the Creative Commons Attribution 4.0 License.
the Creative Commons Attribution 4.0 License.
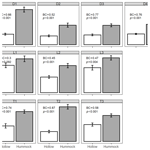
Microtopography is a fundamental organizing structure of vegetation and soil chemistry in black ash wetlands
Daniel L. McLaughlin
Robert A. Slesak
Atticus Stovall
All wetland ecosystems are controlled by water table and soil saturation dynamics, so any local-scale deviation in soil elevation and thus water table position represents variability in this primary control. Wetland microtopography is the structured variability in soil elevation and is typically categorized into a binary classification of local high points (hummocks) and local low points (hollows). Although the influence of microtopography on vegetation composition and biogeochemical processes in wetlands has received attention around the globe, its role in forested wetlands is still less understood. We studied relationships among microtopography and understory vegetation communities, tree biomass, and soil chemistry in 10 black ash (Fraxinus nigra Marshall) wetlands in northern Minnesota, USA. To do so, we combined a 1 cm resolution surface elevation model generated from terrestrial laser scanning (TLS) with colocated water table, vegetation, and soil measurements. We observed that microtopography was an important structural element across sites, where hummocks were loci of greater species richness; greater midstory and canopy basal area; and higher soil concentrations of chloride, phosphorus, and base cations. In contrast, hollows were associated with higher soil nitrate and sulfate concentrations. We also found that the effect of microtopography on vegetation and soils was greater at wetter sites than at drier sites, suggesting that the distance-to-mean water table is a primary determinant of wetland biogeochemistry. These findings highlight clear controls of microtopography on vegetation and soil distributions while also supporting the notion that microtopography arises from feedbacks that concentrate biomass, soil nutrients, and productivity on microsite highs, especially in otherwise wet conditions. We therefore conclude that microtopography is a fundamental organizing structure in black ash wetlands.
- Article
(1837 KB) - Full-text XML
-
Supplement
(1275 KB) - BibTeX
- EndNote
Microtopography is a key component of wetland ecology, influencing a host of fundamental wetland processes. This results from the primacy of shallow water table and soil saturation dynamics in driving wetland vegetation composition and growth and biogeochemical processes (Rodríguez-Iturbe et al., 2007); any variability in soil elevation therefore represents coincident variability in this hydrologic control (Wallis and Raulings, 2011). For example, perhaps incredibly, experiments have demonstrated that even soil surface variability of 2 cm can dramatically increase wetland vegetation germination, overall biomass, and species richness relative to flat soil surfaces (Vivian-Smith, 1997). This microtopographic effect on vegetation community structure is also borne out in real wetlands (though with elevation variation on the order of 10–50 cm), ranging from freshwater sedge meadows (Werner and Zedler, 2002; Peach and Zedler, 2006) to salt marshes (Windham, 1999; Fogel et al., 2004). Further, in many wetlands, primary productivity tends to increase with distance from the water table (Belyea and Clymo, 2001) and high points are often loci of greater primary productivity compared to low points (Strack et al., 2006; Sullivan et al., 2008), driving the rationale behind wetland drainage for increased forestry yields (Laine et al., 1995; Mäkiranta et al., 2010). Microtopography also augments the spatial extent of soil redox gradients (Frei et al., 2012), which largely control wetland biogeochemical processing (DeLaune and Reddy, 2008). However, by far, most studies on wetland microtopography have focused on herbaceous wetlands or northern bog systems dominated by Sphagnum spp. mosses, leaving open questions regarding the commonality of microtopographic influence on wetland processes in forested systems.
The relationship between wetland processes and microtopography is thought to be reciprocal, where vegetation and biogeochemical interactions can in turn support expansion of microtopographic features (Eppinga et al., 2009). That is, wetland microtopography can result from feedbacks among hydrology, vegetation, and soil processes that induce soil elevation divergence into two modes: (1) a high-elevation mode (hummocks) and (2) a low-elevation mode (hollows) (Rietkerk et al., 2004; Eppinga et al., 2008; Heffernan et al., 2013). In previous work, we observed clear microtopographic patterns that we propose arise from these types of ecohydrological feedbacks (Diamond et al., 2019). If these microtopographic patterns are in fact a result of proposed ecohydrological feedbacks, we therefore expect there to be concordant microtopographic differences in vegetation and soils.
The primary hypothesized feedback that results in the observed hummock–hollow microtopography is the productivity–elevation feedback. Preferential colonization by plants on slightly elevated sites leads to local buildup of organic matter, via primary productivity and sediment accretion around roots, and further increased elevation (Gunnarsson and Rydin, 1998; Pouliot et al., 2011). Increased elevation reduces anaerobic stress to plants, leading to further increases in vegetative productivity. This productivity–elevation positive feedback is ultimately constrained and stabilized by increased decomposition rates of accumulated organic matter as hummocks become more aerobic (Belyea and Clymo, 2001; Watts et al., 2010). The resulting microtopography often displays a clear structure, with observations of marked spatial patterns in open bog (Eppinga et al., 2009) and marsh systems (Casey et al., 2016) and potentially in swamp systems as well (Diamond et al., 2019). If these feedbacks are operating in wetlands, the expectation is greater vegetation biomass and productivity on hummocks rather than hollows.
An additional feedback mechanism that can reinforce and maintain wetland microtopography is preferential hummock evapoconcentration of nutrients. Greater productivity and thus greater evapotranspiration rates on hummocks compared to hollows drive a net flow of water and dissolved nutrients toward hummocks (Rietkerk et al., 2004; Wetzel et al., 2005; Eppinga et al., 2008, 2009). Nutrients are consequently rapidly cycled through vascular-plant uptake and plant litter mineralization on the more aerobic hummocks (Malmer et al., 2003), leading to local nutrient concentration effects. This localized nutrient concentration purportedly leads to increased primary productivity, which leads to more nutrient evapoconcentration and so on (Ross et al., 2006). In other words, hummocks may harvest nutrients from hollows, concentrating them there. One clear prediction from this hypothesis is greater nutrient – and conservative water tracer – concentrations in hummock soil relative to hollow soil. To the best of our knowledge, this mechanism remains untested in forested wetlands with hummock–hollow terrain.
In this work, we assessed microtopographic influences on vegetation and soil chemistry in black ash (Fraxinus nigra Marshall) swamps in northern Minnesota, USA. Black ash wetlands are common features throughout the northeastern US and southeastern Canada that range in soil type (e.g., from mineral to peat) and hydrology (e.g., from intermittent to ephemeral). Despite the range in habitat, most late-successional black ash wetlands are characterized by nearly pure black ash stands (i.e., over 90 % canopy cover) with very little regeneration of other tree species (Palik et al., 2012). Importantly, the monotypic nature of black ash systems coupled to existential threats from the invasive emerald ash borer (Agrilus planipennis) has led to black ash being listed as critically endangered (Jerome et al., 2017), making the study of their ecology a timely matter. In a complementary study (Diamond et al., 2019) of black ash wetlands, we observed an evident hummock–hollow structure that was more pronounced at wetter sites. Here, we further investigate the ecological importance of black ash microtopography and ask the following question: to what extent do the integrated controls of water table regimes and microtopography determine spatial variation in vegetation and soil properties?
Specifically, we tested the overall hypothesis that elevation relative to the water table is the primary control on understory composition, tree biomass, and soil chemistry, with the following specific predictions:
-
Understory richness and diversity will be (a) greater at drier sites compared to wetter sites, (b) greater on hummocks than hollows, and (c) positively correlated with elevation relative to the water table.
-
Mid- and canopy-level basal area will be (a) greater at drier sites compared to wetter sites, (b) greater on hummocks than hollows, and (c) positively correlated with elevation relative to the water table.
-
Soil nutrient and conservative tracer (chloride) concentrations will be (a) less variable on drier sites than wetter sites, (b) greater on hummocks than hollows, and (c) positively correlated with elevation relative to the water table.
2.1 Site descriptions
We tested our hypothesis in 10 black ash wetlands of varying size (0.07–0.12 ha) and hydrogeomorphic landscape position in northern Minnesota, USA (Fig. 1). The study region is characterized by a glacial moraine landscape (400–430 m a.s.l.) that is flat to gently rolling, with the black ash wetlands found in lower landscape positions that commonly grade into aspen- or pine-dominated upland forests. Climate is continental with mean annual precipitation of 700 mm and a mean growing season (May–October) temperature of 14.3 ∘C (WRCC, 2019). Annual precipitation is approximately two-thirds rain and one-third snowfall, and potential evapotranspiration is 600–650 mm yr−1 (Sebestyen et al., 2011).
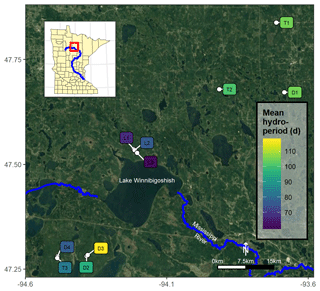
Figure 1Map of the 10 studied black ash wetlands in northern Minnesota, USA, with sites colored by average annual hydroperiod (i.e., number of surface-inundation days per year from May to November) for 2015–2018. © Google Maps 2019.
Black ash stands are commonly highly uneven-aged (Erdmann et al., 1987), with canopy tree ages ranging from 130 to 232 years, and stand development under a gap-scale disturbance regime (D'Amato et al., 2018). Black ash is also typically slow growing, achieving heights of only 10–15 m and diameters at breast height of only 25–30 cm after 100 years (Erdmann et al., 1987).
In previous work (Diamond et al., 2019) and as part of a larger project (D'Amato et al., 2018), we categorized and grouped each wetland site by its hydrogeomorphic characteristics as follows: (1) depression sites (D; n=4) characterized by a convex, pool-type geometry with geographical isolation from other surface water bodies; (2) lowland sites (L; n=3) characterized by flat, gently sloping topography; and (3) transition sites (T; n=3) characterized as flat, linear features between uplands and black spruce (Picea mariana Mill. Britton) bogs. Additional detail on site characteristics is provided in Diamond et al. (2019), but we provide a brief summary of their characteristics here. The studied wetlands varied in soil type, organic layer depth, hydrology, and vegetation but were all characterized by having a black ash canopy dominance of at least 75 %. At the lowland sites, other overstory species were negligible, but at the depression and transition sites there were minor cohorts of northern white-cedar (Thuja occidentalis L.), green ash (Fraxinus pennsylvanica Marshall), red maple (Acer rubrum L.), yellow birch (Betula alleghaniensis Britt.), balsam poplar (Populus balsamifera L.), and black spruce (Picea mariana Mill. Britton). We believe that our sites are late-successional or climax communities and have not been harvested for at least a century. Soils are primarily organic Histosols characterized by deep mucky peats underlain by silty clay mineral horizons, with depression and transition sites having deeper organic layers than lowland sites (Soil Survey Staff, 2019). Depression sites were commonly associated with Terric Haplosaprists with O horizons 30–150 cm deep; lowland sites were associated with poorly drained mineral histic Inceptisols with thin O horizons (<10 cm) underlain by clayey till; transition sites typically had the deepest O horizons (>100 cm) and were associated with Typic Haplosaprists or Typic Haplohemists (Soil Survey Staff, 2019). Although depression and transition sites had thicker O horizons than lowland sites, depression site organic soils were typically muckier and more decomposed than more peat-like transition site soils. We previously characterized hydrology at these sites (Diamond et al., 2019) and found that lowland sites were considerably drier on average than depression or transition sites (note hydroperiods in Fig. 1) and exhibited much more water table variability. Depression sites were typically wetter than transition sites and were more frequently inundated. Depression and transition sites also exhibited significantly more microtopographic structures than lowland sites, with over twice as much elevation variability on average (Diamond et al., 2019).
2.2 Field measurements
We conducted field sampling campaigns to characterize the vegetation and soil chemistry of our study systems. We then coupled these data to previously collected water table and elevation data (Diamond et al., 2019) to address our hypothesis and predictions. Water table data (15 min measurement intervals) were collected at each of our 10 sites from 2016 to 2018, where negative values represent belowground water levels and positive values represent aboveground levels (see Diamond et al., 2019, for details). Elevation data were collected in 2017 using high-resolution terrestrial laser scanning (TLS) measurements within three, randomly placed 300 m2 circular plots at each site (plots were placed at most 50 m from each other to ensure overlap of TLS scans). These data were then used to create 1 cm digital elevation models and to delineate hummock versus hollow features (see method details in Diamond et al., 2019, and Stovall et al., 2019). With these data, we were able to calculate a relative elevation above the mean water table for each vegetation or soil sampling point and to categorize each sampling point as a hummock or hollow.
2.2.1 Understory composition
We characterized understory vegetation at each site to test the prediction that understory richness and diversity will be greater on higher-elevation features. To do so, we used a quasi-random-walk sampling scheme within the 300 m2 plots (three per site) previously used for TLS elevation measurements. We term the sampling design quasi-random because we constrained the random sampling locations by quadrant, allowing us to sample each quadrant of a circular plot approximately equally (13 points per plot; Fig. S1 in the Supplement). At each sampling point, we used a 0.25 m2 square quadrat to classify vascular and moss individuals at the species level, visually estimated their percent foliar cover (1 %, 2 %, and nearest 5 %), and recorded stem count (if possible) for vascular species. We chose a 0.25 m2 size quadrat as it corresponded to the smallest hummock areas that we observed in the field and thus was on the scale of elevation variation at each site. Vegetation surveys occurred during July 2017, coinciding with peak vegetation presence. Vascular-plant identifications were made according to Eggers and Reed (1988), and nonvascular-plant (liverworts and mosses) identifications were made according to Janssens (2014). Species that we were unable to identify in the field were assigned a genus or standard unknown code and collected in a bag for later identification.
2.2.2 Tree biomass
We used two different data sources to assess the prediction that midstory and canopy basal area will be greater on higher-elevation features. The first data source comprised standard stand metrics and was used for our site-level comparisons. These stand data were collected as part of a larger study by the USDA Northern Research Station in Grand Rapids, Minnesota, and included species, basal area, and density for both midstory (2.5 ≤ diameter at breast height (DBH) ≤10 cm) and canopy (>10 cm DBH) for each site.
The second data source was used to relate specific trees and their basal area to a specific base elevation relative to the water table and employed plot-scale point clouds of forest structure from the aforementioned TLS campaign (see Stovall et al., 2019). We estimated overstory DBH (i.e., > 2.5 cm) from these TLS point clouds with the SimpleTree algorithm implemented in Computree (Hackenberg et al., 2015). The SimpleTree algorithm models trees as cylinders by segmenting trees using an iterative nearest-neighbor approach that moves vertically from an initial seed point along the stem while expanding in area with increasing crown size. The best least-squares cylinder at approximately 1.3 m above ground provided estimates of DBH. Following DBH analysis, we matched each processed tree with an elevation value from our original TLS analysis (Stovall et al., 2019). We were only able to apply the SimpleTree algorithm at six of our sites and only on a fraction of our scanned areas at those sites (approximately 300 m2 at each of the six sites), due to resolution issues and understory noise.
2.2.3 Soil chemistry
To assess the prediction that soil chemistry heterogeneity will covary with elevation variability, we cored soil at a subsample of the 390 sampling points in the previously described quasi-random-walk sampling design. We determined the subsampling points prior to site arrival with the intention of sampling all points at a minimum of one plot (13 points) per site. We sampled one plot at sites D2, D3, D4, and T3 but two plots at the remaining sites for a total of 208 sampling points out of our original 390. We first removed the top layer of moss and litter and then used a 15 cm beveled and serrated soil knife to extract our soil samples (approximately 5 cm in diameter at the soil surface) to a depth of 10 cm.
Following standard methods (DeLaune and Reddy, 2008; U.S. EPA, 2008), we air-dried soil samples over 2 weeks; removed visible roots (typically greater than 2 mm in diameter); and hand-ground, mixed, and sieved soils to pass a 2 mm mesh to create a representative sample of the 10 cm soil increment. Total carbon and nitrogen concentrations were determined with dry combustion on 0.25 g subsamples with a CN elemental analyzer (vario MAX; elementar Analysensysteme GmbH, Langenselbold, Germany). For anions and cations, we used a modified water extraction method (Jones and Willett, 2006). Nitrate () and phosphate () were analyzed colorimetrically with a segmented flow analyzer (SEAL AA3; SEAL Analytical, Mequon, WI, USA) using equipment methods G-200-97 and G-175-96, respectively. Chloride (Cl−), sulfate (), calcium (Ca2+), and magnesium (Mg2+) were analyzed with ion chromatography (Standard Methods 4110, Dionex ICS-3000; Thermo Fisher Scientific, Waltham, MA, USA).
2.3 Data analysis
Across our different environmental variables, we took the following general approach: (1) test for site-scale differences focusing on site water table metrics (e.g., mean, median, and variance) as predicting variables, (2) test for microsite-scale (hummock vs. hollow) categorical differences, and (3) test for point-scale influences of elevation relative to the water table.
2.3.1 Understory composition
To test our prediction that site hydrology is a strong control on understory composition, we regressed mean (n=3 plots) site-level richness and diversity with site-level hydrology metrics using simple linear regression. To assess categorical differences in understory vegetation composition among both sites and microsites (i.e., hummock vs. hollow), we used a multivariate permutational analysis of variance (PERMANOVA) on understory vegetation in ordination space. For ordination, we used nonmetric multidimensional scaling (NMDS) with the vegan package (Oksanen et al., 2018) in the R statistical software (R Core Team, 2018). Prior to ordination, we removed rare species (<1 % presence overall) from the understory community matrix (McCune and Grace, 2002). We also evaluated species fidelity and association with particular sites and microsites (hummocks versus hollows) using the indicspecies R package (Cáceres and Legendre, 2009). This analysis generates an indicator value index (IV) for each species within each category (e.g., site or microsite) based on two metrics: specificity (i.e., the probability that a sample point belongs to a particular group, given that the species was found there) and sensitivity (i.e., the probability that the species is found in sample points belonging to a particular group). To remove the influence of rare species on the indicator analysis, we limited the candidate species to those that were present in more than 10 % of their identified microsite or site category. Using this reduced sample, we identified species or species combinations that had a specificity of at least 0.80 and a sensitivity of at least 0.20, based on observations of clear delineations in the indicator species output and on guidance in package materials (Cáceres, 2013). We also conducted Welch's two-sample t test on richness between hummocks and hollows for each site and calculated hummock–hollow Bray–Curtis community dissimilarity indices, which fall between 0 and 1, where 1 indicates complete dissimilarity and 0 indicates identical communities. The t test allowed us to test our prediction that hummocks were more diverse than hollows within a site, and the dissimilarity index allowed us to further determine how different the vegetation communities were. Last, we analyzed within-site relationships between point-scale understory richness and point-scale elevation relative to the mean water table using a generalized linear mixed-effect model (GLMM). We conducted GLMM analysis with the lme4 R package (Bates et al., 2015) using suggested methods for Poisson distributions from Bolker et al. (2009) and chose the best model based on the Akaike information criterion (AIC) and Bayesian information criterion (BIC).
2.3.2 Tree biomass
We tested our prediction that site-scale hydrology influences tree biomass by regressing site-scale (midstory and canopy) basal area with site-scale water table metrics. To determine categorical differences in tree biomass between hummocks and hollows, we used individual tree DBH data (from the TLS data) and compared the cumulative basal area between hummocks and hollows. We then estimated the fraction of tree basal area at each site that occupied hummocks relative to hollows. Finally, we analyzed within-site relationships between point-scale DBH (from the TLS data) and tree base elevations relative to the mean water table using a linear mixed-effect model (using the lme4 R package; Bates et al., 2015).
2.3.3 Soil chemistry
To test the prediction that there would be less variation in soil chemistry at drier sites compared to wetter sites, we conducted standard ANOVA and post hoc Tukey's honestly significant difference t test on soil extraction chemistry. We first examined differences in each analyte among hydrogeomorphic categories (via Levene's test on group variance), and then tested differences among individual sites. To assess microsite influence on soil extraction chemistry, we examined differences between hummocks and hollows, averaged across sites. Across-site comparison of hummocks and hollows (as opposed to within-site comparison) increased the power of our inference because, due to our random sampling, some sites did not have equal measurements of hummocks and hollows. Prior to averaging across sites, we normalized soil extraction concentrations to the site-level average concentration for each analyte to compare relative differences between hummocks and hollows across sites, even when absolute concentrations differed among sites. Normalized concentrations were compared between hummocks and hollows across sites using Welch's two-sample t test; we also calculated ratios of hollow-to-hummock concentrations to assess differences, regardless of among-site variability in absolute concentrations. Finally, we regressed point-scale soil analyte concentrations versus local elevation relative to the water table using best-fit linear mixed-effect modeling (via the lme4 R package; Bates et al., 2015).
3.1 Understory composition
Across all sites (30 plots), we observed 95 distinct understory species: 9 moss species, 85 vascular species, and 1 liverwort species. The most common vascular species were sedges of the Carex genus, grasses of the Glyceria genus, Impatiens capensis Meerb., Aster lateriflorus (L.) Britton, and Caltha palustris (L.). The most common mosses were Calliergon cordifolium (Hedw.) Kindb., Thuidium delicatulum (Hedw.) Schimp., and Rhizomnium magnifolium (Horik.) T. Kop.
We observed a clear influence of site-scale hydrology on site-scale community composition, with the mean and median daily water table being the best predictors. The median daily water table was a linear predictor of both understory richness (; p=0.003) and diversity (; p<0.001; Fig. 2). The mean water table, to a lesser extent, also explained similar amounts of site-level variance in richness (R2=0.25) or diversity (R2=0.29). Lowland sites and transition sites tended to clump together in this relationship, but depression sites exhibited far more intra- and across-site variability in richness and hydrology.
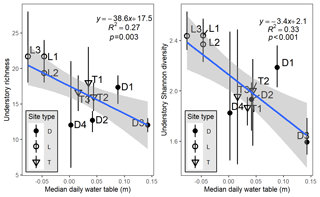
Figure 2Plot-level richness or diversity, aggregated by site, as a function of the site-level median water table relative to the ground surface (negative values indicate belowground). Letters and symbols refer to hydrogeomorphic site types: D refers to depression sites, L refers to lowland sites, and T refers to transition sites. Vertical bars on points indicate bootstrapped 95 % credible intervals calculated from the three plot measurements per site. The linear regression model results presented are also shown. Hydrology summaries are provided in Table S2.
NMDS demonstrated clear ordinal separation of the understory community matrix between hummocks and hollows across sites (PERMANOVA; ; p=0.002) and between hummocks and hollows within sites (PERMANOVA; ; p=0.001; Fig. S2). Hummocks and hollows were more similar for lowland sites, particularly L1 and L3, compared to depression and transition sites (Fig. S2).
Indicator species analysis revealed that four moss species (Climacium dendroides (Hedw.) F. Weber & D. Mohr, Funaria hygrometrica Hedw., Rhizomnium magnifolium (Horik.) T. Kop., and Thuidium delicatulum (Hedw.) Schimp.) were the most distinguishing species found on hummocks across sites (Table 1). The best hummock indicator species was Climacium dendroides (Hedw.) F. Weber & D. Mohr, with it having an 87 % chance of indicating that a sampling point is on a hummock (specificity) and a 59 % chance that it will be present at a point, given that the point is a hummock (sensitivity). Similarly, for hollows, a moss species (Calliergon cordifolium (Hedw.) Kindb.) was the best indicator species, although common duckweed (Lemna minor L.) had a nearly perfect (99 %) chance of indicating that a sampling point was a hollow. When we removed the criterion for across-site species presence (>10 %), we observed approximately an order of magnitude more candidate indicator species for hummocks than for hollows, with most species having very high specificity (Table S1 in the Supplement).
Table 1Indicator species analysis for hummocks and hollows across sites. Species indicator values (IV) range from 0 to 1 and are the product of specificity and sensitivity conditional probabilities. Specificity is the conditional probability that the sampling point belongs to a particular microsite, given the fact that a particular species was found there, and sensitivity is the conditional probability of finding a particular species in a sampling point, given that the sampling point belongs to a particular microsite.
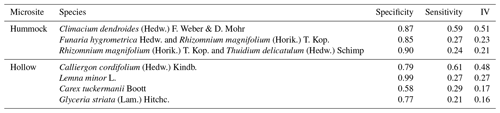
We also observed distinct differences in understory richness between hummock and hollow microsites. Hummocks were nearly always locations of greater combined moss and vascular-plant species richness (Fig. 3). This pattern was identical when separating mosses from vascular plants (Fig. S3). We found the greatest hummock–hollow differences in understory species richness in depression sites (mean water table = 0.01 m), with less difference in transition sites (mean water table = −0.04 m) and lowland sites (mean water table = −0.32 m; see Table S2 for site hydrology summaries). Bray–Curtis dissimilarities for both mosses and understory vascular plants were greatest for depression and transition sites (BC values in Figs. 3 and S3).
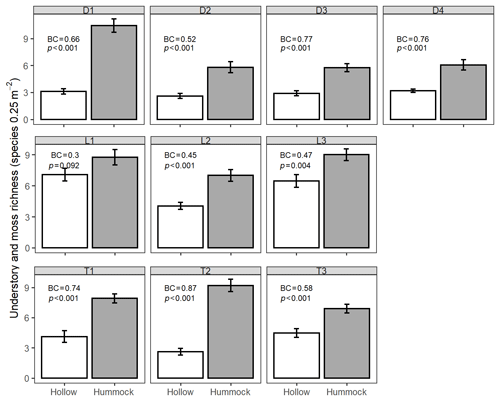
Figure 3Combined understory vascular and moss richness hummock and hollow comparison. Text values indicate community dissimilarity indices and Welch's two-sample t test results. BC text values indicate Bray–Curtis dissimilarity, with a 0–1 range, spanning identical (0) to completely dissimilar (1) vegetation communities.
At the point scale, the best-fit GLMM for richness versus elevation relative to the mean water table was one with site-level random effects for both intercept and slope, as well as a dummy variable for moss (contrasted with vascular understory vegetation; Table 2). Although random site effects modulated the richness–elevation relationship, all site slopes were significantly greater than 0 (see site-specific fits in Fig. S4), implying a positive association between richness and elevation across sites. Importantly, drier lowland sites had lower overall slopes (i.e., more negative random slope effects) compared to wetter sites (Fig. S4a), indicating less rapid increases in richness with increased elevation at dry sites. Overall, we observed that moss richness increased approximately 22 % less than vascular-plant richness with increasing elevation. To visualize more clearly the results from this point-scale analysis, we plotted GLMM-predicted richness values as a function of relative elevation above the water table without considering site effects (Fig. 4). Both moss and vascular plants exhibited only one or two species when at sampling points near or below the mean water table, but species counts increased rapidly beginning near the mean water table, notably for vascular plants.
Table 2GLMM results for species richness versus relative elevation.
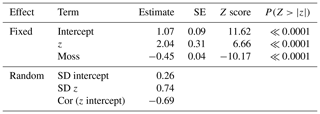
Note: Random effects are presented here as the standard deviation (SD) of all site effects on intercept and slope, with correlation (Cor) between random intercept and slopes. Figure S3 has individual site effects.
3.2 Tree biomass
There was no significant linear relationship (p<0.05) between basal area and hydrologic metrics for either the canopy or midstory level, at the site scale. We observed that T1 was a major outlier in the midstory basal area–elevation relationship, but its omission did not result in a significant fit (p=0.137).
Using our TLS-derived DBH data at a subset of sites (n=6), we further assessed differences between hummocks and hollows. Total basal area was disproportionately (by an order of magnitude) associated with hummocks at the wettest sites (D1, D3, D4, and T1), but the relative lack of hummocks at the drier sites (L1 and L2) inverted this relationship (Table 3). Further, across all size classes, we found that trees in the wettest sites (depression and transition) occupied hummocks 83 %–94 % of the time (Fig. S5). We also observed a size-dependent association with hummocks, especially for sites D1, D3, and T1, where larger trees (i.e., trees with DBH > 20 cm) were 2–3 × more likely to exist on hummocks compared to hollows (Fig. S5). This is in contrast to drier lowland sites, where trees do not prefer hummocks to hollows, at least not in our subsampled areas.
Point-scale linear mixed-effect models of DBH versus relative elevation did not reveal any significant (p>0.05) relationship (fixed effect = 2.2±2.8 cm m−1; t=0.77; and see Fig. S6). We note here, however, that the matching procedures to tie digital elevation models from TLS to tree base height elevations likely have high uncertainty.
3.3 Soil chemistry
There were clear differences in soil chemistry among site hydrogeomorphic groups and among individual sites for all analytes (ANOVA; F2184–202 = 5.1–143.8; p<0.01; Fig. 5). Depression sites had the lowest soil base cation concentrations (Ca2+ and Mg2+), followed by lowland and then transition sites. Depression sites and transition sites had considerably less –N than lowland sites but somewhat more –P and clearly higher C : N. However, there was more variability among sites than among hydrogeomorphic site groupings. There was some indication that drier lowland sites exhibited less variability in soil chemistry than wetter transition and depression sites, but this trend was not consistent across analytes (Table S3). For example, we observed significantly greater variance in %C, –N, and in drier lowland sites than in wetter sites (Table S3).
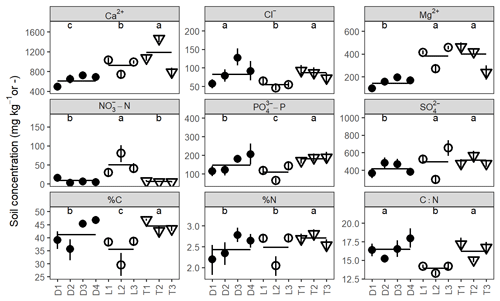
Figure 5Average soil extraction concentrations for every site and solute analyzed. Shapes correspond with site hydrogeomorphic type; vertical bars indicate bootstrapped 95 % confidence intervals about the mean for each site, and horizontal bars indicate means within groups. Significantly different groups at p=0.05 are labeled with letters. Note: %C, %N, and C : N are unitless (–) and are determined from combustion, not soil extraction.
We also found differences (Welch's two-sample t test; p<0.05) in soil chemistry between hummocks and hollows for seven out of nine analytes (Fig. 6). Except for –N, %N, and , hummocks had higher analyte concentrations than hollows. Across-site hummock–hollow differences in mean concentrations ranged from −27 % for –N to +23 % for Cl−. Although some sites varied in their relative differences between hummock and hollow analyte concentrations (Table S4), broad patterns were still discernible wherein hummocks were generally loci of higher Ca2+, Cl−, Mg2+, –P, C, and C : N (though only by 4 %) relative to hollows.
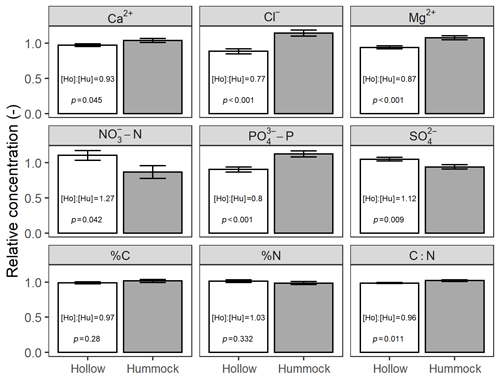
Figure 6Across-site comparison between hummocks and hollows of relative concentrations of soil analytes. Relative concentration for any particular sample is normalized to its site-average concentration. Text in each panel refers to the across-site ratio of hollow-to-hummock relative concentrations and the Welch's two-sample t test of hollow and hummock means.
We found strong linear relationships between concentration and relative elevation above the water table at the sample point scale for six out of nine soil chemistry analytes (Figs. S7, S8). Results from this point-scale linear fitting align with categorical results from hummock and hollow analysis (Fig. S8). Some analytes varied much more among sites in the concentration–elevation relationship than others, leading to large variability in some best-fit lines (e.g., Ca2+, NO3−–N), but most analyte concentrations had clear linear relationships with elevation (Fig. S7). The linear mixed-effect models were fit with a restricted maximum likelihood estimation with uncorrelated random intercepts and slopes; standardized residuals were normally distributed about 0. Random site effects modulated the overall concentration–elevation relationship, implying large variability in responses (direction and magnitude) among sites (Table S5). We did not observe clear patterns in random effects relating to sites or site hydrogeomorphic groupings (Fig. S7), indicating no obvious control of hydrology or setting on the strength of these relationships. However, relationships for –P and Cl− were similar in wetter depression and transition sites in contrast to drier lowland sites, which did not have as steeply positive linear relationships with elevation.
Using integrated measures of fine-scale topography, water table regimes, and vegetation and soil attributes, our work highlights the primary control of elevation and microsite position in black ash wetlands. Findings demonstrate these controls on vegetation distributions, tree biomass, and soil chemistry at both site and within-site scales, driven by distance to the water table and thus decreased anaerobic stress at drier sites and on elevated hummocks at wetter sites. We suggest that these results support feedback models of hummock–hollow development, where increased vegetative productivity at higher microsites leads to increased microsite elevation with reciprocal controls on vegetation composition and soil chemistry at site and microsite scales.
4.1 Controls on understory composition
Site-scale hydrologic behavior of black ash wetlands is a major determinant of site-scale understory richness and diversity. We found that even a simple hydrologic metric like median water table could explain 30 % of intersite understory richness variability (Fig. 2). For example, our wettest site had half of the species richness of our driest site and was two-thirds as diverse. Numerous other studies have observed the influence of hydrologic regime on site-scale species richness (e.g., van der Valk et al., 1994; Nielsen and Chick, 1997; Nielsen et al., 2013), but most have been based on experimental studies of expected hydrologic change or carried out in riparian systems dominated by flood pulses. This study demonstrates that black ash wetlands, which are abundant ecosystems in the Great Lakes region (e.g., they cover approximately 5 % of forested land in Minnesota, Michigan, and Wisconsin; USDA Forest Service, 2016), may exhibit similar hydrology–richness responses as other studied wetland systems and further solidifies hydrology as the primary determinant of understory species distributions in wetlands.
Despite clear site-scale hydrologic controls on understory richness, we also observed a dominant microsite-scale influence on community composition. Both NMDS analysis and Bray–Curtis dissimilatory indices indicated not only that hummocks and hollows separated along community structure but also that the degree of this separation was highly site dependent. For example, drier lowland sites (especially L1 and L3) had considerably less understory community variation between microsites than the wetter depression or transition sites, supporting the notion that distinct and functionally important microsite states arise in response to wet conditions. Assessment of species fidelity to specific microsites provided further support that hummocks and hollows are discrete ecosystem states (cf. Watts et al., 2010). We suggest that the presence of microtopography increases overall site richness, a finding supported by similar studies of richness and microsite variability (Beatty, 1984; Vivian-Smith, 1997; Bruland and Richardson, 2005). Therefore, microtopography greatly expands potential hydrologic and associated habitat niches. It would be informative to take a larger-scale perspective by assessing the importance of each microsite to overall landscape level biodiversity and through comparison of hummock species compositions to those of surrounding upland forests.
In addition to different community structure between hummocks and hollows, we found strong evidence for our prediction that hummocks support a higher number and greater diversity of understory vegetation species than hollows (Fig. 3). In our systems, hummocks were loci for approximately 60 % of total sampled species per site, with larger richness differences between hummocks and hollows in wetter sites. This finding aligns with field observations of visually distinct breaks between the relatively bare organic surface of hollows and the verdant structure of hummocks. We suggest that greater understory richness on hummocks may imply greater understory gross primary production vis-à-vis the richness–productivity relationship (Olde Venterink et al., 2003; van Ruijven and Berendse, 2005), lending credence to a productivity–elevation feedback. Adding further support, modeling demonstrated clear increases in richness with elevation, where the slope of this relationship was greatest at the wettest sites (Figs. 4 and S4). Perhaps these are not surprising results given that distance to the water table may be the most important control on wetland community structure (Bubier et al., 2006; Økland et al., 2008; Malhotra et al., 2016). Our results add black ash swamps to a number of wetland ecosystems with similar understory microtopography–richness structures, including salt marshes (Stribling et al., 2006), alluvial swamps (Bledsoe and Shear, 2000), tidal freshwater swamps (Duberstein and Conner, 2009; Courtwright and Findlay, 2011), tropical swamps (Koponen et al., 2004), boreal swamps (Økland et al., 2008), and northern sedge meadows (Peach and Zedler, 2006). The concordance of similar observations across systems substantiates hypotheses that hummocks play a critical role in supporting wetland plant diversity.
Lastly, we note that, while hollows have fewer species on average than hummocks, they are not devoid of understory productivity. At some sites, we observed large swaths of Carex spp. in the hollow understory, whose thick stems and spanning rhizomes would have contributed to high primary productivity. Follow-up studies could focus on this aspect of hummock–hollow differences in these and other systems.
4.2 Controls on tree biomass
Although not a significant direct association, we found some indirect evidence that links site-scale hydrology to tree basal area. The driest sites (lowland sites) had the greatest basal area (Table S2), supporting observations from floodplain wetlands where sites that received less inundation were more productive and had greater basal area than intermediately or regularly inundated sites (Megonigal et al., 1997). However, other drivers may also influence these differences, including variability in disturbance regime or pedological characteristics that were outside the scope of this study.
Our findings also highlight local hydrologic influences and demonstrate that trees at wetter sites almost exclusively occupy raised hummocks; almost the entirety of live basal area corresponded directly to elevated hummock structures in the wettest black ash systems (Fig. S5). A recent study of canopy competition in black ash wetlands acknowledged this importance of microsite variation in explaining lack of predicted competition and subsequent tree size distributions among black ash trees (Looney et al., 2016). In contrast to our findings at the site level, we did not find support for our prediction that basal area would correlate with tree base elevation within sites. However, this may not be surprising for three primary reasons: (1) black ash trees are extremely slow growing, and there can be very little discernible variability in DBH across trees of different age classes (D'Amato et al., 2018; Looney et al., 2018); (2) hummock heights (and thus tree base elevation), while centered around some site mean, exhibit variation within a site, leading to a range of elevations supporting trees with similar DBH; and (3) tree base elevations were difficult to ascertain using our TLS matching method, leading to high uncertainty in elevation measurements. Perhaps in wetter black ash systems, trees that establish and survive eventually reach similar sizes based on resource constraints or growth patterns, but it is more common that they establish and survive on hummocks at the wettest sites.
4.3 Controls on soil chemistry
We observed clear and significant differences in soil chemistry among sites that could broadly be attributed to site-scale hydrology and site hydrogeomorphic category. For example, the drier lowland sites had an order of magnitude greater –N soil concentrations than wetter depression or transition sites (Fig. 5) despite having nearly equal soil total N (CV = 0.1). We suggest that the water table regimes of our sites correspond directly with expected water table and soil-specific shifts in N processing. In general, where water tables are deeper below the ground surface (e.g., by 30 cm or more), net nitrification dominates; where water tables are shallower (e.g., within 10 cm of the surface), net ammonification dominates (Hefting et al., 2004). Specific to our prediction, we found some support that within-site variation in analytes (specifically for Cl−, –P, C : N, %N, and Mg2+) was greater at wetter sites, suggestive of more variable redox conditions and biogeochemical processing.
We found some support that hummocks can act as evapoconcentrators of mobile soil chemical pools. The strongest evidence for this comes from the relatively high level of the conservative tracer, chloride, in hummocks relative to hollows (23 % greater on average; Fig. 6). Chloride is commonly used across scales and systems as a hydrologic tracer to evaluate hydrologic storage and transport processes (Kirchner et al., 2000; Kirchner et al., 2010), and its concentration in terrestrial waters is widely attributed to evaporation effects (e.g., Thorslund et al., 2018). In northern bogs, encroachment or presence of woody vascular plants can dramatically increase evapotranspiration losses (Takagi et al., 1999; Frankl and Schmeidl, 2000), aligning with results here and suggesting that presence of black ash trees and their associated understory hummock species may be significant drivers of evapotranspiration on hummocks.
The significantly greater amounts of soil phosphorus on hummocks also indicate directional concentration from hollows to hummocks. Eppinga et al. (2008) were the first to empirically test and provide evidence for hummock evapoconcentration of limiting nutrients, which had previously been suggested as a mechanism inducing greater phosphorus on tree islands in the Everglades, Florida (Wetzel et al., 2005; Ross et al., 2006). In addition to evapoconcentration, mechanisms of increased nutrient availability in hummocks relative to hollows may be also attributed to accumulation of debris and litter (Resler and Stine, 2009) and/or higher turnover and cycling rates (Wetzel et al., 2005). Both the mass of absorptive fine roots (Li et al., 2020) and mycorrhizal activity are greater in hummocks than hollows, which may be important for P acquisition from ferric-bound particles (Cantelmo Jr. and Ehrenfeld, 1999). Overall, our finding of greater phosphorus on hummocks aligns with numerous studies where hummocks are consistently found to be zones of greater phosphorus concentrations than hollows (Jones et al., 1996; Wetzel et al., 2005; Eppinga et al., 2008).
Hummocks were also enriched in base cations compared to hollows. We postulate that this base cation enrichment effect may be a result of preferential uptake and rapid nutrient recycling by black ash trees located on hummocks. Black ash trees are known to exhibit considerably higher Ca2+ and Mg2+ in live tissues than neighboring species at the same site or in other nearby ecosystems (Reiners and Reiners, 1970), indicating preferential uptake of these nutrients relative to other species.
In contrast, we observed that hummocks were sites of lower nitrate concentrations, which contrasts with findings from other microtopographic studies (e.g., Bruland and Richardson, 2005). However, Courtwright and Findlay (2011) also observed hollow nitrate enrichment, which they attributed to biologically mediated effects such as enhanced uptake on hummocks and coupled nitrification–denitrification. However, the unexpected –N enrichment on hollows in this study may simply be due to sampling after leaf fall, which may have transferred –N to hollows, or perhaps because soils were recently aerobic, allowing for nitrification to proceed in hollows. Additionally, sample drying procedures may have led to relatively greater oxidation of ammonium in hollow samples compared to hummock samples.
Results for were also in contrast to what we expected, as we had surmised that oxidized would be greater in aerobic hummocks than in more anaerobic hollows. Our results also contrast with observations in saltwater systems, where vegetated zones are areas of increased due to root-zone oxygenation of reduced toxic sulfides (Hsieh and Yang, 1997; Madureira et al., 1997; Ferreira et al., 2007), and also contrast with coupled sulfur hydrology–microtopography modeling exercises for freshwater systems (Frei et al., 2012). Similar to nitrate, some of this discordance may be attributed to soil drying procedures, which could have allowed time for oxidation of previously reduced sulfur compounds in hollow samples.
We found some evidence for our hypothesis that relative elevation, as opposed to simply microsite position, was a major control on soil chemical pools in black ash wetlands (Figs. S7 and S8). Unsurprisingly, all analyte–elevation trends directly corresponded with categorical hummock–hollow trends, both in direction and in strength. We also observed some indication that Cl− and –P exhibited more similar and more positive relationships with elevation in wetter sites compared to drier sites, potentially indicating enhanced evapoconcentration at wetter sites. Whereas most other studies examining relative-elevation effects on soil chemistry use sample depth to water table as their elevation measurement (e.g., Bubier et al., 2006; Stribling et al., 2006), our study took a combined approach where we monitored water table regimes at one location but measured relative surface elevation in high resolution at all points. Hence, two major underlying assumptions in our approach are that the water table is flat across our study area and that capillary fringe and/or hydraulic redistribution effects are negligible in comparison to water table fluctuations. Without field observations we cannot verify this assumption but believe it is reasonable considering the relatively small site areas and flat terrain. We concede that this approach may be inappropriate for other systems with less organic soils or more undulating terrain but note that it appeared to provide reasonable and practical results in our case.
Our work provides a strong foundation for viewing microtopography as a primary determinant of soil chemistry distributions in black ash wetlands. Future studies could explore differences in evapotranspiration rates between hummocks and hollows for further elucidation of evapoconcentration differences. Inferences along these lines would also be bolstered by leaf tissue measurements on hummocks and hollows to test for limiting nutrients. We also suggest investigating, at the microsite level, additional species of nitrogen (e.g., ammonium), sulfur (e.g., sulfide), and other important redox compounds (e.g., iron) and biogeochemical processes (e.g., denitrification) that may explain observed trends in soil chemistry.
This work provides support for ecosystem engineering by vegetation in forested wetlands, where vegetation capitalizes on and amplifies small changes in surface elevation. The result of this engineering is hummock–hollow microtopography, where hummocks and hollows are distinct, self-organized ecosystem states. Here we used the case study of black ash wetlands to illustrate this possibility. Importantly, we found that black ash hummocks are characterized by increased understory species richness, tree biomass, and nutrient availability, all of which are likely due to reduced hydrologic stress. We conclude the following: (1) vegetation preferentially occupies and reinforces hummock structure and (2) hummock and hollow microtopography yields predictable patterns of vegetation and soil chemistry. Therefore, we infer that microtopography is a fundamental organizing structure of many black ash wetlands, particularly those that undergo wetter conditions and have shallow water tables.
All data and code for analysis are available from the authors on request.
The supplement related to this article is available online at: https://doi.org/10.5194/bg-17-901-2020-supplement.
JSD, DLM, and RAS designed the study, and JSD carried it out. JSD conducted all analyses with input from all coauthors. JSD prepared the paper with contributions from all coauthors.
The authors declare that they have no conflict of interest.
This project was funded by the Minnesota Environmental and Natural Resources Trust Fund, the USDA Forest Service Northern Research Station, and the Minnesota Forest Resources Council. Additional funding was provided by the Virginia Tech Department of Forest Resources and Environmental Conservation, the Virginia Tech Institute for Critical Technology and Applied Science, and the Virginia Tech William J. Dann Fellowship. We gratefully acknowledge the fieldwork and data collection assistance provided by Mitch Slater, Alan Toczydlowksi, and Hannah Friesen. We also gratefully acknowledge Breanna Anderson for assistance with soil sample processing, David Mitchem for assistance in sample preparation and analysis, and Kelly Peeler for assistance in soil sample processing.
This paper was edited by Paul Stoy and reviewed by three anonymous referees.
Bates, D., Maechler, M., Bolker, B., and Walker, S.: Fitting Linear Mixed-Effects Models Using lme4, J. Stat. Softw., 67, 1–48, https://doi.org/10.18637/jss.v067.i01, 2015.
Beatty, S. W.: Influence of Microtopography and Canopy Species on Spatial Patterns of Forest Understory Plants, Ecology, 65, 1406–1419, 1984.
Belyea, L. R. and Clymo, R. S.: Feedback control of the rate of peat formation, P. Roy. Soc. B-Biol. Sci., 268, 1315–1321, 2001.
Bledsoe, B. P. and Shear, T. H.: Vegetation along hydrologic and edaphic gradients in a North Carolina coastal plain creek bottom and implications for restoration, Wetlands, 20, 126–147, 2000.
Bolker, B. M., Brooks, M. E., Clark, C. J., Geange, S. W., Poulsen, J. R., Stevens, M. H. H., and White, J. S. S.: Generalized linear mixed models: a practical guide for ecology and evolution, Trends Ecol. Evol., 24, 127–135, 2009.
Bruland, G. L. and Richardson, C. J.: Hydrologic, edaphic, and vegetative responses to microtopographic reestablishment in a restored wetland, Restor. Ecol., 13, 515–523, 2005.
Bubier, J. L., Moore, T. R., and Crosby, G.: Fine-scale vegetation distribution in a cool temperate peatland, Botany, 84, 910–923, 2006.
Cáceres, M. D.: How to use the indicspecies package (ver. 1.7. 1), R Proj, 29, available at: https://cran.r-project.org/web/packages/indicspecies/vignettes/indicspeciesTutorial.pdf (last access: 19 February 2020) 2013.
Cáceres, M. D. and Legendre, P.: Associations between species and groups of sites: indices and statistical inference, Ecology, 90, 3566–3574, 2009.
Cantelmo Jr., A. J. and Ehrenfeld, J. G.: Effects of microtopography on mycorrhizal infection in Atlantic white cedar (Chamaecyparis thyoides (L.) Mills.), Mycorrhiza, 8, 175–180, 1999.
Casey, S. T., Cohen, M. J., Acharya, S., Kaplan, D. A., and Jawitz, J. W.: Hydrologic controls on aperiodic spatial organization of the ridge–slough patterned landscape, Hydrol. Earth Syst. Sci., 20, 4457–4467, https://doi.org/10.5194/hess-20-4457-2016, 2016.
Courtwright, J. and Findlay, S. E. G.: Effects of microtopography on hydrology, physicochemistry, and vegetation in a tidal swamp of the Hudson River, Wetlands, 31, 239–249, 2011.
D'Amato, A., Palik, B., Slesak, R., Edge, G., Matula, C., and Bronson, D.: Evaluating Adaptive Management Options for Black Ash Forests in the Face of Emerald Ash Borer Invasion, Forests, 9, 348, https://doi.org/10.3390/f9060348, 2018.
DeLaune, R. D. and Reddy, K. R.: Biogeochemistry of Wetlands: Science and Applications, p. 590, CRC press, Boca Raton FL, 2008.
Diamond, J. S., McLaughlin, D. L., Slesak, R. A., and Stovall, A.: Pattern and structure of microtopography implies autogenic origins in forested wetlands, Hydrol. Earth Syst. Sci., 23, 5069–5088, https://doi.org/10.5194/hess-23-5069-2019, 2019.
Duberstein, J. A. and Conner, W. H.: Use of hummocks and hollows by trees in tidal freshwater forested wetlands along the Savannah River, Forest Ecol. Manag., 258, 1613–1618, 2009.
Eggers, S. D. and Reed, D. M.: Wetland plants and plant communities of Minnesota & Wisconsin, US Army Corps of Engineers, St. Paul District, 1988.
Eppinga, M. B., Rietkerk, M., Borren, W., Lapshina, E. D., Bleuten, W., and Wassen, M. J.: Regular surface patterning of peatlands: confronting theory with field data, Ecosystems, 11, 520–536, 2008.
Eppinga, M. B., De Ruiter, P. C., Wassen, M. J., and Rietkerk, M.: Nutrients and hydrology indicate the driving mechanisms of peatland surface patterning, Am. Nat., 173, 803–818, 2009.
Erdmann, G. G., Crow, T. R., Ralph Jr., M., and Wilson, C. D.: Managing black ash in the Lake States, General Technical Report NC-115, St. Paul, MN: US Dept. of Agriculture, Forest Service, North Central Forest Experiment Station, 115 pp., 1987.
Ferreira, T. O., Otero, X. L., Vidal-Torrado, P., and Macías, F.: Effects of bioturbation by root and crab activity on iron and sulfur biogeochemistry in mangrove substrate, Geoderma, 142, 36–46, 2007.
Fogel, B. N., Crain, C. M., and Bertness, M. D.: Community level engineering effects of Triglochin maritima (seaside arrowgrass) in a salt marsh in northern New England, USA, J. Ecol., 92, 589–597, 2004.
Frankl, R. and Schmeidl, H.: Vegetation change in a South German raised bog: Ecosystem engineering by plant species, vegetation switch or ecosystem level feedback mechanisms?, Flora, 195, 267–276, 2000.
Frei, S., Knorr, K. H., Peiffer, S., and Fleckenstein, J. H.: Surface micro-topography causes hot spots of biogeochemical activity in wetland systems: A virtual modeling experiment, J. Geophys. Res.-Biogeo., 117, G00N12, https://doi.org/10.1029/2012JG002012, 2012.
Gunnarsson, U. and Rydin, H.: Demography and recruitment of Scots pine on raised bogs in eastern Sweden and relationships to microhabitat differentiation, Wetlands, 18, 133–141, 1998.
Hackenberg, J., Spiecker, H., Calders, K., Disney, M., and Raumonen, P.: SimpleTree – an efficient open source tool to build tree models from TLS clouds, Forests, 6, 4245–4294, 2015.
Heffernan, J. B., Watts, D. L., and Cohen, M. J.: Discharge competence and pattern formation in peatlands: a meta-ecosystem model of the Everglades ridge-slough landscape, PloS one, 8, e64174, https://doi.org/10.1371/journal.pone.0064174, 2013.
Hefting, M., Clément, J. C., Dowrick, D., Cosandey, A. C., Bernal, S., Cimpian, C., Tatur, A., Burt, T. P., and Pinay, G.: Water table elevation controls on soil nitrogen cycling in riparian wetlands along a European climatic gradient, Biogeochemistry, 67, 113–134, 2004.
Hsieh, Y. P. and Yang, C. H.: Pyrite accumulation and sulfate depletion as affected by root distribution in aJuncus (needle rush) salt marsh, Estuaries, 20, 640–645, 1997.
Janssens, J. A.: Noteworthy Mosses & Liverworts of Minnesota, Part I: Illustrated Field Keys, Minnesota Department of Natural Resources 2014, 176 pp., Minneapolis, MN, 2014.
Jerome, D., Westwood, M., Oldfield, S., and Romero-Severson, J.: Fraxinus nigra, The IUCN Red List of Threatened Species, e.T61918683A61918721, https://doi.org/10.2305/IUCN.UK.2017-2.RLTS.T61918683A61918721.en (downloaded on: 27 January 2020), 2017.
Jones, D. L. and Willett, V. B.: Experimental evaluation of methods to quantify dissolved organic nitrogen (DON) and dissolved organic carbon (DOC) in soil, Soil Biol. Biochem., 38, 991–999, 2006.
Jones, R. H., Lockaby, B. G., and Somers, G. L.: Effects of microtopography and disturbance on fine-root dynamics in wetland forests of low-order stream floodplains, Am. Midl. Nat., 136, 57–71, 1996.
Kirchner, J. W., Feng, X., and Neal, C.: Fractal stream chemistry and its implications for contaminant transport in catchments, Nature, 403, 524, https://doi.org/10.1038/35000537, 2000.
Kirchner, J. W., Tetzlaff, D., and Soulsby, C.: Comparing chloride and water isotopes as hydrological tracers in two Scottish catchments, Hydrol. Process., 24, 1631–1645, 2010.
Koponen, P., Nygren, P., Sabatier, D., Rousteau, A., and Saur, E.: Tree species diversity and forest structure in relation to microtopography in a tropical freshwater swamp forest in French Guiana, Plant Ecol., 173, 17–32, 2004.
Laine, J., Vasander, H., and Sallantaus, T.: Ecological effects of peatland drainage for forestry, Environ. Rev., 3, 286–303, 1995.
Li, X., Minick, K. J., Luff, J., Noormets, A., Miao, G., Mitra, B., Domec, J. C., Sun, G., McNulty, S., and King, J. S.: Effects of Microtopography on Absorptive and Transport Fine Root Biomass, Necromass, Production, Mortality and Decomposition in a Coastal Freshwater Forested Wetland, Southeastern USA, Ecosystems, 1–15, in press, 2020.
Looney, C. E., D'Amato, A. W., Fraver, S., Palik, B. J., and Reinikainen, M. R.: Examining the influences of tree-to-tree competition and climate on size-growth relationships in hydric, multi-aged Fraxinus nigra stands, Forest Ecol. Manag., 375, 238–248, 2016.
Looney, C. E., D'Amato, A. W., Fraver, S., Palik, B. J., and Frelich, L. E.: Interspecific competition limits the realized niche of Fraxinus nigra along a waterlogging gradient, Can. J. Forest Res., 48, 1292–1301, 2018.
Madureira, M. J., Vale, C., and Gonçalves, M. S.: Effect of plants on sulphur geochemistry in the Tagus salt-marshes sediments, Mar. Chem., 58, 27–37, 1997.
Mäkiranta, P., Riutta, T., Penttilä, T., and Minkkinen, K.: Dynamics of net ecosystem CO2 exchange and heterotrophic soil respiration following clearfelling in a drained peatland forest, Agr. Forest Meteorol., 150, 1585–1596, 2010.
Malhotra, A., Roulet, N. T., Wilson, P., Giroux-Bougard, X., and Harris, L. I.: Ecohydrological feedbacks in peatlands: an empirical test of the relationship among vegetation, microtopography and water table, Ecohydrology, 9, 1346–1357, 2016.
Malmer, N., Albinsson, C., Svensson, B. M., and Wallén, B.: Interferences between Sphagnum and vascular plants: effects on plant community structure and peat formation, Oikos, 100, 469–482, 2003.
McCune, B. and Grace, J. B.: Analysis of ecological communities, Vol. 28, MjM software design, Gleneden Beach, OR, 2002.
Megonigal, J. P., Conner, W. H., Kroeger, S., and Sharitz, R. R.: Aboveground production in southeastern floodplain forests: a test of the subsidy–stress hypothesis, Ecology, 78, 370–384, 1997.
Nielsen, D. L. and Chick, A. J.: Flood-mediated changes in aquatic macrophyte community structure, Mar. Freshwater Res., 48, 153–157, 1997.
Nielsen, D. L., Podnar, K., Watts, R. J., and Wilson, A. L.: Empirical evidence linking increased hydrologic stability with decreased biotic diversity within wetlands, Hydrobiologia, 708, 81–96, 2013.
Økland, R. H., Rydgren, K., and Økland, T.: Species richness in boreal swamp forests of SE Norway: The role of surface microtopography, J. Veg. Sci., 19, 67–74, 2008.
Oksanen, J. F., Blanchet, B., Friendly, M., Kindt, R., Legendre, P., McGlinn, D., Minchin, P. R., O'Hara, R. B., Simpson, G. L., Solymos, P., Stevens, M. H. H., Szoecs, E., and Wagner, H.: vegan: Community Ecology Package, R package version 2.5-3, available at: https://CRAN.R-project.org/package=vegan, last access: 1 May 2018.
Olde Venterink, H., Wassen, M. J., Verkroost, A. W. M., and De Ruiter, P. C.: Species richness–productivity patterns differ between N-, P-, and K-limited wetlands, Ecology, 84, 2191–2199, 2003.
Palik, B. J., Ostry, M. E., Venette, R. C., and Abdela, E.: Tree regeneration in black ash (Fraxinus nigra) stands exhibiting crown dieback in Minnesota, Forest Ecol. Manag., 269, 26–30, 2012.
Peach, M. and Zedler, J. B.: How tussocks structure sedge meadow vegetation, Wetlands, 26, 322–335, 2006.
Pouliot, R., Rochefort, L., Karofeld, E., and Mercier, C. Initiation of Sphagnum moss hummocks in bogs and the presence of vascular plants: Is there a link?, Acta Oecol., 37, 346–354, 2011.
R Core Team: R: A language and environment for statistical computing, R Foundation for Statistical Computing, Vienna, Austria, available at: https://www.R-project.org/, last access: 1 May 2018.
Reiners, W. A. and Reiners, N. M.: Energy and nutrient dynamics of forest floors in three Minnesota forests, J. Ecol., 58, 497–519, 1970.
Resler, L. M. and Stine, M. B.: Patterns and Processes of Tree Islands in Two Transitional Environments: Alpine Treeline and Bog Forest-Meadow Ecotones, Geography Compass, 3, 1305–1330, 2009.
Rietkerk, M., Dekker, S. C., Wassen, M. J., Verkroost, A. W. M., and Bierkens, M. F. P.: A putative mechanism for bog patterning, Am. Nat., 163, 699–708, 2004.
Rodríguez-Iturbe, I., D'Odorico, P., Laio, F., Ridolfi, L., and Tamea, S.: Challenges in humid land ecohydrology: Interactions of water table and unsaturated zone with climate, soil, and vegetation, Water Resour. Res., 43, W09301, https://doi.org/10.1029/2007WR006073, 2007.
Ross, M. S., Mitchell-Bruker, S., Sah, J. P., Stothoff, S., Ruiz, P. L., Reed, D. L., Jayachandran, K., and Coultas, C. L.: Interaction of hydrology and nutrient limitation in the Ridge and Slough landscape of the southern Everglades, Hydrobiologia, 569, 37–59, 2006.
Sebestyen, S. D., Dorrance, C., Olson, D. M., Verry, E. S., Kolka, R. K., Elling, A. E., and Kyllander, R.: Chapter 2. Long–term monitoring sites and trends at the Marcell Experimental Forest, in: Peatland biogeochemistry and watershed hydrology at the Marcell Experimental Forest, edited by: Kolka, R. K., Sebestyen, S. D., Verry, E. S., and Brooks, K. N., Boca Raton, FL, CRC Press, 15–71, 2011.
Soil Survey Staff: Natural Resources Conservation Service, United States Department of Agriculture, Web Soil Survey, available at: https://websoilsurvey.sc.egov.usda.gov/, last access: 11 February 2019.
Stovall, A. E., Diamond, J. S., Slesak, R. A., McLaughlin, D. L., and Shugart, H.: Quantifying wetland microtopography with terrestrial laser scanning, Remote Sens. Environ., 232, 111271, https://doi.org/10.1016/j.rse.2019.111271, 2019.
Strack, M., Waddington, J. M., Rochefort, L., and Tuittila, E. S.: Response of vegetation and net ecosystem carbon dioxide exchange at different peatland microforms following water table drawdown, J. Geophys. Res.-Biogeo., 111, G02006, https://doi.org/10.1029/2005JG000145, 2006.
Stribling, J. M., Glahn, O. A., Chen, X. M., and Cornwell, J. C.: Microtopographic variability in plant distribution and biogeochemistry in a brackish-marsh system, Mar. Ecol.-Prog. Ser., 320, 121–129, 2006.
Sullivan, P. F., Arens, S. J., Chimner, R. A., and Welker, J. M.: Temperature and microtopography interact to control carbon cycling in a high arctic fen, Ecosystems, 11, 61–76, 2008.
Takagi, K., Tsuboya, T., Takahashi, H., and Inoue, T.: Effect of the invasion of vascular plants on heat and water balance in the Sarobetsu mire, northern Japan, Wetlands, 19, 246–254, 1999.
Thorslund, J., Cohen, M. J., Jawitz, J. W., Destouni, G., Creed, I. F., Rains, M. C., Badiou, P., and Jarsojo, J.: Solute evidence for hydrological connectivity of geographically isolated wetlands, Land Degradation and Development, 29, 4061–4070, https://doi.org/10.1002/ldr.3175, 2018.
USDA Forest Service: Forests of the Northern Forest Inventory and Analysis Program, Web report, U.S. Department of Agriculture, Forest Service, Northern Research Station, Houghton, MI, 2016.
U.S. EPA: Methods for Evaluating Wetland Condition: Biogeochemical Indicators, Office of Water, U.S. Environmental Protection Agency, Washington, DC, EPA-822-R-08-022, p. 32, 2008.
van der Valk, A. G., Squires, L., and Welling, C. H.: Assessing the impacts of an increase in water level on wetland vegetation, Ecol. Appl., 4, 525–534, 1994.
van Ruijven, J. and Berendse, F.: Diversity–productivity relationships: initial effects, long-term patterns, and underlying mechanisms, P. Natl. Acad. Sci. USA, 102, 695–700, 2005.
Vivian-Smith, G.: Microtopographic Heterogeneity and Floristic Diversity in Experimental Wetland Communities, J. Ecol., 85, 71–82, 1997.
Wallis, E. and Raulings, E.: Relationship between water regime and hummock-building by Melaleuca ericifolia and Phragmites australis in a brackish wetland, Aquat. Bot., 95, 182–188, 2011.
Watts, D. L., Cohen, M. J., Heffernan, J. B., and Osborne, T. Z.: Hydrologic modification and the loss of self-organized patterning in the ridge–slough mosaic of the Everglades, Ecosystems, 13, 813–827, 2010.
Werner, K. J. and Zedler, J. B.: How sedge meadow soils, microtopography, and vegetation respond to sedimentation, Wetlands, 22, 451–466, 2002.
Wetzel, P. R., Van Der Valk, A. G., Newman, S., Gawlik, D. E., Troxler Gann, T., Coronado-Molina, C. A., Childers, D. L., and Sklar, F. H.: Maintaining tree islands in the Florida Everglades: nutrient redistribution is the key, Front. Ecol. Environ., 3, 370–376, 2005.
Windham, L.: Microscale spatial distribution of Phragmites australis (common reed) invasion into Spartina patens (salt hay)-dominated communities in brackish tidal marsh, Biol. Invasions, 1, 137–148, 1999.
WRCC (Western Regional Climate Center): Cooperative Climatological Data Summaries, available at: https://wrcc.dri.edu/cgi-bin/cliMAIN.pl?mn4652 (last access: 1 May 2018), 2019.