the Creative Commons Attribution 4.0 License.
the Creative Commons Attribution 4.0 License.
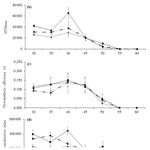
Tolerance of tropical marine microphytobenthos exposed to elevated irradiance and temperature
Andrew McMinn
The photosynthetic productivity of tropical microphytobenthos (MPB) is largely driven by changes in light intensities and temperature at the surface of sediment flats during emersion. Here, the response of the MPB community to temperature and light was examined. Changes in temperature and irradiance during tidal cycles in the Tanjung Rhu estuary, Langkawi, Malaysia, in 2007 significantly affected the photosynthetic capacities of the MPB. Higher photosynthetic parameters, such as the maximum relative electron transport rate (rETRmax), photosynthetic efficiency (α), maximum quantum yield (), and effective quantum yield (), were recorded at high tide when the temperatures were lower. However, when the community was experimentally exposed to irradiances of 1800 µmol photons m−2 s−1, it was only able to photosynthesize at temperatures < 50 ∘C. Above this temperature, no photosynthetic activity was observed. Not only did high temperatures at high irradiance affect the algal communities, but limited photosynthetic activity was also observed in samples when exposed to limited irradiance. Recovery rates were highest at the lowest temperatures and decreased as the temperature increased. The recovery rates for samples exposed to temperatures of 40 ∘C were s−1 and decreased to s−1 at 60 ∘C, indicating irreversible damage to Photosystem II (PSII). These characteristics suggest that the MPB communities in this estuary were able to adapt to temperature variation. However, enhanced photoinhibition would result if exposed to elevated temperatures, especially during low tide where in situ temperature was already 43 ∘C. Hence, if in situ temperature were to further increase during tidal emersion, 50 ∘C could be a temperature threshold for photosynthetic performance of tropical estuarine benthic microalgal communities.
- Article
(1170 KB) - Full-text XML
- BibTeX
- EndNote
Microphytobenthos (MPB) makes a significant contribution to the primary production of shallow coastal and estuarine environments, regularly contributing more than 50 % of the total annual production (Underwood, 2002; Rajesh et al., 2001). A few key environmental factors influence biomass production. For instance, high turbidity, which is a characteristic of many tropical estuaries, reduces the irradiance reaching the microalgae mats which then limits photosynthesis (Underwood, 2002). Intertidal ecosystems in the tropics are affected by large changes in solar irradiance and temperatures, caused by tidal cycles. During prolonged low tides, variation in irradiances, temperatures, and salinity can adversely affect the photosynthetic activity of MPB communities (Underwood, 2002; Laviale et al., 2016). To overcome these problems, many taxa have developed adjustment and avoidance strategies. Some can protect themselves physiologically against the damaging effects of exposure (Blanchard et al., 2004; Perkins et al., 2010) by dissipating the excess energy absorbed by light-harvesting centers, a process known as non-photochemical quenching (NPQ), while others can move vertically in the sediment, adjusting their position to minimize irradiance (Consalvey et al., 2004a; Perkins et al., 2010; Cartaxana et al., 2013). For instance, in a study by Mouget et al. (2008) it was shown that benthic diatoms with a high migratory capacity could experience high photosynthetic activity without any evidence of photodamage, while non-migratory taxa showed a significant depression in photosynthetic activity, which was caused by high irradiance and/or UV-B.
It is widely known that temperature plays an important role in the growth rate of all algal cells (Eppley, 1972; Davison, 1991, Eggert and Wiencke, 2000; Longhi et al., 2003). Prolonged exposure to temperatures significantly above ambient temperature (ideal growth temperature) also leads to a decrease in the concentration of chlorophyll a per cell (Defew et al., 2004). Excess light energy can also subsequently cause a decline in photosynthetic activity, which is reflected in the response of rapid-light-curve (RLC) parameters (Du et al., 2018). With combined stresses, such as high temperature and irradiance and/or UV-B), damage to Photosystem II (PSII) can be induced, impacting the recovery process; this is apparently more significant in planktonic diatoms by comparison with benthic species (Wu et al., 2017). Photoinhibited cells can mostly regain their capacity for photosynthesis when removed from a high-light environment (Wu et al., 2017). The ability of algae to tolerate dynamic intertidal environments such as light and temperature changes during tidal cycles and periods of photoinhibition largely determines estuarine community species composition (Derks and Bruce, 2018). If photoprotective mechanisms are inadequate for mitigating excess light, overexcitation of PSII can occur, leading to the production of reactive oxygen species (ROS), thus damaging the photosynthetic apparatus (Müller et al., 2001). The extent of photooxidative damage and the speed of recovery are related to the degradation rate of the D1 protein and the subsequent re-synthesis and replacement by new proteins (Derks and Bruce, 2018; Domingues et al., 2012). Similarly, benthic diatoms inhabiting sediment surfaces in estuaries are usually able to recover from extreme temperatures and light photoinhibition caused by tidal changes, since photoinhibition is only rarely recorded in these communities (Defew et al., 2004; Lee and McMinn, 2013; Perkins et al., 2006). Furthermore, many have the ability to migrate vertically, hence positioning themselves within the sediment at a depth that provides shading from excess irradiance and provides an optimal light environment for their photosynthetic activities (Cartaxana et al., 2011; Mouget et al., 2008; Perkins et al., 2010).
Due to the major contribution of MPB to ecosystem processes, they have been widely studied in tropical coral reefs and intertidal flats and coastal intertidal zones (Mitbavkar and Anil, 2002, 2004; McMinn et al., 2005; Underwood, 2002). However, there are relatively few studies of them in tropical estuaries (Patil and Anil, 2008). Although the photosynthesis of tropical benthic MPB communities is likely to be highly sensitive to elevated temperature, comparatively little attention has been given to the combined effects of both elevated light and temperature. Water temperatures in tropical marine ecosystems are already high, and relatively small increases can have severe negative impacts. Unfortunately, information on the combined effects of temperature and light on the photosynthetic responses from this region is lacking. To date, the temperature tolerance of tropical microalgae has been studied mostly in plankton, which cannot usually survive temperatures above ∼ 30 ∘C, which is consistent with the maximal temperature they normally experience (Indrayani et al., 2020; Thomas et al., 2012). MPB, however, may be exposed to much higher temperatures during low tide that can be several times higher than in the water column. Thus, the aim of this study is to determine the stress response of MPB physiology to the combined effects of elevated irradiance and temperature and understand its subsequent recovery. We hypothesize that high light could reduce the photoprotection capacity, thus causing severe damage and impairing the recovery process. To this end we exposed diatom-dominated communities to a range of temperatures and used pulse amplitude modulation (PAM) fluorometry to measure photosynthetic characteristics during exposure to different light levels.
2.1 Study area and field sampling
Tanjung Rhu is located on the north coast of Langkawi, Malaysia (Fig. 1). The area has a humid, tropical climate with daily maximum temperatures between 27.0 and 40.0 ∘C. This estuary contains a range of habitats (beaches, mangroves, and seagrass beds) all within close proximity. The estuary is mesotidal with semi-diurnal tides and a tidal range of between 2 and 3 m. Much of the ecosystem is exposed during low tide and submerged during high tide. The estuary is surrounded by a mangrove forest, mainly dominated by Rhizophora and Avicennia species. The benthic habitat is mostly composed of coarse sand with patches of mud. The water normally carries a high suspended load, originating partly from the mangrove forest and from the river sediment itself.
Samples were collected in April 2007 from three sites (Site A, B, and C) all in proximity (20 m) to the intertidal zone of the Tanjung Rhu estuary, at ebb and flood tide. Descriptions of sites A, B, and C are provided in Table 1. Water height at ebb tide was approximately 0 to 0.2 m, and flood tide it was approximately 0.5 to 1 m. On each occasion, seven 15 mm diameter hand-pushed sediment cores were taken: three for photosynthetic parameter analysis, three for chlorophyll a analysis, and one for species composition. For the temperature incubation experiments, the top 10 mm (approximately) of the sediment was manually scraped off and placed in a dark plastic bag (20×20 cm). Samples were stored in the dark and promptly returned (approximately 15 min) to the laboratory for the experiments.
At each sampling site, environmental measurements (temperature, salinity, and photosynthetically active irradiance (PAR)) were measured using a Hydrolab DataSonde 4a (Hach, Loveland, CO, USA). Water samples for all parameters and nutrient analyses were collected during high tide (water level 1.0 m) and low tide (water level 0.2 m). Nitrate, phosphate, and ammonium were analyzed on a Hach kit DR 2000 spectrophotometer (Hach, Loveland, CO, USA). Ammonia, expressed as ammonia nitrogen (NH3-N), was determined using the Nessler method (Hach Company, 1995). Phosphate, expressed as phosphorus phosphate (PO-P) was determined by the PhosVer (ascorbic acid) method (Hach Company, 1995). Nitrate, expressed as nitrate nitrogen (NO-N), was determined by the cadmium reduction method (Hach Company, 1995).
2.2 Chlorophyll a biomass
Chlorophyll a was measured as a proxy for algal biomass. The chlorophyll biomass was determined following Jordan et al. (2010). For chlorophyll a biomass and fluorescence analysis, 45 mm diameter clear polycarbonate cores were manually pushed into the sediment and stoppered using a rubber bung and immediately returned to a temporarily established working place. Three cores were taken for three biomass analyses and three fluorescence analyses (see “PAM chlorophyll fluorescence measurements”). The sediment for chlorophyll a analysis, for both low-tide and high-tide samples, was placed in an ice-filled, light-proof container and immediately transferred to the laboratory. The top 10 mm of sediment was re-suspended in 10 mL of methanol, thoroughly mixed, and then stored in the dark for 12 h at 4 ∘C. After the sediment had settled, the solvent was decanted off to measure the chlorophyll a content using acidification methods (Holm-Hansen and Lorenzen, 1965). A Turner Designs 10AU fluorometer (Sunnyvale, CA, USA) was used to measure the chlorophyll a. The fluorometer was calibrated against a chlorophyll a standard (Sigma-Aldrich, St Louis, MO, USA).
2.3 PAM chlorophyll fluorescence measurements
Variable fluorescence was measured using a pulse amplitude modulation (PAM) (Schreiber, 2004) fluorometer comprising a computer-operated PAM control unit (Walz, Effeltrich, Germany) and a WATER-EDF universal emitter–detector unit (Gademann Instruments GmbH, Würzburg, Germany). The top 10 mm of each core was sectioned on site, with 5 mm for PAM fluorometry analysis. Each sample was mixed with filtered seawater and transferred into a vial wrapped in aluminum foil to protect it from light and to dark-adapt it for 15 min. The samples were shaken vigorously and then allowed to settle for approximately 10 s before analysis. The PAM methodology followed McMinn et al. (2005) and Salleh and McMinn (2011).
To calculate the maximum PSII quantum yield (), the samples were dark-adapted in the field for 15 min by wrapping the jars in foil and placing them in a dark container immediately after sampling. Rapid light curves (RLCs) were taken under software control (WinControl, Walz) to obtain values for , NPQ, and Ek (Ralph and Gademann, 2005). Red light-emitting diodes (LEDs) provided the actinic light used in the RLCs at levels of 0, 85, 125, 194, 289, 413, 517, 1046, and 1554 µmol photons m−2 s−1. The saturation pulse irradiance was 3000 µmol photons m−2 s−1 for a period of 0.8 s. Samples were exposed to each light level for 10 s. Photomultiplier gain settings were between 3 and 8 (WinControl, Walz). Ek, the light saturation parameter, was calculated from the intercept between the maximum relative electron transport rate (rETR) and α, the photosynthetic efficiency (Falkowski and Raven, 2007). The rETR was calculated by multiplying the irradiance by the quantum yield measured at the end of that interval. PAR-versus-rETR curves were described using the model of Jassby and Platt (1976) using multiple non-linear-regression curve-fitting protocols on SPSS software (SPSS Inc., Chicago, IL, USA).
NPQ is a measurement of the activity of the protective mechanism, which is designed to protect against over-reduction of the photosynthetic electron transport chain by dissipation of excess absorbed light energy in the PSII antenna system as heat (Ruban et al., 2004). NPQ is a complex response comprising at least four different, time-dependent responses (Fanesi et al., 2016). It is recognized that it is not possible to distinguish which process is occurring using this approach. NPQ was determined by the following equation (Schreiber, 2004).
Non-photosynthetic quenching (NPQ) values presented here were taken from the RLC data generated by WinControl software (Walz GmbH, Effeltrich, Germany). The short light exposure time in this experiment (10 s RLC duration) would have been insufficient for maximum NPQ development and so provides only a relative measure of the ability of the treatments to develop NPQ (Ralph and Gademann, 2005).
2.4 Temperature and irradiance experiments
For the temperature and irradiance incubation experiments, samples were collected during low tide and exposed to a combination treatment of irradiances (1800, 890, and 0 µmol photons m−2 s−1) and high temperatures (30, 35, 40, 45, 50, 55, and 60 ∘C). Samples were always taken from Site C, where the highest temperatures were recorded during in situ data collection (Table 1). The top 0.5 to 10 mm of the sediment was collected by hand using a clear polycarbonate core during low tide and wrapped in a dark plastic bag prior to analysis. Samples were kept upright in the tubes and were immediately returned to the lab for the experiments. The sediment samples were suspended in a beaker, and excess seawater was decanted. Filtered seawater collected from the same site was added to each vial, and the vials were placed in three chambers of different irradiances in a temperature-controlled water bath (Lauda 20 L, Lauda-Brinkmann, Königshoven, Germany). Triplicate sample vials were placed in each irradiance chamber. Fresh algal samples were used for each temperature change. Since irradiance was provided by a halogen lamp (Thorn 300 W, Borehamwood, UK), it is understood that the spectrum will have differed from that of natural sunlight. The irradiance in each chamber was measured with a Quantum light meter LI-189 (LI-COR, NE, USA). The irradiance of each chamber was adjusted by the addition of several layers of dark plastic filters. These filters also assisted in reducing the heat produced by the halogen lamps. Care was taken to ensure that the temperature remained constant throughout the incubation. Fresh samples were used for each temperature incubation. The algal samples were exposed to three different irradiance treatments – A, 1800 µmol photons m−2 s−1; B, 890 µmol photons m−2 s−1; and C, 0 µmol photons m−2 s−1 – with three replicates for each treatment. Samples were placed in the water bath for a period of 1 h for each temperature treatment. The temperatures selected were 30, 35, 40, 45, 50, 55, and 60 ∘C. Lower temperatures (30 to 35 ∘C) were used as controls since the community had been growing within that temperature range in the natural habitat. As experiments were conducted in a makeshift laboratory, temperatures < 30 ∘C could not be obtained due to equipment limitations. At the end of each incubation, each replicate was immediately transferred into a cuvette for photosynthetic analysis. A WATER-PAM fluorometer was used to determine the quantum yield at the end of each temperature treatment. RLCs (see above) were run to obtain values for , rETRmax, Ek, and α. In each treatment, three replicates (n=3) were measured. Before measurement, samples were gently shaken to ensure even mixing and to limit settlement and were then placed inside the measuring cuvette of the Water PAM fluorometer. Measurements were conducted as soon as possible to ensure thermal change was minimized during the measurements of the RLCs.
2.5 Rate of recovery
The method of determining and analyzing the rate of recovery was adapted from McMinn and Hattori (2006) and Salleh and McMinn (2011). The recovery measurements were made after the 1 h light incubation. The recovery rates suggest the ability of cells to reactivate photosynthetic activity after 1 h of exposure to high light incubation. The measurement of recovery from photoinhibition was made using the pre-installed routine RLC + Recovery of the WATER-PAM. After the last actinic light period of the RLC, the sample was left in the dark, while was determined after 30, 60, 180, 300, and 600 s. The rate of recovery from photoinhibition was calculated following Oliver et al. (2003):
where Φt is the given value of at time t (s), Φ I is the initial value of before recovery measurements have commenced, ΦM is the fully recovered value, and r is an exponential rate constant for the recovery of from photoinhibition (s−1). This formula assumes that the recovery rate of is dependent on the degree of damage.
2.6 Statistical analysis
The mean and standard deviations were calculated from three independent replicates of each parameter. To evaluate the effects of temperature and irradiances (fixed factor) on the photosynthetic parameters (, rETRmax, Ek, α, NPQ, and recovery rate), a two-way analysis of variance (ANOVA) and regression were used with subsequent Tukey post hoc comparisons. Before analyses, the data were checked for normality and homogeneity of variances with the Shapiro–Wilk and Levene tests, respectively. Data were transformed whenever necessary to comply with ANOVA assumptions. Differences were accepted as significant at P<0.05 unless otherwise stated. All statistical analyses were performed using SPSS 18.0.
The diatom genera Cocconeis, Navicula, and Rhopalodia (observed by the authors) dominated the MPB communities, which were predominantly located on coarse sand with mud and/or silt patches. The sediment characteristics were similar among the sampling sites. There were significant differences in environmental parameters between high and low tide (Tables 2 and 4a); however, environmental characteristics between sites were similar except for bottom irradiance. Tidal exposure caused significant differences in the irradiance reaching the bottom and elevated the temperature at low tide for all sites. During high tide, bottom irradiances were at their lowest (173.42±13.73 µmol photons m−2 s−1) at the shaded Site B and were highest in the area that was most exposed, Site C (1964.33±99.68 µmol photons m−2 s−1), when the algal mats were almost fully exposed. There was a more-than-6-fold increase in the irradiance from low tide to high tide (Tables 2 and 4a). Water temperature rose from 28 ∘C during high tide to 43 ∘C during low tide. The highest nutrient levels were recorded during high tide, when seawater re-entered the estuary (Tables 2 and 4a). There was at least a 50 % increase in nitrate and phosphate levels during high tide, although the changes were not significant (Table 2).
Table 3In situ photosynthetic parameters of microphytobenthos at Tanjung Rhu estuary (Site A, B, and C) in the surface 10 mm (depth ∼ 0.2 m at low tide and 1.0 m at high tide). Data are means ± SD; n=3.
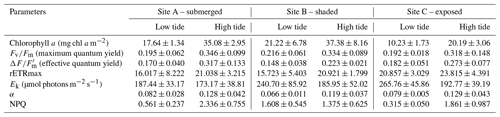
3.1 In situ chlorophyll a and photosynthetic parameters of microphytobenthos
Due to the high turbidity in the Tanjung Rhu estuary, MPB on the sediment surface was generally exposed to significantly lower irradiances at high tide than during low tide. Differences in sediment surface PAR caused a significant decline in chlorophyll a biomass and PSII activity, as measured in the RLC photophysiological parameters. The highest biomass was observed at Site B (shaded area) where PAR was at the lowest during the sampling time. At low tide the chlorophyll a values were between an average of 17.6 to 21.22 mg chl a m−2, but values increased by almost 50 % at high tide (20.19 to 37.38 mg chl a m−2). The photosynthetic parameters for both low and high tide are presented in Table 3. Quantum yield, rETRmax, and Ek were relatively low during both high and low tide. However, the effects of the tidal cycle could be clearly seen, as most values increased as the tide rose, except for Ek. The Ek values declined by 23 % during high tide. The NPQ values were higher at low tide than at high tide. In summary, differences in all parameters were significant between tides by comparison with sites (Table 4b).
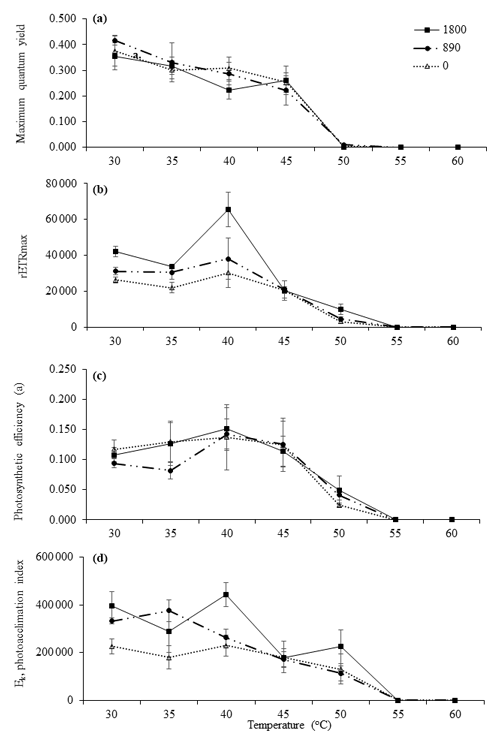
Figure 2Derived photosynthetic parameters of RLCs. Parameters were plotted against experimental temperatures (30, 35, 40, 45, 50, 55, and 60 ∘C) at irradiances of 1800 µmol photons m−2 s−1 (closed squares), 890 µmol photons m−2 s−1 (closed circles), and 0 µmol photons m−2 s−1 (open triangles). (a) Maximum quantum yield, (b) photosynthetic efficiency (α), (c) rETRmax, and (d) photoacclimation index (Ek). Values are means ± SD (n=3).
Table 4Two-way ANOVA for (a) physical parameters (bottom irradiance (PAR), temperature, nitrate, phosphate, ammonium, and chl a and (b) photosynthetic parameters (effective quantum yield, ; maximum quantum yield, ; relative maximum photosynthetic rate, rETRmax; light saturation parameter, Ek; photosynthetic efficiency, α; and non-photochemical quenching, NPQ) with respect to tide (low and high) and site (A, B, and C).
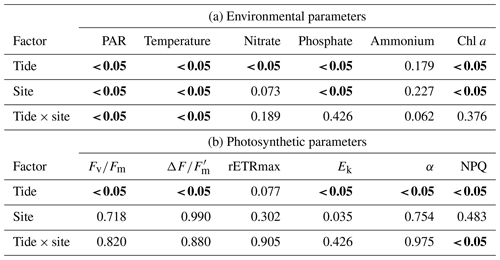
Significant difference (P<0.05) indicated in bold.
3.2 Effects of temperatures and irradiances
As the MPB community composition and photophysiological parameters were not significant between sites A, B, and C, the diatoms collected from Site C (exposed) were used for the incubation experiments. These communities were exposed to the highest irradiances and temperatures occurring naturally during the day. In general, the communities maintained at 30, 35, 40, 45, and 50 ∘C were still able to photosynthesize at all light levels. Statistical analysis indicated that the communities were mostly impacted by elevated temperature rather than by irradiance. Control samples (30 and 35 ∘C) incubated at all irradiance levels had higher by comparison with other temperatures. There were no significant differences between either of these control temperatures (P<0.05, Table 5). The very low values indicate that severe photoinhibitive stress was present when the samples were exposed to high temperatures (55 and 60 ∘C) (Fig. 2a). The rETRmax, α, and Ek values also decreased rapidly as temperatures increased to 50 ∘C (Fig. 2a–d), and no photosynthetic activity was observed at 55 or 60 ∘C at any irradiance level (P<0.05, Table 5). Significant differences (ANOVA, P<0.05) between temperature treatments were observed in all photosynthetic parameters. Although only limited photosynthetic activity was observed at higher temperatures, higher NPQ values were observed at these temperatures (Fig. 3); however, NPQ values were relatively low in all the treatments, especially at higher irradiances.
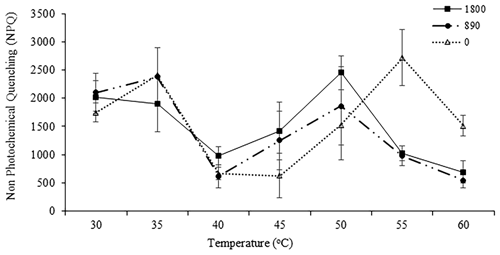
Figure 3The NPQ of samples at each irradiance level (1800 µmol photons m−2 s−1 (closed squares), 890 µmol photons m−2 s−1 (closed circles), and 0 µmol photons m−2 s−1 (closed triangles)) and temperature (30, 35, 40, 45, 50, 55, and 60 ∘C). NPQ was obtained at the end of the RLCs with actinic irradiance of 1554 µmol photons m−2 s−1. Values are means ± SD (n=3).
Table 5Two-way ANOVA of effects of temperature (0 to 60 ∘C) and irradiance level (1800, 890, and 0 µmol photons m−2 s−1) on the photosynthetic parameters (, maximum quantum yield; α, photosynthetic efficiency; rETRmax, relative electron transport rate; Ek, photoacclimation index), NPQ (non-photochemical quenching), and recovery rate of the microphytobenthos after exposure to temperature treatment for 1 h.
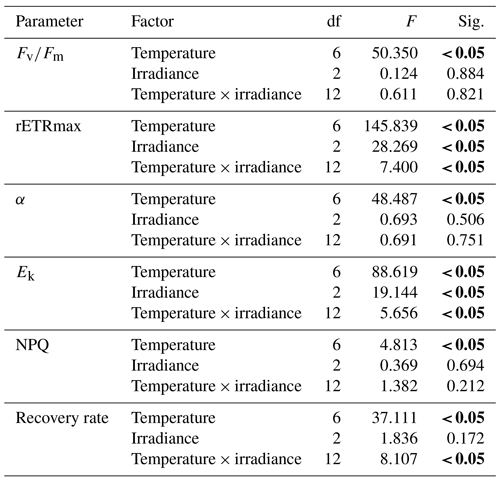
Significant difference (P<0.05) indicated in bold.
3.3 Recovery from temperature treatments
Elevated temperatures affected the recovery rates in the high-temperature and high-irradiance treatments, (P<0.05, Table 5). The highest recovery rate occurred at 40 ∘C. This corresponds with the maximum in situ temperature at Site C, where the samples were collected from, of 42.5 ∘C. The effects of the high temperatures were clearly visible at temperatures of 50, 55, and 60 ∘C, where severe depression of the recovery rate was observed (Fig. 4).
In the present study, we found that the MPB communities inhabiting the Tanjung Rhu estuary were not inhibited by the high- and variable-light environment (range 170–1900 µmol photons m−2 s−1) or temperatures (28.5 to 43 ∘C) during tidal exposure. The surface water temperature increased from 28.5 to 43 ∘C during high to low tide. The emersion at low tide caused the diatoms to migrate into the sediment to avoid excess light as a photoprotective mechanism. During the tidal cycle, the MPB communities were able to acclimate to these changes by optimizing photosynthesis while minimizing photodamage caused by temperature and light fluctuations through physiological changes (e.g., diversion of excess energy away from photosystem reaction centers) (Consalvey et al., 2005; Coelho et al., 2011; Serôdio et al., 2005).
Maximum quantum yield () values are often used as a sensitive indicator of photosynthetic stress (Du et al., 2018; McMinn et al., 2005). In a review by Campbell and Tyystjärvi (2012) it was suggested that for photoinhibition measurements dark-adapted values can be used if measuring the rate of oxygen evolution is not possible. Thus, in this study, dark-adapted values were used as a proxy for diatom health/stress (Consalvey et al., 2005; Du et al., 2018) upon exposure to light and temperature stress. An increase in temperature and irradiance during low tide caused a decline in at all sites in the Tanjung Rhu estuary; these were low compared with optimum values of ∼ 0.650 for healthy microalgae (McMinn and Hegseth, 2004). Similarly, low values were found by McMinn et al. (2005) on the tropical shore of Muka Head and Pulau Songsong, Malaysia, where the average maximum quantum yields, which were only 0.325, were probably caused by either high irradiances or nutrient stress. Falkowski and LaRoche (1991) suggested that nutrient limitation would decrease the optimal quantum efficiency of phytoplankton. However, mangrove ecosystems are highly dynamic and support relatively high microalgae biomass, and nutrient availability should not be a limiting factor (Hilaluddin et al., 2020; Rahaman et al., 2013). Nutrient concentrations (phosphate and nitrate) measured in this study were within the average range for other tropical mangrove estuaries (Rajesh et al., 2001) and are unlikely to be limiting. Thus, it is likely that the high temperatures, of up to 42.5 ∘C during low tide in this study, were contributing to the low values.
Loik and Harte (1996) suggested that PSII reaction centers are susceptible to high temperatures and that the thermal stability of PSII can be influenced by the interaction between irradiance and high temperature since they will usually occur simultaneously in the field. An increase in values may be attributed to a prolonged low-light period during emersion at high tide. Prolonged exposure to high irradiances during low tide also affects values and reduces the algae's ability to fully recover during high tide. Du et al. (2018) also noted that NPQ and in benthic diatoms were not impacted by low light of < 250 µmol photons m−2 s−1 by comparison with high light of > 500 µmol photons m−2 s−1. Furthermore, photoinhibition has rarely been recorded in intertidal diatoms (Perkins et al., 2006). This is almost certainly due to their ability to migrate into the sediment and away from damaging light (Consalvey et al., 2004b; Mouget et al., 2008). Their ability to subsequently recover during high tide suggests that down-regulation of photosynthesis and up-regulation of photoprotection occurs, which prevents serious damage to the photosystems during the high irradiances at low tide. Long and Humphries (1994) suggested that photoinhibition occurs during low tide, where declines, but recovers at high tide. However, this response was not identified by Serôdio et al. (2008), who suggested that this short-term photoinhibition happened due to the incomplete recovery of photosynthetic activity at low light and under nutrient depletion.
The reduction in in situ values at Tanjung Rhu were probably due to the NPQ (non-photochemical quenching) mechanism rather than photoinhibition that characterizes the estuarine intertidal environment (Serôdio et al., 2008). During low tide, the MPB community reacted rapidly to the sudden increase in irradiance and temperature, protecting their photosystem reaction centers by dissipating excess energy in the antenna complex of Photosystem I (PSI) and PSII (Goss and Lepetit, 2015). NPQ values during low tides were higher than during high tides, suggesting that under high irradiance, the community compensated for the possible overexcitation of the photosynthetic machinery through the dissipation of excess absorbed light through xanthophyll-pigment-dependent energy dissipation. These communities were also able to modify their photophysiology by maximizing their light-harvesting efficiency and hence had higher values of α at high tide than at low tide. Ek values were at their highest during low tide at Site C (exposed) (265.76±45.86 µmol photons m−2 s−1), which indicates that they were able to adapt to the changing light climate. Hence, at low tide the community modified its light-harvesting capacity to utilize the high irradiances to which they were exposed. Similarly, Perkins et al. (2006) found that benthic diatoms grown in high light had higher rETRmax and Ek values and lower α values. However, it should be noted that the length of light exposure during RLCs affects these values, as short-term light exposure, e.g., 10 s in this study, is insufficient for complete equilibration to each light level by comparison with P-versus-E curves, based on oxygen measurements (Torres et al., 2014).
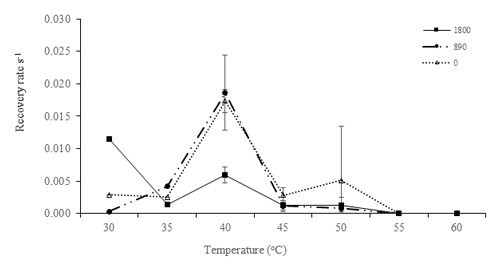
Figure 4Recovery rate (s−1) of after the RLC for low-irradiance experiments. Recovery rates were plotted against experimental temperatures at irradiances of 1800 µmol photons m−2 s−1 (closed squares), 890 µmol photons m−2 s−1 (closed circles), and 0 µmol photons m−2 s−1 (open triangles) with temperatures of 30, 35, 40, 45, 50, 55, and 60 ∘C. In this recovery analysis, samples of 30 and 35 ∘C were analyzed without replicates; thus, no error bar was obtained.
4.1 Effects of irradiance and temperature incubations
Chlorophyll a fluorescence is an ideal tool for measuring the response of photosynthesis to changes in temperature, since PSII is the most thermo-labile component of photosynthesis, and the majority of chlorophyll a fluorescence originates from PSII under normal physiological conditions (Falkowski and Raven, 2007). Photosynthesis is extremely sensitive to stress caused by intense temperatures and irradiance, and it is often inhibited before other cellular functions are harmed. When the diatom-dominated MPB samples were exposed to higher temperatures and irradiance, symptoms of irreversible thermal damage were clearly visible at 55 and 60 ∘C. Limited photosynthetic activity at these temperatures suggests that the cells would suffer damaging effects from periodic increases in seawater temperatures greater than 50 ∘C, especially during low tide when the irradiance levels are also high. This suggests that high temperatures cause structural closure of the PSII reaction centers and chloroplast dysfunction. Furthermore, at high temperatures and irradiances, light-dependent RuBisCO activation is inhibited, and this is closely correlated with a reversible reduction in CO2 fixation. This is consistent with the decrease in the effective quantum yield, which displays a strong, quantitative relationship with the quantum yield of CO2 assimilation (Oxborough and Baker, 1997).
Light and temperature exposure is expected to influence the sensitivity of response to changing light climate (Davison, 1991) during tidal cycles and hence affect values of photosynthetic efficiency (α). However, α can also be impacted by temperature changes, which can have a strong influence on the photosynthetic capacity (Claquin et al., 2008). The α values observed here showed a significant decline when exposed to high irradiances (up to 1800 µmol photons m−2 s−1) and temperatures (up to 60 ∘C). The reduction in α as temperature increased is consistent with the results reviewed by Davison (1991), where α also declined as temperature increased. The effects of temperatures on α values were only significant when the cells were exposed to both adverse irradiances and adverse temperatures. Defew et al. (2004) showed that in temperate microalgae, there were no clear trends in α values, with either temperature or light, when samples were exposed to temperatures ranging from 10 to 26 ∘C and irradiance of up to 350 µmol photons m−2 s−1. This variation between studies in the α response to temperature is probably a result of thermal acclimation being species dependent (Claquin et al., 2008) and also of major impacts only occurring at the extremes. Fanesi et al. (2016) also discussed how temperature affected absorbed light in different freshwater phytoplankton groups but did not examine the effects at temperature extremes. Furthermore, some of the variation could also have been caused by the fluorescence method itself and the duration of RLC exposure. For example, Lefebvre et al. (2011) noted that α was systematically lower due to the slower relaxation of NPQ in 10 s RLCs.
The Ek index provides an indication of the irradiance at which energy is diverted from photochemistry to heat dissipation. It represents the degree of acclimation to the ambient light climate (Schreiber, 2004). Ek is used as an indicator of the photoacclimation status of photosynthetic organisms. Higher values of Ek suggest acclimation to higher irradiances (McMinn et al., 2005). MPB exposed to high irradiance herein (1800 µmol photons m−2 s−1) was not able to adjust its metabolism to maximize its response to these irradiances. Similarly, in the natural habitat of MPB, during low tide the in situ data showed that the samples were light saturated at an in situ irradiance of 1900 µmol photons m−2 s−1. However, the Ek values recorded at all experimental irradiances and temperatures (40 to 50 ∘C) were similar to the in situ Ek values. When higher temperatures (above 50 ∘C) were imposed, significant declines in Ek were observed. Falkowski and Raven (2013) suggested that as temperatures and irradiances increase, Ek also increases. This suggests that these temperatures (above 50 ∘C) were approaching a critical temperature threshold and affecting the cells' capability to photosynthesize. These effects were also observed in the rETRmax values.
The rETRmax parameter has been used in many recent MPB studies (e.g., Cartaxana et al., 2011; Du et al., 2018) as it is closely related to the maximum photosynthetic capacity, which is obtained when the rate of photosynthesis is limited by the activity of the electron transport chain or Calvin cycle enzymes (Ralph and Gademann, 2005). The rETRmax values recovered here were typical of diatom responses to high irradiances, where, as irradiance increases, photosynthetic rates become increasingly non-linear and rise to a saturation level (Blanchard et al., 2004). Perkins et al. (2006) suggested that rETRmax determined after 10 s of actinic light during RLCs are lower when cells have been previously acclimated to higher irradiance. However, to minimize the potential for the RLC irradiance to cause photoacclimation, the RLC duration should be as short as possible (Ralph and Gademann, 2005). Unlike in situ studies, where natural biochemical and physical gradients are preserved, experiments such as those described here, where cells are suspended, will give a slightly different result. Higher Ek and rETRmax values are typical of high-irradiance-acclimated samples, where the cells have modified their light-harvesting pigment to utilize the irradiance to which they were exposed. Benthic microalgae acclimated to low irradiance are able to modify their physiology and maximize their light-harvesting efficiency, thus providing higher values of α. These characteristics were observed in both the low and the high irradiance only at ambient temperatures (up to 45 ∘C). The photosynthetic rate declined when samples were exposed to elevated temperatures. Severe effects were seen in higher-irradiance experiments. Here, as the temperatures increased above 55 ∘C, these algae appeared to be affected by thermal stress and were not able to acclimate to the high temperatures.
Although exposed to variations in irradiance and temperature during tidal cycles, MPB has a range of mechanisms to facilitate and optimize light interception and utilization. Here, cells exposed to high irradiances and temperatures of 40 ∘C did not show any sign of photoinhibition as they were naturally growing at temperatures of up to 43 ∘C during low-tide exposure. The cells were able to dissipate the excess light via NPQ or possibly migration. Although migration was not investigated in this study, behavioral photoprotection by vertical migration is a common response to avoid high light by pennate diatoms (Cartaxana et al., 2011; Du et al., 2018; Mitbavkar and Anil, 2002). In contrast, samples exposed to the same irradiance level but higher temperatures (55 and 60 ∘C) were not able to protect themselves from photodamage and photoinhibition. Exposure to high irradiances coupled with high temperatures caused an excess of absorbed light energy that was unable to be used in the photosynthetic process, resulting in the increases in NPQ values observed in this study. Although no photosynthetic activity was present at the highest temperatures (55 and 60 ∘C), heat dissipation was still active. Xanthophyll cycling is especially significant when short-term changes in the light climate occur (seconds to minutes), such as those happening in the Tanjung Rhu intertidal sediments. Samples incubated in the dark and at high temperatures (55 and 60 ∘C) were seen to have higher NPQ levels. In addition, these samples showed sudden increments in NPQ from 50 to 55 ∘C. However, as no photosynthetic activity was observed at 55 ∘C, it is possible that the NPQ signal was generated from dead diatom cells. Nonetheless, the ability of dark-adapted diatoms to develop NPQ has been considered an adaptive advantage, providing a method to prevent degradation of light-harvesting pigments (Serôdio et al., 2006b; Lavaud and Lepetit, 2013). Hence further investigation is required to better understand the impact of extreme temperatures on the mechanism of NPQ, formation of reactive oxygen species, photodamage, and ultimately inactivation of PSII.
The ability of protists to recover from stress is a major characteristic determining their ability to survive under hostile conditions (Wu et al., 2017; Ralph and Gademann, 2005). In this study, at higher temperatures, there are indications that degradation of D1 proteins may have occurred and that this disrupted the recovery of PSII, thus preventing cells from adapting to the ambient conditions. At 40 ∘C, dark-acclimated samples were able to recover faster than at higher temperatures, suggesting that high irradiances (1800 and 890 µmol photons m−2 s−1) increase PSII damage and contribute to the slower recoveries. In addition, these samples had been exposed to similar temperatures to those of in situ samples during low tide, thus suggesting that combined high light and temperature could have a more significant impact than temperature alone. These experiments showed that samples that were exposed to lower temperatures had a better recovery rate than those exposed to higher temperatures (> 55 ∘C). The lack of recovery at temperatures higher than 55 ∘C suggests that the photosynthetic enzymes had been inactivated or damaged, and acclimation to these temperatures was not possible. Inhibition of photosynthesis is reversible when temperatures are only a little higher than optimal (moderate heat stress), whereas damage to the photosynthetic apparatus is permanent under severe heat stress (Berry and Bjorkman, 1980). At low tide in tropical estuaries, water temperatures already regularly exceed 50 ∘C, temperatures usually considered outside the range of survival of marine phototrophs. The 50 ∘C environmental water temperature seems to be a tipping point above which photosynthesis is irreparably impaired. In these circumstances, it is likely that tropical estuarine MPB community composition will change and benthic primary production will decrease. This will likely have a cascading effect throughout these ecosystems.
This study provides an insight into the capacity of tropical diatom-dominated MPB communities to tolerate environmental stresses and the degree to which those stresses could damage the photosynthetic apparatus. Thus, parameters derived from RLCs were useful for indicating the photosynthetic capacities over a series of temperature and light levels, even though they are derived from measurements over a range of irradiances. Likewise, since most previous studies of the combined impacts of high temperature on benthic communities have focussed on subtropical and temperate communities; responses of tropical communities are unknown and are likely to vary significantly. Short-term changes (hours) in temperatures of up to 45 ∘C, such as those experienced by the community in Tanjung Rhu estuary, will not severely affect their photosynthetic apparatus, but higher temperatures (55 and 60 ∘C) will cause severe damage and irreparable photoinhibition. The responses of MPB to light are also dependent on the temperature regime to which they are exposed. The MPB in Tanjung Rhu experiences these effects during tidal emersion and during the night period. In summary, optimal temperatures for MPB photosynthetic performance is up to 45 ∘C; beyond this temperature the PSII function will be severely damaged and could lead to chronic photoinhibition.
No data sets were used in this article
SS contributed in terms of conceptualization, validation, formal analysis, investigation, data curation, and writing the original draft. AM contributed in terms of validation, resources, review and editing of the writing, visualization, and supervision.
The authors declare that they have no conflict of interest.
Publisher’s note: Copernicus Publications remains neutral with regard to jurisdictional claims in published maps and institutional affiliations.
We thank Mahadi Mohammad for his assistance with the fieldwork. We also thank the three reviewers for their critical comments which helped improve the current manuscript.
This research and article processing charges for this publication were supported by the University of Tasmania and Universiti Sains Malaysia (1001/CDASAR/8011046).
This paper was edited by Aninda Mazumdar and reviewed by Siqueiros-Beltrones, David A, and one anonymous referee.
Berry, J. and Bjorkman, O.: Photosynthetic response and adaptation to temperature in higher plants, Annu. Rev. Plant Physiol., 31, 491–543, https://doi.org/10.1146/annurev.pp.31.060180.002423, 1980.
Blanchard, G., Guarini, J., and Dang, C.: Characterizing and quantifying photoinhibition in intertidal microphytobenthos, J. Phycol., 40, 692–696, https://doi.org/10.1111/j.1529-8817.2004.03063.x, 2004.
Campbell, D. A. and Tyystjärvi, E.: Parameterization of photosystem II photoinactivation and repair, Biochim. Biophys. Acta, 1817, 258–265, https://doi.org/10.1016/j.bbabio.2011.04.010, 2012.
Cartaxana, P., Ruivo, M., Hubas, C., and Davidson, I.: Physiological versus behavioral photoprotection in intertidal epipelic and epipsammic benthic diatom communities, J. Exp. Mar. Bio. Ecol., 405, 120–127, https://doi.org/10.1016/j.jembe.2011.05.027, 2011.
Cartaxana, P., Domingues, N., Cruz, S., and Jesus, B.: Photoinhibition in benthic diatom assemblages under light stress, Aquat. Microb., 70, 87–92, https://doi.org/10.3354/ame01648, 2013.
Claquin, P., Probert, I., Lefebvre, S., and Veron, B.: Effects of temperature on photosynthetic parameters and TEP production in eight species of marine microalgae, Aquat. Microb. Ecol., 51, 1–11, https://doi.org/10.3354/AME01187, 2008.
Coelho, H., Vieira, S., and Serôdio, J.: Endogenous versus environmental control of vertical migration by intertidal benthic microalgae, Eur. J. Phycol., 46, 271–281, https://doi.org/10.1080/09670262.2011.598242, 2011.
Consalvey, M., Paterson, D. M., and Underwood, G. J. C.: The ups and downs of life in a benthic biofilm: Migration of benthic diatoms, Diatom. Res., 19, 181–202, https://doi.org/10.1080/0269249X.2004.9705870, 2004a.
Consalvey, M., Jesus, B., Perkins, R., and Brotas, V.: Monitoring migration and measuring biomass in benthic biofilms: the effects of dark/far-red adaptation and vertical migration on fluorescence measurements, Photosynthesis, 81, 91–101, https://doi.org/10.1023/B:PRES.0000028397.86495.b5, 2004b.
Consalvey, M., Perkins, R., and Paterson, D.: PAM fluorescence: A beginners guide for benthic diatomists, Diatom Res., 20, 1–22, https://doi.org/10.1080/0269249X.2005.9705619, 2005.
Davison, I.: Environmental effects on algal photosynthesis: Temperature, J. Phycol., 27, 2–8, https://doi.org/10.1111/j.0022-3646.1991.00002.x, 1991.
Defew, E., Perkins, R., and Paterson, D.: The influence of light and temperature interactions on a natural estuarine microphytobenthic assemblage, Biofilms, 1, 21–30, https://doi.org/10.1017/S1479050503001054, 2004.
Derks, A. K. and Bruce, D.: Rapid regulation of excitation energy in two pennate diatoms from contrasting light climates, Photosynth. Res., 138, 149–165, https://doi.org/10.1007/s11120-018-0558-0, 2018.
Domingues, N., Matos, A. R., da Silva, J. M., and Cartaxana, P.: Response of the Diatom Phaeodactylum tricornutum to photooxidative stress resulting from high light exposure, PLoS One, 7, 1–6, https://doi.org/10.1371/journal.pone.0038162, 2012.
Du, G., Yan, H., Liu, C., and Mao, Y.: Behavioral and physiological photoresponses to light intensity by intertidal microphytobenthos, Chinese J. Oceanol. Limnol., 36, 293–304, https://doi.org/10.1007/s00343-017-6099-0, 2018.
Eggert, A. and Wiencke, C.: Adaptation and acclimation of growth and photosynthesis of five Antarctic red algae to low temperatures, Polar. Biol., 23, 609–618, https://doi.org/10.1007/s003000000130, 2000.
Eppley, R.: Temperature and phytoplankton growth in the sea, Fish. Bull., 70, 1063–1085, 1972.
Fanesi, A., Wagner, H., Becker, A., and Wilhelm, C.: Temperature affects the partitioning of absorbed light energy in freshwater phytoplankton, Freshw. Biol., 61, 1365-1378, https://doi.org/10.1111/fwb.12777, 2016.
Falkowski, P. and LaRoche, J.: Acclimation to spectral irradiance in algae, J. Phycol., 27, 8–14, https://doi.org/10.1111/j.0022-3646.1991.00008.x, 1991.
Falkowski, P. and Raven, J.: Aquatic photosynthesis, 2nd ed. Princeton University Press, Princeton, NJ, USA, 2007.
Goss, R. and Lepetit, B.: Biodiversity of NPQ, J. Plant Physiol., 172, 13–32, https://doi.org/10.1016/j.jplph.2014.03.004, 2015.
Hach Company.: Nitrogen, ammonia, Nessler method (Method 8038), in: DR/2000 Spectrophotometer Handbook: Procedures Manual, 367–370, 1995.
Hilaluddin, F., Yusoff, F. M., and Toda, T.: Shifts in Diatom Dominance Associated with Seasonal Changes in an Estuarine-Mangrove Phytoplankton Community, J. Mar. Sci. Eng., 8, 528, https://doi.org/10.3390/jmse8070528, 2020.
Indrayani, I., Moheimani, N. R., de Boer, K., Bahri, P. A., and Borowitzka, M.A.: Temperature and salinity effects on growth and fatty acid composition of a halophilic diatom, Amphora sp. MUR258 (Bacillariophyceae), J. App. Phycol., 32, 977–987, 2020.
Holm-Hansen, O. and Lorenzen, C.: Fluorometric determination of chlorophyll, J. du Cons., 30, 3–16, 1965.
Jassby, A. D. and Platt, T.: The relationship between photosynthesis and light for natural assemblages of coastal marine phytoplankton, J. Phycol, 12, 421–430, 1976.
Jordan, L., McMinn, A., and Thompson, P.: Diurnal changes of photoadaptive pigments in microphytobenthos, J. Mar. Biol. Assoc., 90, 1025–1032, https://doi.org/10.1017/S0025315409990816, 2010.
Lee, S. and McMinn, A.: Physiological response of temperate microphytobenthos to freezing temperatures, Mar. Biol. Assoc., 93, 2039–2047, https://doi.org/10.1017/S0025315413000593, 2013.
Lefebvre, S., Mouget, J.. and Lavaud, J.: Duration of rapid light curves for determining the photosynthetic activity of microphytobenthos biofilm in situ, Aquat. Bot., 95, 1–8, https://doi.org/10.1016/j.aquabot.2011.02.010, 2011.
Laviale, M., Frankenbach, S., and Serôdio, J.: The importance of being fast: comparative kinetics of vertical migration and non-photochemical quenching of benthic diatoms under light stress, Mar. Biol., 163, 10, https://doi.org/10.1007/s00227-015-2793-7, 2016.
Lavaud, J. and Lepetit, B.: An explanation for the inter-species variability of the photoprotective non-photochemical chlorophyll fluorescence quenching in diatoms, Biochim. Biophys. Acta, 1827, 294–302, https://doi.org/10.1016/j.bbabio.2012.11.012, 2013.
Loik, M. and Harte, J.: High-temperature tolerance of Artemisia tridentata and Potentilla gracilis under a climate change manipulation, Oecologia, 108, 224–231, https://doi.org/10.1007/BF00334645, 1996.
Long, S. and Humphries, S.: Photoinhibition of photosynthesis in nature, Annu. Rev. Plant, 45, 633–662, https://doi.org/10.1146/annurev.pp.45.060194.003221, 1994.
Longhi, M., Schloss, I., and Wiencke, C.: Effect of irradiance and temperature on photosynthesis and growth of two Antarctic benthic diatoms, Gyrosigma subsalinum and Odontella litigiosa, Bot. Mar., 46, 276–284, https://doi.org/10.1515/BOT.2003.025, 2003.
McMinn, A. and Hegseth, E.: Quantum yield and photosynthetic parameters of marine microalgae from the southern Arctic Ocean, Svalbard, J. Mar. Biol. Assoc., 84, 865–871, https://doi.org/10.1017/S0025315404010112h, 2004.
McMinn, A. and Hattori, H.: Effect of time of day on the recovery from light exposure in ice algae from Saroma Ko lagoon, Hokkaido, Polar Biosci., 20, 30–36, 2006.
McMinn, A., Sellah, S., Llah, W., and Mohammad, M.: Quantum yield of the marine benthic microflora of near-shore coastal Penang, Malaysia, Mar. Freshw., 56, 1047–1053, https://doi.org/10.1071/MF05007, 2005.
Mitbavkar, S. and Anil, A. C.: Diatoms of the microphytobenthic community: Population structure in a tropical intertidal sand flat, Mar. Biol., 140, 41–57, https://doi.org/10.1007/s002270100686, 2002.
Mitbavkar, S. and Anil, A.: Vertical migratory rhythms of benthic diatoms in a tropical intertidal sand flat: influence of irradiance and tides, Mar. Biol., 145, 9–20, https://doi.org/10.1007/s00227-004-1300-3, 2004.
Mitbavkar, S. and Anil, A. C.: Diatoms of the microphytobenthic community in a tropical intertidal sand flat influenced by monsoons: spatial and temporal variations, Mar. Biol., 148, 693–709, https://doi.org/10.1007/s10661-017-5936-0, 2006.
Mouget, J., Perkins, R., and Consalvey, M.: Migration or photoacclimation to prevent high irradiance and UV-B damage in marine microphytobenthic communities, Aquat. Microb., 52, 223–232, https://doi.org/10.3354/ame01218, 2008.
Müller, P., Li, X., and Niyogi, K.: Non-photochemical quenching. A response to excess light energy, Plant Physiol., 125, 1558–1566, https://doi.org/10.1104/pp.125.4.1558, 2001.
Oliver, R., Whittington, J., Lorenz, Z., and Webster, I. T.: The influence of vertical mixing on the photoinhibition of variable chlorophyll a fluorescence and its inclusion in a model of phytoplankton photosynthesis, J. Plankt., 25, 9, 1107–1129, https://doi.org/10.1093/plankt/25.9.1107, 2003.
Oxborough, K. and Baker, N.: Resolving chlorophyll a fluorescence images of photosynthetic efficiency into photochemical and non-photochemical components–calculation of qP and Fv-/Fm-; without measuring Fo-;, Photosynth. Res., 54, 135–142, https://doi.org/10.1023/A:1005936823310, 1997.
Patil, J. S. and Anil, A. C.: Temporal variation of diatom benthic propagules in a monsoon-influenced tropical estuary, Cont. Shelf Res., 28, 2404–2416, https://doi.org/10.1016/j.csr.2008.06.001, 2008.
Perkins, R. G., Mouget, J. L., Lefebvre, S., and Lavaud, J.: Light response curve methodology and possible implications in the application of chlorophyll fluorescence to benthic diatoms, Mar. Biol., 149, 703–712, https://doi.org/10.1007/s00227-005-0222-z, 2006.
Perkins, R., Lavaud, J., and Serôdio, J., Mouget, J., Cartaxana, P., Rosa, P., Barille, L., Brotas, V., and Jesus, B.: Vertical cell movement is a primary response of intertidal benthic biofilms to increasing light dose, Mar. Ecol., 416, 103, https://doi.org/10.3354/meps08787, 2010.
Rahaman, S., Golder, J., Rahaman, M., Hasanuzzaman, A., Huq, K., Begum, S., Islam, S., and Bir, J.: Spatial and Temporal Variations in Phytoplankton Abundance and Species Diversity in the Sundarbans Mangrove Forest of Bangladesh, J. Mar. Sci. Res. Dev., 3, 126, https://doi.org/10.4172/2155-9910.1000126, 2013.
Rajesh, K., Gowda, G., and Mendon, M.: Primary Production of Benthic Microalgae in the Tropical Semi-enclosed Brackishwater Pond, Southwest Coast of India, Asian Fish, 14, 357–366, 2001.
Ralph, P. and Gademann, R.: Rapid light curves: a powerful tool to assess photosynthetic activity, Aquat. Bot., 82, 222–237, https://doi.org/10.1016/j.aquabot.2005.02.006, 2005.
Ruban, A., Lavaud, J., Rousseau, B., and Guglielmi, G.: The super-excess energy dissipation in diatom algae: comparative analysis with higher plants, Photosynth. Res., 82, 165, https://doi.org/10.1007/s11120-004-1456-1, 2004.
Salleh, S. and McMinn, A.: The effects of temperature on the photosynthetic parameters and recovery of two temperate benthic microalgae, Amphora cf. coffeaeformis and Cocconeis cf. sublittoralis (Bacillariophyceae), J. Phycol., 47, 1413–1424, https://doi.org/10.1111/j.1529-8817.2011.01079.x, 2011.
Schreiber, U.: Pulse-amplitude-modulation (PAM) fluorometry and saturation pulse method: an overview, in: Chlorophyll a Fluorescence, Advances in Photosynthesis and Respiration, edited by: Papageorgiou, G. and Govindjee, C., Springer, Dordrecht, 19, https://doi.org/10.1007/978-1-4020-3218-9_11, 2004.
Serôdio, J., Cruz, S., Vieira, S., and Brotas, V.: Non-photochemical quenching of chlorophyll fluorescence and operation of the xanthophyll cycle in estuarine microphytobenthos, J. Exp. Mar. Bio. Ecol., 326, 127–169, https://doi.org/10.1016/j.jembe.2005.05.011, 2005.
Serôdio, J., Coelho, H., Vieira, S., and Cruz, S.: Microphytobenthos vertical migratory photoresponse as characterised by light-response curves of surface biomass, Estuar. Coast. Shelf Sci., 68, 547–556, https://doi.org/10.1016/j.ecss.2006.03.005, 2006a.
Serôdio, J., Vieira, S., Cruz, S., and Coelho, H.: Rapid light-response curves of chlorophyll fluorescence in microalgae: relationship to steady-state light curves and non-photochemical quenching in benthic diatom-dominated assemblages, Photosynth. Res., 90, 29–43, https://doi.org/10.1007/s11120-006-9105-5, 2006b.
Serôdio, J., Vieira, S., and Cruz, S.: Photosynthetic activity, photoprotection and photoinhibition in intertidal microphytobenthos as studied in situ using variable chlorophyll fluorescence, Cont. Shelf Res., 28, 1363–1375, https://doi.org/10.1016/j.csr.2008.03.019, 2008.
Thomas, M. K., Kremer, C. T., Klausmeier, C. A., and Litchman, E.: A global pattern of thermal adaptation in marine phytoplankton, Science, 338, 1085–1088, 2012.
Torres, M. A., Ritchie, R. J., Lilley, R. M. C., Grillet, C., and Larkum, A. W. D.: Measurement of photosynthesis and photosynthetic efficiency in two diatoms, New Zeal. J. Bot., 52, 6–27, https://doi.org/10.1080/0028825X.2013.831917, 2014.
Underwood, G.: Adaptations of tropical marine microphytobenthic assemblages along a gradient of light and nutrient availability in Suva Lagoon, Fiji, Eur. J. Phycol., 37, 449–462, https://doi.org/10.1017/S0967026202003785, 2002.
Wu, Y., Yue, F., Xu, J., and Beardall, J.: Differential photosynthetic responses of marine planktonic and benthic diatoms to ultraviolet radiation under various temperature regimes, Biogeosciences, 14, 5029–5037, https://doi.org/10.5194/bg-14-5029-2017, 2017.