the Creative Commons Attribution 4.0 License.
the Creative Commons Attribution 4.0 License.
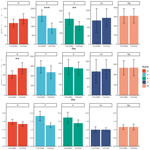
Contrasting strategies of nutrient demand and use between savanna and forest ecosystems in a neotropical transition zone
Marina Corrêa Scalon
Imma Oliveras Menor
Renata Freitag
Karine S. Peixoto
Sami W. Rifai
Beatriz Schwantes Marimon
Ben Hur Marimon Junior
Yadvinder Malhi
The total demand for and uptake of nutrients by vegetation is rarely quantified or compared across vegetation types. Here, we describe different nutrient use and allocation strategies in neotropical savanna (cerrado) and transitional forest (cerradão) tree communities composed of different species, report leaf nutrient resorption and calculate ecosystem-level nutrient use efficiency. We couple net primary productivity (NPP) estimates with nutrient stoichiometry to quantify nutrient demand and nutrient flows at the whole-stand scale for different components of vegetation biomass. Species from the two vegetation communities showed similar mean nutrient concentrations and nutrient resorption efficiency, except for wood P concentration that was fourfold higher in cerrado than cerradão species. The cerradão showed higher canopy NPP, while fine roots and wood NPP were similar for the two vegetation types. Nutrient requirement in the two vegetation types was dominated by the demands of the canopy, with canopy resorption generally contributing more than 50 % of the total canopy demand for nutrients, while less than 35 % of N, P, K, Ca and Mg were allocated to wood or fine roots. Proportionally, cerrado showed higher nutrient demand from fine roots (over 35 % of the total nutrient demand) and for the wood component (over 13 % of the total nutrient demand), while ∼ 60 %–70 % of the cerradão nutrient demand was allocated to the canopy. The proportional difference in nutrient allocation to the different biomass components suggests cerrado species allocate less nutrients to a given fine root biomass, but more nutrients to a given wood biomass. Our findings suggest that cerradão species are more limited in P and K than cerrado species, inducing higher resorption to compensate for low uptake. Moreover, we found that N uptake for cerradão was higher with lower N use efficiency, i.e. the amount of production per nutrient unit, leading higher N demand compared to the cerrado. This difference in nutrient dynamics explains how similar soils and the same climate dominated by savanna vegetation can also support forest-like formations. Tree species composition is likely the major factor regulating nutrient use, limiting vegetation transitions and influencing nutrient demand at landscape scales.
- Article
(1192 KB) - Full-text XML
-
Supplement
(801 KB) - BibTeX
- EndNote
Net primary productivity (NPP), together with carbon and nutrient cycling, are important ecosystem processes that may be primarily limited by nutrient availability (Aragão et al., 2009; Quesada et al., 2009; Fernández-Martínez et al., 2014). Even though there is major interest in the relationship between nutrients and carbon for predictive models of how tropical ecosystems will respond to increasing atmospheric CO2 and climate change (Malhi, 2012), the influence of nutrient availability on carbon balance and sequestration is not well understood. Most studies of nutrient demand are based on simple concentrations and stocks of nutrients in, for example, leaves, wood and soil. In order to assess the flow of and demand for nutrients in vegetation, it is necessary to couple the stoichiometry of key plant organs to the rates of production of those organs, which are assessed through measurements of NPP. By definition, nutrient use efficiency is the amount of production per nutrient unit (Chapin, 1980) and can be estimated as the ratio of NPP per unit of nutrient demand (Bridgham et al., 1995). Nutrient demand is the sum of nutrients accumulated in above- and belowground biomass and nutrient returns to the soil via litterfall including resorption efficiency, while nutrient uptake excludes nutrient resorption efficiency. Nutrient resorption is defined as the process by which plants withdraw nutrients from senescent leaves prior to leaf abscission, and its efficiency is calculated as the proportional resorption from green to senesced leaves (Killingbeck 1996).
In the context of tropical ecosystems, the availability of forest sub-component NPP data has increased substantially in recent years, most notably through the activity of the Global Ecosystems Monitoring (GEM) network (Malhi et al., 2021). The coupling of NPP and stoichiometry data opens the potential for detailed description of plant nutrient demand and nutrient cycling in a variety of ecosystems. Here, we demonstrate how such coupling of NPP estimates to nutrient stoichiometry can be conducted in the context of neotropical savanna and transitional vegetation types.
Although climatic fluctuations have a major role shaping the occurrence and dynamics of forest and savannas (e.g. expansions and retractions) globally, mesic and humid savannas normally persist over areas where climatic conditions could support forest formations (Cole, 1986; Solbrig, 1996). Therefore, in addition to climatic conditions, nutrient availability and species shifts are expected to be important environmental filters (Lehmann et al., 2011). It is suggested that nutrients may be the primary determinant of tree biomass worldwide, since trees experiencing higher nutrient availability may potentially have faster growth rates and therefore be more competitive against grasses and avoid the grass fire trap (Hoffmann et al., 2012). Key limiting nutrients are usually presumed to be nitrogen or phosphorus (Reich and Schoettle, 1988; Reich et al., 2009), but potassium (K), calcium (Ca) and magnesium (Mg) may also play a role (Chapin III, 1980; Grime, 2006). Savanna soils tend to be highly deficient in multiple nutrients (Bucci et al., 2006; Haridasan, 2008; Bustamante et al., 2012), and some authors consider soil fertility as the main factor driving abrupt transitions between savannas and forests (Bond, 2010; Wang et al., 2010; Viani et al., 2011). In the neotropical mesic savannas, when fire is excluded, gradual forest expansion occurs by encroachment of trees, forming forest-like vegetation (Durigan and Ratter, 2006; Hoffmann et al., 2012; Rosan et al., 2019). This means that nutrient availability alone is also not the ultimate constraint on forest formation (Pellegrini, 2016). However, nutrients might influence vegetation encroachment processes by either restricting tree growth rates, maintaining a grass layer that facilitates fire or constraining the ability of forests to form regardless of fire (Case and Staver, 2017).
Soil nutrient availability is determined not only by soil properties, but also by water availability and vegetation structure and composition. For instance, high biomass results in high nutrient turnover and availability (Nardoto et al., 2006; Pellegrini, 2016), and species-specific leaf functional traits can affect the demand for and turnover of nutrients (Craine et al., 2008). While savanna-like vegetation, such as the typical cerrado, is defined by shrubs and trees distributed in a continuous grass and herbaceous layer, savanna–forest transitional vegetation, such as the cerradão, is composed of an almost continuous canopy of trees (usually 70 %–80 % canopy cover) with a thin herbaceous understorey layer (Oliveras and Malhi, 2016). Few plant species are shared between cerrado and cerradão vegetation, implying a distinct set of traits and ecological strategies (Hoffmann et al., 2003; Marimon-Junior and Haridasan, 2005; Marimon et al., 2006). There is an expected evolutionary trade-off involved in slow- and fast-growing species in relation to high-nutrient and low-nutrient habitats, in which plants adapted to nutrient-poor habitats should be more efficient (i.e. build more organic matter for a given unit of nutrient required) than fast-growing plants from nutrient-rich environments (Vitousek, 1982, 1984; Chapin III et al., 1986). Indeed, higher resorption rates are expected in low-nutrient environments (Aerts and Chapin, 1999; Wright and Westoby, 2003; Pellegrini, 2016).
Leaf nutrient concentration and leaf nutrient resorption are obviously major aspects that influence nutrient cycling in a plant community (Vergutz et al. 2012). However, nutrient allocation to different organs (e.g. leaves, roots, wood and bark) can also have important effects on nutrient use and supply in the ecosystem, since different species can have distinct demands for nutrients in terms of tissue stoichiometries. In addition, nutrient deposition and mineralization from different plant material will directly influence nutrient availability (Wardle et al., 2004). While wood can provide a well-defended and long-lived storage organ for nutrients, water and carbohydrates (Chapin III et al., 1990), leaves and fine roots are short-lived and much more vulnerable to herbivores, especially under high nutrient concentrations (Coley et al., 1985; Moles et al., 2013).
To effectively evaluate nutrient use in forest–savanna transitions, there is a need to quantify the nutrient requirement of each vegetation type, which should consider differences in biomass investment for the distinct components (e.g. canopy, wood, roots). By calculating nutrient demand in the savanna–forest transition using literature proxy equations and data, Pellegrini (2016) found that populations of Brazilian savanna species, compared to forest species, required double the N and P to form closed canopies, but the N and P annual demand was substantially greater for forest tree species. These findings suggest that even with lower nutrient requirements to form a forest, transitional forest species may experience higher limitations due to their higher nutrient demand. Species composition and vegetation structure are very distinct between the cerrado and cerradão vegetation (de Oliveira et al., 2017). The mechanism by which these differences occur is still poorly understood, but may involve trade-offs between nutrient requirements and adaptations to fire (Silva et al., 2013) and vegetation encroachment by nucleation of keystone species, such as Tachigali vulgaris (Morandi et al., 2016). Here, we describe different nutrient use and allocation strategies in savanna (cerrado) and transitional forest (cerradão) species, report leaf nutrient resorption and calculate ecosystem-level nutrient use efficiency based on dynamic nutrient flows rather than one-off static estimates of nutrient stocks. We set out to address the following questions and test the following hypotheses:
- i.
How do leaf nutrient concentration and resorption vary across savanna–forest transition species? At the species scale, we expect that there will be more nutrients in different plant organs in cerradão compared to cerrado species. Moreover, based on plant strategies and resource availability limitations, we expect that cerrado species will show higher nutrient resorption efficiency, both at the species and at the community scale.
- ii.
What is the nutrient demand of the cerrado and cerradão communities? We expect that cerradão will have higher NPP for all biomass components and, therefore, higher nutrient demand.
- iii.
What is the partitioning of nutrient demand between canopy, wood and fine roots, and how much of this demand is met by translocation of leaf nutrients rather than by new uptake? We expect that the canopy will dominate nutrient demand in both plant communities, but that the majority of this demand will be met by nutrient translocation from senescing leaves.
2.1 Field site
The study plots, part of the GEM intensive carbon plot network (Malhi et al., 2021), are located in a typical mosaic area of cerrado and adjacent cerradão vegetation in the Bacaba Municipal Park (BMP; 14∘42′22′′ S, 52∘21′07′′ W), Nova Xavantina, Mato Grosso State, Brazil. The park of approximately 500 ha is located in the transition between the Cerrado and Amazon biomes; the climate is Köppen's Aw (i.e. tropical savanna climate), with highly seasonal rainfall and a prolonged and intense dry period from May to September (Marimon-Junior and Haridasan, 2005).
One 1 ha plot of each vegetation type has been monitored every year since 2010 for vegetation dynamics following the standard RAINFOR protocol (Phillips et al., 2002). The plots are 100 m apart from each other. Tree identity, size, location, and growth data for each plot and census are curated and available upon request at the https://forestplots.net (last access: 26 July 2022) database (Lopez-Gonzalez et al., 2011). Our plots represent long-term fire-protected vegetation types, and a rare case of very well-preserved typical cerrado and cerradão vegetation in the Amazon and Cerrado transition (de Oliveira et al., 2017). Typical cerrado is a cerrado stricto sensu subtype with predominantly arboreal shrubby vegetation, 20 % to 50 % tree cover and tree heights between 3 and 6 m (Ribeiro and Walter, 1998). The cerradão is considered an ecotonal community (Ratter et al., 1973), characterized by a mostly continuous canopy and an abundance of species that indicate the transition between forests and savannas on the southern Amazonian border, such as Hirtella glandulosa and Emmotum nitens (Marimon et al., 2006).
Within each 1 ha plot, 25 subplots were delimited, from which soil samples at 0–10 and 10–20 cm depth were collected in all four corners and at the centre of each subplot, totalling 200 samples for each vegetation type. Soil chemistry data were analysed according to the EMBRAPA procedure (EMBRAPA, 1997) and were provided by the ForestPlots database (Lopez-Gonzalez et al. 2011; Table S1 in the Supplement). Despite slightly sandier soils in the cerrado, the soil in both areas is very similar and characterized as dystrophic yellow oxisols, with low nutrient concentration, reduced sum of bases and low cation-exchange capacity (Marimon-Junior and Haridasan, 2005). They are highly acidic, with alic properties, well drained and without concretions up to 2 m deep (Table S1).
2.2 Nutrient sampling
In each plot, we randomly selected five individuals of the seven most abundant species representing approximately 70 % of the community basal area (Table 1). Calculation of relative dominance was based on previous census data collected in the plots (2009, 2012, 2014), by dividing the species dominance (i.e. total basal area of the species) by the community total basal area, and by multiplying it by 100. The IVI was calculated as the sum of the relative frequency, the relative density and the relative dominance of each species within the community. We chose only adult trees of at least 5 cm in diameter at breast hight (DBH) in the cerrado and 10 cm DBH in the cerradão, following standardized criteria for individual inclusion in forest and savanna vegetation. For each species, five individuals were chosen, from which we collected samples from different tissues: heartwood and sapwood, inner and outer bark, branch, mature and old leaves, and fine roots. Inner and outer bark can be easily distinguished visually, but we also used magnified lenses to confirm our classification whenever there was any uncertainty. Ten fully expanded mature sun leaves were collected during the wet season (January 2018) and 10 recently senescent leaves were collected from the same individuals during the dry season (July 2018) because of the deciduousness of most species. We considered senescent leaves to be those that fall with a gentle flick to the branch (Wright and Westoby, 2003). Collected branches were approximately 1 cm in diameter without bark, which was removed before analysis. Wood core samples and bark were collected at breast height (DBH ≈ 1.30 m). Wood samples were extracted using a 4.3 mm Haglof increment borer to a depth of half the DBH of the tree.
Fine root samples were collected at 20 cm soil depth near the coarse root of each individual. While this might include roots from outside the target individual, we were interested in root nutrient composition at the community scale; therefore, this approach was sufficient to address our key question. Samples were sieved and washed with tap water. After being oven dried at 70 ∘C until constant weight, dried samples were sent to Laboratório de Análises de Viçosa-MG for nutrient concentration analyses. N and P (g kg−1) were determined by Kjedahl digestion and UV–Vis spectroscopy, respectively, and the other nutrients (Ca, Mg and K; g kg−1) were determined by atomic absorption spectrometry.
Nutrient resorption efficiency (RE) was calculated following Eq. (1):
where Nutmat is the nutrient concentration of mature leaves and Nutold is the nutrient concentration of senesced leaves and MLCF is the green to senesced leaf Ca ratio (Vitousek and Sanford, 1986). This calculation is based on the assumption that Ca is not translocated, and enables correction for differences in leaf mass per area between mature and senescent leaves.
2.3 Estimation of NPP
Net primary productivity is the rate of organic material production and can be quantified at the scale of an individual plant or an ecosystem (Malhi et al., 2011). Here we quantified the major components of NPP, including the canopy (leaves, twigs and reproductive parts), wood (stem, coarse roots and branches), and fine roots, during the period from 2014 to 2016. Data were collected following GEM protocols (Malhi et al., 2021) and methods are described in detail in Marthews et al. (2014) and Malhi et al. (2021). Briefly, for the canopy NPP component estimation, litter traps sampled biweekly together with monthly canopy leaf area index were used. For wood component estimation, annual census and dendrometers measuring growth rates were converted into woody biomass production. Fine root production was measured with ingrowth cores installed and sampled every 3 months. Estimates of NPP are usually biased because it is very challenging to measure small NPP terms, such as volatile emissions, herbivory losses and mycorrhizae exudates. Nevertheless, these terms are likely minor components of carbon budget (Malhi et al., 2021), and here we focus on major components of NPP (canopy, wood and fine roots), recognizing that, inevitably, these missing terms exist.
For stoichiometric calculations, carbon was assumed to be 50 % of the biomass for wood and canopy components, 48 % for fine roots and 39 % for litter (Biot et al., 1998; Paiva et al., 2011). Nutrient uptake () and nutrient demand () were calculated following Eqs. (2) and (3), respectively:
where NPP is plot-mean NPP () and C:Nut is plot-mean nutrient stoichiometry.
Thus, we calculated nutrient use efficiency (kg C (kg [Nut])−1) as the ratio of total NPP (NPPcanopy + NPPwood + NPPfine roots) to Nutuptake (NutUEuptake) and to Nutdemand (NutUEdemand). Hence, NutUEdemand takes into account that a large part of nutrient demand can be met by translocation from senescing leaves and therefore does not require uptake from the soil.
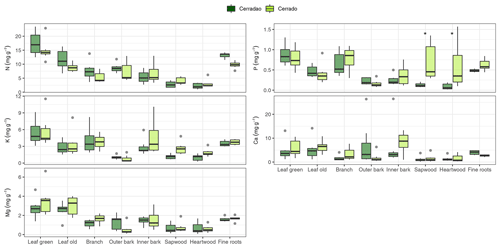
Figure 1Macronutrient (N, P, K, Ca and Mg) concentrations (%) for different plant organs (leaf, branch, outer bark, inner bark, sapwood, heartwood and fine roots) in cerradão (dark green boxes) and cerrado (light green boxes) vegetation. The continuous line within the box shows the median, and error bars show 10th and 90th percentiles (n = 7 species per vegetation type). Asterisks (∗) represent differences between the two sites (P < 0.05). Species-specific concentrations are shown in Fig. S1 in the Supplement.
2.4 Statistical analyses
To upscale the species value for the whole communities, we calculated the community-weighted mean (CWM) for each organ using the species relative dominance to weight the nutrient concentration (Muscarella and Uriarte, 2016). To compare nutrient concentration average means and resorption efficiencies between the two vegetation types and between different organs, we performed a two-way multivariate analysis of variance (MANOVA) followed by two-way univariate analysis of variances (ANOVAs) and Tukey HSD post-hoc test. The independent variables were site and plant organ, while the dependent variables for the MANOVA were the different nutrient concentrations (N, P, K, Ca, Mg) or resorption. Data normality and homogeneity of variance assumptions were previously checked with Shapiro–Wilk multivariate normality test using package “mvnormtest” (Jarek, 2012) and Levene test using package “car” (Fox et al., 2012), respectively. All statistical analyses were performed in R software version 4.0.1 (R Core Team, 2019).
To account for the systematic uncertainties intrinsic to the methods used for NPP calculations for the different components, we assigned sampling uncertainties to each measurement and propagated them through our calculations. Following the standard rules of quadrature (Hughes and Hase, 2010), we calculated the standard error of the mean (SE) and propagated it by adding the components in quadrature, assuming the uncertainties in the independent variables were uncorrelated. To calculate differences between sites for NPP, nutrient demand of different components, and nutrient use and uptake efficiencies, we performed z-tests using the average value for each site and the propagated error.
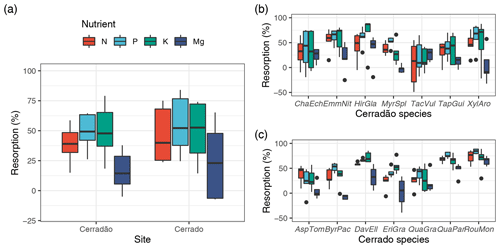
Figure 2Nutrient (N, P, K, Mg) resorption efficiency (%) averaged for both vegetation types considering the community-weighted mean for each species (a) and calculated for each of the species representing the transition forest (cerradão, b), savanna (cerrado, c). The continuous line within the box shows the median, and error bars show 10th and 90th percentiles (n = 5 individuals per species). Species abbreviations refer to the first three letters of the genera followed by the first three letters of the specific epithet, as followed from left to right: cerradão species (Chaetocarpus echinocarpus, Emmotum nitens, Hirtella glandulosa, Myrcia splendens, Tachigali vulgaris, Tapirira guianensis, Xylopia aromatica); and savanna species (Aspidosperma tomentosum, Byrsonima pachyphylla, Davilla elliptica, Eriotheca gracipiles, Qualea grandiflora, Qualea parviflora, Roupala montana).
3.1 Savanna–forest differences
For species mean comparisons, transition forest species showed higher leaf (green and old leaves), branch and root N concentration, while savanna species showed higher wood nutrients in general (higher N, P, K) for both sapwood and heartwood (MANOVA: F = 1.52, P = 0.029, Fig. 1). Cerrado species also showed higher leaf Mg concentrations and higher P and K inner bark concentrations. For community-weighted means, however, we did not find an identifiable interaction effect between the different plant organs and vegetation types (MANOVA: F = 1.28, P = 0.134), meaning that there was generally no difference in nutrient concentration between cerrado and cerradão across the different tree components when species abundance and basal area were taken into account. Moreover, despite slightly higher nutrient resorption in the savanna, there were no differences between the two vegetation types (MANOVA: F = 0.23, P = 0.916; Fig. 2a). In general, we found higher P resorption (cerrado: 58.50 ± 18.97 % and cerradão: 50.44 ± 15.72 %) than N (cerrado: 49.46 ± 19.44 % and cerradão: 39.58 ± 15.39 %) for both sites and all species (Fig. 2).
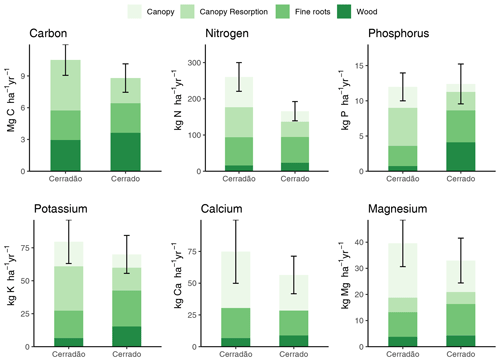
Figure 3Net primary productivity (NPP; ) and nutrient demands (N, P, K, Ca and Mg) partitioned into biomass components: canopy demand met by resorption (canopy resorption), canopy demand met by new uptake (canopy), wood and fine roots in cerradão (transition forest) and typical cerrado (savanna). Error bars indicate ± SE for all components pulled together (see Table S2 for comparison test results and Table 2 for individual values and errors).
3.2 Nutrient demand and partition
Cerradão yielded higher NPP for canopy (cerradão: 4.78 ± 0.12 and cerrado: 2.38 ± 0.08 ; P < 0.001) but similar NPP values were observed for wood (cerradão: 2.77 ± 0.58 and cerrado: 2.79 ± 0.45 ; P = 0.111) and fine roots (cerradão: 2.96 ± 0.76 and cerrado: 3.63 ± 0.81 ; P = 0.954, Fig. 3a, Table S2 in the Supplement) components. In agreement with our expectations, nutrient demand was generally higher for cerradão species for all nutrients, except for nutrient demand for the wood component, especially for phosphorus, which was fourfold higher in the cerrado plot, but also for N and K (Fig. 3).
Table 2The total (mean ± SE, ) and proportional (%) partitioning of nutrient demand between canopy, wood and fine roots, together with the component of overall nutrient demand met via resorption from senescent leaves, for transitional forest (cerradão) and savanna (cerrado) sites.
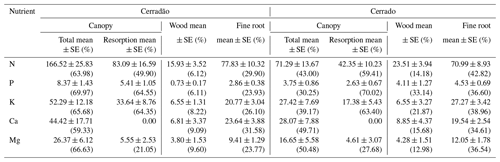
Nutrient demand in the cerradão was predominantly allocated to the canopy, with nutrient demand met by resorption generally corresponding to ∼ 50 % of the total canopy demand, while less than 35 % of the N, P, K, Ca and Mg were allocated to wood or fine roots (Table 2). For cerrado vegetation, the proportional allocation of nutrients was more equally partitioned across the different organs. For instance, in the cerrado, root nutrient demand was similar to total canopy nutrient demand for all nutrients, while P demand of the wood component corresponded to ∼ 50 % of the total phosphorus demand. In contrast, cerradão demanded proportionally more nutrients in the canopy, while cerrado demanded proportionally more N, P and K, Ca and Mg in the wood and fine roots (Table 2).
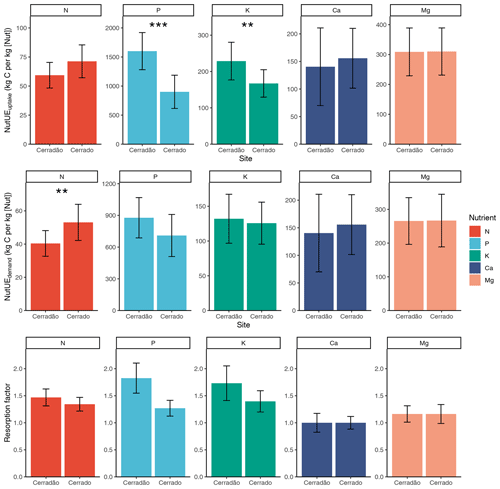
Figure 4Nutrient uptake efficiency (kg C (kg [Nut])−1), nutrient use efficiency (kg C (kg [Nut])−1), resorption factor (i.e. the demand-to-uptake ratio) in cerradão (transition forest) and typical cerrado (savanna) vegetation. Error bars indicate ± SE and asterisks show significant differences between sites (z-tests, 0.01 < P < 0.05; P < 0.001; Table S3).
PUEuptake and KUEuptake were ∼ 45 % and ∼ 30 % higher for the cerradão plot, respectively (P < 0.001 and P = 0.011, respectively; Table S3 in the Supplement), while NUEuptake was ∼ 17 % higher in the cerrado plot (Fig. 4, non-significant, P = 0.078; Table S3). NUEuptake, CaUEuptake and MgUEuptake were similar between cerradão and cerrado (Fig. 4; Table S3). For nitrogen, cerradão uptake was similar, with similar resorption factor and lower (∼ 24 %) NUEdemand (P = 0.012; Table S3), leading to higher N demand compared to the cerrado (Fig. 4). For P, there was a decrease in uptake in the cerradão coupled with an increase in resorption and 24 % higher PUEdemand (non-significant, P = 0.107; Table S3), leading to little overall change in P demand (Fig. 4). There was little difference in K uptake between the sites, but the increased resorption in cerradão supports greater demand (Fig. 4). Comparing cerrado and cerradão plots, Mg uptake matched demand and there was little adjustment in resorption or nutrient use efficiency (Fig. 4).
Unexpectedly, there was no major difference between cerradão and cerrado species nutrient content in the different plant organs. The only and most remarkable difference was in wood P concentration, where we found a clear trend of all cerrado species allocating very high amounts of P to the sapwood and heartwood. Wood nutrient allocation directly affects nutrient residence time at the whole-tree level: nutrients allocated to the canopy lead to larger flux via fast turnover, meaning a shorter residence time, compared to nutrients allocated to wood biomass (Tsujii et al., 2020). Our results agree with the current literature suggesting that P in tropical ecosystems is a key limiting nutrient (Malhi et al., 2009; Quesada et al., 2010) and may affect transition ecosystem dynamics (Dionizio et al., 2018), suggesting two distinct strategies in the cerrado and cerradão vegetation. In the cerradão, transition forest species may allocate P to the canopy as an adaptive mechanism to maintain higher photosynthetic rates (Gleason et al., 2009; Reich et al., 2009, Aoyagi and Kitayama, 2016) to support the high net primary productivity in low-P soils. In cerrado, savanna species might allocate P to the wood as a mechanism to extend P residence time in the living biomass (Tsujii et al., 2020).
The strategy of storing P in wood biomass of cerrado species may be important to avoid P loss by recurrent fires. Although soil P may even increase shortly after fire (Schaller et al., 2015), P-binding components in ash may decrease P availability, by forming low-soluble P forms that are not readily available to plants (Schaller et al., 2015, Ngoc Nguyen et al., 2014). Furthermore, the loss of P stocks after fire because of volatilization remains anecdotal, because P has a high volatilization temperature (above 770 ∘C, DeBano, 2000) and cerrado fires are usually very fast and do not achieve these high temperatures (Miranda et al., 1993). Although some experimental studies comparing the amount of nutrients in the fuel and in the ash after fire found a ∼ 50 % reduction of P content (Pivello and Coutinho, 1992; Kauffman et al., 1994), Resende and others (2011) suggested that the decrease in organic P fraction and P availability in cerrado burned plots compared to unburned plots was probably caused by the decrease in organic inputs through litterfall, rather than a direct effect of fire. In the same study area as ours, de Oliveira and others (2017) found higher decomposition of the total biomass and higher nutrient cycling through litterfall in the cerradão compared to the cerrado plot. Indeed, Mendes and others (2012) have shown that forest-like vegetation types in the Cerrado biome (cerradão and gallery forests) presented higher microbial biomass and soil biological functioning compared to savannas. Therefore, we suggest that cerradão species may have adopted the strategy of relying on the soil biota activity and higher litterfall production to promote rapid nutrient cycling, whereas cerrado vegetation species may have evolved by natural selection to minimize P loss, by storing P in the wood. Nevertheless, further research into nutrient dynamics in these vegetation types is needed to test this hypothesis.
Nutrient resorption efficiency was also similar between cerrado and cerradão vegetation, and our results are in agreement with the global average range for woody angiosperms (Vergutz et al., 2012). Our fine-scale leaf resorption results are consistent with previous reports suggesting that effective nutrient cycling in the cerradão compensates for the low saturation of exchangeable bases in the soil (de Oliveira et al., 2017).
While the cerradão showed higher total NPP and higher nutrient demand in all elements of canopy production, cerrado vegetation allocated more nutrients to root and wood, demanding a higher amount of most nutrients (particularly P and K) despite showing similar woody NPP. Differences in fine root nutrient demand between cerrado and cerradão varied by nutrient. The cerrado plot showed similar fine root NPP to the cerradão and demanded a similar amount of N, Ca and Mg. However, cerrado species demanded more P and K to the fine roots, suggesting that generally, cerrado species require more nutrients for fine roots production. Fine roots are an important component of the biomass, reflected in a higher uptake of limiting nutrients in soil (Loiola et al., 2016), and are essential for overcoming the strong water deficit during the dry season in the Cerrado (Oliveira et al., 2005). Therefore, the efficient fine root production in the cerradão vegetation may be an important adaptation to readily absorb water and nutrients during the strongly seasonal rainfall period (February et al., 2013). However, since our sampling strategy may have measured non-target species, such as grasses, our results could be biased.
Ultimately, cerradão species showed higher NUEuptake for both P and K, which may explain how similar soils and the same climate can support forest-like formations. Cerradão vegetation requires more N, Ca and Mg, and less P, but adjusts the stoichiometry and boosts P resorption, leading to similar overall usage of P, while K resorption is increased, which supports greater NPP despite a similar net uptake. The relatively similar Ca and Mg NUEuptake between sites suggests that both ecosystems are able to satisfy their Ca and Mg requirements (uptake tracks demand, with only modest adjustments in stoichiometry), whereas P, N and to some extent K, may be more limiting nutrients.
In particular, the high P use efficiency (PUE) exhibited for both cerradão and cerrado vegetation is an expected adaptation for dealing with low-P soils, and extending P residence time is an adaptive trait to increase PUE. Indeed, in Bornean and Australian low-P tropical forest species, P residence time in the aboveground component was shown to increase with P deficiency (Paoli et al., 2005; Gleason et al., 2009; Tsujii et al., 2020). However, direct measurements of nutrient concentrations and stocks in wood and fine roots are scarce in tropical forests (Johnson et al., 2001; Feldpausch et al., 2004; Li et al., 2010; Heineman et al., 2016) or limited to a single nutrient (Imai et al., 2010; Tsujii et al. 2020).
The lower N use efficiency and the higher P and K use efficiencies in the cerradão suggests that differences in tree community composition and the species-specific functional traits may considerably influence nutrient cycling. For example, Tachigali vulgaris, which contributes ∼ 20 % to the basal area in the cerradão), was described as a key N-fixing species (de Castro et al., 1998) that promotes tree encroachment by increasing enriched litterfall production and facilitating growth and development of other tree species (Morandi et al., 2016; de Oliveira et al., 2017). Indeed, the increased N uptake in the cerradão allows for a reduced NUE, meaning more N in stoichiometry. However, it is important to note that our results are based on two plots, and there may be potential misinterpretation due to any particularity of these studied sites. Even though we examined the only cerrado and cerradão established sites with intensive monthly data collection and monitoring, our findings would obviously benefit from further testing with more savanna and transition forest sites.
By using the direct relationship between nutrient stoichiometry and NPP for different biomass components, we showed that cerrado vegetation is less efficient in fine root and wood production, requiring more nutrients per unit of NPP compared to the cerradão. Ultimately, we also report the different strategies in P use between cerrado and cerradão that may reflect savanna vegetation adaptation to the indirect effects of fire in the soil biota, which is a recurrent disturbance that increases the risk of losing P. With the increasing fire frequency associated with extreme drought events due to climate change, cerradão vegetation, which strongly relies on rapid nutrient cycling, may suffer further P limitation.
Dataset and code are available through figshare (https://doi.org/10.6084/m9.figshare.20387082, Scalon, 2022).
The supplement related to this article is available online at: https://doi.org/10.5194/bg-19-3649-2022-supplement.
MCS, IO and YM conceived the idea, questions and hypotheses; IO and YM secured funding for research; MCS, IO, BSM, BHMJ and YM developed the study design and methodology; MCS, RF and KSP collected the data; MCS and SR analysed the data; MCS led the writing of the manuscript, and all authors critically contributed to the drafts and gave final approval for publication.
The contact author has declared that none of the authors has any competing interests.
Publisher's note: Copernicus Publications remains neutral with regard to jurisdictional claims in published maps and institutional affiliations.
We thank NERC (Natural Environmental Research Council) BIO-RED (#ACR00460) for funding this research. We also thank Camila Borges Silva, Halina Jancoski, Edmar Almeida and Igor Araújo for the valuable help during fieldwork. Marina Corrêa Scalon is currently supported by a postdoctoral fellowship from CAPES (Coordenação de Aperfeiçoamento de Pessoal de Nível Superior), Brazil. Yadvinder Malhi is supported by the Jackson Foundation.
This research has been supported by the Natural Environment Research Council (grant no. ACR00460).
This paper was edited by Sara Vicca and reviewed by two anonymous referees.
Aerts, R. and Chapin, F. S.: The mineral nutrition of wild plants revisited: a re-evaluation of processes and patterns, Adv. Ecol. Res., 30, 1–67, https://doi.org/10.1016/S0065-2504(08)60016-1, 1999.
Aoyagi, R. and Kitayama, K.: Nutrient allocation among plant organs across 13 tree species in three Bornean rain forests with contrasting nutrient availabilities, J. Plant Res., 129, 675–684, https://doi.org/10.1007/s10265-016-0826-z, 2016.
Aragão, L. E. O. C., Malhi, Y., Metcalfe, D. B., Silva-Espejo, J. E., Jiménez, E., Navarrete, D., Almeida, S., Costa, A. C. L., Salinas, N., Phillips, O. L., Anderson, L. O., Alvarez, E., Baker, T. R., Goncalvez, P. H., Huamán-Ovalle, J., Mamani-Solórzano, M., Meir, P., Monteagudo, A., Patiño, S., Peñuela, M. C., Prieto, A., Quesada, C. A., Rozas-Dávila, A., Rudas, A., Silva Jr., J. A., and Vásquez, R.: Above- and below-ground net primary productivity across ten Amazonian forests on contrasting soils, Biogeosciences, 6, 2759–2778, https://doi.org/10.5194/bg-6-2759-2009, 2009.
Biot, Y., Higuchi, N., Minette, L., dos Santos, J., and Ribeiro, R. J.: Biomassa da parte aérea da vegetação da floresta tropical úmida de terra-firme da Amazônia brasileira, Acta Amazon., 28, 153–166, https://doi.org/10.1590/1809-43921998282166, 1998.
Bond, W. J.: Do nutrient-poor soils inhibit development of forests? A nutrient stock analysis, Plant Soil, 334, 47–60, https://doi.org/10.1007/s11104-010-0440-0, 2010.
Bridgham, S. D., Pastor, J., McClaugherty, C. A., and Richardson, C. J.: Nutrient-use efficiency: a litterfall index, a model, and a test along a nutrient-availability gradient in North Carolina peatlands, Am. Nat., 145, 1–21, https://doi.org/10.1086/285725, 1995.
Bucci, S. J., Scholz, F. G., Goldstein, G., Meinzer, F. C., Franco, A. C., Campanello, P. I., Villalobos-Vega, R., Bustamante, M., and Miralles-Wilhelm, F.: Nutrient availability constrains the hydraulic architecture and water relations of savannah trees, Plant Cell Environ., 29, 2153–2167, https://doi.org/10.1111/j.1365-3040.2006.01591.x, 2006.
Bustamante, M. M., de Brito, D. Q., Kozovits, A. R., Luedemann, G., de Mello, T. R., de Siqueira Pinto, A., Munhoz, C. B., and Takahashi, F. S.: Effects of nutrient additions on plant biomass and diversity of the herbaceous-subshrub layer of a Brazilian savanna (Cerrado), Plant Ecol., 213, 795–808, https://doi.org/10.1007/s11258-012-0042-4, 2012.
Case, M. F. and Staver, A. C.: Fire prevents woody encroachment only at higher-than-historical frequencies in a South African savanna, J. Appl. Ecol., 54, 955–962, https://doi.org/10.1111/1365-2664.12805, 2017.
Chapin III, F. S.: The mineral nutrition of wild plants, Annu. Rev. Ecol. Evol. S., 11, 233–260, https://doi.org/10.1146/annurev.es.11.110180.001313, 1980.
Chapin III, F. S., Schulze, E. D., and Mooney, H. A.: The ecology and economics of storage in plants, Annu. Rev. Ecol. Evol. S., 21, 423–447, https://doi.org/10.1146/annurev.es.21.110190.002231, 1990.
Chapin III, F. S., Vitousek, P. M., and Van Cleve, K.: The nature of nutrient limitation in plant communities, Am. Nat., 127, 48–58, https://doi.org/10.1086/284466, 1986.
Cole, M. M.: The savannas, Prog. Phys. Geogr., 11, 334–355, https://doi.org/10.1177/030913338701100302, 1986.
Coley, P. D., Bryant, J. P., and Chapin III, F. S.: Resource availability and plant antiherbivore defense, Science, 230, 895–899, https://doi.org/10.1126/science.230.4728.895, 1985.
Craine, J. M., Morrow, C., and Stock, W. D.: Nutrient concentration ratios and co-limitation in South African grasslands, New Phytol., 179, 829–836, https://doi.org/10.1111/j.1469-8137.2008.02513.x, 2008.
de Castro, A. W. V., de Farias, N., Tomé, J., and da Silva Cavalcante, E.: Efeito do espaçamento na produtividade de biomassa de taxi-branco (Sclerolobium paniculatum Vogel), Acta Amazon., 28, 141–141, https://doi.org/10.1590/1809-43921998282146, 1998.
de Oliveira, B., Junior, B. H. M., Mews, H. A., Valadão, M. B. X., and Marimon, B. S.: Unraveling the ecosystem functions in the Amazonia–Cerrado transition: evidence of hyperdynamic nutrient cycling, Plant Ecol., 218, 225–239, https://doi.org/10.1007/s11258-016-0681-y, 2017.
DeBano, L. F.: The role of fire and soil heating on water repellency in wildland environments: a review, J. Hydrol., 231, 195–206, https://doi.org/10.1016/S0022-1694(00)00194-3, 2000.
Dionizio, E. A., Heil Costa, M., Castanho, A. D. D. A., Ferreira Pires, G., Schwantes Marimon, B., Hur Marimon-Junior, B., Lenza, E., Martins Pimenta, F., Yang, X., and Jain, A. K.: Influence of climate variability, fire and phosphorus limitation on vegetation structure and dynamics of the Amazon–Cerrado border, Biogeosciences, 15, 919–936, https://doi.org/10.5194/bg-15-919-2018, 2018.
Durigan, G. and Ratter, J.: Successional changes in cerrado and cerrado/forest ecotonal vegetation in western Sao Paulo State, Brazil, 1962–2000, Edinb. J. Bot., 63, 119–130, https://doi.org/10.1017/S0960428606000357, 2006.
EMBRAPA (Empresa Brasileira de Pesquisa Agropecuária): Manual de métodos de análise de solo, Centro Nacional de Pesquisa de Solos, Rio de Janeiro, 1997.
February, E., Cook, G., and Richards, A. E.: Root dynamics influence tree–grass coexistence in an Australian savanna, Austral. Ecol., 38, 66–75, https://doi.org/10.1111/j.1442-9993.2012.02376.x, 2013.
Feldpausch, T. R., Rondon, M. A., Fernandes, E. C., Riha, S. J., and Wandelli, E.: Carbon and nutrient accumulation in secondary forests regenerating on pastures in central Amazonia, Ecol. Appl., 14, 164–176, https://doi.org/10.1890/01-6015, 2004.
Fernández-Martínez, M., Vicca, S., Janssens, I. A., Sardans, J., Luyssaert, S., Campioli, M., Chapin III, F. S., Ciais, P., Malhi, Y., and Obersteiner, M.: Nutrient availability as the key regulator of global forest carbon balance, Nat. Clim. Change, 4, 471, https://doi.org/10.1038/nclimate2177, 2014.
Fox, J., Weisberg, S., Adler, D., Bates, D., Baud-Bovy, G., Ellison, S., Firth, D., Friendly, M., Gorjanc, G., and Graves, S.: Package 'car', R Foundation for Statistical Computing, Vienna, 2012.
Gleason, S. M., Read, J., Ares, A., and Metcalfe, D. J.: Phosphorus economics of tropical rainforest species and stands across soil contrasts in Queensland, Australia: understanding the effects of soil specialization and trait plasticity, Funct. Ecol., 23, 1157–1166, https://doi.org/10.1111/j.1365-2435.2009.01575.x, 2009.
Grime, J. P.: Plant strategies, vegetation processes, and ecosystem properties, John Wiley & Sons, England, 2006.
Haridasan, M.: Nutritional adaptations of native plants of the cerrado biome in acid soils, Braz. J. Plant. Physiol., 20, 183–195, https://doi.org/10.1590/S1677-04202008000300003, 2008.
Heineman, K. D., Turner, B. L., and Dalling, J. W.: Variation in wood nutrients along a tropical soil fertility gradient, New Phytol., 211, 440–454, https://doi.org/10.1111/nph.13904, 2016.
Hoffmann, W. A., Orthen, B., and do Nascimento, P. K. V.: Comparative fire ecology of tropical savanna and forest trees, Funct. Ecol., 17, 720–726, https://doi.org/10.1111/j.1365-2435.2003.00796.x, 2003.
Hoffmann, W. A., Geiger, E. L., Gotsch, S. G., Rossatto, D. R., Silva, L. C., Lau, O. L., Haridasan, M., and Franco, A. C.: Ecological thresholds at the savanna-forest boundary: how plant traits, resources and fire govern the distribution of tropical biomes, Ecol. Lett., 15, 759–768, https://doi.org/10.1111/j.1461-0248.2012.01789.x, 2012.
Hughes, I. and Hase, T.: Measurements and their uncertainties: a practical guide to modern error analysis, Oxford University Press, New York, 2010.
Imai, N., Kitayama, K., and Titin, J.: Distribution of phosphorus in an above-to below-ground profile in a Bornean tropical rain forest, J. Trop. Ecol., 26, 627–636, https://doi.org/10.1017/S0266467410000350, 2010.
Jarek, S.: Package “mvnormtest”, R package version 0.1–9, http://cran.r-project.org/web/packages/mvnormtest/index (last access: 28 July 2022), 2012.
Johnson, C. M., Vieira, I. C. ., Zarin, D. J., Frizano, J., and Johnson, A. H. Carbon and nutrient storage in primary and secondary forests in eastern Amazônia, Forest Ecol. Manag., 147, 245–252, https://doi.org/10.1016/S0378-1127(00)00466-7, 2001.
Kauffman, J. B., Cummings, D., and Ward, D.: Relationships of fire, biomass and nutrient dynamics along a vegetation gradient in the Brazilian cerrado, J. Ecol., 82, 519–531, https://doi.org/10.2307/2261261, 1994.
Killingbeck, K. T.: Nutrients in senesced leaves: keys to the search for potential resorption and resorption proficiency, Ecology, 77, 1716–1727, https://doi.org/10.2307/2265777, 1996.
Lehmann, C. E., Archibald, S. A., Hoffmann, W. A., and Bond, W. J.: Deciphering the distribution of the savanna biome, New Phytol., 191, 197–209, https://doi.org/10.1111/j.1469-8137.2011.03689.x, 2011.
Li, A., Guo, D., Wang, Z., and Liu, H.: Nitrogen and phosphorus allocation in leaves, twigs, and fine roots across 49 temperate, subtropical and tropical tree species: a hierarchical pattern, Funct. Ecol., 24, 224–232, https://doi.org/10.1111/j.1365-2435.2009.01603.x, 2010.
Loiola, P. P., Carvalho, G. H., and Batalha, M. A.: Disentangling the roles of resource availability and disturbance in fine and coarse root biomass in savanna, Austral. Ecol., 41, 255–262, https://doi.org/10.1111/aec.12306, 2016.
Lopez-Gonzalez, G., Lewis, S. L., Burkitt, M., and Phillips, O. L.: ForestPlots.net: a web application and research tool to manage and analyse tropical forest plot data, J. Veg. Sci., 22, 610–613, https://doi.org/10.1111/j.1654-1103.2011.01312.x, 2011.
Malhi, Y.: The productivity, metabolism and carbon cycle of tropical forest vegetation, J. Ecol., 100, 65–75, https://doi.org/10.1111/j.1365-2745.2011.01916.x, 2012.
Malhi, Y., Aragao, L. E. O., Metcalfe, D. B., Paiva, R., Quesada, C. A., Almeida, S., Anderson, L., Brando, P., Chambers, J. Q., and Da Costa, A. C.: Comprehensive assessment of carbon productivity, allocation and storage in three Amazonian forests, Glob. Change Biol., 15, 1255–1274, https://doi.org/10.1111/j.1365-2486.2008.01780.x, 2009.
Malhi, Y., Doughty, C., and Galbraith, D.: The allocation of ecosystem net primary productivity in tropical forests, Philos. T. R. Soc. B, 366, 3225–3245, https://doi.org/10.1098/rstb.2011.0062, 2011.
Malhi, Y., Girardin, C., Metcalfe, D. B., Doughty, C. E., Aragão, L. E., Rifai, S. W., Oliveras, I., Shenkin, A., Aguirre-Gutiérrez, J., and Dahlsjö, C. A.: The Global Ecosystems Monitoring network: Monitoring ecosystem productivity and carbon cycling across the tropics, Biol Conserv, 253, 108889, https://doi.org/10.1016/j.biocon.2020.108889, 2021.
Marimon, B., Lima, E. D. S., Duarte, T., Chieregatto, L., and Ratter, J.: Observations on the vegetation of northeastern Mato Grosso, Brazil. IV. An analysis of the Cerrado–Amazonian Forest ecotone, Edinb. J. Bot., 63, 323–341, https://doi.org/10.1017/S0960428606000576, 2006.
Marimon-Junior, B. H. and Haridasan, M.: Comparação da vegetação arbórea e características edáficas de um cerradão e um cerrado sensu stricto em áreas adjacentes sobre solo distrófico no leste de Mato Grosso, Brasil, Acta Bot. Bras., 19, 913–926, https://doi.org/10.1590/S0102-33062005000400026, 2005.
Marthews, T. R., Riutta, T., Oliveras Menor, I., Urrutia, R., Moore, S., Metcalfe, D., Malhi, Y., Phillips, O., Huaraca Huasco, W., Ruiz Jaén, M., and Girardin, C.: Measuring tropical forest carbon allocation and cycling: a RAINFOR-GEM field manual for intensive census plots (v3.0), Manual, Global Ecosystems Monitoring network, http://gem.tropicalforests.ox.ac.uk/ (last access: 26 July 2022), 2014.
Mendes, I., Fernandes, M. F., Chaer, G. M., and dos Reis Junior, F. B.: Biological functioning of Brazilian Cerrado soils under different vegetation types, Plant Soil, 359, 183–195, https://doi.org/10.1007/s11104-012-1195-6, 2012.
Miranda, A. C., Miranda, H. S., Dias, I. F. O., and Dias, B. F. S.: Soil and air temperatures during prescribed cerated fires in Central Brazil, J. Trop. Ecol., 9, 313–320, https://doi.org/10.1017/S0266467400007367, 1993.
Moles, A. T., Peco, B., Wallis, I. R., Foley, W. J., Poore, A. G., Seabloom, E. W., Vesk, P. A., Bisigato, A. J., Cella-Pizarro, L., and Clark, C. J.: Correlations between physical and chemical defences in plants: tradeoffs, syndromes, or just many different ways to skin a herbivorous cat?, New Phytol., 198, 252–263, https://doi.org/10.1111/nph.12116, 2013.
Morandi, P., Marimon-Junior, B., de Oliveira, E., Reis, S., Valadão, M. X., Forsthofer, M., Passos, F., and Marimon, B.: Vegetation succession in the Cerrado–Amazonian forest transition zone of Mato Grosso state, Brazil, Edinb. J. Bot., 73, 83–93, https://doi.org/10.1017/S096042861500027X, 2016.
Muscarella, R. and Uriarte, M.: Do community-weighted mean functional traits reflect optimal strategies?, P. Roy. Soc. B-Biol. Sci., 283, 20152434, https://doi.org/10.1098/rspb.2015.2434, 2016.
Nardoto, G. B., da Cunha Bustamante, M. M., Pinto, A. S., and Klink, C. A.: Nutrient use efficiency at ecosystem and species level in savanna areas of Central Brazil and impacts of fire, J. Trop. Ecol., 22, 191–201, https://doi.org/10.1017/s0266467405002865, 2006.
Ngoc Nguyen, M., Dultz, S., and Guggenberger, G.: Effects of pretreatment and solution chemistry on solubility of rice‐straw phytoliths, J. Plant Nutr. Soil Sc., 177, 349–359, https://doi.org/10.1002/jpln.201300056, 2014.
Oliveira, R., Bezerra, L., Davidson, E., Pinto, F., Klink, C., Nepstad, D., and Moreira, A.: Deep root function in soil water dynamics in cerrado savannas of central Brazil, Funct. Ecol., 19, 574–581, https://doi.org/10.1111/j.1365-2435.2005.01003.x, 2005.
Oliveras, I. and Malhi, Y.: Many shades of green: the dynamic tropical forest–savannah transition zones, Philos. T. R. Soc. B, 371, 20150308, https://doi.org/10.1098/rstb.2015.0308, 2016.
Paiva, A. O., Rezende, A. V., and Pereira, R. S.: Estoque de carbono em cerrado sensu stricto do Distrito Federal, Rev. Arvore, 35, 527–538, https://doi.org/10.1590/S0100-67622011000300015, 2011.
Paoli, G. D., Curran, L. M., and Zak, D. R.: Phosphorus efficiency of Bornean rainforest productivity: Evidence against the unimodal efficiency hypothesis, Ecology, 86, 1548–1561, https://doi.org/10.1890/04-1126, 2005.
Pellegrini, A. F.: Nutrient limitation in tropical savannas across multiple scales and mechanisms, Ecology, 97, 313–324, https://doi.org/10.1890/15-0869.1, 2016.
Phillips, O., Baker, T., Feldspauch, T., and Brienen, R.: Field manual for plot establishment and remeasurement (RAINFOR), Sixth Framework Programme (2002–2006), Amazon Forest Inventory Network, https://doi.org/10.13140/RG.2.1.1735.7202, 2002.
Pivello, V. R. and Coutinho, L. M.: Transfer of macro-nutrients to the atmosphere during experimental burnings in an open cerrado (Brazilian savanna), J. Trop. Ecol., 8, 487–497, https://doi.org/10.1017/S0266467400006829, 1992.
Quesada, C. A., Lloyd, J., Schwarz, M., Patiño, S., Baker, T. R., Czimczik, C., Fyllas, N. M., Martinelli, L., Nardoto, G. B., Schmerler, J., Santos, A. J. B., Hodnett, M. G., Herrera, R., Luizão, F. J., Arneth, A., Lloyd, G., Dezzeo, N., Hilke, I., Kuhlmann, I., Raessler, M., Brand, W. A., Geilmann, H., Moraes Filho, J. O., Carvalho, F. P., Araujo Filho, R. N., Chaves, J. E., Cruz Junior, O. F., Pimentel, T. P., and Paiva, R.: Variations in chemical and physical properties of Amazon forest soils in relation to their genesis, Biogeosciences, 7, 1515–1541, https://doi.org/10.5194/bg-7-1515-2010, 2010.
Quesada, C. A., Phillips, O. L., Schwarz, M., Czimczik, C. I., Baker, T. R., Patiño, S., Fyllas, N. M., Hodnett, M. G., Herrera, R., Almeida, S., Alvarez Dávila, E., Arneth, A., Arroyo, L., Chao, K. J., Dezzeo, N., Erwin, T., di Fiore, A., Higuchi, N., Honorio Coronado, E., Jimenez, E. M., Killeen, T., Lezama, A. T., Lloyd, G., López-González, G., Luizão, F. J., Malhi, Y., Monteagudo, A., Neill, D. A., Núñez Vargas, P., Paiva, R., Peacock, J., Peñuela, M. C., Peña Cruz, A., Pitman, N., Priante Filho, N., Prieto, A., Ramírez, H., Rudas, A., Salomão, R., Santos, A. J. B., Schmerler, J., Silva, N., Silveira, M., Vásquez, R., Vieira, I., Terborgh, J., and Lloyd, J.: Basin-wide variations in Amazon forest structure and function are mediated by both soils and climate, Biogeosciences, 9, 2203–2246, https://doi.org/10.5194/bg-9-2203-2012, 2012.
R Core Team: R: a language and environment for statistical computing, R Foundation for Statistical Computing, Vienna, Austria, http://www.r-project.org (last access: 28 August 2021), 2019.
Ratter, J. A., Richards, P., Argent, G., and Gifford, D.: Observations on the vegetation of northeastern Mato Grosso: I. The woody vegetation types of the Xavantina-Cachimbo Expedition area, Philos. T. R. Soc. B, 266, 449–492, https://doi.org/10.1098/rstb.1973.0053, 1973.
Reich, P. and Schoettle, A.: Role of phosphorus and nitrogen in photosynthetic and whole plant carbon gain and nutrient use efficiency in eastern white pine, Oecologia, 77, 25–33, https://doi.org/10.1007/BF00380920, 1988.
Reich, P. B., Oleksyn, J., and Wright, I. J.: Leaf phosphorus influences the photosynthesis–nitrogen relation: a cross-biome analysis of 314 species, Oecologia, 160, 207–212, https://doi.org/10.1007/s00442-009-1291-3, 2009.
Resende, J. C. F., Markewitz, D., Klink, C. A., da Cunha Bustamante, M. M., and Davidson, E. A.: Phosphorus cycling in a small watershed in the Brazilian Cerrado: impacts of frequent burning, Biogeochemistry, 105, 105–118, https://doi.org/10.1007/s10533-010-9531-5, 2011.
Ribeiro, J. F. and Walter, B. M. T.: Fitofisionomias do bioma Cerrado, in: edited by: Sano, S. M. and de Almeida, S. P., Cerrado: ambiente e flora, EMBRAPA Cerrados, Planaltina, Brazil, 1998.
Rosan, T. M., Aragão, L. E., Oliveras, I., Phillips, O. L., Malhi, Y., Gloor, E., and Wagner, F. H.: Extensive 21st-century woody encroachment in South America's savanna, Geophys. Res. Lett., 46, 6594–6603, https://doi.org/10.1029/2019GL082327, 2019.
Scalon, M.: Dataset_Scalon et al_Biogeosci.csv, figshare [data set, code], https://doi.org/10.6084/m9.figshare.20387082.v2, 2022.
Schaller, J., Tischer, A., Struyf, E., Bremer, M., Belmonte, D. U., and Potthast, K.: Fire enhances phosphorus availability in topsoils depending on binding properties, Ecology, 96, 1598–1606, https://doi.org/10.1890/14-1311.1, 2015.
Silva, L. C., Hoffmann, W. A., Rossatto, D. R., Haridasan, M., Franco, A. C., and Horwath, W. R.: Can savannas become forests? A coupled analysis of nutrient stocks and fire thresholds in central Brazil, Plant Soil, 373, 829–842, https://doi.org/10.1007/s11104-013-1822-x, 2013.
Solbrig, O. T.: The diversity of the savanna ecosystem, in: Biodiversity and savanna ecosystem processes, edited by: Solbrig O. T., Medina J. F., and Silva, J. F., Springer, Berlin, Heidelberg, 1–27, https://doi.org/10.1007/978-3-642-78969-4, 1996.
Tsujii, Y., Aiba, S. i., and Kitayama, K.: Phosphorus allocation to and resorption from leaves regulate the residence time of phosphorus in above-ground forest biomass on Mount Kinabalu, Borneo, Funct. Ecol., 34, 1702–1712, https://doi.org/10.1111/1365-2435.13574, 2020.
Vergutz, L., Manzoni, S., Porporato, A., Novais, R. F., and Jackson, R. B.: Global resorption efficiencies and concentrations of carbon and nutrients in leaves of terrestrial plants, Ecol. Monogr., 82, 205–220, https://doi.org/10.1890/11-0416.1, 2012.
Viani, R. A., Rodrigues, R. R., Dawson, T. E., and Oliveira, R. S.: Savanna soil fertility limits growth but not survival of tropical forest tree seedlings, Plant Soil, 349, 341–353, https://doi.org/10.1007/s11104-011-0879-7, 2011.
Vitousek, P.: Nutrient cycling and nutrient use efficiency, Am. Nat., 114, 553–572, https://doi.org/10.1086/283931, 1982.
Vitousek, P. and Sanford R. Nutrient cycling in moist tropical forest, Annu. Rev. Ecol. Syst., 17, 137–167, https://doi.org/10.1146/annurev.es.17.110186.001033, 1986.
Vitousek, P. M.: Litterfall, nutrient cycling, and nutrient limitation in tropical forests, Ecology, 65, 285–298, https://doi.org/10.2307/1939481, 1984.
Wang, L., D'Odorico, P., O'Halloran, L. R., Caylor, K., and Macko, S.: Combined effects of soil moisture and nitrogen availability variations on grass productivity in African savannas, Plant Soil, 328, 95–108, https://doi.org/10.1007/s11104-009-0085-z, 2010.
Wardle, D. A., Bardgett, R. D., Klironomos, J. N., Setälä, H., Van Der Putten, W. H., and Wall, D. H.: Ecological linkages between aboveground and belowground biota, Science, 304, 1629–1633, https://doi.org/10.1126/science.1094875, 2004.
Wright, I. and Westoby, M.: Nutrient concentration, resorption and lifespan: leaf traits of Australian sclerophyll species, Funct. Ecol., 17, 10–19, https://doi.org/10.1046/j.1365-2435.2003.00694.x, 2003.