the Creative Commons Attribution 4.0 License.
the Creative Commons Attribution 4.0 License.
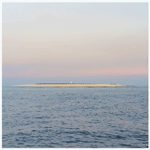
Production and accumulation of reef framework by calcifying corals and macroalgae on a remote Indian Ocean cay
M. James McLaughlin
Cindy Bessey
Gary A. Kendrick
John Keesing
Ylva S. Olsen
Coral reefs face increasing pressures in response to unprecedented rates of environmental change at present. The coral reef physical framework is formed through the production of calcium carbonate (CaCO3) and maintained by marine organisms, primarily hermatypic corals, and calcifying algae. The northern part of Western Australia, known as the Kimberley, has largely escaped land-based anthropogenic impacts and this study provides important metabolic data on reef-building organisms from an undisturbed set of marine habitats. From the reef platform of Browse Island, located on the mid-shelf just inside the 200 m isobath off the Kimberley coast, specimens of the dominant coral (six species) and algal (five species) taxa were collected and incubated ex situ in light and dark shipboard experimental mesocosms for 4 h to measure rates of calcification and production patterns of oxygen. During experimental light and dark incubations, all algae were net autotrophic producing 6 to 111 . In contrast, most corals were net consumers of O2 with average net fluxes ranging from −42 to 47 . The net change in pH was generally negative for corals and calcifying algae (−0.01 to −0.08 h−1). Resulting net calcification rates (1.9 to 9.9 ) for corals and calcifying algae (Halimeda and Galaxura) were all positive and were strongly correlated with net O2 production. In intertidal habitats around Browse Island, estimated relative contributions of coral and Halimeda to the reef production of CaCO3 were similar at around 600 to 840 . The low reef platform had very low coral cover of < 3 % which made a smaller contribution to calcification of ∼ 240 . Calcification on the subtidal reef slope was predominantly from corals, producing ∼ 1540 , twice that of Halimeda. These data provide the first measures of community metabolism from the offshore reef systems of the Kimberley. The relative contributions of the main reef builders, in these undisturbed areas, to net community metabolism and CaCO3 production is important to understand exclusively climate-driven negative effects on tropical reefs.
- Article
(3102 KB) - Full-text XML
- BibTeX
- EndNote
The functioning of healthy coral reefs, as some of the world's most biologically (Stuart-Smith et al., 2018) and structurally complex ecosystems (Hughes et al., 2017b), results in a number of ecosystem services. They provide coastal protection, with reef structures acting to dampen wind- and wave-driven surges (Perry et al., 2018) and support a diverse range of species that provide critically important resources (such as food) for coastal livelihoods (Hoegh-Guldberg et al., 2007). However, coastal coral reefs in the Anthropocene era have been degraded for more than a century by overfishing and pollution (Hughes et al., 2017b). With the current unprecedented rate of environmental change, coral reefs face growing pressures. These range from localised eutrophication (Hewitt et al., 2016) and sedimentation (Hughes et al., 2017a) to larger-scale recurrent weather events (marine heat waves; Moore et al., 2012) and rising atmospheric greenhouse gases (especially carbon dioxide, CO2; IPCC, 2014) that result in increasing ocean temperatures (due to atmospheric heat absorption) and ocean acidification (OA; Hoegh-Guldberg et al., 2007; Doney et al., 2009; Perry et al., 2018). Once thought to be protected by the very nature of their isolation, remote reefs are also now showing impacts of increasing stressors brought about by anthropogenic climate change (Hughes et al., 2017b).
As one of the most important determinants of overall reef function, the construction and maintenance of the calcium carbonate (CaCO3) reef structure (the accumulation of which requires the net production of calcium carbonate by resident taxa; Cornwall et al., 2021) is vital to the myriad of ecosystem services that coral reefs provide (Hoegh-Guldberg et al., 2007; Andersson and Gledhill, 2013; Moberg and Folke, 1999). The coral reef physical framework is formed and maintained through the production of calcium carbonate (CaCO3) by marine organisms, primarily hermatypic corals, crustose coralline algae (CCA), and other calcifying algae (Vecsei, 2004; Perry et al., 2008, 2012). Scleractinian corals are primary reef builders in tropical environments, producing CaCO3 through skeletal deposition. This net calcium carbonate production is a balance between gross production minus the loss due to physical, chemical, and biological erosion (Cornwall et al., 2021). The net calcium carbonate production and related potential vertical accretion of reefs are increasingly threatened by anthropogenic climate change (Perry et al., 2018). For scleractinian corals, one of the most significant consequences of OA is the decrease in the concentration of carbonate ions () (Kleypas and Yates, 2009). Projections suggest that future rates of coral reef community dissolution may exceed rates of CaCO3 production (calcification), with the majority of coral reefs unable to maintain positive net carbonate production globally by 2100 (i.e. net loss) (Cornwall et al., 2021; Silverman et al., 2009; Hoegh-Guldberg et al., 2007).
These global climate change pressures are causing shifts in the composition of coral reef species, and the urgent focus now is on identifying, quantifying and maintaining reef ecosystem function so that coral reefs can continue to persist and deliver ecosystem services into the future (Harborne et al., 2017). To do this, it is necessary to characterise reef health in terms of metabolism which includes calcification but also fundamental processes such as photosynthesis and respiration (Madin et al., 2016; Carlot et al., 2022). Photosynthesis fixes CO2 in organic materials, whereas the reverse reaction (dark respiration) releases it. In scleractinian corals with zooxanthellae, the precipitation of CaCO3 through calcification is tightly coupled to photosynthetic fixation of CO2 and on average tends to be 3 times higher in daylight conditions than in darkness (Gattuso et al., 1999). Calcification rates can increase further through feeding on phytoplankton and suspended particles (Houlbreque and Ferrier-Pages, 2009). Overall, the excess organic production in a coral reef community (i.e. the difference between gross primary production and dark respiration) acts as a CO2 sink, while calcification acts as a source of CO2 (Lewis, 1977; Kinsey, 1985). Most reef flats are sources of CO2 to the atmosphere despite the drawdown of CO2 during the day via photosynthetic processes. This is due to their low net fixation of CO2 and rather large release of CO2 by precipitation of calcium carbonate (Ware et al., 1992; Gattuso et al., 1993, 1995, 1996b; Smith, 1995; Frankignoulle et al., 1996).
One notable exception to this is in algal-dominated reef communities, which are sinks for atmospheric CO2. They exhibit larger excess community production and/or a lower community calcification, (e.g. Kayanne et al., 1995; Gattuso et al., 1996a, 1997). The morphological diversity of reef algae provides food (Overholtzer and Motta, 1999), habitat and shelter (Price et al., 2011) for a number of invertebrate and fish species, with productivity sustaining higher trophic levels. Calcified macroalgae can also contribute significantly to the deposition of carbonates (Nelson, 2009). In particular, species of the genus Halimeda are widely distributed across tropical and subtropical environments and contribute significantly to reef calcification and productivity rates because of their fast growth and rapid turnover rates (Vroom et al., 2003; Smith et al., 2004; Nelson, 2009) compared to corals or coralline red algae (CRA). Calcification rates of Halimeda make it a major contributor to CaCO3 in reefs in the Caribbean (Blair and Norris, 1988; Nelson, 2009), Tahiti and the Great Barrier Reef (Drew, 1983; Payri, 1988). In certain locations, precipitation of calcium carbonate can approach 2.9 , positioning Halimeda as a major contributor to carbonate budgets within shallow waters around the globe (Price et al., 2011). This group further occupies a diverse range of environments (mangroves, seagrass beds, and coral reefs) and can produce structurally complex mounds that serve as critical habitat for a diversity of important marine life (Rees et al., 2007).
Here, we compare metabolic and calcification rates of the dominant intertidal taxa of coral and macroalgae at Browse Island, a small island in the Kimberley bioregion located in the northern part of Western Australia. Unlike southwestern Australia, which has one of the fastest increasing rates of change from cumulative human impacts (Halpern et al., 2019), the Kimberley represents one of the few “very low impact” tropical coast and shelf areas globally – only 3.7 % of the global oceans fall in this category (Halpern et al., 2008). Few process studies have been carried out in the region due to the remoteness of these reef habitats, some of which are located hundreds of kilometres from the coastline, meaning that fieldwork and data acquisition can be difficult and costly. Rates of metabolism and calcification were determined in on-ship incubations in October 2016, April 2017 and October 2017. Using the proportional cover of the dominant benthic community, these rates were upscaled to gain whole of community metabolism estimates for the Browse Island habitats and provide new insights into reef ecosystem health and functioning in the absence of localised land- and sea-based anthropogenic variables (Harley et al., 2006; 157 Schindler, 2006; Walther, 2010).
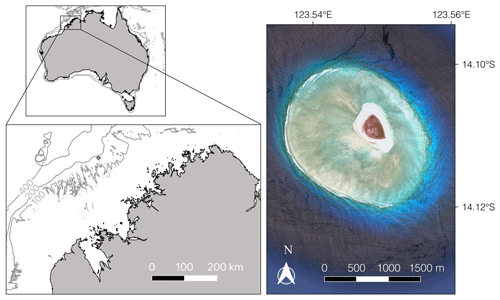
Figure 1The study site, Browse Island (diamond, bottom left map), is located just inside the 200 m isobath on the continental shelf. The small map (top left) shows the location of the island relative to the Australian coastline with the 100, 200 and 400 m isobaths marked in grey. The satellite image (right; © Google Earth, 2018) shows the extent of the reef.
2.1 Study site
Browse Island is located on the mid-shelf just inside the 200 m isobath off the Kimberley coast in the northern part of Western Australia (14∘6′ S, 123∘32′ E; Fig. 1). The island is surrounded by a small (∼ 4.5 km2) planar platform reef consisting of a shallow lagoon, an extensive reef flat that is conspicuously absent to the northeast of the island, and a well-defined reef crest and slope. Tides are semidiurnal with a maximum range of < 5 m, exposing the reef crest and reef platform habitats during low tides. The intertidal habitats are characterised by low species richness and dominated by small turfing algae and calcified macroalgae of the genus Halimeda (15 %–22 % and 6 %–9 % cover, respectively) (Olsen et al., 2017). Coral assemblages are well developed with cover of 5 %–8 % in the intertidal habitats and 18 % on the shallow reef slope (< 10 m) (Olsen et al., 2017).
2.2 Algae and coral collection
Specimens of the dominant coral and algal taxa were collected from the reef platform by hand during low tide; it was brought back to the vessel immediately and kept in a holding tank with circulating seawater. Macroalgae included the calcifying green alga Halimeda opuntia, which was the dominant species of Halimeda on the reef platform, the green alga Caulerpa sp., and the calcifying red alga Galaxaura sp. Pieces of turf algae (turf) as well as turf attached to a piece of rock (turf + substrate) were measured. In April 2016, drift algae of the genus Sargassum found floating on the water surface were also included, although this taxon was not found growing anywhere on the reef during sampling trips. Hermatypic corals included Pocillopora sp., Goniastrea sp., Porites sp., Heliopora sp., Acropora sp. and Seriatopora sp. Whole pieces of coral small enough to fit inside the incubation cores (inner diameter ∼ 90 mm) were collected to minimise tissue damage. All coral samples were > 50 mm diameter and therefore operationally defined as adults and estimated to be at least 2–7 years old, depending on the taxa (Trapon et al., 2013).
2.3 Light and dark incubations
Light and dark incubations were undertaken on the back deck of the research vessel. Four 60 L holding tanks were placed in a shade-free spot under natural light conditions, filled with seawater and connected to a flow-through seawater system driven by an Ozito PSDW-350 W dirty water submersible water pump with a maximum flow rate of 7000 L h−1, which ensured that the setup remained at ambient temperature (Fig. 2). The intensity of photosynthetically active radiation (PAR) was recorded for each set of incubations with a HOBO Micro Station logger (H21-002, Onset) placed inside one of the tanks. Six 1.56 L clear Perspex incubation cores (24 in total per incubation) fitted with stirring caps were placed in each holding tank and spaced evenly apart to minimise shading (Fig. 2).
Table 1Taxa measured in on-ship incubation experiments including the number of replicate specimens measured (one specimen per incubation core). Some of the specimens were not included in the final analysis due to sampling errors or due to O2 not increasing during both of the light intervals or not decreasing during both of the dark intervals; the resulting number of specimens used are shown in brackets.
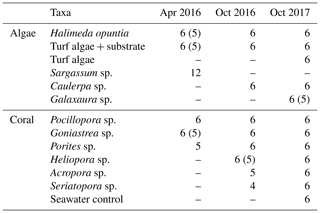
Depending upon abundance, individual specimens of algae and coral were placed in 6–12 replicate incubation cores per taxa, except where not enough individuals could be found. Table 1 shows the taxa incubated during each sampling trip and the number of replicates. Water samples from the holding tanks were measured at each time point as controls and, additionally, in October 2017, a separate seawater control (6 replicate incubation cores with seawater) was included. After a period of acclimation (1–2 h), incubations were run over a 4 h period. The light incubations were conducted while the sun was at its zenith providing full irradiance to the samples. After 2 h, the tubs were covered with a black lid ensuring no light could enter and the samples incubated for 2 h in the dark.
To estimate oxygen production or consumption during the incubations, a 40 mL water sample was extracted from each of the 24 cores and the 4 tubs at the start of the incubations and hourly thereafter. A port in the cap of each core allowed for sample collection using a syringe. As the sample was removed, the same volume of liquid was automatically replaced from the flow-through tank into the core so that the core volume remained constant throughout the experiment. Samples were immediately analysed for temperature and dissolved oxygen (O2) using a YSI 5100 bench-top oxygen and temperature meter with YSI 5010 BOD stirring probe, calibrated daily in air. Sample pH was determined using a TPS Aqua pH meter with an Ionode probe, calibrated daily with pH 7.00 and 10.00 buffers. A second 35 mL water sample was collected from each core and tub and split between one 10 mL glass vacutainer for alkalinity and duplicate 10 mL sterile vials for nutrient analyses. Nutrient samples were immediately frozen and alkalinity samples were stored in a cool and dark environment. At the end of the incubation, algal and coral specimens were frozen. All samples were transported to Perth, Western Australia, to be analysed.
2.4 Surface areas of coral and algal specimens
Metabolic measurements were standardised by surface area of the incubated specimens since this represents the area available for photosynthesis and nutrient uptake. The surface area of specimens of coral, Halimeda and turf + substrate were estimated using a single wax dipping method (Veal et al., 2010). Specimens were dried, weighed and then dipped in paraffin wax at 65 ∘C. The waxed samples were weighed again, and the weight of the wax calculated. The surface area was estimated from the wax weights against a calibration curve constructed by wax dipping geometric wooden objects of known size. The surface areas of the remaining taxa were estimated from photographs in ImageJ (Rueden et al., 2017). The “footprint” of each sample, i.e. the surface area of reef occupied by the organism, was also estimated by tracing the outline of the specimen photographed from straight above in ImageJ.
2.5 Chemical analyses
Concentrations of nitrate + nitrite (hereafter referred to as nitrate), ammonium, phosphate and dissolved silica in water samples were analysed in duplicate by flow-injection analysis (Lachat QuickChem 8000) with detection by absorbance at specific wavelengths for silica (QuikChem Method 31-114-27-1-D), nitrate (Quikchem Method 31-107-04-1-A) and phosphate (QuikChem Method 31-115-01-1-G), and by fluorescence for ammonia according to Watson et al. (2005). Detection limits were 0.02 µmol L−1 for all inorganic nutrient species, with a standard error of < 0.7 %.
From SOP3b in Dickson et al. (2007), total alkalinity was determined for single replicates to the nearest 5 µmol L−1 equivalent (hereafter referred to as µmol L−1) using an open-cell Metrohm titrator (841 Titrando, Burette: 800 Dosino 10 mL) with a Metrohm micro-glass pH probe calibrated with Certipur buffer solutions at pH 2.00, 4.01, 7.00 and 10.00 (at 25.0 ∘C). Samples were kept in a Jubalo F12 temperature-controlled water bath prior to decanting a 10 mL aliquot of sample into a vessel with a water jacket maintaining temperature at 25.0 ∘C. Samples were titrated with 0.012 N HCl and standardised against sodium carbonate (99.95 wt % to 100.05 wt %) with an initial volume of titrant added to reach pH 3.5. Titrations were run to an endpoint of pH 3 with a Gran plot (Excel macro) to determine the total alkalinity endpoint near pH 4.2. Carbonate system parameters were calculated from pH (measured during the incubations) and total alkalinity using the package “seacarb” (Gattuso et al., 2018) in R (R Core Team, 2018). Alkalinity and carbonate parameters were not determined in April 2016.
2.6 Oxygen fluxes and calcification rate calculations
The changes in O2 concentrations during light and dark incubations were expressed as millimoles per day assuming stable hourly production rates over 24 h. Any replicates where O2 did not increase during both of the light intervals or did not decrease during both of the dark intervals were excluded from further analysis. Net fluxes of O2 per day () were calculated for each sample, assuming a photoperiod of 12 h. Calcification rates of corals and calcifying algae (H. opuntia and Galaxaura sp.) were estimated using the alkalinity anomaly method (Smith and Key, 1975), uncorrected for changes in nutrient concentration (Chisholm and Gattuso, 1991), where precipitation of 1 mol of CaCO3 leads to the reduction of total alkalinity by 2 mol equivalents. Rates per surface area () were obtained by dividing these values by the surface area of each specimen.
A census-based approach was used to estimate the amount of CaCO3 and O2 produced by a single taxon per unit area of reef surface per year (Shaw et al., 2016). The rates of calcification and net O2 production per day were divided by the “footprint” area of each specimen. To estimate the relative contributions from each taxon to community production per square metre of reef, these rates were multiplied by the relative percent cover in each of the major habitats. Estimates of percent cover based on drop-camera image analysis were obtained from Olsen et al. (2017). The productivity rates for individual coral species were combined into one value for coral.
2.7 Statistical analyses
The relationships between net changes in pH and O2 and between net O2 production and net calcification (in light and dark incubations) were examined by linear regression. Significance of regressions were calculated for algae, calcified algae and corals; the 95 % confidence intervals were calculated for the slope of each line in R (R Core Team, 2018). Regressions were examined with ANOVA and deemed significant if p < 0.05.
Table 2Ambient concentrations of parameters measured during incubations (mean ± SE): nutrients ( + = nitrate + nitrite, = ammonium, = orthophosphate, Si = silica) and oxygen (O2), total alkalinity (TAlk), photosynthetically active radiation (PAR), temperature (T) and salinity. Calculated carbonate system parameters (mean ± SE): CO2 partial pressure (pCO2), concentrations of , and dissolved inorganic carbon (DIC) and the saturation state of aragonite (Ω Aragonite). In April 2016, two replicate PAR measurements were taken at 11:00, 12:00 and 13:00 AWST (Australian Western Standard Time). In October 2016 and 2017, PAR was measured every minute and values between 11:00 and 13:00 averaged.
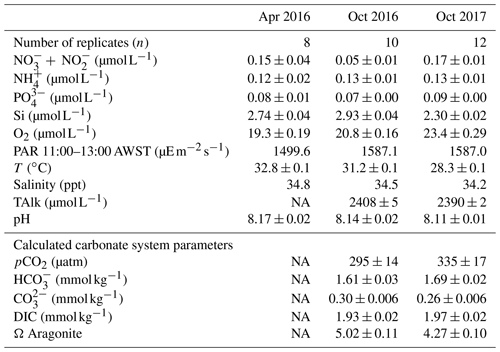
NA – not available.
3.1 Experimental conditions
Nutrient concentrations were low and similar among sampling trips (Table 2), as is characteristic of the tropical eastern Indian Ocean offshore waters (McLaughlin et al., 2019). Concentrations were as follows: nitrate – 0.05 to 0.17 µmol L−1, ammonium – 0.12 to 0.13 µmol L−1, phosphate – 0.07 to 0.1 µmol L−1 and silicate – 2.3 to 3 µmol L−1. Oxygen was 0.19 to 0.22 mmol L−1 and salinity was 34.2 to 34.8 ppt. Light and temperature conditions in the incubations were representative of in situ conditions on the reef platform and were similar among trips. The PAR levels were 1500 in April and slightly higher to 1587 in October. Temperatures were 28.3 to 32.8 ∘C and highest in April. Carbonate system parameters were not obtained for April 2016 due to instrument error, and some minor differences in pCO2, , , DIC and Ω Aragonite were noted between October 2016 and 2017 (Table 2). Alkalinity and pH were both higher in 2016, and there were associated minor differences in the concentrations of the carbonate species and the aragonite saturation state (Table 2).
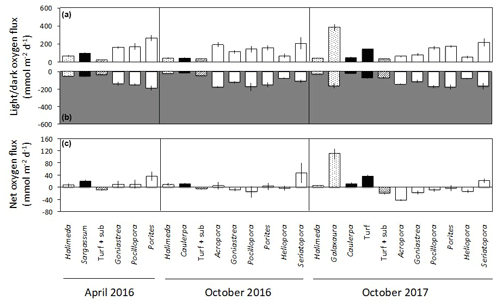
Figure 3Net changes in oxygen (mean ± SE) in light (a) and dark (b) incubations of calcifying algae (stippled), macroalgae and turf (black), turf + substrate (diagonal stripes) and coral (white) standardised by specimen surface area. Panel (c) shows the net daily production of oxygen (mean ± SE), assuming a 12 h photoperiod and stable rates of photosynthesis and dark respiration over a 24 h period.
3.2 Changes in oxygen and pH
Changes in dissolved O2 differed among taxa and between light and dark incubations. In the seawater controls, O2 changed by < 0.01 mmol h−1 in both light and dark incubations, showing that the contribution of any organisms in the seawater itself to O2 production and dark respiration was minimal. No corrections were therefore applied. In the light incubations, O2 productivity fluxes were positive for all taxa (Fig. 3a). The highest light flux of O2 of ∼ 380 was measured for Galaxaura in October 2017 (Fig. 3a). Corals generally produced 100 to 260 in the light, except Heliopora, which had a flux of 50 to 80 . All taxa consumed O2 during the dark incubations when changes in O2 are due to dark respiration, with mean fluxes of −15 to −190 (Fig. 3b). All algae were net autotrophic and produced 6 to 111 with the highest net O2 flux measured for Galaxaura and turf at 111 and 36 , respectively (Fig. 3c). In contrast, around half of the corals were net consumers of O2 and average net fluxes spanned a wide range from −42 to 47 .
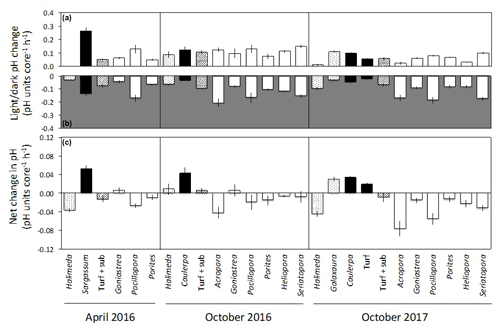
Figure 4Net changes in pH per hour for each 1.56 L incubation core (mean ± SE) in light (a) and dark (b) incubations of calcifying algae (stippled), macroalgae and turf (black), turf + substrate (diagonal stripes) and coral (white). Panel (c) shows the net change in pH per hour (mean ± SE), assuming equal periods of light and darkness.
In the light incubations, pH generally increased by 0.03 to 0.25 h−1 for all taxa, except for Halimeda in April 2016 and October 2017, which showed no change or a very small increase (Fig. 4a). In dark incubations, mean pH decreased for all taxa by 0.02 to 0.21 h−1, indicative of a net increase in CO2 through dark respiration (Fig. 4b). Non-calcifying algae (Sargassum, Caulerpa and turf) raised net pH by 0.02 to 0.05 h−1 (assuming equal periods of light and darkness) (Fig. 4c). The net change in pH was generally negative for corals and calcifying algae (−0.01 to −0.08 h−1), except for the coral Goniastrea in April and October 2016 (0.01 h−1) and the calcifying alga Galaxaura (0.03 h−1; Fig. 4c).
Net changes in pH are largely driven by metabolic uptake and release of CO2. We found positive relationships between changes in pH and net production or consumption of O2, except in seawater controls where changes in O2 and pH were minor (Fig. 5). The relationships for algae, calcifying algae and coral were all significant but had relatively low adjusted r2 values of 0.59, 0.46 and 0.19, respectively, suggesting significant variability among species and individuals within each of these groups.
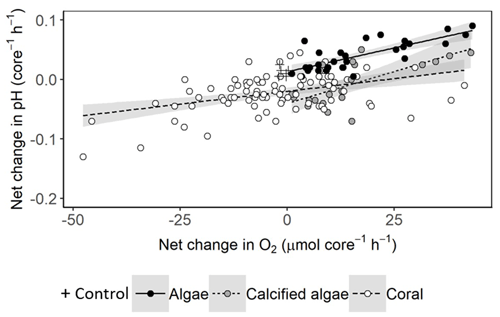
Figure 5Net change in pH versus O2 per 1.56 L incubation core, assuming equal periods of light and darkness. Linear relationships are fitted with 95 % confidence intervals shown in grey. For algae, net change in pH = 0.13 + 0.0016 × net change in O2 (ANOVA: F1,27 = 41.15, p < 0.001). For calcified algae, net change in pH = −0.04 + 0.0021 × net change in O2 (ANOVA: F1,19 = 17.86, p < 0.001). For corals, net change in pH = −0.02 + 0.00086 × net change in O2 (ANOVA: F1,82 = 18.88, p < 0.001).
3.3 Calcification rates
Corals, Halimeda and Galaxaura had positive calcification rates in light ranging from 4.2 to 18.4 (Fig. 6a). In the dark, calcifying rates were smaller and just under half of the rates were negative, suggesting dissolution of CaCO3 (Fig. 6b). The resulting net calcification rates (based on equal periods of light and dark – monthly average sunrise and sunset at Browse Island of 05:52 and 17:39 AWST for April, and 05:19 and 17:54 AWST for October; WillyWeather, 2022) were all positive and ranged from 1.9 to 9.9 (Fig. 6c). Rates of calcification were strongly and linearly correlated with net O2 production and were significantly higher in light than in darkness for both corals and algae (Fig. 7).
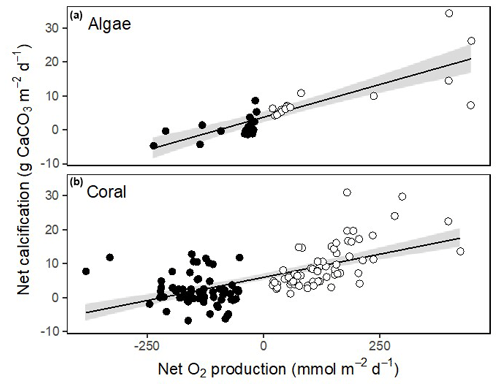
Figure 7Relationship between net calcification rate and net productivity for calcifying algae (a) and corals (b). Open circles indicate rates measured in light and closed circles show rates measured in dark. Linear fits are shown with 95 % confidence intervals in grey. For calcified algae, net calcification = 3.6 + 0.039 × net O2 production (ANOVA: F1,32 = 67.0, p < 0.001). For corals, net calcification = 5.99 + 0.027 × net O2 production (ANOVA: F1,126 = 82.2, p < 0.001).
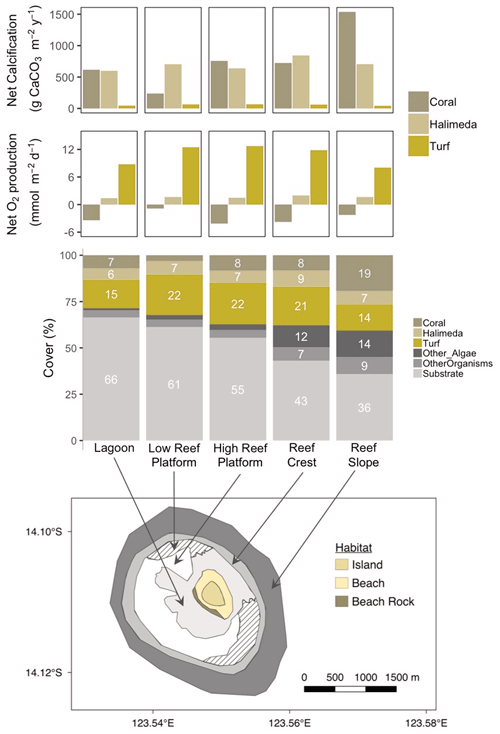
Figure 8Map of the reef around Browse Island showing the major habitat types (bottom panel). Reef surface percent cover of coral, Halimeda, turf and other categories in each habitat (middle panel) based on drop-camera image analysis data from Olsen et al. (2017). Net calcification and net oxygen production by coral, Halimeda and turf per square metre of reef (top two panels) scaled up by multiplying rates obtained from incubations of each taxon by the percent cover in each habitat.
3.4 Contributions to community production
In intertidal habitats (lagoon and high reef platform) around Browse Island, the estimated relative contributions of coral (8 % cover) and Halimeda (7 % cover) to the reef production of CaCO3 were similar, around 600 to 840 (Fig. 8, top panel). The low reef platform had very low coral cover of < 3 % (Fig. 8, middle), which therefore made a smaller contribution to calcification of ∼ 240 in this habitat (Fig. 8, top). In contrast, calcification on the subtidal reef slope was predominantly from corals (19 % cover) which produced ∼ 1540 , around twice the amount compared to Halimeda (7 % cover). Galaxaura, which had high measured rates of productivity and calcification, was extremely rare (0.02 % total cover found only in October 2017; Olsen et al., 2017) and thus its contribution to community calcification and productivity were negligible. Turf was responsible for the majority of the O2 production in all habitats and produced an estimated 8 to 13 compared to < 2 for Halimeda and −4 to −1 for corals (Fig. 8, second panel from top).
Mesocosm experiments have shown that reef-building (hermatypic) corals tend to reduce pH and consume O2 (e.g. Gattuso et al., 1999; Smith et al., 2013), whereas calcifying macroalgae increase pH and O2 during daytime (Borowitzka and Larkum 1987; Smith et al., 2013). Both corals and calcifying macroalgae reduce pH and O2 concentrations due to respiration during nighttime, but the rates of change differ among species (Smith et al., 2013). The organisms investigated in the present study showed typical patterns of O2 production in daylight and consumption in darkness, similar to other island reef systems as a result of photosynthesis and dark respiration, but the metabolic measurements showed clear differences among taxonomic groups. Algae had higher positive net O2 fluxes with rates of 18 to 350 , of which the red calcifying alga Galaxaura sp. had the highest rate of net productivity by far. For corals, the relatively high O2 increase measured in daylight was coupled with high rates of respiration in darkness, creating a negligible or negative net O2 production for most species, except Porites sp. in April 2016 and Seriatopora sp. in October 2016 and 2017, which were net positive. Although autotrophic, our data indicate that the majority of the corals we studied utilise heterotrophic supply through feeding to help sustain growth in addition to photosynthesis by zooxanthellae (Houlbreque and Ferrier-Pages, 2009). These patterns are generally in agreement with those reported elsewhere. For example, fleshy and calcifying algae showed net diel O2 production, whereas corals generally consumed O2, i.e. were net heterotrophic, on islands in the South Pacific (Porites sp.) and the Caribbean (Madracis sp.) (Smith et al., 2013).
Concurrent with changes in O2 were changes in seawater pH, where pH increased in daylight (except for Halimeda in April 2016 where no change was measured) and decreased in darkness. The effects of metabolic activity on bulk pH (uptake and release of CO2 through photosynthesis and dark respiration) cannot be directly separated from that of calcification, which is associated with the release of H+ ions thereby decreasing pH (Jokiel, 2011). However, differences were observed in the net pH change in incubations between calcifiers and non-calcifiers. The net effect of non-calcifiers on seawater pH was positive, while the majority of calcifiers caused net pH to decline. In the present study, Halimeda (April 2016) and Goniastrea (April and October 2016) caused relatively minor increases in pH, whereas the calcifying alga Galaxaura elevated pH by, on average, 0.03 units, comparable to the net effect of non-calcifiers. This is not surprising given the high rate of O2 production measured for Galaxaura, which is associated with sufficient levels of CO2 fixation to compensate for the reduction in pH associated with calcification in this species. A strong link was observed between metabolism and pH in all taxa, demonstrated as linear relationships between changes in pH and O2 during the incubations. Previous research by Smith et al. (2013) identified two broad patterns: metabolic changes in O2 in non-calcifiers (fleshy and turf algae) linked to large changes in pH (steep slopes), and metabolic changes in O2 in calcifying organisms (Porites sp. Madracis sp. and Halimeda sp.) producing little or no change in pH (shallow slopes). This is contrary to the present study's observations where pH and O2 relationship gradients were similar for calcifiers and non-calcifiers. Non-calcifying organisms were found to consistently have a net positive effect on both pH and O2. Change in pH for the same net change in O2 was elevated for non-calcifiers compared to calcifiers.
Production and accumulation of reef framework carbonate is controlled by the relative rates of, and the interactions between, a range of ecologically, physically and chemically driven production and erosion processes (Perry et al., 2008; Montaggioni and Braithwaite, 2009), with the relative importance of different taxa for CaCO3 production differing among reefs and among habitats within reefs. Coral growth can be measured in several ways: linear extension rate, global skeletal growth and calcification rate (measured using the alkalinity technique or by 45Ca incorporation) (Houlbreque and Ferrier-Pages, 2009). Methods to calculate calcification can vary in accuracy. Overestimates of calcification rates can result from calculations based on changes in alkalinity, while those relying on CaCO3 content and growth measurements (either through staining or tagging segments), may produce minimum estimates as loss of new tissue is not accounted for (Hart and Kench, 2007; Houlbreque and Ferrier-Pages, 2009). The alkalinity method employed in the present study was the best possible option when working in a remote location where actual growth rates cannot be easily assessed, or use of radioisotopes was limited. Rates of net community calcification for reef flats worldwide range from 7.3 to 90 mol (730 to 9000 g) with an average of 47 mol (4700 g) (Atkinson, 2011). The patterns found in the present study – higher calcification rates in daylight compared to the rates in darkness for all corals and calcifying algae – are typical. However, the coral CaCO3 production rates per reef area (7 % to 8 % cover low reef platform, 19 % reef slope) measured here (240 for low reef platform, 610 to 756 in the other intertidal habitats, and 1536 on the reef slope) were somewhat lower than values reported elsewhere. In 2016, the dark rates of calcification by corals were less than 50 % of the rates in light, with some (Porites and Heliopora) being negative. Dark rates of calcification in 2017 were negative or near zero for all species except Porites, Pocillopora and Seriatopora. Houlbreque et al. (2004) showed that coral feeding enhances dark calcification rates in scleractinian corals, but incubations in our study were done in the absence of supplemental feeding. The trend observed here may be due to some dissolution of CaCO3 due to the reduced pH during dark incubations or it could be an artefact of the experimental conditions. This result should therefore be taken with some caution, in particular for Porites in October 2016, which saw the largest decrease (Fig. 5, middle panel). However, the resulting strong relationship between net carbonate production and net carbonate consumption is consistent with previous studies, both in situ and in mesocosms (Albright et al., 2013).
Corals are typically the primary framework-producing components on a tropical reef and dominate carbonate production per unit area (Vecsei, 2004). However, additional CaCO3 is produced by calcareous crustose coralline algae (CCA) and calcareous algae of the genus Halimeda, (e.g. Payri, 1988). Sprawling lithophytic species of Halimeda, like the majority of the Halimeda around Browse Island, tend to be fast growing and have high calcification rates (Hart and Kench, 2007). Rates of calcification per area of 100 % Halimeda cover have been estimated from 400 to 1667 (in Hart and Kench, 2007, their Supplement). In other locations, Halimeda has been estimated to contribute around 1100 to 2400 to benthic carbonate production (Drew, 1983; Freile et al., 1995; Hudson, 1985; Kangwe et al., 2012; Payri, 1988; Rees et al., 2007), which is higher than the 600 to 840 estimated for H. opuntia in the intertidal habitats in the present study. These rates depend on both the intrinsic calcification rates and the abundance or cover of algae (6.1 % to 8.7 % cover on Browse Island, which corresponds to ∼ 150 to 250 g dw m−2).
Nutrient capacity is one important driver of productivity in many reef ecosystems. The rate at which nutrients are recycled between the constituents of the system (the ambient nutrient availability, and the nutrients stored within plant and animal biomass) depends on input from a variety of sources (e.g. associated with seasonal rains or upwelling) (DeAngelis, 1992; Hatcher, 1990). Coral reefs typically have low ambient nutrient availability and receive little sustained exogenous nutrient input (Hatcher, 1990; Szmant, 2002), thus the high rates of production found within these ecosystems are largely attributed to the nutrients stored and cycled by living biomass (Pomeroy, 1974; DeAngelis et al., 1989; Sorokin, 1995). Fishes typically make up a substantial component of living biomass on coral reefs and represent an important reservoir of nutrients in these ecosystems (Allgeier et al., 2014). Contrary to our expectations given its remote location in an area of apparently low anthropogenic impacts, the reef platform around Browse Island was depauperate with a conspicuous lack of diversity in key groups including macroalgae, macroinvertebrates and teleost browsers (Bessey et al., 2020). McLaughlin et al. (2019) found surface water standing-stock nutrient concentrations to be low along the Kimberley shelf. Conditions at Browse Island were similar with low water column nutrients for nitrate, ammonia and phosphate during all trips. Understanding how changes in animal populations alter nutrient dynamics on large ecological scales is a relatively recent endeavour (Doughty et al., 2015). Allgeier et al. (2016) showed that targeted fishing of higher trophic levels reduces the capacity of coral reef fish communities to store and recycle nutrients by nearly half. Fish-mediated nutrients enhance coral growth (Meyer et al., 1983) and primary production (Allgeier et al., 2013) and may regulate nutrient ratios at the ecosystem scale (Allgeier et al., 2014).
The Kimberley region-wide averages of coral cover and macroalgal cover are 23.8 % and 7.1 % (Richards et al., 2015), respectively. However, this relationship at Browse Island is reversed, with macroalgae more dominant at 28 % total cover to that of coral at 9 % total cover. On the Browse Island reef platform, the same pattern is observed where averages were 5 % to 8 % for coral and 32 % for macroalgae, differing from those of the regional averages of 14.4 % and 15.5 % of coral and macroalgae, respectively (Richards et al., 2015). While the estimates provided here approximate the relative contributions of Halimeda and coral to CaCO3 production, they do not add up to a whole system budget. There are other organisms likely to contribute significantly. For example, the present study did not measure metabolic or calcification rates of encrusting coralline algae, which, although making up a modest 1.0 % to 3.0 % of the benthic cover in the lagoon and reef platform habitats at Browse Island, become more prominent at 11.8 % to 14.1 % on the reef crest and slope (Ylva S. Olsen, unpublished data). To calculate the true CaCO3 production per area of reef, the calcification rate would need to be multiplied by the benthic cover of coralline algae and the square of the benthic rugosity (Eakin, 1996). Using typical values for rugosity from Eakin (1996) of 1 to 1.4 for the lagoon and reef platform and 1.7 to 2 for the reef crest and slope, and assuming a typical calcification rate of 1500 to 2500 (for 100 % flat-surface cover) (Hart and Kench, 2007), the contribution of encrusting coralline algae to calcification in the lagoon and reef platform would be minor at 70 to 134 . However, they could produce a significant amount of 980 to 1360 on the reef crest and slope, which is somewhere in between the production rates estimated for Halimeda and corals. Encrusting coralline algae may therefore contribute significantly to the CaCO3 budget at Browse Island, at least in deeper habitats. These values are similar to those measured elsewhere, for example 870 to 3770 at Uva reef in the eastern Pacific Ocean (Eakin, 1996).
Metabolic rates of primary producers are clearly influenced by a multitude of factors including hydrodynamics, irradiance, and nutrient availability (Smith et al., 2013). We were able to detect considerable diurnal changes in water chemistry due to metabolic rates since our experiments were conducted in small enclosed mesocosms. The effect of metabolism on water chemistry is expected to dissipate downstream in a more turbulent or dynamic environment (Anthony et al. 2011). However, coral and algae metabolic rates and resultant flux from the diffusive boundary layer also increases with flow rates (Carpenter et al., 1991; Lesser et al., 1994; Bruno and Edmunds, 1998; Mass et al., 2010). Because our experiments were conducted in near no-flow chambers (mesocosm water was replenished with fresh seawater in small amounts during sample extraction), our measurements are conservative values and likely represent the lower range of potential effects that these reef organisms have on surrounding water chemistry. However, where residence times can be extended, particularly when trapping of water on the reef at low tides occurs, our results are likely reflective of how these benthic organisms affect water chemistry in the lagoonal habitats of Browse Island.
This study investigated the metabolism of coral and algae on the reef of remote Browse Island, found on the mid-shelf region of the Kimberley in Western Australia. Due to its remoteness, Browse Island presented a unique opportunity to observe these organisms in a pristine habitat where direct anthropogenic pressures are minimal. Browse Island is the only emergent mid-shelf reef in the Kimberley bioregion having semidiurnal tides that reach a maximum range of 5 m (Olsen et al., 2017) which is half the magnitude of tides experienced by reefs closer to the coast (McLaughlin et al., 2019). Its benthic structure is very different from both the Kimberley inner (e.g. Montgomery Reef, Adele and Cassini Islands) and outer (e.g. Ashmore Reef and Rowley Shoals) shelf reefs. The relative contributions of algae and corals to reef productivity are likely to differ across the Kimberley shelf, with corals becoming more important in offshore waters and algal calcifiers being important on the mid-shelf. Estimated areal production rates did not take into account the relief (differences in height from place to place on the reef surface) of the substrate. The reef platform surrounding Browse Island has relatively low surface relief, whereas the reef slope and crest have high rugosity, which means production rates in the latter environments may be underestimated. Despite these limitations, the rates estimated in this study are similar to those measured elsewhere.
The higher cover of Halimeda and the low coral cover at Browse Island compared to other reefs in the region mean that corals and Halimeda contribute equally to productivity rates of CaCO3 on the Browse Island reef flat. However, their relative contributions to the reef framework and sedimentary budget of the reef is unknown. To gain an understanding of the relationships between carbonate production and sinks on the reef, further study into the types and amounts of CaCO3 material found in each reef sink is necessary. The Kimberley coastal shelf, which is characterised by coral reef environments with clear, low nutrient waters and low productivity, has largely escaped land-based anthropogenic impacts but has been negatively affected by climate-driven coral bleaching and mortality, for example from heat waves at Scott Reef in 1998 and 2016 (Smith et al., 2008; Gilmour et al., 2013; Hughes et al., 2017b) and Ashmore Reef in 2003 and 2010 (Ceccarelli et al., 2011; Heyward, 2011).
There is lack of sufficient observations of pCO2, nutrients and research on the upper ocean carbon cycle from the Indian Ocean (Sreeush et al., 2020), which are critical to modelling of ocean acidification in the region (Panchang and Ambokar, 2021). The uptake of carbon dioxide by the ocean alters the composition of seawater chemistry with elevated partial pressures of carbon dioxide (pCO2), causing seawater pH and the CaCO3 saturation state to decrease (Feely et al., 2004). Ocean acidification directly threatens crucial trophic levels of the marine ecosystem. Baseline reef measurements in undisturbed areas like Browse Island are important to understand exclusively climate-driven stressors in lieu of local anthropogenic pressures normally associated with coastal tropical reefs. The effects of temperature stressors on reef communities and their productivity remain to be investigated in this region. Different components of the reef around Browse Island are likely to have different vulnerabilities to warming and heat waves. Future environmental stressors leading to changes in benthic community composition, structure and subsequent changes in reef productivity and in rates of production of CaCO3 could have major implications for Browse Island.
All code used in this paper is freely available for use in RStudio:
-
ggplot2, https://ggplot2.tidyverse.org (Wickham, 2016);
-
plyr, https://doi.org/10.18637/jss.v040.i01 (Wickham, 2011);
-
MASS, https://www.stats.ox.ac.uk/pub/MASS4/ (Venables and Ripley, 2002);
-
Lattice, http://lmdvr.r-forge.r-project.org (Sarkar, 2008);
-
gridExtra, https://cran.r-project.org/web/packages/gridExtra/index.html (Auguie and Antonov, 2017);
-
seacarb, https://cran.r-project.org/web/packages/seacarb/index.html (Gattuso et al., 2018).
Data can be accessed through https://www.shell.com.au/sustainability/environment/_jcr_content/par/toptasks_b64e.stream/1536897804284/e4d8489abff3f2bc193cc7bda779d6eeda0e59ab/arp7-2milestone-2017-report-2.pdf (Olsen et al., 2017).
MJM: conceptualisation, formal analysis, investigation, resources, methodology, visualisation, and writing (original draft preparation); CB: investigation, resources, project administration, and writing (review and editing); GAK: conceptualisation, funding acquisition, project administration, supervision, and writing (review and editing); JK: conceptualisation, funding acquisition, investigation, resources, supervision, and writing (review and editing); YSO: conceptualisation, formal analysis, investigation, project administration, resources, methodology, visualisation, and writing (original draft preparation).
The contact author has declared that none of the authors has any competing interests.
Publisher's note: Copernicus Publications remains neutral with regard to jurisdictional claims in published maps and institutional affiliations.
The authors thank Max Rees, Mark Tonks and Peter Hughes for their support of this work, the staff at Quest Maritime for vessel logistics, and the crews of the Browse Invincible and the Browse Express for help in the field.
The authors acknowledge the financial support of Shell Australia Pty Ltd and the INPEX-operated Ichthys liquefied natural gas (LNG) project in conducting this research.
This paper was edited by Tyler Cyronak and reviewed by Isaiah Bolden and one anonymous referee.
Albright, R., Langdon, C., and Anthony, K. R. N.: Dynamics of seawater carbonate chemistry, production, and calcification of a coral reef flat, central Great Barrier Reef, Biogeosciences, 10, 6747–6758, https://doi.org/10.5194/bg-10-6747-2013, 2013.
Allgeier, J. E., Yeager, L. A., and Layman, C. A.: Consumers regulate nutrientlimitation regimes and primary production in seagrass ecosystems, Ecology, 94, 521–529, https://doi.org/10.1890/12-1122.1, 2013.
Allgeier, J. E., Layman, C. A., Mumby, P. J., and Rosemond, A. D.: Consistent nutrient storage and supply mediated by diverse fish communities in coral reef ecosystems, Glob. Change Biol., 20, 2459–2472, https://doi.org/10.1111/gcb.12566, 2014.
Allgeier, J. E., Valdivia, A., Cox, C., and Layman, C. A.: Fishing down nutrients on coral reefs, Nat. Commun., 7, 1–5, https://doi.org/10.1038/ncomms12461, 2016.
Andersson, A. J. and Gledhill, D.: Ocean acidification and coral reefs: Effects on breakdown, dissolution, and net ecosystem calcification, Annu. Rev. Mar. Sci., 5, 321–48, https://doi.org/10.1146/annurev-marine-121211-172241, 2013.
Anthony, K. R. N., Kleypas, J. A., and Gattuso, J. P.: Coral reefs modify their seawater carbon chemistry—implications for impacts of ocean acidification, Glob. Change Biol. , 17, 3655–3666, https://doi.org/10.1111/j.1365-2486.2011.02510.x, 2011.
Atkinson, M. J.: Biogeochemistry of nutrients, in: Coral Reefs: An ecosystem in transition, edited by: Dubinsky, Z. and Stambler, N., Springer, London, https://doi.org/10.1007/978-94-007-0114-4_13, 2011.
Auguie, B. and Antonov, A.: gridExtra: Miscellaneous Functions for “Grid” Graphics, CRAN [code], https://cran.r-project.org/web/packages/gridExtra/index.html (last access: 26 March 2019), 2017.
Bessey, C., Keesing, J. K., Mclaughlin, M. J., Rees, M., Tonks, M., Kendrick, G. A., and Olsen, Y. S.: Teleost community composition and the role of herbivory on the intertidal reef of a small isolated island in north-west Australia, Mar. Freshwater Res., 76, 684–696, https://doi.org/10.1071/MF19066, 2020.
Blair, S. M. and Norris, J. N.: The deep-water species of Halimeda Lamouroux (Halimediaceae, Chlorophyta) from San Salvador Island, Bahamas: species composition, distribution and depth records, Coral Reefs, 6, 227–236, https://doi.org/10.1007/BF00302019, 1988.
Borowitzka, M. A. and Larkum, A. W. D.: Calcification in algae: Mechanisms and the role of metabolism, Crit. Rev. Plant Sci., 6, 1–45, https://doi.org/10.1080/07352688709382246, 1987.
Bruno, J. F. and Edmunds, P. J.: Metabolic consequences of phenotypic plasticity in the coral Madracis mirabilis: the effect of morphology and water flow on aggregate respiration, J. Exp. Mar. Biol. Ecol., 229, 187–195, https://doi.org/10.1016/s0022-0981(98)00050-1, 1998.
Carlot, J., Rouzé, H., Barneche, D. R., Mercière, A., Espiau, B., Cardini, U., Brandl, S. J., Casey, J. M., Pérez-Rosales, G., Adjeroud, M., Hédouin, L., and Parravicini, V.: Scaling up calcification, respiration, and photosynthesis rates of six prominent coral taxa, Ecol. Evol., 12, e8613, https://doi.org/10.1002/ece3.8613, 2022.
Carpenter, R. C., Hackney, J. M., and Adey, W. H.: Measurements of primary productivity and nitrogenase activity of coral reef algae in a chamber incorporating oscillatory flow, Limnol. Oceanogr., 36, 40–49, https://doi.org/10.4319/lo.1991.36.1.0040, 1991.
Ceccarelli, D. M., Jones, G. P., and McCook, L. J.: Interactions between herbivorous fish guilds and their influence on algal succession on a coastal coral reef, J. Exp. Mar. Biol. Ecol., 399, 60–67, https://doi.org/10.1016/j.jembe.2011.01.019, 2011.
Chisholm, J. R. M. and Gattuso, J.-P.: Validation of the alkalinity anomaly technique for investigating calcification of photosynthesis in coral reef communities, Limnol. Oceanogr., 36, 1232–1239, https://doi.org/10.4319/lo.1991.36.6.1232, 1991.
Cornwall, C. E., Comeau, S., Komder, N. A., Perry, C. T., van Hooidonk, R., DeCarlo, T. M., Pratchett, M. S., Anderson, K. D., Browne, N., Carpenter, R., Diaz-Pulido, G., D'Olivo, J. P., Doo, S. S., Figueiredo, J., Fortunata, S. V. A., Kennedy, E., Lantz, C. A., McCulloch, M. T., Gonzalez-Rivero, M., Schoepf, V., Smithers, S. G., and Lowe, R. J.: Global declines in coral reef calcium carbonate production under ocean acidification and warming, P. Natl. Acad. Sci. USA, 118, e2015265118, https://doi.org/10.1073/pnas.2015265118, 2021.
DeAngelis, D. L.: Dynamics of Nutrient Cycling and Food Webs, Chapman and Hall, London, ISBN 940112342X, 9789401123426, 1992.
DeAngelis, D. L., Mulholland, P. J., Palumbo, A. V., Steinman, A. D., Huston, M. A., and Elwood, J. W.: Nutrient dynamics and food-web stability, Annu. Rev. Ecol. Syst., 20, 71–95, https://www.jstor.org/stable/2097085 (last access: 27 October 2022), 1989.
Dickson, A., Gilmore, C. L., Christian, S., and Christian, J. R.: Guide to best practices for ocean CO2 measurements, North Pacific Marine Science Organization, ISBN 1-897176-07-4, 2007.
Doney, S. C., Fabry, V. J., Feely, R. A., and Kleypas, J. A.: Ocean acidification: the other CO2 problem, Annu. Rev. Mar. Sci., 1, 169–192, https://doi.org/10.1146/annurev.marine.010908.163834, 2009.
Doughty, C. E. Roman, J., Faurbyd, S., Wolfe, A., Haquea, A., Bakkerf, E. S., Malhia, Y., Dunning Jr., J. B., and Svenninget, J.-C.: Global nutrient transport in a world of giants, P. Natl. Acad. Sci. USA, 113, 868–873, https://doi.org/10.1073/pnas.1502549112, 2015.
Drew, E. A.: Halimeda biomass, growth rates and sediment generation on reefs in the central great barrier reef province, Coral Reefs, 2, 101–110, https://doi.org/10.1007/BF02395280, 1983.
Eakin, C. M.: Where have all the carbonates gone? A model comparison of calcium carbonate budgets before and after the 1982–1983 El Nino at Uva Island in the eastern Pacific, Coral Reefs, 15, 109–119, https://doi.org/10.1007/BF01771900, 1996.
Feely, R. A., Sabine, C. L., Lee, K., Berelson, W., Kleypas, J., Fabry, V. J., and Millero, F. J.: Impact of anthropogenic CO2 on the CaCO3 system in the oceans, Science, 305, 362–66, https://doi.org/10.1126/science.1097329, 2004.
Frankignoulle, M., Gattuso, J. P., Biondo, R., Bourge, I., CopinMontegut, G., and Pichon, M.: Carbon fluxes in coral reefs II: Eulerian study of inorganic carbon dynamics and measurement of air–sea CO2 exchanges, Mar. Ecol.-Prog. Ser., 145, 123–132, https://doi.org/10.3354/meps145123, 1996.
Freile, D., Milliman, J. D., and Hillis, L.: Leeward bank margin Halimeda meadows and draperies and their sedimentary importance on the western Great Bahama Bank slope, Coral Reefs, 14, 27–33, https://doi.org/10.1007/BF00304068, 1995.
Gattuso, J.-P., Pichon, M., Delesalle, B., and Frankignoulle, M.: Community metabolism and air–sea CO2, fluxes in a coral reef ecosystem (Moorea, French Polynesia). Mar. Ecol.-Prog. Ser., 96, 259–267, http://www.jstor.org/stable/24833554 (last access: 29 August 2022), 1993.
Gattuso, J.-P., Pichon, M., and Frankignoulle, M.: Biological control of air–sea CO2 fluxes: Effect of photosynthetic and calcifying marine organisms and ecosystems. Mar. Ecol.-Prog. Ser., 129, 307–312, https://doi.org/10.3354/meps129307, 1995.
Gattuso, J.-P., Frankignoulle, M., Smith, S. V., Ware, J. R., and Wollast, R.: Coral reefs and carbon dioxide, Science, 271, 1298, https://doi.org/10.1126/science.271.5253.1298-a, 1996a.
Gattuso, J.-P., Pichon, M., Delesalle, B., Canon, C., and Frankignoulle, M.: Carbon fluxes in coral reefs I: lagrangian measurement of community metabolism and resulting air–sea CO2 disequilibrium, Mar. Ecol.-Prog. Ser., 145, 109–121, https://doi.org/10.3354/meps145109, 1996b.
Gattuso, J.-P., Payri, C. E., Pichon, M., Delesalle, B., and Frankignoulle, M.: Primary production, calcification, and air–sea CO2 fluxes of a macroalgal dominated coral reef community (Moorea, French Polynesia), J. Phycol., 33, 729–738, https://doi.org/10.1111/j.0022-3646.1997.00729.x, 1997.
Gattuso, J. P., Allemand, D., and Frankignoulle, M.: Photosynthesis and calcification at cellular, organismal and community levels in coral reefs: A review on interactions and control by carbonate chemistry, Am. Zool., 39, 160–183, https://doi.org/10.1093/icb/39.1.160, 1999.
Gattuso, J.-P., Epitalon, J.-M., Orr, J., Gentili, B., Hagens, M., Hofmann, A., Mueller, J.-D., Proye, A., Rae, J., and Soetaert, K.: seacarb: Seawater Carbonate Chemistry, CRAN [code], https://cran.r-project.org/web/packages/seacarb/index.html (last access: 26 March 2019), 2018.
Gilmour, J. P., Smith, L. D., Heyward, A. J., Baird, A. H., and Pratchett, M. S.: Recovery of an Isolated Coral Reef System Following Severe Disturbance, Science, 340, 69–71, https://doi.org/10.1126/science.1232310, 2013.
Halpern, B. S., Walbridge, S., Selkoe, K. A., Kappel, C. V., Micheli, F., Agrosa, C., Bruno, J. F., Casey, K. S., Ebert, C., Fox, H. E., Fujita, R., Heinemann, D., Lenihan, H. S., Madin, E. M. P., Perry, M. T., Selig, E. R., Spalding, M., Steneck, R., and Watson, R.: A Global Map of Human Impact on Marine Ecosystems, Science, 319, 948, https://doi.org/10.1126/science.1149345, 2008.
Halpern, B. S., Frazier, M., Afflerbach, J., Lowndes, J. S., Micheli, F., O'Hara, C., Scarborough, C., and Selkoe, K. A.: Recent pace of change in human impact on the world's ocean, Sci. Rep., 9, 11609, https://doi.org/10.1038/s41598-019-47201-9, 2019.
Harborne, A. R., Rogers, A., Bozec, Y.-M., and Mumby, P. J.: Multiple stressors and the functioning of coral reefs, Annu. Rev. Mar. Sci., 9, 445–468, 2017.
Harley, C. D., Hughes, A. R., Hultgren, K. M., Miner, B. J., Sorte, C. J. B., Thornber, C. S., Rodriguez, L. F., Tomanek, L., and Williams S. L.: The impacts of climate change in coastal marine systems, Ecol. Lett., 9, 228–241, https://doi.org/10.1111/j.1461-0248.2005.00871.x, 2006.
Hart, D. E. and Kench, P. S.: Carbonate production of an emergent reef platform, Warraber Island, Torres Strait, Australia, Coral Reefs, 26, 53–68, https://doi.org/10.1007/s00338-006-0168-8, 2007.
Hatcher, B. G.: Coral reef primary productivity–a hierarchy of patterns and process, Trends Ecol. Evol., 5, 149–155, https://doi.org/10.1016/0169-5347(90)90221-X, 1990.
Hewitt, J. E., Ellis, J. I., and Thrush, S. F.: Multiple stressors, nonlinear effects and the implications of climate change impacts on marine coastal ecosystems, Glob. Change Biol., 2, 2665–2675. https://doi.org/10.1111/gcb.13176, 2016.
Heyward, A., Jones, R., Meeuwig, J., Burns, K., Radford, B., Colquhoun, J., Cappo, M., Case, M., O'Leary, R., Fisher, R., Meekan, M., and Stowar, M.: Monitoring Study S5. Banks and Shoals, Montara 2011 Offshore Banks Assessment Survey Report for PTTEP Australasia (Ashmore Cartier) Pty. Ltd., Australian Institute of Marine Science, Townsville, 253 pp., 2011.
Hoegh-Guldberg, O., Mumby, P. J., Hooten, A. J., Steneck, R. S., Greenfield, P., Gomez, E., Harvell, C. D., Sale, P. F., Edwards, A. J., Caldeira, K., Knowlton, N., Eakin, C. M., Iglesias-Prieto, R., Muthiga, N., Bradbury, R. H., Dubi, A., and Hatziolos, M. E.: Coral reefs under rapid climate change and ocean acidification, Science, 318, 1737–1742, https://doi.org/10.1126/science.1152509, 2007.
Houlbreque, F. and Ferrier-Pages, C.: Heterotrophy in tropical scleractinian corals, Biol. Rev. Camb. Philos., 84, 1–17, https://doi.org/10.1111/j.1469-185X.2008.00058.x, 2009.
Houlbreque, F., Tambutte, E., Allemand, D., and Ferrier-Pages, C.: Interactions between zooplankton feeding, photosynthesis and skeletal growth in the Scleractinian coral Stylophora pistillata, J. Exp. Biol., 207, 1461–1469, https://doi.org/10.1242/jeb.00911, 2004.
Hudson, J. H.: Growth rate and carbonate production in Halimeda opuntia: Marquesas Keys, Florida, in: Paleoalgology: contemporary research and applications, edited by: Toomey, D. F. and Nitecki, M. H., Springer-Verlag, Berlin, https://doi.org/10.1007/978-3-642-70355-3_20, 1985.
Hughes, T. P., Kerry, J. T., Álvarez-Noriega, M., Álvarez-Romero, J. G., Anderson, K. D., Baird, A. H., Babcock, R. C., Beger, M., Bellwood, D. R., Berkelmans, R., Bridge, T. C., Butler, I. R., Byrne, M., Cantin, N. E., Comeau, S., Connolly, S. R., Cumming, G. S., Dalton, S. J., Diaz-Pulido, G., Eakin, C. M., Figueira, W. F., Gilmour, J. P., Harrison, H. B., Heron, S. F., Hoey, A. S., Hobbs, J. A., Hoogenboom, M. O., Kennedy, E. V., Kuo, C.-Y., Lough, J. M., Lowe, R. J., Liu, G., McCulloch, M. T., Malcolm, H. A., McWilliam, M. J., Pandolfi, J. M., Pears, R. J., Pratchett, M. S., Schoepf, V., Simpson, T., Skirving, W. J., Sommer, B., Torda, G., Wachenfeld, D. R., Willis, B. L., and Wilson, S. K.: Global warming and recurrent mass bleaching of corals, Nature, 543, 373–377, https://doi.org/10.1038/nature21707, 2017a.
Hughes, T. P., Barnes, M. L., Bellwood, D. R., Cinner, J. E., Cumming, G. S., Jackson, J. B. C., Kleypas, J., van de Leemput, I. A., Lough, J. M., Morrison, T. H., Palumbi, S. R., van Nes, E. H., and Scheffer, M.: Coral reefs in the Anthropocene, Nature, 546, 82–90, https://doi.org/10.1038/nature22901, 2017b.
IPCC: Climate Change 2014: Impacts, Adaptation, and Vulnerability, Part A: Global and Sectoral Aspects, Contribution of Working Group II to the Fifth Assessment Report of the Intergovernmental Panel on Climate Change, Cambridge University Press, Cambridge, United Kingdom and New York, 2014.
Jokiel, P. L.: Ocean Acidification and Control of Reef Coral Calcification by Boundary Layer Limitation of Proton Flux, B. Mar. Sci., 87, 639–657, https://doi.org/10.5343/bms.2010.1107, 2011.
Kangwe, J., Semesi, I. S., Beer, S., Mtolera, M., and Björk, M.: Carbonate Production by Calcareous Algae in a Seagrass-Dominated System: The Example of Chwaka Bay, Chapter 8, in: People, Nature and Research in Chwaka Bay, Zanzibar, Tanzania, edited by: de la Torre-Castro, M. and Lyimo, T. J., WIOMSA, Zanzibar, ISBN 978-9987-9559-1-6, 2012.
Kayanne, H., Suzuki, A., and Saito, H.: Diurnal changes in the partial pressure of carbon dioxide in coral reef water, Science, 269, 214—216, https://doi.org/10.1126/science.269.5221.214, 1995.
Kinsey, D. W.: Metabolism, calcification and carbon production: I: System level studies, Paper presented at 5th International Coral Reef Congress, Int. Coral Reef Soc., 27 May–1 June 1985, Tahiti, ISBN 2905630035, 1985.
Kleypas, J. A. and Yates, K. K.: Coral reefs and ocean acidification, Oceanography, 22, 108–117, https://doi.org/10.5670/oceanog.2009.101, 2009.
Lesser, M. P., Weis, V. M., Patterson, M. R., and Jokiel, P. L.: Effects of morphology and water motion on carbon delivery and productivity in the reef coral, Pocillopora damicornis: diffusion barriers, inorganic carbon limitation, and biochemical plasticity, J. Exp. Mar. Biol. Ecol., 178, 153–179, https://doi.org/10.1016/0022-0981(94)90034-5, 1994.
Lewis, J. B.: Process of organic production on coral reefs, Biol. Rev., 52, 305–347, https://doi.org/10.1111/j.1469-185X.1977.tb00836.x, 1977.
Madin, J. S., Anderson, K. D., Andreasen, M. H., Bridge, T. C. L., Cairns, S. D., Connolly, S. R., Darling, E. S., Diaz, M., Falster, D. S., Franklin, E. C., Gates, R. D., Harmer, A. M. T., Hoogenboom, M. O., Huang, D., Keith, S. A., Kosnik, M. A., Kuo, C.-Y., Lough, J. M., Lovelock, C. E., Luiz, O., Martinelli, J., Mizerek, T., Pandolfi, J. M., Pochon, X., Pratchett, M. S., Putnam, H. M., Roberts, T. E., Stat, M., Wallace, C. C., Widman, E., and Baird, A. H.: The Coral Trait Database, a curated database of trait information for coral species from the global oceans, Scientific Data, 3, 160017, https://doi.org/10.1038/sdata.2016.17, 2016.
Mass, T., Genin, A., Shavit, U., Grinstein, M., and Tchernov, D.: Flow enhances photosynthesis in marine benthic autotrophs by increasing the efflux of oxygen from the organism to the water, P. Natl. Acad. Sci. USA, 107, 2527–2531, https://doi.org/10.1073/pnas.0912348107, 2010.
McLaughlin, M. J., Lourey, M. J., Hanson, C. E., Cherukuru, N, Thompson, P. A., and Pattiaratchi, C.: Biophysical oceanography of tidally-extreme waters of the southern Kimberley coast, Western Australia, Cont. Shelf Res., 173, 1–12, https://doi.org/10.1016/j.csr.2018.12.002, 2019.
Meyer, J. L., Schultz, E. T., and Helfman, G. S.: Fish schools–an asset to corals, Science 220, 1047–1049, https://doi.org/10.1126/science.220.4601.1047, 1983.
Moberg F., and Folke C.: Ecological goods and services of coral reef ecosystems, Ecol. Econ., 29, 215–233, https://doi.org/10.1016/S0921-8009(99)00009-9, 1999.
Montaggioni, L. F. and Braithwaite, C. J. R.: Quaternary coral reef systems, edited by: Chamley, H., Elsevier, p. 532, ISBN 978-0-444-53247-3, 2009.
Moore, J. A. Y., Bellchambers, L. M., Depczynski, M. R., Evans, R. D., Evans, S. N., Field, S. N., Friedman, K. J., Gilmour, J. P., Holmes, T. H., Middlebrook, R., Radford, B. T., Ridgway, T., Shedrawi, G., Taylor, H., Thomson, D. P., and Wilson, S. K.: Unprecedented Mass Bleaching and Loss of Coral across 12∘ of Latitude in Western Australia in 2010–11, PLoS ONE, 7, e51807, https://doi.org/10.1371/journal.pone.0051807, 2012.
Nelson, W. A.: Calcified macroalgae – critical to coastal ecosystems and vulnerable to change: a review, Mar. Freshwater Res., 60, 787–801, https://doi.org/10.1071/MF08335, 2009.
Olsen, Y. S., Bessey, C., McLaughlin, J., and Keesing, J.: 2017 Annual Report: Patterns in primary producers, herbivory and reef metabolism around Browse Island, Shell/INPEX Applied Research Program, UWA and CSIRO, https://www.shell.com.au/sustainability/environment/_jcr_content/par/toptasks_b64e.stream/1536897804284/e4d8489abff3f2bc193cc7bda779d6eeda0e59ab/arp7-2milestone-2017-report-2.pdf (last access: 12 March 2023), 2017.
Overholtzer, K. L. and Motta, P. J.: Comparative resource use by juvenile parrotfishes in the Florida Keys, Mar. Ecol.-Prog. Ser., 177, 177–187, 1999.
Panchang, R. and Ambokar, M.: Ocean acidification in the Northern Indian ocean: A review, J. Asian Earth Sci., 219, 104904, https://doi.org/10.1016/j.jseaes.2021.104904, 2021.
Payri, C. E.: Halimeda contribution to organic and inorganic production in a Tahitian reef system, Coral Reefs, 6, 251–262, https://doi.org/10.1007/BF00302021, 1988.
Perry, C. T., Spencer, T., and Kench, P.: Carbonate budgets and reef production states: a geomorphic perspective on the ecological phase-shift concept, Coral Reefs, 27, 853–866, https://doi.org/10.1007/s00338-008-0418-z, 2008.
Perry, C. T., Edinger, E. N., Kench, P. S., Murphy, G. N., Smithers, S. G., Steneck, R. S., and Mumby, P. J.: Estimating rates of biologically driven coral reef framework production and erosion: a new census-based carbonate budget methodology and applications to the reefs of Bonaire, Coral Reefs, 31, 853–868, https://doi.org/10.1007/s00338-012-0901-4, 2012.
Perry, C. T., Alvarez-Filip, L., Graham, N. A. J., Mumby, P. J., Wilson, S. K., Kench, P. S., Manzello, D. P., Morgan, K. M., Slangen, A. B. A., Thomson, D. P., Januchowski-Hartley, F., Smithers, S. G., Steneck, R. S., Carlton, R., Edinger, E. N., Enochs, I. C., Estrada-Saldívar, N., Haywood, M. D. E., Kolodziej, G., Murphy, G. N., Pérez-Cervantes, E., Suchley, A., Valentino, L., Boenish, R., Wilson, M., and Macdonald, C.: Loss of coral reef growth capacity to track future increases in sea level, Nature, 558, 396–400, https://doi.org/10.1038/s41586-018-0194-z, 2018.
Pomeroy, L. R.: The ocean's food web, a changing paradigm, Bioscience, 24, 499–504, https://doi.org/10.2307/1296885, 1974.
Price, N. N., Hamilton, S. L., Tootell, J. S., and Smith, J. E.: Species-specific consequences of ocean acidification for the calcareous tropical green algae Halimeda, Mar. Ecol.-Prog. Ser., 440, 67–78, https://doi.org/10.3354/meps09309, 2011.
R Core Team: R: A language and environment for statistical computing, R Foundation for Statistical Computing, Vienna, Austria, https://www.R-project.org/ (last access: 26 March 2019), 2018.
Rees, S. A., Opdyke, B. N., Wilson, P. A., and Henstock, T. J.: Significance of Halimeda bioherms to the global carbonate budget based on a geological sediment budget for the Northern Great Barrier Reef, Australia, Coral Reefs, 26, 177–188, https://doi.org/10.1007/s00338-006-0166-x, 2007.
Richards, Z. T., Garcia, R. A., Wallace, C. C., Rosser, N. L., and Muir, P. R.: A diverse assemblage of reef corals thriving in a dynamic intertidal reef setting (Bonaparte Archipelago, Kimberley, Australia), PLOS ONE, 10, e0117791, https://doi.org/10.1371/journal.pone.0117791, 2015.
Rueden, C. T., Schindelin, J., Hiner, M. C., DeZonia, B. E., Walter, A. E., Arena, E. T., and Eliceiri, K. W.: ImageJ2: ImageJ for the next generation of scientific image data, BMC Bioinformatics, 18, 529, https://doi.org/10.1186/s12859-017-1934-z, 2017.
Sarkar, D.: Lattice: Multivariate Data Visualization with R, Springer, New York., ISBN 978-0-387-75968-5, 2008 (code available at: http://lmdvr.r-forge.r-project.org, last access: 26 March 2019).
Schindler, D. W.: Recent advances in the understanding and management of eutrophication, Limnol. Oceanogr., 51, 356–363, https://doi.org/10.4319/lo.2006.51.1_part_2.0356, 2006.
Shaw, E. C., Hamylton, S. M., and Phinn, S. R.: Incorporating benthic community changes into hydrochemical-based projections of coral reef calcium carbonate production under ocean acidification, Coral Reefs, 35, 739–750, https://doi.org/10.1007/s00338-016-1407-2, 2016.
Silverman, J., Lazar, B., Cao, L., Caldeira, K., and Erez, J.: Coral reefs may start dissolving when atmospheric CO2 doubles, Geophys. Res. Lett., 36, L05606, https://doi.org/10.1029/2008gl036282, 2009.
Smith, J. E., Hunter, C. L., Conklin, E. J., Most, R., Sauvage, T., Squair, C., and Smith, C. M.: Ecology of the Invasive Red Alga Gracilaria salicornia (Rhodophyta) on O'ahu, Hawai'i, Pac. Sci., 58, 325–343, https://doi.org/10.1353/psc.2004.0023, 2004.
Smith, J. E., Price, N. N., Nelson, C. E., and Haas, A. F.: Coupled changes in oxygen concentration and pH caused by metabolism of benthic coral reef organisms, Mar. Biol., 160, 2437–2447, https://doi.org/10.1007/s00227-013-2239-z, 2013.
Smith, S. V.: Reflections on the measurement and significance of carbon metabolism on coral reefs, Kansas Geological Survey Open-File Report 95-96a, Kansas Geological Survey, Lawrence, Kansas, https://cir.nii.ac.jp/crid/1570291224753885568 (last access: 13 March 2023), 1995.
Smith, S. V. and Key, G. S.: Carbon dioxide and metabolism in marine environments, Limnol. Oceanogr., 20, 493–495, https://doi.org/10.4319/lo.1975.20.3.0493, 1975.
Smith, T. B., Nemeth, R. S., Blondeau, J., Calnan, J. M., Kadison, E., and Herzlieb S.: Assessing coral reef health across onshore to offshore stress gradients in the US Virgin Islands, Mar. Pollut. Bull., 56, 1983–1991, https://doi.org/10.1016/j.marpolbul.2008.08.015, 2008.
Sorokin, Y.: Coral Reef Ecology, edited by: Heldmaier, G., Lange, O. L., Mooney, H. A., and Sommer, U., Springer, Berlin, Heidelberg, 215–249, https://doi.org/10.1007/978-3-642-80046-7, 1995.
Sreeush, M. G., Valsala, V., Santanu, H., Pentakota, S., Prasad, K. V. S. R., Naidu, C. B., and Murtugudde, R.: Biological production in the Indian Ocean upwelling zones – Part II: Data based estimates of variable compensation depths for ocean carbon mdels via Cyclostationary Bayesian Inversion, Deep-Sea Res. Pt. II, 179, 104619, https://doi.org/10.1016/j.dsr2.2019.07.007, 2020.
Stuart-Smith, R. D., Brown, C. J., Ceccarelli, D. M., and Edgar, G. J.: Ecosystem restructuring along the Great Barrier Reef following mass coral bleaching, Nature, 560, 92–96, 2018.
Szmant, A. M.: Nutrient enrichment on coral reefs: is it a major cause of coral reef decline?, Estuaries, 25, 743–766, https://doi.org/10.1007/BF02804903, 2002.
Trapon, M. L., Pratchett, M. S., and Hoey, A. S.: Spatial variation in abundance, size and orientation of juvenile corals related to the biomass of parrotfishes on the Great Barrier Reef, Australia, PLOS One, 8, e57788, https://doi.org/10.1371/journal.pone.0057788, 2013.
Veal, C. J., Carmi, M., Fine, M., and Hoegh-Guldberg, O.: Increasing the accuracy of surface area estimation using single wax dipping of coral fragments, Coral Reefs, 29, 893–897, https://doi.org/10.1007/s00338-010-0647-9, 2010.
Vecsei, A.: A new estimate of global reefal carbonate production including the fore-reefs, Global Planet. Change, 43, 1–18, https://doi.org/10.1016/j.gloplacha.2003.12.002, 2004.
Venables, W. N. and Ripley, B. D.: Modern Applied Statistics with S, 4th Edn., Springer, New York, ISBN 0-387-95457-0, 2002 (code available at: https://www.stats.ox.ac.uk/pub/MASS4/, last access: 26 March 2019).
Vroom, P. S., Smith, C. M., Coyer, J. A. Walters, L. J., Hunter, C. L., Beach, K. S., and Smith, J. E.: Field biology of Halimeda tuna (Bryopsidales, Chlorophyta) across a depth gradient: comparative growth, survivorship, recruitment, and reproduction, Hydrobiologia, 501, 149–166, https://doi.org/10.1023/A:1026287816324, 2003.
Walther, G.-R.: Community and ecosystem responses to recent climate change, Philos. T. R. Soc. B, 365, 2019–2024, https://doi.org/10.1098/rstb.2010.0021, 2010.
Watson, R. J., Butler, E. C. V., Clementson, L. A., and Berry, K. M.: Flow-injection analysis with fluorescence detection for the determination of trace levels of ammonium in seawater, J. Environ. Monitor., 7, 37–42, https://doi.org/10.1039/b405924g, 2005.
Ware, J. R., Smith, S. V., and Reaka-Kudla, M. L.: Coral reefs: Sources or sinks of atmospheric CO2?, Coral Reefs, 11, 127–130, https://doi.org/10.1007/BF00255465, 1992.
Wickham, H.: The Split-Apply-Combine Strategy for Data Analysis, J. Stat. Softw., 40, 1–29, https://doi.org/10.18637/jss.v040.i01, 2011.
Wickham, H.: ggplot2: Elegant Graphics for Data Analysis, Springer-Verlag New York, ISBN 978-3-319-24277-4, 2016 (code available at: https://ggplot2.tidyverse.org, last access: 26 March 2019).
WillyWeather: Browse Island Sunrise/Sunset Times website, https://sunrisesunset.willyweather.com.au/wa/kimberley/browse-island.html, last access: 2 November 2022.