, 2013.
Targulian, V. O. and Goryachkin, S. V.: Soil memory: Tapes of record, carriers, hierarchie and diversity, Revista Mexicana de Ciencias Geológicas, 21, 1–8, 2004.
Tiessen, H., Cuevas, E., and Chacon, P.: The role of soil organic matter in sustaining soil fertility, Nature, 371, 783–785, https://doi.org/10.1038/371783a0, 1994.
Tranter, G., Minasny, B., Mcbratney, A. B., Murphy, B., Mckenzie, N. J., Grundy, M., and Brough, D.: Building and testing conceptual and empirical models for predicting soil bulk density, Soil Use Manag., 23, 437–443, https://doi.org/10.1111/j.1475-2743.2007.00092.x, 2007.
Trost, B., Ellmer, F., Baumecker, M., Meyer-Aurich, A., Prochnow, A., and Drastig, K.: Effects of irrigation and nitrogen fertilizer on yield, carbon inputs from above ground harvest residues and soil organic carbon contents of a sandy soil in Germany, Soil Use Manag., 30, 209–218, https://doi.org/10.1111/sum.12123, 2014.
Tugel, A. J., Herrick, J. E., Brown, J. R., Mausbach, M. J., Puckett, W., and Hipple, K.: Soil Change, Soil Survey, and Natural Resources Decision Making, Soil Sci. Soc. Am. J., 69, 738–747, https://doi.org/10.2136/sssaj2004.0163, 2005.
Velde, B. and Peck, T.: Clay mineral changes in the Morrow experimental plots, University of Illinois, Clays Clay Miner., 50, 364–370, 2002.
Wei, G., Zhou, Z., Guo, Y., Dong, Y., Dang, H., Wang, Y., and Ma, J.: Long-Term Effects of Tillage on Soil Aggregates and the Distribution of Soil Organic Carbon, Total Nitrogen, and Other Nutrients in Aggregates on the Semi-Arid Loess Plateau, China, Arid Land Res. Manag., 28, 291–310, https://doi.org/10.1080/15324982.2013.845803, 2014.
Weiss, H., Courty, M., Wetterstrom, W., Guichard, F., Senior, L., Meadow, R., and Curnow, A.: The Genesis and Collapse of 3rd Millennium North Mesopotamian Civilization, Science, 261, 995–1004, https://doi.org/10.1126/science.261.5124.995, 1993.
WRB: World reference base for soil resources 2014: International soil classification system for naming soils and creating legends for soil maps – Update 2015, Food & Agriculture Org., Rome, Italy, 2014.
Yaalon, D. H. and Yaron, B.: Framework for man-made soil changes – an outline of metapedogenesis, Soil Sci., 102, 272–277, 1966.
Zakharov, S. A.: A Course of Soil Science, Gosizdat, Moscow, 1927.
Zamanian, K. and Kuzyakov, Y.: Contribution of soil inorganic carbon to atmospheric CO2: More important than previously thought, Glob. Change Biol., 25, E1–E3, https://doi.org/10.1111/gcb.14463, 2019.
Zhang, Y., Zhao, W., and Fu, L.: Soil macropore characteristics following conversion of native desert soils to irrigated croplands in a desert-oasis ecotone, Northwest China, Soil Tillage Res., 168, 176–186, https://doi.org/10.1016/j.still.2017.01.004, 2017.
Note that in chemistry, mineralogy and materials sciences, a phase diagram is a type of chart used to show conditions (pressure, temperature, volume, etc.) at which thermodynamically distinct phases (e.g. solid, liquid or gaseous states) are at equilibrium.
- Article
(10815 KB) - Full-text XML