the Creative Commons Attribution 4.0 License.
the Creative Commons Attribution 4.0 License.
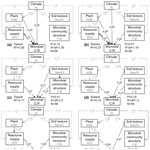
A comparison of patterns of microbial C : N : P stoichiometry between topsoil and subsoil along an aridity gradient
Yuqing Liu
Wenhong Ma
Dan Kou
Xiaxia Niu
Tian Wang
Yongliang Chen
Dima Chen
Xiaoqin Zhu
Mengying Zhao
Baihui Hao
Jinbo Zhang
Yuanhe Yang
Huifeng Hu
Microbial stoichiometry and its potential driving factors play crucial roles in understanding the balance of chemical elements in ecological interactions and nutrient limitations along the aridity gradient. However, little is known about the variation in these features along the aridity gradient due to the lack of comprehensive field investigations. Additionally, previous studies focused on the topsoil (0–10 or 0–20 cm); however, the minimum sampling depth for topsoil could impact the results of the vertical distribution of microbial stoichiometry. In the present study, we measured the variation in microbial stoichiometry, examined the major influential factors (climatic, edaphic, and biotic factors) along an aridity gradient, and determined whether the sampling depth affected microbial C : N : P stoichiometry. From the topsoil to the subsoil, the microbial C : N, C : P, and N : P ratios varied from 6.59 to 6.83, from 60.2 to 60.5, and from 9.29 to 8.91, respectively. Only the microbial C : N ratio significantly increased with soil depth. The microbial C : N ratio significantly increased with increasing aridity in both topsoil and subsoil, while the microbial N : P ratio decreased along the aridity gradient only for the topsoil. This result implied that drought-stimulated microbes tend to be more N conservative, especially those in topsoil. Among all the factors, the soil organic carbon (SOC) content and the fungi-to-bacteria ratio exerted the largest influence on the microbial C : N, C : P, and N : P ratios at both soil depths, implying that the substrate supply and microbial structure together controlled the microbial stoichiometry. The results also revealed that the aridity index (AI) and plant aboveground biomass (AGB) exerted negative impacts on the microbial C : N ratio at both soil depths, and the effects of AI decreased in the subsoil. The results of this study suggested that the flexibility of the microbial N : P ratio should be considered when establishing the sampling depth for microbial stoichiometry study.
- Article
(7462 KB) - Full-text XML
-
Supplement
(777 KB) - BibTeX
- EndNote
Ecological stoichiometry is a powerful tool for understanding the balance of chemical elements required by organisms and the functions of ecosystems (Elser et al., 2000a; Sterner and Elser, 2002). C, N, and P are regarded as critical elements in global biogeochemical cycling, and C : N : P stoichiometry in soil microorganisms offers essential insight into the nutrient limitations of the organisms and communities in an ecosystem (Manzoni et al., 2010; McGroddy et al., 2004).
A few studies have addressed the pattern of microbial stoichiometry along latitudinal (Cleveland and Liptzin, 2007; Li et al., 2015; Xu et al., 2013) or environmental gradients (Li and Chen, 2004; Li et al., 2012, 2015). For example, Cleveland and Liptzin (2007) analyzed microbial stoichiometry at the global scale and showed an increasing trend in the microbial N : P ratio with higher latitude. However, Li et al. (2015) summarized the data and found that the microbial N : P ratio decreased with latitude. Undoubtedly, there is uncertainty in the values of microbial C : N, C : P, and N : P ratios in global studies, and the variations in these patterns might have been partially caused by the different methods that were used in the various studies (Xu et al., 2013; Chen et al., 2016). Furthermore, less exploration of soil microbial stoichiometry along an aridity gradient at the regional scale impedes our ability to disentangle the trend of the changes in microbial stoichiometry amid climate changes. Climate change, such as global warming, is increasing the degree of aridity in drylands owing to the decreased precipitation and increased evaporation (Wang et al., 2014; Li et al., 2017). Given this background, key ecosystem processes that are regulated by soil microbes, such as soil respiration and nutrient mineralization, may be dramatically impacted by the increased degree of aridity, especially in fragile areas of arid and semiarid ecosystems (Delgado-Baquerizo et al., 2013; Chen et al., 2014). Therefore, we conducted a field investigation across a 2100 km climatic transect in the Inner Mongolian grasslands to determine how the microbial C : N, C : P, and N : P ratios were affected by changing environmental conditions.
Previous studies have also shown that a variety of abiotic factors impact microbial C : N : P stoichiometry (Cleveland and Liptzin, 2007; Manzoni et al., 2010; Hartman and Richardson, 2013). For instance, control experiments have found that warming can indirectly affect the turnover of microbial biomass N by stimulating soil respiration (Veraart et al., 2011; Butterbach-Bahl et al., 2013). Climate could exert an influence on microbial stoichiometry through changes to the microenvironment, such as soil moisture and temperature, and it could also impact the availability of substrates in the soil (Nielsen et al., 2009). Moreover, edaphic variables, such as soil organic carbon (SOC; Maria et al., 2014; Chen et al., 2016) and soil texture (Li et al., 2015), could be associated with nutrient mineralization and availability, thus influencing the C : N : P stoichiometry in microbial biomass (Griffiths et al., 2012). A labeled incubation experiment showed that the mineralization of organic P was mainly driven by the microbial C demands in P-poor soils (Aponte et al., 2010; Heuck et al., 2015). In addition, microbial C : N, C : P, and N : P ratios were also affected by biotic factors such as plant productivity and the composition of the microbial community (Fanin et al., 2013; Chen et al., 2016). Generally, fungi exhibit a higher C : N ratio than bacteria (Strickland and Rousk, 2010); thus, a shift in the fungi-to-bacteria ratio is expected to result in microbial stoichiometry changes (Li et al., 2012; Heuck et al., 2015). However, those findings were based on literature analyses or small-scale experiments, and the variations in microbial C : N, C : P, and N : P ratios at the regional scale have rarely been assessed systematically, and the drivers of these variations need to be addressed more specifically with appropriate experimental designs. Moreover, most research has focused on the top 10 cm of soil, which often has high C availability and nutrient contents. It can be assumed that the effects of potential driving factors exhibit minimal differentiation at deeper soil depths. However, soil at a deeper depth might contain microbial communities that are specialized for their environment, and their functions may differ from the functions of the communities in the topsoil (Fritze et al., 2000; Blume et al., 2002). Certainly, the drivers that are responsible for the variations in microbial C : N, C : P, and N : P ratios in deeper soil remain poorly understood. Such knowledge of the nature of soil microbial stoichiometry is fundamental for understanding ecosystem function, especially at the 10–20 cm soil depth, which remains highly uncertain in published studies.
Substrates for microorganisms, such as available nutrients and water, decline exponentially with depth, and the top 20 cm of soil accumulates the greatest amount of microbial biomass, thereby attracting the attention of most researchers (Fierer et al., 2003; Xu et al., 2013). Soil at a 0–20 cm depth was regarded as the topsoil in some studies, while other researchers divided the soil from 0 to 20 cm into different soil depths to explore the vertical differences between these depths (Aponte et al., 2010; Peng and Wang, 2016). However, most studies used 0–10 cm as the topsoil to facilitate sampling and comparative research (Li and Chen, 2004; Cleveland and Liptzin, 2007; Chen et al., 2016). The depth of topsoil varies among studies, and sampling depth can therefore have impacts on the study of the vertical patterns in soil microbial stoichiometry (Tischer et al., 2014). Given that soil represents a highly heterogeneous environment, especially in terms of site-specific soil development history, it is difficult to draw general conclusions (Xu et al., 2013; Camenzind et al., 2018). In addition, if a large difference existed between the soil at 0–10 cm and that at 10–20 cm, microbial stoichiometry would be underestimated due to the ambiguous limitation of topsoil (Tischer et al., 2014). To identify the soil depth that is appropriate for sampling and to improve the understanding of topsoil research at a global scale, we designed a study that divided the topsoil into 0–10 and 10–20 cm depths to compare the differences in microbial stoichiometry at the regional scale.
In Inner Mongolia grasslands, the aridity exhibits a gradient that increases from northeast to southwest (aridity index, calculated as precipitation divided by potential evapotranspiration, ranges from 0.16 to 0.54), thus providing an ideal platform to better estimate the patterns and drivers of microbial C : N : P stoichiometry along an aridity gradient (Chen et al., 2014; Li et al., 2017). In this study, we aim to access the effect of soil depth on soil microbial C : N : P stoichiometry along the aridity gradient. We hypothesized that the microbial C : N and C : P ratios decrease and the microbial N : P ratio increases with temperature (Cleveland and Liptzin, 2007; Li et al., 2015; Xu et al., 2013), and the microbial C : N and C : P ratios decrease and the microbial N : P ratio increases with decreasing aridity index (Wang et al., 2014; Li et al., 2017). In addition, the identification of soil depth for vertical study is different in some published literature (Li and Chen, 2004; Aponte et al., 2010; Tischer et al., 2014; Peng and Wang, 2016). We predicted that variation in bacterial and fungal taxa between soil depths might contribute to the shifts in C : N : P stoichiometry, especially in the N : P ratio (Mouginot et al., 2014; Camenzind et al., 2018). Therefore, we focus on (i) the effects of potential driving factors on microbial C : N, C : P, and N : P ratios in topsoil and subsoil and (ii) the response of the microbial C : N, C : P, and N : P ratios to soil depth.
2.1 Study area
This study was performed across the Inner Mongolian temperate grassland, which is a central part of the Eurasian steppe. The study area is located at 39.2–49.6∘ N latitude and 107.8–120.1∘ E longitude and covers an area of 440 000 km2. From northeast to southwest, the mean annual temperature increases from −1.7 to 7.7 ∘C, and the mean annual precipitation decreases from 402 to 154 mm, approximately 80 % of which falls in the growing season from May to August. Three grassland types, meadow steppe, typical steppe, and desert steppe, are distributed along the northeastern-to-southwestern gradient and are dominated by Stipa baicalensis and Leymus chinensis, S. grandis, and S. klemenzii. The soil types corresponding to the three grassland types are categorized as Chernozems, Kastanozems, and Calcisols according to the soil classification system of the Food and Agriculture Organization of the United Nations.
2.2 Sampling and data collection
Along this transect, a total of 58 sites that were slightly disturbed by humans and domestic animals were sampled, including 10 in the meadow steppe, 28 in the typical steppe, and 20 in the desert steppe (Fig. S1 in the Supplement). Five 1 m × 1 m subplots were established, one at each corner and one in the center of a 10 m × 10 m plot. The plant community in the subplots was identified, and the aboveground biomass (AGB) was harvested. At each site, three replicate soil samples at depths of 0–10 and 10–20 cm were collected from three 1 m × 1 m subplots arranged diagonally in a 10 m × 10 m plot. The samples were mixed to form one composite sample. After gentle homogenization and removal of roots, the soil was sieved through a 2 mm mesh and stored to conduct further experiments. The total carbon concentrations were measured using an elemental analyzer (Vario EL Ø., Elementar, Germany). The soil inorganic carbon content was determined with a carbonate content analyzer (Eijkelkamp 08.53, the Netherlands). The SOC content was calculated by subtracting the soil inorganic carbon from the total carbon. The soil elemental contents were reported in millimoles per kilogram. Soil pH was measured in a suspension with a soil : water ratio of 1 : 2.5. After the removal of organic matter and carbonates, the soil texture was determined using a particle size analyzer (Malvern Mastersizer 2000, UK).
2.3 Aridity index
The aridity index was extracted from the Global Aridity Index (Global-Aridity) dataset, which provides high-resolution (30 arcsec or ∼1 km at the Equator) global raster climate data for the period 1950–2000 (https://cgiarcsi.community/data/global-aridity-and-pet-database/, last access: 9 April 2020) (Zomer et al., 2008; Trabucco and Zomer, 2009). The specific calculation formula is as follows (Eq. 1):
where MAP represents the mean annual precipitation, obtained from the WorldClim Global Climate Data (https://www.worldclim.org, last access: 14 April 2020), MAE represents the mean annual potential evapotranspiration (PET), Tmean represents the monthly mean temperature, TD is calculated as the difference between the monthly maximum and minimum temperatures, and RA represents the extraterrestrial radiation above the atmosphere.
2.4 Soil microbial analyses
Microbial biomass carbon (MBC) and microbial biomass nitrogen (MBN) were determined following the chloroform fumigation–K2SO4 extraction method, according to Vance et al. (1987) and Wu et al. (1990). The soil was preincubated at 25 ∘C for 2 weeks at a field water capacity of 40 %. Then, the soil was fumigated with chloroform for 24 h in a vacuum. The fumigated and nonfumigated samples were extracted using 0.5 M K2SO4 with a soil : solution mass ratio of 1 : 4. The C and N contents were measured with a multi N/C analyzer (Analytik Jena, Germany). Using a universal conversion factor of 0.45 (Jenkinson and Powlson, 1976), the amounts of MBC and MBN were calculated by subtracting the amounts of extractable C and N in the nonfumigated samples from those in the fumigated samples (Vance et al., 1987; Wu et al., 1990; Joergensen, 1996). Microbial biomass phosphorus (MBP) was estimated according to the method described in Hedley and Stewart (1982) and modified by Wu et al. (1990). The fumigation procedure was the same as that for MBC and MBN. The fumigated and nonfumigated samples were extracted using 0.5 mol L−1 NaHCO3 and were analyzed to determine the total phosphorus concentration using a colorimetric method. Using a universal conversion factor of 0.40, the amount of MBP was calculated by subtracting the amount of extractable P in the nonfumigated samples from that in the fumigated samples (Hedley and Stewart, 1982). Phospholipid fatty acids (PLFAs) were extracted from the soil using the method described by Bossio and Scow (1998). Briefly, 8 g of soil (dry weight) was used for PLFA analysis. The resultant fatty acid methyl esters were separated, quantified, and identified using capillary gas chromatography. The following PLFAs were used as markers for each of the specific groups: for fungi, 18 : 1ω9c, 18 : 2ω6c, and 18 : 3ω6c; for bacteria, i13 : 0, a13 : 0, i14 : 0, i15 , 0, a15 : 0, 15 : 1ω6, 2OH16 : 0, i16 : 0, 16 : 1ω7c, 16 : 1ω9c, a17 : 0, i17:0, 17 : 1ω8c, cy17 : 0, i18 : 0, 18 : 1ω7, 18 : 1ω5, and cy19 : 0.
2.5 Statistical analyses
The C : N, C : P, and N : P ratios in the soil microbial biomass were log 10-transformed before analysis to improve their normality (Fig. S3). Paired-sample t tests were used to determine the differences in the soil microbial biomass C, N, and P between the topsoil and subsoil and the differences in the C : N : P stoichiometry ratios in the soil microbial biomass. Ordinary least-squares regression analyses were conducted to evaluate the relationship between the C : N : P ratios in the soil microbial biomass and latitude, aridity index, AGB, SOC, sand percentage, and fungi-to-bacteria ratio (F : B ratio). The analyses were performed with SPSS 19.0 software (IBM Corporation, Armonk, NY, USA). A structural equation model (SEM) was used to test the multivariate effects (direct and indirect) on the C : N : P ratios in the microbial biomass through hypothetical factor pathways (Fig. S4). The SEM was constructed using the Amos 17.0 software package (Smallwaters Corporation, Chicago, IL, USA).
3.1 The variation in microbial C : N : P stoichiometry between soil depths along the environmental gradient
The results indicate well-constrained relationships among C, N, and P in soil microbial biomass (Fig. S2). The soil microbial biomass C : N, C : P, and N : P ratios varied by an order of magnitude. Significantly different water content, soil bulk density, sand percentages, and SOC content were found between soil depths (P<0.05, Fig. 1a, b, c, f). The microbial biomass C, N, and P concentrations in the topsoil were significantly higher than those in the subsoil (P<0.05, Table 2). The C : N, C : P, and N : P ratios in the microbial biomass of the topsoil were 6.59, 60.2, and 9.29, respectively, while those in the subsoil were 6.83, 60.5, and 8.91, respectively (Table 2). Moreover, the microbial C : N ratio in the subsoil was significantly higher than that in the topsoil (Table 2).
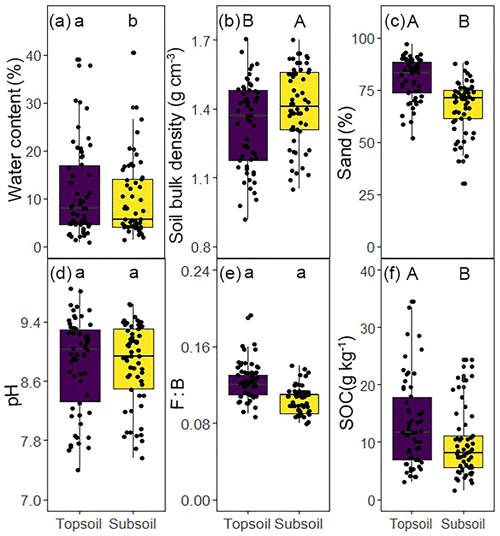
Figure 1Basic characteristics of study sites across the Inner Mongolian grassland at different soil depths. Different letters indicate significant differences between soil depths on log 10-transformed data (paired t test, lowercase letter, P<0.05; uppercase letter, P<0.001).
Table 1Basic information of study sites.
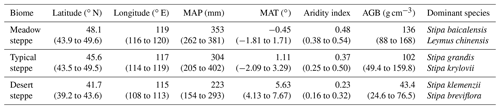
Note that data represent the means, with minimum and maximum values in parentheses. MAT, mean annual temperature; MAP, mean annual precipitation; AGB, aboveground biomass.
Table 2The microbial biomass C, N, and P concentrations and microbial C : N : P stoichiometric ratios across the Inner Mongolian grassland at different soil depths.
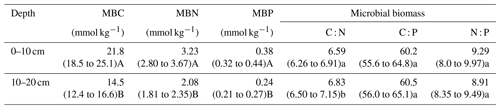
Note that different letters indicate significant differences between soil depths based on log10-transformed data (paired t test, lowercase letter, P<0.05; uppercase letter, P<0.001).
The results revealed a significant positive relationship between the aridity index (AI) and the microbial C : N ratio (topsoil, R2=0.10, P<0.05; subsoil, R2=0.09, P<0.05, Fig. 2a) and the decreasing trend between the AI and the microbial N : P ratio (topsoil, R2=0.10, P<0.01; Fig. 2c). In addition, decreasing trend was found between the microbial C : N ratio and MAT (topsoil, R2=0.14, P<0.01; subsoil, R2=0.10, P<0.01, Fig. 2d), while a significant negative relationship was found between the microbial N : P ratio and MAT (topsoil, R2=0.19, P<0.001; Fig. 2f). The increasing trend between the microbial C : N ratio and latitude was found in topsoil, and significant positive relationships were found in subsoil (topsoil, R2=0.14, P<0.01; subsoil, R2=0.12, P<0.05, Fig. 2g), while a negative relationship was found between the microbial N : P ratio and latitude (topsoil, R2=0.18, P<0.001; Fig. 2i). The microbial C : N ratio was positively related to AGB (topsoil, R2=0.06, P<0.05, Fig. 3a) and SOC (topsoil, R2=0.12, P<0.01; subsoil, R2=0.09, P<0.05, Fig. 3d) and was negatively related to the sand percentage (topsoil, R2=0.11, P<0.01; subsoil, R2=0.11, P<0.01, Fig. 3g). A significant positive relationship was found between the microbial C : P ratio and the content of soil organic matter (Subsoil, R2=0.08, P<0.05, Fig. 3e). No or only weak association was found between the microbial C : N, C : P, and N : P ratios and the AGB and F : B ratio in the subsoil (Fig. 3).
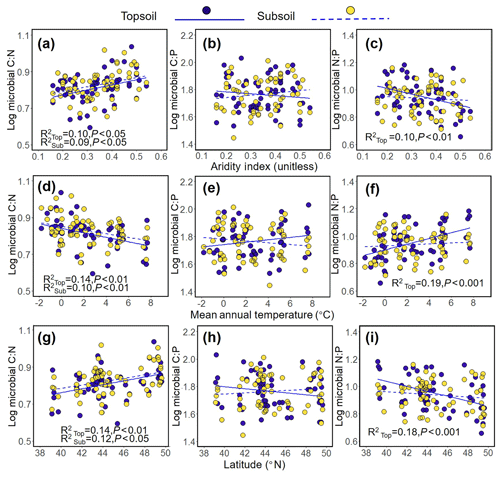
Figure 2Relationships between the C : N, C : P, and N : P ratios in soil microbial biomass and aridity index (a–c), mean annual temperature (d–e), and latitude (g–i) in the Inner Mongolian grassland.
3.2 Effects of potential driving factors on the microbial C : N : P stoichiometry in topsoil and subsoil
The final SEM adequately fit the data, as shown by several robust goodness-of-fit measures (P value and minimum discrepancy). The model explained 38 % (topsoil) and 27 % (subsoil) of the variation in the microbial C : N ratio, 17 % and 19 % of that in the microbial C : P ratio, and 29 % and 16 % of that in the microbial N : P ratio (Fig. 4). Effects of AI, AGB, and SOC content on the microbial C : N ratio were found at both soil depths (Fig. 4a, b). The SOC content made the largest positive contribution to the variation in the microbial C : N ratio in the topsoil (Fig. 4a, b). We found direct effects of the sand percentage, SOC content, and F : B ratio on the microbial C : P ratio at both soil depths, and the SOC content made the largest contribution to the variation in the microbial C : P ratio in the topsoil, which was higher than that in the subsoil (Fig. 4e, f). Influences of sand percentage and the SOC content on the microbial N : P ratio were found in the topsoil, while the F : B ratio and the SOC content explained most of the variation in the microbial N : P ratio in the subsoil (Fig. 4e, f).
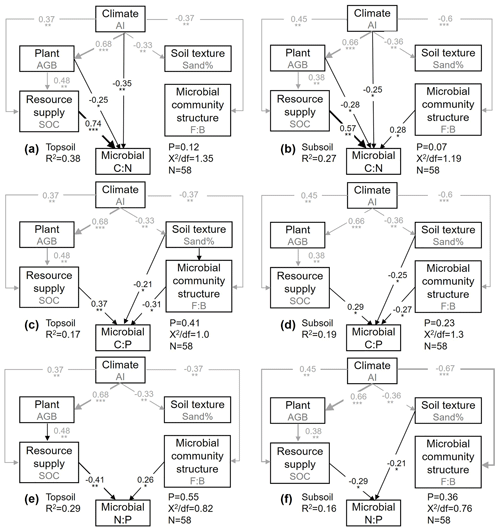
Figure 4The structural equation model (SEM) shows the direct and indirect influences of various ecological factors on the microbial C : N (a, b), C : P (c, d), and N : P (e–f) ratios in the topsoil and subsoil. Black and gray arrows indicate direct and indirect pathways, respectively. Numbers on the arrows indicate standardized path coefficients, proportional to the arrow width. R2 indicates the variation in the microbial C : N and C : P ratios explained by the model. * P<0.05; P<0.01; P<0.001.
4.1 The pattern of microbial C : N, C : P, and N : P ratios along the aridity and latitude gradients
As stated in our hypothesis, the increase in the microbial C : N ratio and the decrease in the microbial N : P ratio that were found along a temperature gradient in this study are in accordance with the findings of Li et al. (2015) and Chen et al. (2016), who reported similar variations in microbial stoichiometry along latitudinal gradient. Temperature drives the variation in the growth of the microbial community, as high growth rates at low latitudes require high RNA contents, causing the N : P ratio to decline (Chadwick et al., 1999; Kooijman et al., 2009; Xu et al., 2013). In addition, we observed that the microbial C : N ratio significantly increased with increasing aridity index, while the microbial N : P ratio decreased with increasing aridity index, indicating that drought (decreasing aridity index) affects ecological stoichiometry by mediating the growth rate of microorganisms in semiarid regions (Elser et al., 2000b; Peng and Wang, 2016). Wang et al. (2014) and Kou et al. (2018) both noted that increasing aridity reduced the soil microbial abundance in drylands, and a decreased growth rate in dry areas might result in decreased allocation to P-rich ribosomal RNA (and thus higher C : P and N : P ratios) (Maestre et al., 2015). Additionally, microbial C : N ratio decreased with the decreasing aridity index, which serves as a protective mechanism as microbes decrease their nitrogen use efficiency (NUE, the ratio of N invested in growth over total N uptake) and tend to be more N conservative under drier climatic conditions (Mooshammer et al., 2014; Delgado-Baquerizo et al., 2017). Moreover, under drier climate conditions, the soil microbial communities shift from acting as r-strategists (fast-growing copiotrophs) to acting as K-strategists (slow-growing oligotrophs), as microorganisms with K-strategies have lower nutrient demands (N and P) and growth rates, invest more nutrients into extracellular enzymes to gain limited nutrients and thus have higher cellular C : N : P ratios than r-strategists (Fierer et al., 2007, 2010).
The microbial C : N ratio demonstrated an increasing trend with increasing latitude, in contrast to the decreasing trend that was demonstrated for the microbial N : P ratio. Such results paralleled the results of studies on ecological stoichiometry, which revealed that the C : N ratio of microorganisms increased with latitude, while the N : P ratio decreased with latitude, suggesting increasing N limitations in microorganism ecosystems in high-latitude areas (Li et al., 2015; Chen et al., 2016). The regional-scale microbial stoichiometry followed the global-scale stoichiometry patterns that were observed for plant leaves (Reich and Oleksyn, 2004; Yuan et al., 2011), litter (McGroddy et al., 2004), and soil (Sardans et al., 2012), conforming to the substrate age hypothesis, which predicts young soils to be N-limited, whereas old soils tend to be P-limited (Walker and Syers, 1976; Vitousek et al., 2010). Our study further illustrated the latitudinal pattern of microbial stoichiometry and first attempted to examine the variation in microbial stoichiometry along an aridity gradient at the regional scale.
4.2 Direct effects of ecological factors on controlling microbial C : N, C : P, and N : P ratios in topsoil and subsoil
Among the ecological factors examined, our study found that the patterns of microbial C : N and C : P ratios were associated with SOC content and the F : B ratio, suggesting that the available C and microbial community structure together regulated the shift in microbial stoichiometry. If the environmental parameters were considered individually, SOC content was found to be significantly positively related to the microbial C : N and C : P ratios, which is consistent with the results observed from global data analysis, suggesting that SOC content may control microbial stoichiometry by mediating the substrate stoichiometry, e.g., the soil C : N and C : P ratios (Hartman and Richardson, 2013; Maria et al., 2014; Mooshammer et al., 2014). In deeper soil, microbial metabolic processes are limited by C availability and energy (C), such as denitrification and P mineralization, thus resulting in the effect of SOC on microbial C : N : P stoichiometry in subsoil (Fierer et al., 2003; Peng and Wang, 2016; Camenzind et al., 2018).
SEM also illustrated that the microbial community structure is an important feature in determining microbial stoichiometry. The F : B ratio has recently been found to have a vital influence on the patterns of microbial C : N and N : P ratios in soil at a large scale (Chen et al., 2016). An experiment indicated that fungi have lower resource requirements and higher C : N and C : P ratios than bacteria; thus, the shift in the F : B ratio impacted microbial C : N : P stoichiometry (Mouginot et al., 2014). In our study, the lower F : B ratio might have led to a shift in the microbial nutrient stoichiometry at deeper soil depths (Tischer et al., 2014). Overall, the SEM highlighted the important role of the C availability and microbial community structure in driving the variations in microbial C : N, C : P, and N : P ratios at both soil depths.
Moreover, AGB and AI also exerted direct influences on microbial C : N or C : P ratios, and those impacts mainly acted in the topsoil but were weaker in the subsoil. The climate imposes important controls on both the plant community and the microbial taxa along with their interactions with soil nutrients (Chadwick et al., 1999; Vitousek et al., 2010; Reich and Oleksyn, 2004). In particular, drier weather conditions, and the decreasing aridity index, could affect the growth and productivity of plants and then shape a shift in vegetation types along this grassland transect (Jaleel et al., 2009; Cherwin and Knapp, 2012). At the same time, the meadow steppe ecosystem with high productivity maintained relatively high soil C and N contents, which resulted in high C : P and N : P ratios in these regions; thus, plant productivity exerted a positive influence on microbial C : N (Aponte et al., 2010; Manzoni et al., 2010). Because of the vertical distribution of the influence of the AI, the effect decreased with soil depth.
Interestingly, our results revealed that the microbial N : P ratio was mainly impacted by the F : B ratio and SOC content, while the sand percentage and SOC content had direct negative effects on the ratio in the subsoil, suggesting the flexibility of microbial stoichiometry in response to distinct resource supplies between topsoil and subsoil (Peng and Wang, 2016). The soil depth affected the microbial biomass N and P, which decreased nearly 2-fold from the topsoil to the subsoil (Table 2). However, the results showed that the N and P cycles responded asymmetrically to soil depth, which might be attributed to the high variability in P availability (Li et al., 2015; Zechmeister-Boltenstern et al., 2016). Generally, P is mostly derived from parent material, while N is mainly a biological element (Vitousek and Farrington, 1997; Vitousek et al., 2010). Therefore, it is believed that P variations regulate large-scale patterns in microbial N : P stoichiometry and nutrient use strategies (Heuck et al., 2015; Camenzind et al., 2018). With a high proportion of sand, the soil becomes porous, which may lead to increased leaching of available P to deeper soil depths (Otten et al., 1999; Achbergerová and Nahálka, 2011). Similarly, P leaching caused by weathering led to a shift in the N : P ratio in the soil, and a vertical study found a high variation in the N : P ratio between soil depths across a large scale (Tian et al., 2010). The high variability of the N : P ratio in soil and soil microbial biomass therefore indicates that the N : P ratio could be an indicator of the ecosystem nutrient status at deeper soil depths (Cleveland and Liptzin, 2007; Li et al., 2015).
4.3 How deep should we dig to evaluate the topsoil microbial stoichiometry in a vertical study?
The results showed significant differences in the water content and SOC content between topsoil and subsoil, suggesting that the resource supplies between topsoil and subsoil were significantly different. We also observed that the microbial C : N, C : P, and N : P ratios varied between topsoil and subsoil, and a significant difference was found in the microbial C : N ratio. Those results indicated that the flexibility of the microbial stoichiometry responds to different resource supplies between soil depths (Tian et al., 2010; Peng and Wang, 2016). Similar findings were found in the top 16 cm of soil in a Mediterranean oak forest (0–8 and 8–16 cm), where the microbial nutrient ratios (C : N, C : P, and N : P) varied with soil depths (Aponte et al., 2010). Tischer et al. (2014) sampled the top 20 cm of soil (0–5, 5–10, 10–20 cm) and observed that the microbial C : N ratio changed with soil depth. Moreover, sampling to a depth of 10 cm showed a significant difference in the microbial N : P ratio (Tischer et al., 2014). The detection of the differences in the microbial N : P ratio in our study depended strongly on the sampling depth, suggesting that the microbial N : P ratio might provide insight into the nature of ecosystem nutrient limitations in a vertical study (Cleveland and Liptzin, 2007; Fierer et al., 2010). In addition, SEM also showed that the microbial N : P ratio was controlled by multiple potential driving factors at different soil depths, indicating that a 0–10 cm or shallower sampling interval should be used when studying the vertical patterns in the microbial N : P ratio.
The first uncertainty was related to the determination of fungal and bacterial biomasses by PLFA markers, which have limited targets for fungi and bacteria. This uncertainty should be noted when interpreting the results in the present study. Methodological advances in sequencing approaches might be used to more accurately index the microbial community and reveal insights into the regulation of microbial C : N : P stoichiometry in distinct soil microbial taxa or functional groups. Second, the theory used to construct the model is another source of uncertainty; the theory related to the drivers of microbial stoichiometry used in this study was mostly derived from a literature review and summarized data. In future research, more control experiments with the manipulation of C availability, especially at deeper soil depths, would further improve our understanding of the changes in microbial stoichiometry and nutrient limitations under the impacts of global change.
The ratios of C, N, and P in the microbial biomass were 6.59 : 60.2 : 9.29 in the topsoil, which deviated from the 6.83 : 60.5 : 8.91 ratio in the subsoil. Moreover, significant differences were found in the microbial C : N and N : P ratios between topsoil and subsoil, indicating that the flexibility of microbial stoichiometry should be considered for vertical study. In addition, the trend of microbial C : N ratio increasing with aridity index is consistent with the perspective that microbes mediate their nitrogen use efficiency and tend to be more N conservative under drier climatic conditions. The microbial N : P ratio trend along the aridity gradient was consistent with the growth rate hypothesis that a decreased growth rate in dry areas results in decreased allocation to P-rich ribosomal RNA and thus a higher N : P ratio. These findings confirmed the importance of SOC content, the microbial structure, and soil texture in shaping the pattern of microbial stoichiometry in semiarid grassland systems. The influence of ecological factors decreased from topsoil to subsoil, and the climatic, edaphic, and biotic factors declined as well. Overall, these results illustrated N and P limitation in microbial biomass at deeper soil depths along the aridity gradient and limited responses to ecological factors in the subsoil.
Data for aridity index are available on the global aridity and PET database at https://cgiarcsi.community/data/global-aridity-and-pet-database/ (Trabucco and Zomer, 2009). Data are available upon request by contacting the corresponding author.
The supplement related to this article is available online at: https://doi.org/10.5194/bg-17-2009-2020-supplement.
HH, WM, and YY devised the study. YL carried out the experiment and data analyses. DK, YC, and DC assisted with the data analyses and interpretation. XN, TW, XZ, MZ, and HB assisted with the experiment. All authors contributed to the preparation of the paper.
The authors declare that they have no conflict of interest.
We thank the sampling team members of Institute of Botany, Chinese Academy of Science for assistance with the field data collection.
This research has been supported by the National Basic Research Program of China (grant nos. 2015CB954201 and 2014CB954303) and the National Natural Science Foundation of China (grant no. 31470498).
This paper was edited by Yakov Kuzyakov and reviewed by three anonymous referees.
Achbergerová, L. and Nahálka, J.: Polyphosphate – an ancient energy source and active metabolic regulator, Microb. Cell Fact., 10, 63, https://doi.org/10.1186/1475-2859-10-63, 2011.
Aponte, C., Marañón, T., García, L. V., Johnson, D., Vile, M., and Wieder, K.: Microbial C, N and P in soils of Mediterranean oak forests: influence of season, canopy cover and soil depth, Biogeochemistry, 101, 77–92, 2010.
Blume, E., Bischoff, M., Reichert, J. M., Moorman, T., Konopka, A., and Turco, R. F.: Surface and subsurface microbial biomass, community structure and metabolic activity as a function of soil depth and season, Appl. Soil Ecol., 20, 171–181, https://doi.org/10.1016/S0929-1393(02)00025-2, 2002.
Bossio, D. A. and Scow, K. M.: Impacts of Carbon and Flooding on Soil Microbial Communities: Phospholipid Fatty Acid Profiles and Substrate Utilization Patterns, Microb. Ecol., 35, 265–278, 1998.
Butterbach-Bahl, K., Baggs Elizabeth, M., Dannenmann, M., Kiese, R., and Zechmeister-Boltenstern, S.: Nitrous oxide emissions from soils: how well do we understand the processes and their controls?, Philos. T. R. Soc. B, 368, 20130122, https://doi.org/10.1098/rstb.2013.0122, 2013.
Camenzind, T., Hättenschwiler, S., Treseder, K. K., Lehmann, A., and Rillig, M. C.: Nutrient limitation of soil microbial processes in tropical forests, 88, 4–21, https://doi.org/10.1002/ecm.1279, 2018.
Chadwick, O. A., Derry, L. A., Vitousek, P. M., Huebert, B. J., and Hedin, L. O.: Changing sources of nutrients during four million years of ecosystem development, Nature, 397, 491–497, https://doi.org/10.1038/17276, 1999.
Chen, D., Mi, J., Chu, P., Cheng, J., Zhang, L., Pan, Q., Xie, Y., and Bai, Y.: Patterns and drivers of soil microbial communities along a precipitation gradient on the Mongolian Plateau, Landscape Ecol., 30, 1669–1682, 2014.
Chen, Y. L., Chen, L. Y., Peng, Y. F., Ding, J. Z., Li, F., Yang, G. B., Kou, D., Liu, L., Fang, K., and Zhang, B. B.: Linking microbial C : N : P stoichiometry to microbial community and abiotic factors along a 3500 km grassland transect on the Tibetan Plateau, Global Ecol. Biogeogr., 25, 1416–1427, 2016.
Cherwin, K. and Knapp, A.: Unexpected patterns of sensitivity to drought in three semi-arid grasslands, Oecologia, 169, 845–852, https://doi.org/10.1007/s00442-011-2235-2, 2012.
Cleveland, C. C. and Liptzin, D.: C : N : P Stoichiometry in Soil: Is There a “Redfield Ratio” for the Microbial Biomass?, Biogeochemistry, 85, 235–252, 2007.
Delgado-Baquerizo, M., Powell, J. R., Hamonts, K., Reith, F., Mele, P., Brown, M. V., Dennis, P. G., Ferrari, B. C., Fitzgerald, A., and Young, A.: Circular linkages between soil biodiversity, fertility and plant productivity are limited to topsoil at the continental scale, New Phytol., 215, 1186–1196, https://doi.org/10.1111/nph.14634, 2017.
Delgado-Baquerizo, M., Maestre, F. T., Gallardo, A., Bowker, M. A., Wallenstein, M. D., Quero, J. L., Ochoa, V., Gozalo, B., Garcíagómez, M., and Soliveres, S.: Decoupling of soil nutrient cycles as a function of aridity in global drylands, Nature, 502, 672–676, https://doi.org/10.1038/nature12670, 2013.
Elser, J. J., Fagan, W. F., Denno, R. F., Dobberfuhl, D. R., Folarin, A., Huberty, A., Interlandi, S., Kilham, S. S., Mccauley, E., and Schulz, K. L.: Nutritional constraints in terrestrial and freshwater food webs, Nature, 408, 578–580, 2000a.
Elser, J. J., Sterner, R. W., Gorokhova, E., Fagan, W. F., Markow, T. A., Cotner, J. B., Harrison, J. F., Hobbie, S. E., Odell, G. M., and Weider, L. W.: Biological stoichiometry from genes to ecosystems, Ecol. Lett., 3, 540–550, 2000b.
Fanin, N., Fromin, N., Buatois, B., and Hättenschwiler, S.: An experimental test of the hypothesis of non-homeostatic consumer stoichiometry in a plant litter-microbe system, Ecol. Lett., 16, 764–772, https://doi.org/10.1111/ele.12108, 2013.
Fierer, N., Schimel, J. P., and Holden, P. A.: Variations in microbial community composition through two soil depth profiles, Soil Biol. Biochem., 35, 167–176, 2003.
Fierer, N., Bradford, M. A., and Jackson, R. B.: Toward an ecological classification of soil bacteria, Ecology, 88, 1354–1364, 2007.
Fierer, N., Strickland, M. S., Liptzin, D., Bradford, M. A., and Cleveland, C. C.: Global patterns in belowground communities, Ecol. Lett., 12, 1238–1249, 2010.
Fritze, H., Pietikäinen, J., and Pennanen, T.: Distribution of microbial biomass and phospholipid fatty acids in Podzol profiles under coniferous forest, Eur. J. Soil Sci., 51, 565–573, https://doi.org/10.1111/j.1365-2389.2000.00346.x, 2000.
Griffiths, B. S., Spilles, A., and Bonkowski, M.: C : N : P stoichiometry and nutrient limitation of the soil microbial biomass in a grazed grassland site under experimental P limitation or excess, Ecol. Process., 1, 6, https://doi.org/10.1186/2192-1709-1-6, 2012.
Hartman, W. H. and Richardson, C. J.: Differential Nutrient Limitation of Soil Microbial Biomass and Metabolic Quotients (qCO2): Is There a Biological Stoichiometry of Soil Microbes?, PloS one, 8, e57127, https://doi.org/10.1371/journal.pone.0057127, 2013.
Hedley, M. J. and Stewart, J. W. B.: Method to measure microbial phosphate in soils, Soil Biol. Biochem., 14, 377–385, 1982.
Heuck, C., Weig, A., and Spohn, M.: Soil microbial biomass C : N : P stoichiometry and microbial use of organic phosphorus, Soil Biol. Biochem., 85, 119–129, 2015.
Jaleel, C. A., Manivannan, P., Wahid, A., Farooq, M., Al-Juburi, H. J., Somasundaram, R., and Panneerselvam, R.: Drought stress in plants: a review on morphological characteristics and pigments composition, Int. J. Agr. Biol., 11, 100–105, 2009.
Jenkinson, D. S. and Powlson, D. S.: The effects of biocidal treatments on metabolism in soil – I. Fumigation with chloroform, Soil Biol. Biochem., 8, 167–177, https://doi.org/10.1016/0038-0717(76)90001-8, 1976.
Joergensen, R. G.: The fumigation-extraction method to estimate soil microbial biomass: Calibration of the kEC value, Soil Biol. Biochem., 28, 25–31, https://doi.org/10.1016/0038-0717(95)00102-6, 1996.
Kooijman, A. M., van Mourik, J. M., and Schilder, M. L. M.: The relationship between N mineralization or microbial biomass N with micromorphological properties in beech forest soils with different texture and pH, Biol. Fert. Soils, 45, 449, https://doi.org/10.1007/s00374-009-0354-2, 2009.
Kou, D., Ma, W. H., Ding, J. Z., Zhang, B. B., Fang, K., Hu, H., Yu, J. C., Wang, T., Qin, S. Q., Zhao, X., Fang, J. Y., and Yang, Y. H.: Dryland soils in northern China sequester carbon during the early 2000s warming hiatus period, Funct. Ecol., 32, 1620–1630, https://doi.org/10.1111/1365-2435.13088, 2018.
Li, H., Zhang, J., Hu, H., Chen, L., Zhu, Y., Shen, H., and Fang, J.: Shift in soil microbial communities with shrub encroachment in Inner Mongolia grasslands, China, Eur. J. Soil Biol., 79, 40–47, 2017.
Li, P., Yang, Y., Han, W., and Fang, J.: Global patterns of soil microbial nitrogen and phosphorus stoichiometry in forest ecosystems, Global Ecol. Biogeogr., 23, 979–987, 2015.
Li, X. Z. and Chen, Z. Z.: Soil microbial biomass C and N along a climatic transect in the Mongolian steppe, Biol. Fert. Soils, 39, 344–351, 2004.
Li, Y., Wu, J., Liu, S., Shen, J., Huang, D., Su, Y., Wei, W., and Syers, J. K.: Is the C : N : P stoichiometry in soil and soil microbial biomass related to the landscape and land use in southern subtropical China?, Global Biogeochem. Cy., 26, GB4002, https://doi.org/10.1029/2012gb004399, 2012.
Maestre, F. T., Delgado-Baquerizo, M., Jeffries, T. C., Eldridge, D. J., Ochoa, V., Gozalo, B., Quero, J. L., García-Gómez, M., Gallardo, A., Ulrich, W., Bowker, M. A., Arredondo, T., Barraza-Zepeda, C., Bran, D., Florentino, A., Gaitán, J., Gutiérrez, J. R., Huber-Sannwald, E., Jankju, M., Mau, R. L., Miriti, M., Naseri, K., Ospina, A., Stavi, I., Wang, D., Woods, N. N., Yuan, X., Zaady, E., and Singh, B. K.: Increasing aridity reduces soil microbial diversity and abundance in global drylands, P. Natl. Acad. Sci. USA, 112, 15684, https://doi.org/10.1073/pnas.1516684112, 2015.
Manzoni, S., Trofymow, J. A., Jackson, R. B., and Porporato, A.: Stoichiometric controls on carbon, nitrogen, and phosphorus dynamics in decomposing litter, Ecol. Monogr., 80, 89–106, 2010.
Maria, M., Wolfgang, W., Sophie, Z. B., and Andreas, R.: Stoichiometric imbalances between terrestrial decomposer communities and their resources: mechanisms and implications of microbial adaptations to their resources, Front. Microbiol., 5, 22, https://doi.org/10.3389/fmicb.2014.00022, 2014.
McGroddy, M. E., Daufresne, T., and Hedin, L. O.: Scaling of C : N : P stoichiometry in forests worldwide: Implications of terrestrial redfield-type ratios, Ecology, 85, 2390–2401, 2004.
Mooshammer, M., Wanek, W., Hämmerle, I., Fuchslueger, L., Hofhansl, F., Knoltsch, A., Schnecker, J., Takriti, M., Watzka, M., Wild, B., Keiblinger, K. M., Zechmeister-Boltenstern, S., and Richter, A.: Adjustment of microbial nitrogen use efficiency to carbon : nitrogen imbalances regulates soil nitrogen cycling, Nat. Commun., 5, 3694, https://doi.org/10.1038/ncomms4694, 2014.
Mouginot, C., Kawamura, R., Matulich, K. L., Berlemont, R., Allison, S. D., Amend, A. S., and Martiny, A. C.: Elemental stoichiometry of Fungi and Bacteria strains from grassland leaf litter, Soil Biol. Biochem., 76, 278–285, 2014.
Nielsen, P. L., Andresen, L. C., Michelsen, A., Schmidt, I. K., and Kongstad, J.: Seasonal variations and effects of nutrient applications on N and P and microbial biomass under two temperate heathland plants, Appl. Soil Ecol., 42, 279–287, https://doi.org/10.1016/j.apsoil.2009.05.006, 2009.
Otten, W., Gilligan, C. A., Watts, C. W., Dexter, A. R., and Hall, D.: Continuity of air-filled pores and invasion thresholds for a soil-borne fungal plant pathogen, Rhizoctonia solani, Soil Biol. Biochem., 31, 1803–1810, 1999.
Peng, X. and Wang, W.: Stoichiometry of soil extracellular enzyme activity along a climatic transect in temperate grasslands of northern China, Soil Biol. Biochem., 98, 74–84, 2016.
Reich, P. B. and Oleksyn, J.: Global patterns of plant leaf N and P in relation to temperature and latitude, P. Natl. Acad. Sci. USA, 101, 11001, https://doi.org/10.1073/pnas.0403588101, 2004.
Sardans, J., Rivas-Ubach, A., and Peñuelas, J.: The elemental stoichiometry of aquatic and terrestrial ecosystems and its relationships with organismic lifestyle and ecosystem structure and function: a review and perspectives, Biogeochemistry, 111, 1–39, 2012.
Sterner, R. W. and Elser, J. J.: Ecological Stoichiometry: The Biology of Elements from Molecules to the Biosphere, Princeton University Press, Princeton, 2002.
Strickland, M. S. and Rousk, J.: Considering fungal:bacterial dominance in soils – Methods, controls, and ecosystem implications, Soil Biol. Biochem., 42, 1385–1395, https://doi.org/10.1016/j.soilbio.2010.05.007, 2010.
Tian, H. Q., Chen, G. S., Zhang, C., Melillo, J. M., and Hall, C. A. S.: Pattern and variation of C : N : P ratios in China's soils: a synthesis of observational data, Biogeochemistry, 98, 139–151, 2010.
Tischer, A., Potthast, K., and Hamer, U.: Land-use and soil depth affect resource and microbial stoichiometry in a tropical mountain rainforest region of southern Ecuador, Oecologia, 175, 375–393, 2014.
Trabucco, A. and Zomer, R. J.: Global Aridity Index (Global-Aridity) and Global Potential Evapo-Transpiration (Global-PET) Geospatial Database, CGIAR Consortium for Spatial Information, CGIAR-CSI GeoPortal, available at: https://cgiarcsi.community/data/global-aridity-and-pet-database/ (last access: 10 April 2020), 2009.
Vance, E. D., Brookes, P. C., and Jenkinson, D. S.: An extraction method for measuring soil microbial biomass C, Soil Biol. Biochem., 19, 703–707, 1987.
Veraart, A. J., de Klein, J. J. M., and Scheffer, M.: Warming Can Boost Denitrification Disproportionately Due to Altered Oxygen Dynamics, PLOS ONE, 6, e18508, https://doi.org/10.1371/journal.pone.0018508, 2011.
Vitousek, P. M. and Farrington, H. J. B.: Nutrient limitation and soil development: Experimental test of a biogeochemical theory, Biogeochemistry, 37, 63–75, https://doi.org/10.1023/A:1005757218475, 1997.
Vitousek, P. M., Porder, S., Houlton, B. Z., and Chadwick, O. A.: Terrestrial phosphorus limitation: mechanisms, implications, and nitrogen-phosphorus interactions, Ecol. Appl., 20, 5–15, https://doi.org/10.1890/08-0127.1, 2010.
Walker, T. W. and Syers, J. K.: The fate of phosphorus during pedogenesis, Geoderma, 15, 1–19, 1976.
Wang, C., Wang, X., Liu, D., Wu, H., Lü, X., Fang, Y., Cheng, W., Luo, W., Jiang, P., Shi, J., Yin, H., Zhou, J., Han, X., and Bai, E.: Aridity threshold in controlling ecosystem nitrogen cycling in arid and semi-arid grasslands, Nat. Commun., 5, 4799, https://doi.org/10.1038/ncomms5799, 2014.
Wu, J., Joergensen, R. G., Pommerening, B., Chaussod, R., and Brookes, P. C.: Measurement of soil microbial biomass C by fumigation-extraction – an automated procedure, Soil Biol. Biochem., 22, 1167–1169, 1990.
Xu, X., Thornton, P. E., and Post, W. M.: A global analysis of soil microbial biomass carbon, nitrogen and phosphorus in terrestrial ecosystems, Global Ecol. Biogeogr., 22, 737–749, 2013.
Yuan, Z. Y., Chen, H. Y. H., and Reich, P. B.: Global-scale latitudinal patterns of plant fine-root nitrogen and phosphorus, Nat. Commun., 2, 344, https://doi.org/10.1038/ncomms1346, 2011.
Zechmeister-Boltenstern, S., Keiblinger, K. M., Mooshammer, M., Peñuelas, J., Richter, A., Sardans, J., and Wanek, W.: The application of ecological stoichiometry to plant–microbial–soil organic matter transformations, Ecol. Monogr., 85, 133–155, 2016.
Zomer, R. J., Trabucco, A., Bossio, D. A., and Verchot, L. V.: Climate change mitigation: A spatial analysis of global land suitability for clean development mechanism afforestation and reforestation, Agr. Ecosyst. Environ., 126, 67–80, doi:10.1016/j.agee.2008.01.014, 2008.