the Creative Commons Attribution 4.0 License.
the Creative Commons Attribution 4.0 License.
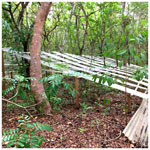
Throughfall exclusion and fertilization effects on tropical dry forest tree plantations, a large-scale experiment
German Vargas Gutiérrez
Daniel Pérez-Aviles
Nanette Raczka
Damaris Pereira-Arias
Julián Tijerín-Triviño
L. David Pereira-Arias
David Medvigy
Bonnie G. Waring
Ember Morrisey
Edward Brzostek
Jennifer S. Powers
Across tropical ecosystems, global environmental change is causing drier climatic conditions and increased nutrient deposition. Such changes represent large uncertainties due to unknown interactions between drought and nutrient availability in controlling ecosystem net primary productivity (NPP). Using a large-scale manipulative experiment, we studied for 4 years whether nutrient availability affects the individual and integrated responses of aboveground and belowground ecosystem processes to throughfall exclusion in 30-year-old mixed plantations of tropical dry forest tree species in Guanacaste, Costa Rica. We used a factorial design with four treatments: control, fertilization (F), drought (D), and drought + fertilization (D + F). While we found that a 13 %–15 % reduction in soil moisture only led to weak effects in the studied ecosystem processes, NPP increased as a function of F and D + F. The relative contribution of each biomass flux to NPP varied depending on the treatment, with woody biomass being more important for F and root biomass for D + F and D. Moreover, the F treatment showed modest increases in maximum canopy cover. Plant functional type (i.e., N fixation or deciduousness) and not the experimental manipulations was the main source of variation in tree growth. Belowground processes also responded to experimental treatments, as we found a decrease in nodulation for F plots and an increase in microbial carbon use efficiency for F and D plots. Our results emphasize that nutrient availability, more so than modest reductions in soil moisture, limits ecosystem processes in tropical dry forests and that soil fertility interactions with other aspects of drought intensity (e.g., vapor pressure deficit) are yet to be explored.
- Article
(3587 KB) - Full-text XML
-
Supplement
(3553 KB) - BibTeX
- EndNote
Global environmental change is affecting primary productivity in tropical forest ecosystems. Among the main factors behind this variation in productivity are the changes in the hydrologic regime due to an increase in rainfall seasonality (Feng et al., 2013), increases in atmospheric water demand (McDowell et al., 2020), and regional decreases in soil moisture (Seneviratne et al., 2010). In other words, the tropics are getting drier. Results from observational studies found that droughts may increase tropical tree mortality rates (Powers et al., 2020; Chazdon et al., 2005), reduce aboveground biomass productivity (Phillips et al., 2009; Castro et al., 2018), reduce the production of seeds and flowers (O'Brien et al., 2018), and increase the abundance of high wood density and deciduous tree species (Swenson et al., 2020; Aguirre-Gutiérrez et al., 2020). However, how tropical forests respond to drought depends on other environmental factors such as soil fertility and the interaction with other disturbances (Brando et al., 2014; Brodribb et al., 2020; Becknell et al., 2021). Accounting for how these environmental variables modulate ecosystem responses to drought will enhance our understanding of the impacts of global environmental change on tropical forests, which play a disproportionate role in global carbon dynamics and provide ecosystem services to one-fourth of the world's population (Wright, 2005; Lewis, 2006).
One largely overlooked factor is the potential role of nutrient availability in mediating tropical forests' vulnerability to drought. Tropical forests exist in a highly heterogeneous mosaic of soil fertility, parent material, and topography (Townsend et al., 2008; Augusto et al., 2017; Waring et al., 2021), properties that shape forest composition (Condit et al., 2013; Werden et al., 2018a) and function (Clark and Clark, 2000; Cunha et al., 2022). Nutrient-limited environments harbor a greater proportion of slow-growing, drought-tolerant species, whereas fast-growing drought-avoiding species dominate nutrient-rich environments (Oliveira et al., 2021), which suggests that soils play an important role in determining the heterogeneity of tropical forest responses to drought. Moreover, anthropogenic activities can cause an increase in atmospheric nitrogen and phosphorus deposition across ecosystems (Wang et al., 2017), and yet the consequences of these changes in combination with rainfall variation remain unknown in tropical forests (Matson et al., 1999; Hietz et al., 2011). In summary, the empirical evidence needed to characterize drought–nutrient interactions has yet to be documented but is highlighted as a priority to parameterize vegetation dynamics models (Smith et al., 2014).
1.1 Nutrient and water availability modulates ecosystem processes
Soil fertility is an important factor modulating the responses of forest productivity to rainfall variation. For instance, tropical dry forest (TDF) stands growing in more fertile soils tend to show higher increases in productivity with higher rainfall than stands in nutrient-poor soils (Medvigy et al., 2019; Becknell et al., 2021). In low-nutrient environments, plants maximize transpiration rates to increase mass flow nutrient uptake, but variations in water availability could limit these processes with potential costs to ecosystem productivity (Santiago, 2015). Other processes besides primary productivity provide insight into ecosystem responses to global environmental change. Leaves, and more precisely canopy cover, are the center for carbon assimilation in forest ecosystems. Recent evidence suggests that the patterns of leaf flushing and leaf shedding are changing at a global scale because of climate change (Piao et al., 2019). While it is well documented that TDF leaf phenological cycles depend on plant water status and the start of the rainy season (Frankie et al., 1974; Borchert, 1994), phosphorus fertilization seems to reduce leaf lifespan in eastern Amazon forests (Cunha et al., 2022). A decrease in leaf canopy cover affects productivity by decreasing the photosynthetic area (Doughty and Goulden, 2008), while changes in the timing of leaf flushing or shedding may create a cascade of effects with unknown consequences, which will affect organisms that depend on these processes (Coley, 1998). Thus, quantifying the combined effects of rainfall reductions and soil fertility on leaf production is key to disentangling the interactions between primary productivity, canopy processes, nutrient availability, and climate.
The extent to which nutrient and water availability interactions affect belowground processes is highly uncertain, particularly in TDFs (Phillips et al., 2016; Allen et al., 2017). The increase in specific nutrients (i.e., via nitrogen deposition) might cause an imbalance in stoichiometry or increase water demand, which plants will adjust by increasing transpiration rates or producing more root biomass (Lu et al., 2018; Waring et al., 2019; Cunha et al., 2022). It is also highly uncertain how these changes may affect the microbial processes that determine carbon cycling. For instance, whether soil microbial carbon use efficiency (CUE; the proportion of total carbon consumed that is used to grow new biomass) can acclimate in response to changes in water and nutrient availability is not known. Current knowledge suggests that microbes with high CUE produce more biomass that upon death becomes protected from future microbial attack by adhering to mineral surfaces (Cotrufo et al., 2013). Under drought, the CUE of the microbial community may decrease owing to the need to use carbon for survival strategies rather than for growth (Schimel et al., 2007). However, it is possible that reducing microbial nutrient limitation may alleviate the impacts of drought on CUE due to microbes investing less energy in resource acquisition (Schimel et al., 2007). Other aspects of soil microbial processes may be affected by drought or modulated by soil nutrient availability (Ahmed et al., 2018). Soil priming refers to the decomposition of older recalcitrant organic matter following the soil microbial community's stimulation by adding labile organic matter (Liu et al., 2020). If drought alters patterns of fine-root growth and rhizodeposition (Preece and Peñuelas, 2016), this may lead to altered priming with altered consequences of soil organic carbon storage. Identifying the extent to which shifts in nutrient and precipitation regimes alter soil carbon cycling in TDFs is critical to increasing our understanding of climate change consequences in this important biome (Knorr et al., 2005; Chadwick et al., 2016).
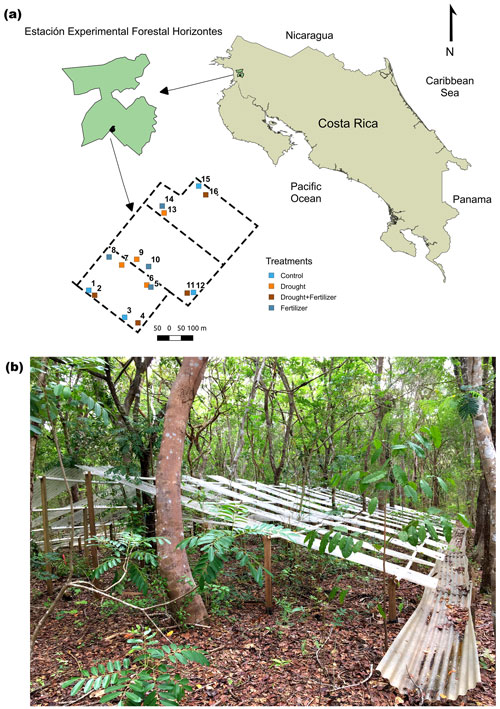
Figure 1(a) Geographical location and layout of a throughfall exclusion by fertilization experiment in northwestern Costa Rica. (b) Picture of a throughfall exclusion structure in a 30-year-old Swietenia macrophylla King. and Hymenaea courbaril L. plantation. The boundary of Horizontes, locations of mixed plantations, and trails were obtained from the public geographic information system and layer repository for the Área de Conservación Guanacaste (https://www.acguanacaste.ac.cr/biodesarrollo/sistemas-de-informacion-geografica/capas-sig, last access: August 2016).
Table 1Focal tree species present in the experimental manipulations and their functional and hydraulic traits measured in other studies (data from Powers and Tiffin, 2010; Powers et al., 2020). Here we present species leaf habit (LH) as deciduous (DC), semi-deciduous (SD), or evergreen (EV), whether the species is nitrogen fixer (NF), specific leaf area (SLA, cm2 g−1), wood density (WD, g cm−3), water potential at turgor loss point (ΨTLP, Mpa), or water potential at 50 % accumulation of embolisms (ΨP50, Mpa).
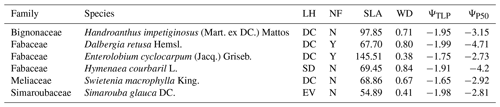
1.2 Experimental framework
Carbon cycling in TDFs is likely limited by both water and nutrient availability (Lugo and Murphy, 1986; Castro et al., 2018; Medvigy et al., 2019; Becknell et al., 2021). This colimitation of resources highlights the importance of quantifying the individual and interactive roles of these two factors in shaping ecosystem processes in this important and threatened biome (Hoekstra et al., 2005; Miles et al., 2006). Large-scale manipulative experiments are needed to understand the interactions between drought and nutrient limitation, although to date an experiment testing these two factors simultaneously has not been implemented in tropical forests. While nutrient addition experiments have shown mixed (strong, weak, and none) effects on tree growth in tropical forests (Wright et al., 2018; Hou et al., 2020; Cunha et al., 2022), results from throughfall exclusion experiments suggest an initial decrease in woody productivity over the first 2 years and an increase in mortality after 5 years (Meir et al., 2015). Most of these large-scale experiments have been conducted in wet tropical forests (mean annual rainfall > 2000 mm) (Meir et al., 2015; Wright et al., 2018), despite TDFs' documented vulnerability to drought (Powers et al., 2020).
To investigate whether nutrient availability modulates changes in ecosystem processes in response to reduced rainfall manipulations, we established a large-scale, fully factorial experiment in mixed-species plantations as model TDF stands. We used rainout shelters covering 50 % of the forest floor area to reduce soil moisture and/or fertilizer applications to increase soil nutrient availability (Fig. 1). We focused data collection on aboveground and belowground ecosystem processes that contribute to carbon cycling. Over a 4-year period, we performed measurements on tree diameter growth, canopy cover, and litterfall production as aboveground processes and measurements of fine-root production, nodulation, microbial CUE, and soil priming as belowground processes. We then integrated ecosystem-level responses to the experimental manipulations by quantifying net primary productivity, aboveground primary productivity, and the carbon allocation between aboveground and belowground biomass.
Our experiment was conducted for 4 years (2016–2020) at Estación Experimental Forestal Horizontes (hereafter Horizontes) in northwestern Costa Rica (10.711∘ N, 85.578∘ W) (Fig. 1). Before Horizontes was incorporated into Área de Conservación Guanacaste (ACG), the lands were used for grazing and crops (Werden et al., 2018b). Since 1989, Horizontes has served as a large-scale ecological and forestry research laboratory, and the ∼ 7500 ha area encompasses a mosaic of TDFs at different successional stages (0–80 years), 64 ha of timber plantation trials of native TDF species (Gutiérrez-Leitón, 2018), restoration trials (Werden et al., 2020), and a Mesoamerican TDF arboretum (http://www.arbnet.org/morton-register/arboretum-del-bosque-seco-tropical, last access: July 2021). During the study period, total annual rainfall averaged ∼ 1547 mm, and median temperatures were 26.5 ± 1.6 ∘C during the dry season and 25.6 ± 1.5 ∘C during the wet season (Fig. S1 in the Supplement). Precipitation values were in the range with the historical average of ∼ 1500 mm (Vargas G. et al., 2015). In Horizontes the start of the wet season is defined when cumulative rainfall reaches 100 mm, which usually occurs in May and defines the beginning of the hydrological year (i.e., 12 months after cumulative rainfall reaches 100 mm) (Aragão et al., 2007; Waring et al., 2019). Therefore, our temporal scale is the hydrological year, as in TDFs the start of the rainy season determines the beginning of leaf production, seed germination, and other ecological processes (Murphy and Lugo, 1986).
2.1 Experimental design
We conducted our experiment in tree plantations that were established in 1991 (Gutiérrez-Leitón, 2018). The plantations consist of three 8–10 ha blocks that each contain one of three focal species combined with 1 of 4 species from a pool of 11 species native to northwestern Costa Rica (Fig. S2). The plantations have not received any management for 25 years prior to our experiment, trees were planted at a spacing of 3 m × 3 m, and the understory now contains a diverse community of 15 lianas and 50 trees/shrubs (Fig. 1). We selected six species that represent functional types common to the TDF based on species' ability to fix atmospheric nitrogen and leaf habit phenology (Table 1) (Xu et al., 2016; Powers and Tiffin, 2010). We took a tree-centered approach in locating the plots to include at least six individuals of each focal species in the four treatments, with a minimum of 12 trees per plot. For this reason, the plot area ranged from 120 to 360 m2 and contained a two-species combination that we designated as a stand (Table S1 in the Supplement). This experimental design was a compromise that allowed us to have at least four individuals of each species within plots per treatment. Before selecting the plot locations, we did extensive surveys of tree diameters to ensure that there were no systematic differences in tree diameters within species among treatments (Fig. S3). Soil samples (0–10 cm depth) were collected in 2016 and 2021 by taking 7 to 10 cores (2.5 cm diameter, 1 on each corner and 3 to 6 in the center line of the plot) and compositing cores by the plot. Particle size distribution was collected in 2016 (Table S2) and extractable elements (Olson extractable Cu, Zn, Mn, Fe, K, and P) and total C and N for samples collected in the fifth year (2021).
We performed nutrient and drought manipulations using a fully factorial design with four treatments: fertilization (F), drought (D), drought + fertilization (D + F), and unmanipulated control. We established four plot replicates per experimental treatment that each contained two of the six tree species, such that every species was represented in one plot of each treatment. The D and D + F treatments consisted of a series of structures that covered 50 % of the surface area at each plot and were suspended at a 40∘ angle at distances from 0.4 to 2.5 m above the ground (Fig. 1). The throughfall exclusion shelters were built with transparent polycarbonate corrugated sheets, wood poles, and polyvinyl chloride pipes. To avoid lateral fine-root growth outside the throughfall exclusion structures, we dug a 50 cm-deep trench around each exclusion plot that was covered with a barrier of double-folded 0.075 mm-thick polyethylene film and then backfilled. Precipitation was routed off the throughfall exclusion plots by a system of gutters and ground channels (Fig. 1). For the F and D + F treatments, a slow-release complete-formula (macronutrients and micronutrients) nutrient fertilizer was broadcast uniformly over the entire plot area in two applications during the rainy season each year. From 2016 to 2018 we used Basacote® Plus 3M (Compo Expert GmbH), and then, due to low market availability from 2018 through 2020, we used Osmocote® Plus (Scotts Company LLC) (Table S3). Nutrient addition rates were targeted to 150 (Table S1), similar to other large-scale tropical forest fertilization experiments (Wright et al., 2011; Alvarez-Clare et al., 2013; Waring et al., 2019). We placed fertilized plots more than 50 m away from other plots or down the slope from control and drought plots whenever we could not find enough trees 50 m away. These measures considered the possibility of nutrient leaching from one plot to another one. Finally, because litterfall accumulated on the surfaces of the plastic panels, every 2 weeks we used long brooms to sweep the litter off the panels and then place it under each panel.
2.2 Soil moisture
We quantified volumetric soil moisture at a 30 min frequency for the duration of the experiment with an EM50 Digital data logger equipped with four 10-HS soil moisture probes (METER Group, Inc. USA). Probes were distributed in two opposite pairs from the center of each plot, each pair consisting of a probe at 10 cm depth and another probe at 40 cm depth in the soil.
2.3 Aboveground processes
2.3.1 Tree growth
From December 2016 to December 2020, we measured the diameter at breast height (DBH) for all stems greater than 2.5 cm DBH annually at the end of the growing season. These measurements included the plantation trees and every stem that recruited into the 2.5 DBH size class before and during the experiment. All trees and shrubs were identified to species level and classified into nitrogen fixation and leaf habit (evergreen or deciduous) functional types. For stems between 2.5 and 10 cm DBH, diameter was measured with a diameter tape at a marked point 130 cm above the ground. In the case of stems > 10 cm DBH, we measured DBH increments using band dendrometers set at 130 cm. For each stem we calculated relative growth (RGR) as , where DC represents the day of the century, i and f final DBH and DC values for a given stem (Wright et al., 2011).
2.3.2 Canopy productivity
We measured canopy productivity from January 2017 through December 2020 using two complementary methods: litterfall traps and leaf area index. To measure litterfall production, we deployed three 0.25 m2 traps ∼ 0.4 m above the ground in a transect along the center of each plot. In plots with throughfall exclusion structures, traps were in the spaces between the polycarbonate sheets. Litter was collected monthly from each trap, dried for 72 h at 60 ∘C, sorted into leaves, small branches, flowers, fruits, and frass, and then weighed separately. We then calculated the annual litterfall productivity () for total litterfall (leaves, small branches, flowers, and fruits), only leaves, and reproductive litterfall (flowers and fruits).
Leaf area index (LAI) was measured at seven points at each plot (four in each corner and three along the center) every 10 to 30 d with an LAI-2200C Plant Canopy Analyzer (LI-COR Biosciences, Lincoln, NE, USA). The variation in sampling frequency was caused by logistical constraints that wet seasons occasionally imposed on our ability to reach the plots. Because of the high abundance of species from the Fabaceae family in the plots, LAI measurements were performed after sunrise (between 09:00 and 11:00 GMT−6) given the associated nastic movements in leaves after dawn and before dusk (Minorsky, 2019). For that reason, we took each measurement using a 45∘ angle cap towards the center of the plot and performed scattering correction before and after each measurement cycle throughout the entire experiment. LAI data were subsequently estimated from the first four gap fractions using the software application FIV-2200 (LI-COR Biosciences, Lincoln, NE, USA). In 2017, tropical storm Nate, which impacted 85 % of the whole Costa Rican territory (Quesada-Román et al., 2020), caused a significant LAI decrease during the month of November (Fig. S4). For that reason, we dropped the measurements of November and December 2017 from all the analyses involving LAI data. From the LAI data, we extracted leaf area duration (LAD, ), which describes the temporal dynamics and leaf persistence in the canopy of broadleaf plant communities (Ewert and Pleijel, 1999; Norby et al., 2003). LAD is defined as the area under the nonlinear curve of LAI as a function of the Julian day:
where b describes the beginning of the growing season in Julian days, e the end of the growing season in Julian days, and LAI(t) the function of LAI temporal variation during the given growing season (Pokorný et al., 2008). The growing season in this case is defined by leaf flushing and leaf fall (Norby et al., 2003). We obtained the growing season parameters b and e from plot-specific and year-specific generalized additive models by estimating the Julian days on which LAI starts to increase (positive slope change) from the minimum and when it starts to decrease (negative slope change) after the maximum LAI (Methods S1 in the Supplement). Then we fitted the LAI temporal variation during the growing season and integrated it from b to e to obtain the area under the nonlinear function LAI(t) (Fig. S5). In addition to LAD, for each plot, we calculated the maximum LAI value during the growing season (LAImax, m2 m−2), minimum LAI during the dry season (LAImin, m2 m−2), leafless period (LLP, d), start of leaf flushing defined as the growing season beginning (GSB, d), and seasonal LAI enlargement (LAE, %), which is the percent change in LAI from the dry season to the wet season (Pokorný et al., 2008).
2.4 Belowground processes
2.4.1 Fine-root and nodule production
We measured fine-root production from July 2016 through December 2020 using the ingrowth core method (Waring et al., 2016). To do this, we installed seven ingrowth cores in each plot to a depth of 15 cm. With this method, we quantified fine roots as the biomass of new root growth inside an 8 cm diameter cylindrical ingrowth bag with a 2 mm nylon mesh. The cores were collected 2 months after deployment, and a subsequent new set of cores was installed right after collection. While deploying the cores, we filled them with sieved, root-free soil collected on site. During the first year of the experiment, cores were sampled in the dry season. However, the clay-rich soils harden greatly during the dry season, which increased the difficulty of deploying new bags during these times. For the following 3 years, ingrowth bags were harvested in June, August, and November, with the modification that the bags harvested in June were deployed in November. We acknowledge that roots may have grown, died, or decomposed during the dry season (Kummerow et al., 1990). However, this effect will lead to minimal bias in annual totals, as dry-season root growth and decomposition are expected to be negligible in the TDF (Kavanagh and Kellman, 1992). After collecting the cores, fine roots were separated from the soil by washing them over a 2 mm sieve. We counted the number of nodules on each root sample if present. Finally, root samples were dried for 72 h at 60 ∘C and weighed to estimate total fine-root productivity ().
2.4.2 Microbial CUE and priming
To analyze microbial CUE, we collected 10 soil samples (5 cm diameter, 15 cm depth) from each plot during the wet season in August 2019 and homogenized them into 1 soil sample per plot. The samples were expedited back to the University of Minnesota, where a laboratory microcosm experiment was performed. Microbial CUE was assessed using the 13C-glucose tracing method (Frey et al., 2013): briefly, > 97 % 13C glucose (Cambridge Isotope Laboratories) at a rate of 400 µg C g−1 soil was mixed with 25 g of each homogenized soil sample in 32 oz mason jars (946.3 mL) with septa in the lids. Soil samples that did not rewet to maximum water-holding capacity (WHC) from control, D, F, and D + F plots were brought to 20 % WHC with the addition of the glucose solution, with laboratory replications yielding n=4. To examine the effects of rewetting, additional soil samples from each field treatment were rehydrated with a glucose solution to maximum soil WHC (n=4). Additional control soils were incubated without the addition of glucose and received the same amount of deionized water as non-rewet samples as a non-amended control, bringing the total to 48 jar incubations. Soil microcosms were well mixed with water or substrate solution and incubated for 1 week at room temperature. During this time the productions of 13CO2 and total CO2 were assayed every other day by taking gas samples from the microcosm headspace through the septa and inserting them into 12 mL Exetainer vials (Labco Limited). After gas samples were taken, jars were opened for ∼ 20 min to allow for gas exchange. After the experiment was complete, Exetainer vials were shipped to West Virginia University, where each gas sample was measured using an LI-6400 (LI-COR Biosciences, Lincoln, NE, USA) and a Picarro G2201-i (Picarro Inc., Santa Clara, CA, USA). Glucose- and soil-organic-matter-derived CO2–C was calculated via mass balance as described in Morrissey et al. (2017). Priming was then calculated as the difference in soil organic matter CO2–C between the microcosms that received substrate solution and those that received water. At the cessation of the incubation, total microbial biomass was obtained by a chloroform fumigation method (Witt et al., 2000). Briefly, 8 g of soil was suspended in 45 mL of 0.1 M K2SO4 with or without an additional 1 mL of ethanol-free chloroform and shaken for 4 h (chloroform) or 2 h (no chloroform) and filtered (90 mm GF/A filter paper). Extracts were stored at −20 ∘C until dissolved organic carbon was oxidized to CO2 via a persulfate digestion (Doyle et al., 2004). Digestion efficiency was determined using a standard curve of yeast extract solution ranging from 0 to 200 mg C L−1. The concentration and isotopic enrichment of the resulting CO2 gas were measured on the Picarro G2201-i (Picarro Inc., Santa Clara, CA, USA). Total and substrate-derived microbial biomass – C was calculated as the difference in C (mg) between chloroformed and non-chloroformed soil extracts. Microbial CUE was calculated as substrate-derived biomass – C divided by the total carbon consumed (substrate-derived CO2–C and biomass – C), where we distinguished substrate-derived microbial biomass C from total microbial biomass by using the atom percent 13C to calculate the total amount of 13C-labeled biomass per gram of dry soil (Kane et al., 2023).
2.5 Ecosystem productivity
To quantify total net primary productivity (NPP) (), we summed total litterfall, wood, and root production in each plot for a given year. To estimate wood production, we calculated stem aboveground biomass (AGB) using allometric equations for tropical tree species (Chave et al., 2014). For the allometric equations, we measured the height of each stem using a Haglöf EC II-D electronic clinometer (Haglof Inc., Madison, MS, USA) and obtained wood density data from a functional trait database for the TDF of Guanacaste (Powers and Tiffin, 2010). Mean wood density was substituted for species without wood density data. Annual woody productivity then represented the sum of biomass increments from trees newly recruited into the 2.5 cm DBH size class plus biomass gain from increased diameters in planted and existing recruited trees. Additionally, we calculated aboveground net primary productivity (ANPP) by summing only woody and litterfall productivity and the aboveground : belowground productivity ratios (AGB : BGB) by dividing ANPP by the root production in each plot for each year.
2.6 Statistical analysis
To test whether the throughfall exclusion structures affected soil moisture, we performed a linear mixed model with the change in soil moisture for a given plot as the response variable, the presence of the throughfall exclusion structure and the weekly time points from January 2017 to December 2020 as fixed effects, and probe nested within plot nested within stand as a random intercept. This approach allowed us to test the effect on soil moisture after the onset of the throughfall exclusion structures (Reid et al., 2015) while also accounting for the intra-annual variation in soil moisture typical of the TDF (Schwartz et al., 2022). We ran separate models for each depth (10 and 40 cm) and for the wet season and dry season due to the strong rainfall seasonality. To obtain the change in soil moisture per plot, we divided the observation time into two periods, a pretreatment (May 2016 to late August 2016), which consisted of wet-season soil moisture data before the shelters were set up, and an experimental period (January 2017 to December 2020). We excluded from this analysis the data collected between September and December 2016 as we finished establishing the rainout shelters 3 months into the rainy season. After removing outliers using the interquartile method, we calculated the median pretreatment soil moisture (SMPT, m3 m−3) for each probe in each plot. We then calculated the treatment effect as the percent change between each soil moisture observation (SMi, m3 m−3) and the SMPT. To investigate interannual variation in wet-season soil moisture, we fitted additional linear mixed models to test whether soil moisture in plots without throughfall exclusion varied as a function of year and depth with the probe nested within the plot nested within stands as a random intercept. In both cases, we calculated type-III sum squares and the F value for each model and performed Tukey's honest significance difference test (Tukey's HSD) for multiple comparisons.
We tested the effects of the experimental treatments on aboveground and belowground ecosystem processes by fitting a series (one for each response variable) of a two-factorial linear mixed-effect model. For tree diameter RGR, we studied responses by understory and plantation trees separately due to differences in the life history of individuals and the possible biases in growth associated with tree size (Iida et al., 2014). Moreover, in addition to the treatment effects, we quantified the effects of two plant functional type classifications. For this, we fitted a model that included leaf phenology (e.g., deciduous and evergreen) and a model that included whether the species was a nitrogen fixer or not. Functional types are linked to physiological differences among tree species (Vargas G. et al., 2021; Powers and Tiffin, 2010; Vargas G. et al., 2015) and are important drivers explaining tree growth responses to nutrient additions and water availability (Waring et al., 2019; da Costa et al., 2010; Wright et al., 2011; Toro et al., 2022). In these models, RGR was the response variable, and drought, fertilizer, and functional type were the predictors. Additionally, we included the species' identities of each stem nested within the plot nested within the stand as random effects. In the case of biomass fluxes, microbial CUE, and LAI-derived metrics, these processes (e.g., total litterfall) were the response variables, the drought treatment was one factor, and the fertilizer treatment was the second factor; we included their interaction and the experimental unit (e.g., litterfall basket) nested within the plot nested within stands as a random intercept. With these models, we were able to estimate the main effect of drought, the main effect of fertilization, and the interaction between drought and fertilization while also accounting for the effects of the plantation stand and the plot and, in the case of RGR, plant functional type. We then calculated type-III sum squares and the F value for each model in an analysis of variance (ANOVA) given our unbalanced design and used Tukey's HSD test for multiple comparisons. To analyze the response of CUE from soils that were held at field soil moisture to soils that were rewet, we calculated the natural log response ratio (i.e., ln (RR)), defined here as the mean of the rewet soils' CUE divided by the mean CUE of the field soil moisture soils. Values of ln (RR) below 0 indicate a decline in CUE to rewetting. All data management and statistical analyses were done using R software for statistical computing version 3.6.3 (R Core Team, 2021) and the packages mcvg (Wood, 2004, 2011), nlme (Pinheiro et al., 2019), car (Fox and Weisberg, 2019), and tidyverse (Wickham et al., 2019).
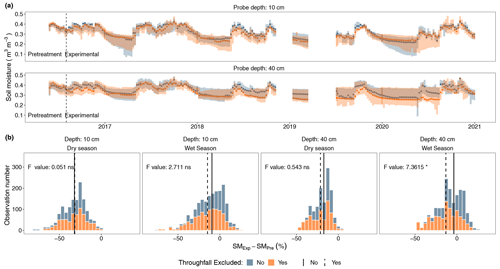
Figure 2Volumetric soil moisture records at two depths for plots with a throughfall exclusion structure and plots without it. (a) Temporal variability at a weekly resolution median volumetric soil moisture with its associated 75th and 25th percentiles, where the dashed vertical line represents the date when the structures were established. (b) Histogram distribution of the percent difference between the soil moisture during the experiment (SMExp) and the soil moisture preceding the experimental treatments (SMPre) for each depth during the dry season and the wet season, where the vertical lines represent the median SMExp − SMPre percent value for plots with a throughfall exclusion structure (dashed) and plots without it (continuous). Reported results from a linear mixed-effect model comparing weekly SMExp − SMPre percent values for each depth during the dry and wet seasons.
3.1 Soil moisture and fertilization
At 40 cm depth, we found evidence (p < 0.05) of a ∼ 13 % reduction in soil moisture as a function of the pretreatment period in the plots with a throughfall exclusion structure, contrary to a weak ∼ 4 % reduction in soil moisture in the plots without throughfall exclusion (Fig. 2). At 10 cm we observed an average change of −15 % in throughfall exclusion plots, but this was not significantly different when compared to the non-drought plots (−9.43 %) (Fig. 2). In both plots with throughfall exclusion and plots without exclusion there was strong evidence (p < 0.001), across all depths and in both seasons, that soil moisture increased or decreased following seasonal rainfall patterns. Weekly median soil moisture values in the throughfall exclusion plots oscillated between 0.21–0.42 m3 m−3 at 10 cm depth and 0.25–0.44 m3 m−3 at 40 cm depth compared to 0.22–0.43 m3 m−3 at 10 cm depth and 0.25–0.45 m3 m−3 at 40 cm depth for plots without throughfall exclusion (Fig. 2). Wet-season soil moisture followed the interannual rainfall variability in which the average volumetric water content was around 0.39 m3 m−3 during 2016 and 2017, while it was around 0.32 m3 m−3 from 2018 to 2020 (Fig. S6). At the end of 4 years, extractable soil P increased by 2–3-fold in plots receiving fertilizer, and extractable Fe also increased (Fig. S7); however, none of the other soil chemical variables we measured differed among treatments.
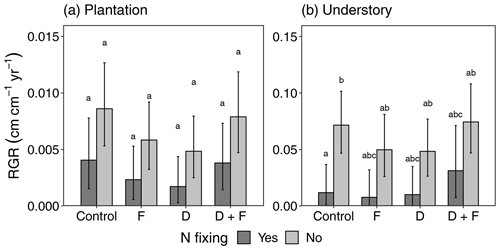
Figure 3Diameter relative growth rate (RGR) responses of plantation (a) and understory (b) trees to fertilization (F), drought (D), and drought plus fertilization (D + F) over a period of 4 years (2016–2020). Bar plots showing the mean RGR with the associated standard error (error bars) were obtained from a total of 194 plantation trees and 462 understory trees in 16 experimental plots. Lowercase letters stand for multiple comparisons among experimental treatments from a post hoc Tukey honest significance difference test.
3.2 Aboveground responses
3.2.1 Tree diameter RGRs
We found no evidence of changes in RGR as a function of D and F additions for either understory (D: F = 0.03, = 1, p = 0.8601; F: F = 0.22, = 1, p = 0.6580) or plantation (D: F = 2.35, = 1, p = 0.1489; F: F = 1.14, = 1, p = 0.3041) trees. We found moderate evidence of an interaction between drought and fertilizer for plantation trees (F = 5.16, = 1, p = 0.0499) but not for understory trees (F = 5.04, = 1, p = 0.0659) (Fig. 3), whereas the effects of fertilization caused an increase in RGR for trees in drought plots and a decrease for trees in non-drought plots (Fig. S8). The nitrogen-fixing plant functional type (PFT) explained the differences in RGRs for understory (F = 21.11, = 1, p = 0.0001) and plantation (F = 4.18, = 1, p = 0.0512) (Fig. 3) trees, with non-N fixers showing higher growth rates than N fixers in both cases. On the other hand, the deciduous PFT showed weaker effects on RGR for plantation trees (F = 3.95, = 1, p = 0.0639) (Table S4). RGR varied idiosyncratically among plantation species in response to the experimental treatments, but we did not find evidence of significant treatment effects (Fig. S9). We also found a higher number of dead trees over the 4 years in plots with experimental manipulations and hence higher biomass losses (Table S5). Mortality, recruitment, and survival for the trees and shrubs that were recruited in the plots did not differ in response to the experimental treatments (Fig. S10).
3.2.2 Canopy productivity
The experimental manipulations showed no effects on fine litter production (drought: F = 0.96, = 1, p = 0.3473; fertilizer: F = 1.33, = 1, p = 0.2724) and the production of leaves (drought: F = 0.64, = 1, p = 0.4404; fertilizer: F = 1.39, = 1, p = 0.2646). Nevertheless, the control plots produced on average 0.69 ± 0.14 kg m−2 of fine litter, which was 12 % lower than in the fertilized plots with 0.78 ± 0.14 , 13 % less than 0.79 ± 0.24 of the drought plots, and 8 % lower than 0.75 ± 0.23 in drought + fertilizer plots. We also found a 40 % decrease in the production of flowers, seeds, and fruits with nutrient additions (F = 4.84, = 1, p = 0.0539) (Fig. S10) but no effects with the throughfall exclusion (F = 1.54, = 1, p = 0.2449). In all the plots LAI increased by ∼ 73 % from the dry season (median LAI: 1.22) to the wet season (median LAI: 5.10). None of the metrics obtained from the LAI measurements changed in response to the experimental manipulations (Fig. S12), the only exception being maximum LAI (LAImax), which we found to be marginally higher for fertilized plots (F = 3.36, = 1, p = 0.0928).
3.3 Belowground responses
3.3.1 Fine-root and nodule production
We found no evidence that differences in the production of fine roots were due to the throughfall exclusions (F = 0.25, = 1, p = 0.6227) or nutrient additions (F = 0.73, = 1, p = 0.4105); despite that, root productivity in the control plots (0.112 ± 0.06 ) was ∼ 15 % less than in the drought plots with 0.133 ± 0.09 , ∼ 27 % less than in the fertilized plots with 0.154 ± 0.09 , and ∼ 24 % less than in the drought + fertilizer plots with 0.149 ± 0.12 . In general, we observed a decrease in the production of nodules in the fertilization treatment (χ2 = 4.95, = 1, p = 0.0262), because only one nodule was observed in plots with nutrient additions during the experimental manipulations. Interestingly, nodule production was similar for drought, drought + fertilizer, and control plots with 69, 57, and 53, respectively (χ2 = 0.03, = 1, p = 0.8589).
3.3.2 Microbial CUE
CUE was ∼ 38 % higher in soils from both the drought (F = 4.31, = 1, p = 0.0621) and fertilized (F = 4.10, = 1, p = 0.0678) plots relative to the control plots (Fig. 4). When the soils were rewet in the laboratory, the CUE exhibited a negative response as quantified by the ln (RR) for both the drought (F = 5.66, = 1, p = 0.0366) and fertilization (F = 0.73, = 1, p = 0.0809) treatments (Fig. 4). There were interaction effects between experimental treatments for both the CUE (F = 5.33, = 1, p = 0.0462) and ln (RR) (F = 4.76, = 1, p = 0.0597), showing evidence of different responses to drought depending on nutrient availability and how CUE was negatively affected by rewetting for drought plots (Fig. 4). Soil priming was similarly influenced by rewetting, and across all the treatments, the soils held at field soil moisture showed negative priming (Fig. S13). Rewetting the soils in the laboratory led to greater soil C priming in the drought plots (F = 5.33, = 1, p = 0.0497) but not in the fertilized plots (F = 0.0191, = 1, p = 0.8932) (Fig. S13).
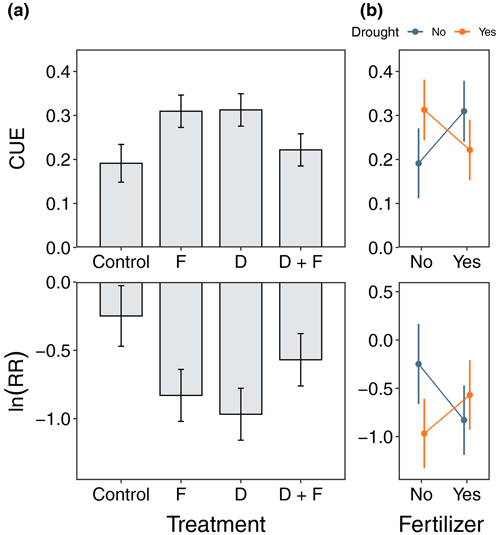
Figure 4Microbial carbon use efficiency (CUE) and the log-response ratio between laboratory rewet and non-rewet samples ln (RR) in control, F, D, and D + F during the wet season of 2019. Panel (a) shows bar plots with the mean response with the associated standard error (n=4), and panel (b) shows interaction plots among experimental treatments. No significant differences were present after performing a post hoc Tukey honest significance difference test, despite the evidence of a moderate effect of F and D on both CUE and ln (RR).
3.4 Ecosystem productivity and biomass allocation
Ecosystem-level fluxes were more responsive to fertilization than to the throughfall exclusion (Fig. 5). NPP increased with nutrient additions (F = 7.86, = 1, p = 0.0178), which led to 17 % and 19 % higher NPP in fertilizer and drought + fertilizer plots, respectively, relative to the control plots (Fig. 5). Although we observed a 14 % NPP increase in the drought plots (F = 5.29, = 1, p = 0.0431), we found no evidence that this was different from the control plots after looking at the multiple comparisons (Fig. 5). Consistently, when considering only ANPP, we found that fertilizer increased the amount of biomass produced (F = 5.81, = 1, p = 0.0362), which was 15 % and 19 % higher for fertilizer and drought + fertilizer plots, respectively, relative to the control plots (Fig. 5). Moreover, the drought treatment increased ANPP (F = 4.58, = 1, p = 0.0575). We found no evidence that the drought (F = 0.30, = 1, p = 0.5960) or fertilizer (F = 0.35, = 1, p = 0.5645) plots allocated more belowground biomass (Fig. 5). We did not observe interaction effects by the experimental treatments in either NPP (F = 1.13, = 1, p = 0.30), ANPP (F = 0.77, = 1, p = 0.3991), or AGB : BGB (F = 0.34, = 1, p = 0.5695), although the response to nutrient additions in the plots without throughfall exclusions was slightly higher for NPP and ANPP relative to plots in the drought treatment (Fig. 5b).
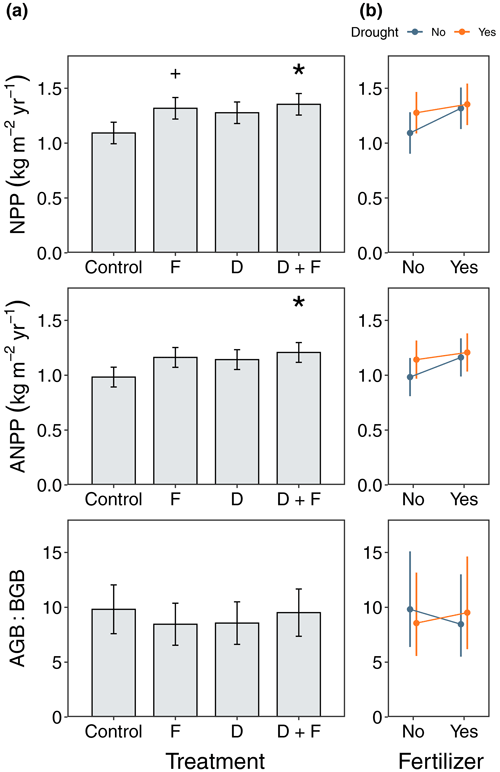
Figure 5Responses of ecosystem net primary productivity (NPP), aboveground net primary productivity (ANPP), and aboveground-to-belowground ratios to F, D, and D + F over a period of 4 years (2016–2020). Panel (a) shows median values for each experimental manipulation with their associated standard error (n=4) with significance values after performing a post hoc Tukey honest significance difference test where p < 0.05 (∗) and p < 0.1. Panel (b) shows the interactions between F and D treatments where for NPP and ANPP there was a greater response of non-drought plots to fertilization.
Here we present the first attempt to experimentally test whether integrated ecosystem responses to rainfall manipulations are limited by nutrient availability in the TDF biome (Beier et al., 2012; Meir et al., 2015). We found that a 13 %–15 % reduction in soil moisture only leads to modest effects in the studied ecosystem processes. By contrast, extractable P increased in the fertilized plots several fold (Fig. S7), causing an increase in primary productivity (both NPP and ANPP) (Fig. 5), a decrease in the nodule production, a decrease in the production of seeds and flowers (Fig. S10), increases in LAImax (Fig. S11), and an increase in CUE when compared to the control plots (Fig. 4). Variations in tree RGRs were mostly due to plant functional types rather than the experimental treatments. However, there was a significant interaction in how understory trees responded to both treatments, leading to a reduction in the differences between N-fixing and non-N-fixing trees (Fig. 3). Collectively, these results suggest that reducing soil moisture by a modest amount is not sufficient to drive large reorganizations in ecosystem processes and that soil nutrient availability mildly modulates short-term changes in productivity. Below, we further explore the implications of these results in the context of how soil fertility could affect tropical ecosystem responses to global environmental change.
4.1 Nutrient and water limitations on ecosystem productivity
In a broad sense, we found that nutrient availability had a stronger control on forest productivity than a ∼ 15 % reduction in soil moisture. While this result does not resonate with the expectation that water availability imposes a greater limitation on productivity across environmental gradients than soil fertility (Harrington et al., 1995; Santiago and Mulkey, 2005; Toledo et al., 2011; Sala et al., 2012; Poorter et al., 2016), it provokes the question to what extent tropical dry forests are resilient to drought stress. Our data point to other aspects related to drought intensity and not soil moisture alone, which could be key factors in how water availability shapes TDF primary productivity (Anderegg et al., 2013). Recent studies from northwestern Costa Rica have shown that abnormal drought stress due to a strong El Niño–Southern Oscillation (ENSO) event in 2015 caused biomass loss due to an increase in tree mortality, a decrease in reproductive biomass production, and reductions in productivity (O'Brien et al., 2018; Castro et al., 2018; Powers et al., 2020). The main characteristics of the 2015 ENSO were the elevated temperatures and a substantial rainfall reduction for the region (Santoso et al., 2017), which can increase the severity of drought effects in forest ecosystems due to increased atmospheric water demand (Brodribb et al., 2020; McDowell et al., 2020). Thus, while throughfall exclusion experiments manipulate soil moisture, it is possible that a combination of factors such as the vapor pressure deficit, the rainfall patterns (intensity and seasonality), and their linkages to soil moisture is a more important aspect of drought stress for forested ecosystems than soil moisture alone.
We observed the strongest experimental signal in the fertilization treatment (F and D + F) regardless of the throughfall reductions. Such responses agree with known evidence of nutrient limitation on productivity in tropical forests (Alvarez-Clare et al., 2013; Wright et al., 2018; Waring et al., 2019; Cunha et al., 2022), which has also been observed in ecosystem models for the TDF (Medvigy et al., 2019). Interestingly, the contribution of each biomass flux to NPP depended on the combined effects of drought and fertilization, with root productivity contributing more in drought plots and woody productivity in fertilized plots (Fig. S14), although the observed changes in woody and root production were not statistically significant when analyzed individually. Changes in root and woody productivity in response to nutrient additions have been observed in secondary wet tropical forests (Wright et al., 2018) and eastern Amazon forests (Cunha et al., 2022). In a nearby secondary TDF, Waring et al. (2019) found no significant effect of nitrogen and/or phosphorus additions on productivity; however, in contrast to that study, our experiment included the additions of both macronutrients and micronutrients (Table S2). Moreover, the increase in productivity as a function of fertilization showed a bigger yet not significant increase without the presence of throughfall structures (Fig. 5b). This trend resembles observed patterns in nearby stands of TDF, where forests in fertile soils are more responsive to increases in rainfall than forests in infertile soils (Becknell et al., 2021). Our results are comparable to other throughfall exclusion experiments in which fine litter production was not affected by the drought treatment (Nepstad et al., 2002; Brando et al., 2006; Schwendenmann et al., 2010), with most of its variation linked to interannual climatic variability rather than the experimental manipulations (Brando et al., 2008).
4.1.1 Canopy dynamics and tree growth
While maximum canopy cover (LAImax) showed a mild increase in the fertilization treatment (p = 0.09), the temporal dynamics of leaf phenology showed no changes (Fig. S12). It is possible that the timing of leaf phenology may also depend on intraspecific and interspecific responses to environmental factors that shape soil water availability, including temperature, atmospheric water demand, and soil water retention. For example, the tree species Coussarea racemosa A. Rich modified its vegetative and reproductive phenology in response to a rainfall manipulation in the eastern Amazon (Brando et al., 2006), while at the forest level changes were observed in LAImax but not the timing of leaf production (Brando et al., 2008). However, the opposite was observed in a fertilization experiment in the same region, where nutrient additions reduced leaf lifespan and had no effects on LAImax (Cunha et al., 2022). In a throughfall exclusion experiment combined with fertilization in loblolly pine (Pinus taeda L.) plantations, there were no changes in the LAImax in response to rainfall reduction but an increase in the LAImax in the fertilized plots (Samuelson et al., 2014), which is qualitatively consistent with our data. This body of knowledge suggests that the effects of experimental manipulations on canopy cover might be context-dependent.
No species showed significant changes in RGR, but the understory trees showed a reduction in the differences between N-fixing and non-N-fixing trees. For F and D this was due to a reduction in growth rates by non-N-fixing trees and for D + F due to an increase in the growth rates by N-fixing trees (Fig. 4). One possible reason for these patterns could be increased resource availability due to decreased competition. The D + F plots in which these three species were present experienced the highest biomass losses due to mortality during the 4 years of experimental manipulation (Table S5; Fig. S10). Even though it is hard to determine the cause of death, an increase in growth rates of understory trees has been observed after the mortality of larger trees (Rowland et al., 2015). The lack of responsiveness in the F and D plots, in addition to the biomass losses in some of the D + F plots (Table S5), supports the idea that the availability of resources such as light could be the cause of higher RGR in the D + F compared to the other treatments (Fig. S15). The lowest RGRs were found in plots with the D treatment, with the strongest experimental effect on D. retusa, E. cyclocarpum, and S. glauca (Fig. S9). While not significant, these results are very similar to what has been found in other tropical throughfall exclusion experiments (Meir et al., 2015), in which there is an overall negative effect on tree diameter growth by a decrease in soil moisture.
4.1.2 Belowground responses
The fertilized plots showed only one nodule during the length of the experiment. This observed trend suggests that fertilizer addition alleviates nutrient limitations for legumes (Toro et al., 2022) and confirms the facultative nature of nodulation (Barron et al., 2011). While not statistically significant, nodule production was the highest for both drought treatments (D and D + F). In part, a decrease in soil moisture slows down the rate of nitrogen mineralization and limits plant nutrient uptake (Borken and Matzner, 2009; He and Dijkstra, 2014). Comparable to our results, the legume species Robinia pseudoacacia L. also increased nodulation in a drought experiment (Wurzburger and Miniat, 2014). Moreover, trees tend to rely more on deeper water sources with less access to nutrients (Querejeta et al., 2021). This allocation of root biomass might also enhance nodulation in legumes as there might be changes in the vertical profile of nutrients in the soil, particularly in arid environments where plants can have deep roots (Tumber-Dávila et al., 2022). However, the lack of data on root production beyond the top 15 cm in our experiment makes it hard to confirm that this is the case.
Our soil incubation results suggest that global environmental change has the potential to alter microbial CUE and the susceptibility of soil carbon to pulse rainfall events in tropical dry forests. After 3 years of treatment, soil microbes in the D and F soils had significant increases in glucose-based quantifications of CUE (Fig. 4). Increases in CUE are commonly attributed to shifts in the microbial community (Domeignoz-Horta et al., 2020), changes in the availability of soil C (Morris et al., 2022), or a reduction in carbon investment by microbes in enzymes to fuel the nutrient acquisition (Manzoni et al., 2012). In this experiment, however, the increases in CUE in the D and F soils but not the D + F soils hinder our ability to narrow down which of these mechanisms may be driving our results. Quantifying the shifts in microbial community composition and the identity of microbes that are active decomposers may shed light on the mechanistic underpinning of the CUE response observed here. Importantly, these differences in CUE across treatments also appeared to impact the response of the soils to large, simulated rainfall events. Regardless of treatment, rewetting the soils to water-holding capacity led to a large reduction in CUE (Fig. 4). While not statistically significant, there was a clear trend of greater CUE declines in the treatment soils, particularly the D soils. This trend suggests that, when large rainfall events occur in disturbed soils, a decrease in microbial CUE could potentially lead to a stronger Birch effect and enhance the soil C loss (Schimel, 2018). In support, we found that rewetting the soils also led to the glucose addition driving greater priming of soil carbon losses, a result that was particularly pronounced for the D soils (Fig. S13). By contrast, the glucose addition in soils that were held under field soil moisture conditions led to the net mineralization of soil C by the microbial community. Collectively, our soil incubation results highlight a critical need for more research on the potential for global change to lead to shifts in microbial community composition and traits in TDFs.
4.2 Conclusions
Our results highlight that forest productivity is sensitive to soil fertility and that this might interact with changes in soil moisture. However, despite adding both macronutrients and micronutrients, our results confirm that the short-term responses of tropical dry forest trees to fertilization treatments are modest at best, contrary to the observed strong responses in nutrient-depleted eastern Amazon forests (Cunha et al., 2022). At the same time, the nodulation data indicate that there might be a tight coupling between nutrient availability and water availability in this system. Studying the role of soil moisture in plant nutrient acquisition dynamics remains a largely unexplored avenue in TDF ecology. Considering the observed patterns, a total throughfall exclusion will be necessary to cause soil moisture to decrease by more than 15 %, and manipulations of the atmospheric water demand (e.g., vapor pressure deficit) could help to improve our understanding of drought in tropical forests. Moreover, little is known about how these belowground processes interact with microbial community dynamics, such as CUE, also affected by nutrient additions or reductions in soil moisture. Beyond these processes, disentangling the causes and consequences of colimitation by water and nutrients in productivity could help to elucidate how future climatic conditions will affect carbon cycling in the TDF.
The data that support the findings presented in this study and the R scripts used for statistical analyses can be found in the following Dryad Digital Repository: https://doi.org/10.5061/dryad.5x69p8d6r (Vargas G. et al., 2023).
The supplement related to this article is available online at: https://doi.org/10.5194/bg-20-2143-2023-supplement.
GVG, JSP, BGW, and DM conceptualized the study and designed the experiment. GVG, JSP, BGW, NR, EM, and EB, designed the sampling scheme. GVG, DPAv, DPAr, LDPA, JTT, and NR performed the investigation with the supervision of JSP, EM, and EB. GVG and NR curated and analyzed the data with input from JSP, BGW, and EB. GVG wrote the original draft with input from NR. All the coauthors contributed to the review and editing of subsequent drafts.
At least one of the (co-)authors is a member of the editorial board of Biogeosciences. The peer-review process was guided by an independent editor, and the authors also have no other competing interests to declare.
Publisher's note: Copernicus Publications remains neutral with regard to jurisdictional claims in published maps and institutional affiliations.
This article is part of the special issue “Ecosystem experiments as a window to future carbon, water, and nutrient cycling in terrestrial ecosystems”. It is not associated with a conference.
We thank the United States Department of Energy for funding through the research grants DE-SC0014363 and DE-SC0020344. We also thank the Explorers Club Washington Group graduate student research grant assigned to Nanette Raczka. We are grateful for the outstanding support in the field by Julio Zúñiga Marín, Laura Toro Gonzáles, Erick Calderón Morales, Pedro Alvarado, Duncan Coles, Caroline Bray, Michelle Monge Velasquez, and Ronny Hernández. We are also grateful for logistical support to Milena Gutiérrez-Leitón and all the staff of the Estación Experimental Forestal Horizontes. Additionally, we thank Joseph S. Wright for constructive feedback on a previous version of this paper. This research was done in accordance with Costa Rica's Ministerio Nacional de Ambiente, Energía y Telecomunicaciones.
This research has been supported by the U.S. Department of Energy (grant nos. DE-SC0014363 and DE-SC0020344).
This paper was edited by Richard Nair and reviewed by Laynara F. Lugli and Kendalynnn Morris.
Aguirre-Gutiérrez, J., Malhi, Y., Lewis, S. L., Fauset, S., Adu-Bredu, S., Affum-Baffoe, K., Baker, T. R., Gvozdevaite, A., Hubau, W., Moore, S., Peprah, T., Ziemińska, K., Phillips, O. L., and Oliveras, I.: Long-term droughts may drive drier tropical forests towards increased functional, taxonomic and phylogenetic homogeneity, Nat. Commun., 11, 3346, https://doi.org/10.1038/s41467-020-16973-4, 2020.
Ahmed, M. A., Sanaullah, M., Blagodatskaya, E., Mason-Jones, K., Jawad, H., Kuzyakov, Y., and Dippold, M. A.: Soil microorganisms exhibit enzymatic and priming response to root mucilage under drought, Soil Biol. Biochem., 116, 410–418, https://doi.org/10.1016/j.soilbio.2017.10.041, 2018.
Allen, K., Dupuy, J. M., Gei, M. G., Hulshof, C., Medvigy, D., Pizano, C., Salgado-Negret, B., Smith, C. M., Trierweiler, A., Bloem, S. J. V., Waring, B. G., Xu, X., and Powers, J. S.: Will seasonally dry tropical forests be sensitive or resistant to future changes in rainfall regimes?, Environ. Res. Lett., 12, 023001, https://doi.org/10.1088/1748-9326/aa5968, 2017.
Alvarez-Clare, S., Mack, M. C., and Brooks, M.: A direct test of nitrogen and phosphorus limitation to net primary productivity in a lowland tropical wet forest, Ecology, 94, 1540–1551, https://doi.org/10.1890/12-2128.1, 2013.
Anderegg, L. D. L., Anderegg, W. R. L., and Berry, J. A.: Not all droughts are created equal: translating meteorological drought into woody plant mortality, Tree Physiol., 33, 672–683, https://doi.org/10.1093/treephys/tpt044, 2013.
Aragão, L. E. O. C., Malhi, Y., Roman-Cuesta, R. M., Saatchi, S., Anderson, L. O., and Shimabukuro, Y. E.: Spatial patterns and fire response of recent Amazonian droughts, Geophys. Res. Lett., 34, L07701, https://doi.org/10.1029/2006GL028946, 2007.
Augusto, L., Achat, D. L., Jonard, M., Vidal, D., and Ringeval, B.: Soil parent material – A major driver of plant nutrient limitations in terrestrial ecosystems, Glob. Change Biol., 23, 3808–3824, https://doi.org/10.1111/gcb.13691, 2017.
Barron, A. R., Purves, D. W., and Hedin, L. O.: Facultative nitrogen fixation by canopy legumes in a lowland tropical forest, Oecologia, 165, 511–520, https://doi.org/10.1007/s00442-010-1838-3, 2011.
Becknell, J. M., Vargas G., G., Pérez-Aviles, D., Medvigy, D., and Powers, J. S.: Above-ground net primary productivity in regenerating seasonally dry tropical forest: Contributions of rainfall, forest age and soil, J. Ecol., 109, 3903–3915, https://doi.org/10.1111/1365-2745.13767, 2021.
Beier, C., Beierkuhnlein, C., Wohlgemuth, T., Penuelas, J., Emmett, B., Körner, C., Boeck, H. de, Christensen, J. H., Leuzinger, S., Janssens, I. A., and Hansen, K.: Precipitation manipulation experiments – challenges and recommendations for the future, Ecol. Lett., 15, 899–911, https://doi.org/10.1111/j.1461-0248.2012.01793.x, 2012.
Borchert, R.: Soil and Stem Water Storage Determine Phenology and Distribution of Tropical Dry Forest Trees, Ecology, 75, 1437–1449, https://doi.org/10.2307/1937467, 1994.
Borken, W. and Matzner, E.: Reappraisal of drying and wetting effects on C and N mineralization and fluxes in soils, Glob. Change Biol., 15, 808–824, https://doi.org/10.1111/j.1365-2486.2008.01681.x, 2009.
Brando, P. M., Ray, D., Nepstad, D., Cardinot, G., Curran, L. M., and Oliveira, R.: Effects of partial throughfall exclusion on the phenology of Coussarea racemosa (Rubiaceae) in an east-central Amazon rainforest, Oecologia, 150, 181–189, https://doi.org/10.1007/s00442-006-0507-z, 2006.
Brando, P. M., Nepstad, D. C., Davidson, E. A., Trumbore, S. E., Ray, D., and Camargo, P.: Drought effects on litterfall, wood production and belowground carbon cycling in an Amazon forest: results of a throughfall reduction experiment, Philos. T. R. Soc. B, 363, 1839–1848, https://doi.org/10.1098/rstb.2007.0031, 2008.
Brando, P. M., Balch, J. K., Nepstad, D. C., Morton, D. C., Putz, F. E., Coe, M. T., Silvério, D., Macedo, M. N., Davidson, E. A., Nóbrega, C. C., Alencar, A., and Soares-Filho, B. S.: Abrupt increases in Amazonian tree mortality due to drought–fire interactions, P. Natl. Acad. Sci. USA, 111, 6347–6352, https://doi.org/10.1073/pnas.1305499111, 2014.
Brodribb, T. J., Powers, J., Cochard, H., and Choat, B.: Hanging by a thread? Forests and drought, Science, 368, 261–266, https://doi.org/10.1126/science.aat7631, 2020.
Castro, S. M., Sanchez-Azofeifa, G. A., and Sato, H.: Effect of drought on productivity in a Costa Rican tropical dry forest, Environ. Res. Lett., 13, 045001, https://doi.org/10.1088/1748-9326/aaacbc, 2018.
Chadwick, R., Good, P., Martin, G., and Rowell, D. P.: Large rainfall changes consistently projected over substantial areas of tropical land, Nat. Clim. Change, 6, 177–181, https://doi.org/10.1038/nclimate2805, 2016.
Chave, J., Réjou-Méchain, M., Búrquez, A., Chidumayo, E., Colgan, M. S., Delitti, W. B. C., Duque, A., Eid, T., Fearnside, P. M., Goodman, R. C., Henry, M., Martínez-Yrízar, A., Mugasha, W. A., Muller-Landau, H. C., Mencuccini, M., Nelson, B. W., Ngomanda, A., Nogueira, E. M., Ortiz-Malavassi, E., Pélissier, R., Ploton, P., Ryan, C. M., Saldarriaga, J. G., and Vieilledent, G.: Improved allometric models to estimate the aboveground biomass of tropical trees, Glob. Change Biol., 20, 3177–3190, https://doi.org/10.1111/gcb.12629, 2014.
Chazdon, R. L., Redondo Brenes, A., and Vilchez Alvarado, B.: Effects of Climate and Stand Age on Annual Tree Dynamics in Tropical Second-Growth Rain Forests, Ecology, 86, 1808–1815, https://doi.org/10.1890/04-0572, 2005.
Clark, D. B. and Clark, D. A.: Landscape-scale variation in forest structure and biomass in a tropical rain forest, Forest Ecol. Manag., 137, 185–198, https://doi.org/10.1016/S0378-1127(99)00327-8, 2000.
Coley, P. D.: Possible Effects of Climate Change on Plant/Herbivore Interactions in Moist Tropical Forests, Climatic Change, 39, 455–472, https://doi.org/10.1023/A:1005307620024, 1998.
Condit, R., Engelbrecht, B. M. J., Pino, D., Pérez, R., and Turner, B. L.: Species distributions in response to individual soil nutrients and seasonal drought across a community of tropical trees, P. Natl. Acad. Sci. USA, 110, 5064–5068, https://doi.org/10.1073/pnas.1218042110, 2013.
Cotrufo, M. F., Wallenstein, M. D., Boot, C. M., Denef, K., and Paul, E.: The Microbial Efficiency-Matrix Stabilization (MEMS) framework integrates plant litter decomposition with soil organic matter stabilization: do labile plant inputs form stable soil organic matter?, Glob. Change Biol., 19, 988–995, https://doi.org/10.1111/gcb.12113, 2013.
Cunha, H. F. V., Andersen, K. M., Lugli, L. F., Santana, F. D., Aleixo, I. F., Moraes, A. M., Garcia, S., Di Ponzio, R., Mendoza, E. O., Brum, B., Rosa, J. S., Cordeiro, A. L., Portela, B. T. T., Ribeiro, G., Coelho, S. D., de Souza, S. T., Silva, L. S., Antonieto, F., Pires, M., Salomão, A. C., Miron, A. C., de Assis, R. L., Domingues, T. F., Aragão, L. E. O. C., Meir, P., Camargo, J. L., Manzi, A. O., Nagy, L., Mercado, L. M., Hartley, I. P., and Quesada, C. A.: Direct evidence for phosphorus limitation on Amazon forest productivity, Nature, 608, 558–562, https://doi.org/10.1038/s41586-022-05085-2, 2022.
da Costa, A. C. L., Galbraith, D., Almeida, S., Portela, B. T. T., da Costa, M., de A. S. Junior, J., Braga, A. P., de Gonçalves, P. H. L., de Oliveira, A. A., Fisher, R., Phillips, O. L., Metcalfe, D. B., Levy, P., and Meir, P.: Effect of 7 yr of experimental drought on vegetation dynamics and biomass storage of an eastern Amazonian rainforest, New Phytol., 187, 579–591, https://doi.org/10.1111/j.1469-8137.2010.03309.x, 2010.
Domeignoz-Horta, L. A., Pold, G., Liu, X.-J. A., Frey, S. D., Melillo, J. M., and DeAngelis, K. M.: Microbial diversity drives carbon use efficiency in a model soil, Nat. Commun., 11, 3684, https://doi.org/10.1038/s41467-020-17502-z, 2020.
Doughty, C. E. and Goulden, M. L.: Seasonal patterns of tropical forest leaf area index and CO2 exchange, J. Geophys. Res.-Biogeo., 113, G00B06, https://doi.org/10.1029/2007JG000590, 2008.
Doyle, A., Weintraub, M. N., and Schimel, J. P.: Persulfate Digestion and Simultaneous Colorimetric Analysis of Carbon and Nitrogen in Soil Extracts, Soil Sci. Soc. Am. J., 68, 669–676, https://doi.org/10.2136/sssaj2004.6690, 2004.
Ewert, F. and Pleijel, H.: Phenological development, leaf emergence, tillering and leaf area index, and duration of spring wheat across Europe in response to CO2 and ozone, Eur. J. Agron., 10, 171–184, https://doi.org/10.1016/S1161-0301(99)00008-8, 1999.
Feng, X., Porporato, A., and Rodriguez-Iturbe, I.: Changes in rainfall seasonality in the tropics, Nat. Clim. Change, 3, 811–815, https://doi.org/10.1038/nclimate1907, 2013.
Fox, J. and Weisberg, S.: An 〈R〉 Companion to Applied Regression, 3rd Edn., Sage, Thousand Oaks, CA, 2019.
Frankie, G. W., Baker, H. G., and Opler, P. A.: Comparative Phenological Studies of Trees in Tropical Wet and Dry Forests in the Lowlands of Costa Rica, J. Ecol., 62, 881–919, https://doi.org/10.2307/2258961, 1974.
Frey, S. D., Lee, J., Melillo, J. M., and Six, J.: The temperature response of soil microbial efficiency and its feedback to climate, Nat. Clim. Change, 3, 395–398, https://doi.org/10.1038/nclimate1796, 2013.
Gutiérrez-Leitón, M.: Opciones para reforestación comercial con especies nativas en zonas secas de Costa Rica, Ambientico, 267, 28–31, 2018.
Harrington, R. A., Fownes, J. H., Meinzer, F. C., and Scowcroft, P. G.: Forest growth along a rainfall gradient in Hawaii: Acacia koa stand structure, productivity, foliar nutrients, and water- and nutrient-use efficiencies, Oecologia, 102, 277–284, https://doi.org/10.1007/BF00329794, 1995.
He, M. and Dijkstra, F. A.: Drought effect on plant nitrogen and phosphorus: a meta-analysis, New Phytol., 204, 924–931, https://doi.org/10.1111/nph.12952, 2014.
Hietz, P., Turner, B. L., Wanek, W., Richter, A., Nock, C. A., and Wright, S. J.: Long-Term Change in the Nitrogen Cycle of Tropical Forests, Science, 334, 664–666, https://doi.org/10.1126/science.1211979, 2011.
Hoekstra, J. M., Boucher, T. M., Ricketts, T. H., and Roberts, C.: Confronting a biome crisis: global disparities of habitat loss and protection, Ecol. Lett., 8, 23–29, https://doi.org/10.1111/j.1461-0248.2004.00686.x, 2005.
Hou, E., Luo, Y., Kuang, Y., Chen, C., Lu, X., Jiang, L., Luo, X., and Wen, D.: Global meta-analysis shows pervasive phosphorus limitation of aboveground plant production in natural terrestrial ecosystems, Nat. Commun., 11, 637, https://doi.org/10.1038/s41467-020-14492-w, 2020.
Iida, Y., Poorter, L., Sterck, F., Kassim, A. R., Potts, M. D., Kubo, T., and Kohyama, T. S.: Linking size-dependent growth and mortality with architectural traits across 145 co-occurring tropical tree species, Ecology, 95, 353–363, https://doi.org/10.1890/11-2173.1, 2014.
Kane, J. L., Kotcon, J. B., Freedman, Z. B., and Morrissey, E. M.: Fungivorous nematodes drive microbial diversity and carbon cycling in soil, Ecology, 104, e3844, https://doi.org/10.1002/ecy.3844, 2023.
Kavanagh, T. and Kellman, M.: Seasonal Pattern of Fine Root Proliferation in a Tropical Dry Forest, Biotropica, 24, 157, https://doi.org/10.2307/2388669, 1992.
Knorr, W., Prentice, I. C., House, J. I., and Holland, E. A.: Long-term sensitivity of soil carbon turnover to warming, Nature, 433, 298–301, https://doi.org/10.1038/nature03226, 2005.
Kummerow, J., Castillanos, J., Maas, M., and Larigauderie, A.: Production of fine roots and the seasonality of their growth in a Mexican deciduous dry forest, Vegetatio, 90, 73–80, https://doi.org/10.1007/BF00045590, 1990.
Lewis, S. L.: Tropical forests and the changing earth system, Philos. T. R. Soc. B, 361, 195–210, https://doi.org/10.1098/rstb.2005.1711, 2006.
Liu, X.-J. A., Finley, B. K., Mau, R. L., Schwartz, E., Dijkstra, P., Bowker, M. A., and Hungate, B. A.: The soil priming effect: Consistent across ecosystems, elusive mechanisms, Soil Biol. Biochem., 140, 107617, https://doi.org/10.1016/j.soilbio.2019.107617, 2020.
Lu, X., Vitousek, P. M., Mao, Q., Gilliam, F. S., Luo, Y., Zhou, G., Zou, X., Bai, E., Scanlon, T. M., Hou, E., and Mo, J.: Plant acclimation to long-term high nitrogen deposition in an N-rich tropical forest, P. Natl. Acad. Sci. USA, 115, 5187–5192, https://doi.org/10.1073/pnas.1720777115, 2018.
Lugo, A. E. and Murphy, P. G.: Nutrient dynamics of a Puerto Rican subtropical dry forest, J. Trop. Ecol., 2, 55–72, https://doi.org/10.1017/S0266467400000602, 1986.
Manzoni, S., Taylor, P., Richter, A., Porporato, A., and Ågren, G. I.: Environmental and stoichiometric controls on microbial carbon-use efficiency in soils, New Phytol., 196, 79–91, https://doi.org/10.1111/j.1469-8137.2012.04225.x, 2012.
Matson, P. A., McDowell, W. H., Townsend, A. R., and Vitousek, P. M.: The globalization of N deposition: ecosystem consequences in tropical environments, Biogeochemistry, 46, 67–83, https://doi.org/10.1007/BF01007574, 1999.
McDowell, N. G., Allen, C. D., Anderson-Teixeira, K., Aukema, B. H., Bond-Lamberty, B., Chini, L., Clark, J. S., Dietze, M., Grossiord, C., Hanbury-Brown, A., Hurtt, G. C., Jackson, R. B., Johnson, D. J., Kueppers, L., Lichstein, J. W., Ogle, K., Poulter, B., Pugh, T. A. M., Seidl, R., Turner, M. G., Uriarte, M., Walker, A. P., and Xu, C.: Pervasive shifts in forest dynamics in a changing world, Science, 368, eaaz9463, https://doi.org/10.1126/science.aaz9463, 2020.
Medvigy, D., Wang, G., Zhu, Q., Riley, W. J., Trierweiler, A. M., Waring, B. G., Xu, X., and Powers, J. S.: Observed variation in soil properties can drive large variation in modelled forest functioning and composition during tropical forest secondary succession, New Phytol., 223, 1820–1833, https://doi.org/10.1111/nph.15848, 2019.
Meir, P., Wood, T. E., Galbraith, D. R., Brando, P. M., Da Costa, A. C. L., Rowland, L., and Ferreira, L. V.: Threshold Responses to Soil Moisture Deficit by Trees and Soil in Tropical Rain Forests: Insights from Field Experiments, BioScience, 65, 882–892, https://doi.org/10.1093/biosci/biv107, 2015.
Miles, L., Newton, A. C., DeFries, R. S., Ravilious, C., May, I., Blyth, S., Kapos, V., and Gordon, J. E.: A global overview of the conservation status of tropical dry forests, J. Biogeogr., 33, 491–505, https://doi.org/10.1111/j.1365-2699.2005.01424.x, 2006.
Minorsky, P. V.: The functions of foliar nyctinasty: a review and hypothesis, Biol. Rev., 94, 216–229, https://doi.org/10.1111/brv.12444, 2019.
Morris, K. A., Richter, A., Migliavacca, M., and Schrumpf, M.: Growth of soil microbes is not limited by the availability of nitrogen and phosphorus in a Mediterranean oak-savanna, Soil Biol. Biochem., 169, 108680, https://doi.org/10.1016/j.soilbio.2022.108680, 2022.
Morrissey, E. M., Mau, R. L., Schwartz, E., McHugh, T. A., Dijkstra, P., Koch, B. J., Marks, J. C., and Hungate, B. A.: Bacterial carbon use plasticity, phylogenetic diversity and the priming of soil organic matter, ISME J., 11, 1890–1899, https://doi.org/10.1038/ismej.2017.43, 2017.
Murphy, P. G. and Lugo, A. E.: Ecology of tropical dry forest, Annu. Rev. Ecol. Syst., 17, 67–88, https://doi.org/10.1146/annurev.es.17.110186.000435, 1986.
Nepstad, D. C., Moutinho, P., Dias-Filho, M. B., Davidson, E., Cardinot, G., Markewitz, D., Figueiredo, R., Vianna, N., Chambers, J., Ray, D., Guerreiros, J. B., Lefebvre, P., Sternberg, L., Moreira, M., Barros, L., Ishida, F. Y., Tohlver, I., Belk, E., Kalif, K., and Schwalbe, K.: The effects of partial throughfall exclusion on canopy processes, aboveground production, and biogeochemistry of an Amazon forest, J. Geophys. Res.-Atmos., 107, LBA 53-1–LBA 53-18, https://doi.org/10.1029/2001JD000360, 2002.
Norby, R. J., Sholtis, J. D., Gunderson, C. A., and Jawdy, S. S.: Leaf dynamics of a deciduous forest canopy: no response to elevated CO2, Oecologia, 136, 574–584, https://doi.org/10.1007/s00442-003-1296-2, 2003.
O'Brien, M. J., Peréz-Aviles, D., and Powers, J. S.: Resilience of seed production to a severe El Niño-induced drought across functional groups and dispersal types, Glob. Change Biol., 24, 5270–5280, https://doi.org/10.1111/gcb.14416, 2018.
Oliveira, R. S., Eller, C. B., Barros, F. de V., Hirota, M., Brum, M., and Bittencourt, P.: Linking plant hydraulics and the fast–slow continuum to understand resilience to drought in tropical ecosystems, New Phytol., 230, 904–923, https://doi.org/10.1111/nph.17266, 2021.
Phillips, O. L., Aragão, L. E. O. C., Lewis, S. L., Fisher, J. B., Lloyd, J., López-González, G., Malhi, Y., Monteagudo, A., Peacock, J., Quesada, C. A., Heijden, G. van der, Almeida, S., Amaral, I., Arroyo, L., Aymard, G., Baker, T. R., Bánki, O., Blanc, L., Bonal, D., Brando, P., Chave, J., de Oliveira, Á. C. A., Cardozo, N. D., Czimczik, C. I., Feldpausch, T. R., Freitas, M. A., Gloor, E., Higuchi, N., Jiménez, E., Lloyd, G., Meir, P., Mendoza, C., Morel, A., Neill, D. A., Nepstad, D., Patiño, S., Peñuela, M. C., Prieto, A., Ramírez, F., Schwarz, M., Silva, J., Silveira, M., Thomas, A. S., ter Steege, H., Stropp, J., Vásquez, R., Zelazowski, P., Dávila, E. A., Andelman, S., Andrade, A., Chao, K.-J., Erwin, T., Fiore, A. D., C, E. H., Keeling, H., Killeen, T. J., Laurance, W. F., Cruz, A. P., Pitman, N. C. A., Vargas, P. N., Ramírez-Angulo, H., Rudas, A., Salamão, R., Silva, N., Terborgh, J., and Torres-Lezama, A.: Drought Sensitivity of the Amazon Rainforest, Science, 323, 1344–1347, https://doi.org/10.1126/science.1164033, 2009.
Phillips, R. P., Ibáñez, I., D'Orangeville, L., Hanson, P. J., Ryan, M. G., and McDowell, N. G.: A belowground perspective on the drought sensitivity of forests: Towards improved understanding and simulation, Forest Ecol. Manag., 380, 309–320, https://doi.org/10.1016/j.foreco.2016.08.043, 2016.
Piao, S., Liu, Q., Chen, A., Janssens, I. A., Fu, Y., Dai, J., Liu, L., Lian, X., Shen, M., and Zhu, X.: Plant phenology and global climate change: Current progresses and challenges, Glob. Change Biol., 25, 1922–1940, https://doi.org/10.1111/gcb.14619, 2019.
Pinheiro, J., Bates, D., DebRoy, S., Starkar, S., and R Core Team: nlme: Linear and Nonlinear Mixed Effects Models, https://cran.r-project.org/web/packages/nlme/citation.html (last access: 12 June 2023), 2019.
Pokorný, R., Tomášková, I., and Havránková, K.: Temporal variation and efficiency of leaf area index in young mountain Norway spruce stand, Eur. J. Forest Res., 127, 359–367, https://doi.org/10.1007/s10342-008-0212-z, 2008.
Poorter, L., Bongers, F., Aide, T. M., Almeyda Zambrano, A. M., Balvanera, P., Becknell, J. M., Boukili, V., Brancalion, P. H. S., Broadbent, E. N., Chazdon, R. L., Craven, D., de Almeida-Cortez, J. S., Cabral, G. A. L., de Jong, B. H. J., Denslow, J. S., Dent, D. H., DeWalt, S. J., Dupuy, J. M., Durán, S. M., Espírito-Santo, M. M., Fandino, M. C., César, R. G., Hall, J. S., Hernandez-Stefanoni, J. L., Jakovac, C. C., Junqueira, A. B., Kennard, D., Letcher, S. G., Licona, J.-C., Lohbeck, M., Marín-Spiotta, E., Martínez-Ramos, M., Massoca, P., Meave, J. A., Mesquita, R., Mora, F., Muñoz, R., Muscarella, R., Nunes, Y. R. F., Ochoa-Gaona, S., de Oliveira, A. A., Orihuela-Belmonte, E., Peña-Claros, M., Pérez-García, E. A., Piotto, D., Powers, J. S., Rodríguez-Velázquez, J., Romero-Pérez, I. E., Ruíz, J., Saldarriaga, J. G., Sanchez-Azofeifa, A., Schwartz, N. B., Steininger, M. K., Swenson, N. G., Toledo, M., Uriarte, M., van Breugel, M., van der Wal, H., Veloso, M. D. M., Vester, H. F. M., Vicentini, A., Vieira, I. C. G., Bentos, T. V., Williamson, G. B., and Rozendaal, D. M. A.: Biomass resilience of Neotropical secondary forests, Nature, 530, 211–214, https://doi.org/10.1038/nature16512, 2016.
Powers, J. S. and Tiffin, P.: Plant functional type classifications in tropical dry forests in Costa Rica: leaf habit versus taxonomic approaches, Funct. Ecol., 24, 927–936, https://doi.org/10.1111/j.1365-2435.2010.01701.x, 2010.
Powers, J. S., Vargas G., G., Brodribb, T. J., Schwartz, N. B., Pérez-Aviles, D., Smith-Martin, C. M., Becknell, J. M., Aureli, F., Blanco, R., Calderón-Morales, E., Calvo-Alvarado, J. C., Calvo-Obando, A. J., Chavarría, M. M., Carvajal-Vanegas, D., Jiménez-Rodríguez, C. D., Chacon, E. M., Schaffner, C. M., Werden, L. K., Xu, X., and Medvigy, D.: A catastrophic tropical drought kills hydraulically vulnerable tree species, Glob. Change Biol., 26, 3122–3133, https://doi.org/10.1111/gcb.15037, 2020.
Preece, C. and Peñuelas, J.: Rhizodeposition under drought and consequences for soil communities and ecosystem resilience, Plant Soil, 409, 1–17, https://doi.org/10.1007/s11104-016-3090-z, 2016.
Querejeta, J. I., Ren, W., and Prieto, I.: Vertical decoupling of soil nutrients and water under climate warming reduces plant cumulative nutrient uptake, water-use efficiency and productivity, New Phytol., 230, 1378–1393, https://doi.org/10.1111/nph.17258, 2021.
Quesada-Román, A., Ballesteros-Cánovas, J. A., Granados-Bolaños, S., Birkel, C., and Stoffel, M.: Dendrogeomorphic reconstruction of floods in a dynamic tropical river, Geomorphology, 359, 107133, https://doi.org/10.1016/j.geomorph.2020.107133, 2020.
R Core Team: R: A language and environment for statistical computing, Vienna, Austria, 2021.
Reid, J. P., Schnitzer, S. A., and Powers, J. S.: Short and Long-Term Soil Moisture Effects of Liana Removal in a Seasonally Moist Tropical Forest, PLOS ONE, 10, e0141891, https://doi.org/10.1371/journal.pone.0141891, 2015.
Rowland, L., da Costa, A. C. L., Galbraith, D. R., Oliveira, R. S., Binks, O. J., Oliveira, A. A. R., Pullen, A. M., Doughty, C. E., Metcalfe, D. B., Vasconcelos, S. S., Ferreira, L. V., Malhi, Y., Grace, J., Mencuccini, M., and Meir, P.: Death from drought in tropical forests is triggered by hydraulics not carbon starvation, Nature, 528, 119–122, https://doi.org/10.1038/nature15539, 2015.
Sala, O. E., Gherardi, L. A., Reichmann, L., Jobbágy, E., and Peters, D.: Legacies of precipitation fluctuations on primary production: theory and data synthesis, Philos. T. R. Soc. B, 367, 3135–3144, https://doi.org/10.1098/rstb.2011.0347, 2012.
Samuelson, L. J., Pell, C. J., Stokes, T. A., Bartkowiak, S. M., Akers, M. K., Kane, M., Markewitz, D., McGuire, M. A., and Teskey, R. O.: Two-year throughfall and fertilization effects on leaf physiology and growth of loblolly pine in the Georgia Piedmont, Forest Ecol. Manag., 330, 29–37, https://doi.org/10.1016/j.foreco.2014.06.030, 2014.
Santiago, L. S.: Nutrient limitation of eco-physiological processes in tropical trees, Trees, 29, 1291–1300, https://doi.org/10.1007/s00468-015-1260-x, 2015.
Santiago, L. S. and Mulkey, S. S.: Leaf productivity along a precipitation gradient in lowland Panama: patterns from leaf to ecosystem, Trees, 19, 349–356, https://doi.org/10.1007/s00468-004-0389-9, 2005.
Santoso, A., Mcphaden, M. J., and Cai, W.: The Defining Characteristics of ENSO Extremes and the Strong 2015/2016 El Niño, Rev. Geophys., 55, 1079–1129, https://doi.org/10.1002/2017RG000560, 2017.
Schimel, J., Balser, T. C., and Wallenstein, M.: Microbial Stress-Response Physiology and Its Implications for Ecosystem Function, Ecology, 88, 1386–1394, https://doi.org/10.1890/06-0219, 2007.
Schimel, J. P.: Life in Dry Soils: Effects of Drought on Soil Microbial Communities and Processes, Annu. Rev. Ecol. Evol. Syst., 49, 409–432, https://doi.org/10.1146/annurev-ecolsys-110617-062614, 2018.
Schwartz, N. B., Medvigy, D., Tijerin, J., Pérez-Aviles, D., Rivera-Polanco, D., Pereira, D., Vargas G., G., Werden, L., Du, D., Arnold, L., and Powers, J. S.: Intra-annual variation in microclimatic conditions in relation to vegetation type and structure in two tropical dry forests undergoing secondary succession, Forest Ecol. Manag., 511, 120132, https://doi.org/10.1016/j.foreco.2022.120132, 2022.
Schwendenmann, L., Veldkamp, E., Moser, G., Hölscher, D., Köhler, M., Clough, Y., Anas, I., Djajakirana, G., Erasmi, S., Hertel, D., Leitner, D., Leuschner, C., Michalzik, B., Propastin, P., Tjoa, A., Tscharntke, T., and Straaten, O. V.: Effects of an experimental drought on the functioning of a cacao agroforestry system, Sulawesi, Indonesia, Glob. Change Biol., 16, 1515–1530, https://doi.org/10.1111/j.1365-2486.2009.02034.x, 2010.
Seneviratne, S. I., Corti, T., Davin, E. L., Hirschi, M., Jaeger, E. B., Lehner, I., Orlowsky, B., and Teuling, A. J.: Investigating soil moisture–climate interactions in a changing climate: A review, Earth-Sci. Rev., 99, 125–161, https://doi.org/10.1016/j.earscirev.2010.02.004, 2010.
Smith, N. G., Rodgers, V. L., Brzostek, E. R., Kulmatiski, A., Avolio, M. L., Hoover, D. L., Koerner, S. E., Grant, K., Jentsch, A., Fatichi, S., and Niyogi, D.: Toward a better integration of biological data from precipitation manipulation experiments into Earth system models, Revi. Geophys., 52, 412–434, https://doi.org/10.1002/2014RG000458, 2014.
Swenson, N. G., Hulshof, C. M., Katabuchi, M., and Enquist, B. J.: Long-term shifts in the functional composition and diversity of a tropical dry forest: a 30-yr study, Ecol. Monogr., 90, e01408, https://doi.org/10.1002/ecm.1408, 2020.
Toledo, M., Poorter, L., Peña-Claros, M., Alarcón, A., Balcázar, J., Leaño, C., Licona, J. C., Llanque, O., Vroomans, V., Zuidema, P., and Bongers, F.: Climate is a stronger driver of tree and forest growth rates than soil and disturbance, J. Ecol., 99, 254–264, https://doi.org/10.1111/j.1365-2745.2010.01741.x, 2011.
Toro, L., Pereira-Arias, D., Perez-Aviles, D., Vargas G., G., Soper, F. M., Gutknecht, J., and Powers, J. S.: Phosphorus limitation of early growth differs between nitrogen-fixing and nonfixing dry tropical forest tree species, New Phytol., 237, 766–779, https://doi.org/10.1111/nph.18612, 2022.
Townsend, A. R., Asner, G. P., and Cleveland, C. C.: The biogeochemical heterogeneity of tropical forests, Trends Ecol. Evol., 23, 424–431, https://doi.org/10.1016/j.tree.2008.04.009, 2008.
Tumber-Dávila, S. J., Schenk, H. J., Du, E., and Jackson, R. B.: Plant sizes and shapes above and belowground and their interactions with climate, New Phytol., 235, 1032–1056, https://doi.org/10.1111/nph.18031, 2022.
Vargas G., G., Werden, L. K., and Powers, J. S.: Explaining Legume Success in Tropical Dry Forests Based on Seed Germination Niches: A New Hypothesis, Biotropica, 47, 277–280, https://doi.org/10.1111/btp.12210, 2015.
Vargas G., G., Brodribb, T. J., Dupuy, J. M., González-M., R., Hulshof, C. M., Medvigy, D., Allerton, T. A. P., Pizano, C., Salgado-Negret, B., Schwartz, N. B., Van Bloem, S. J., Waring, B. G., and Powers, J. S.: Beyond leaf habit: generalities in plant function across 97 tropical dry forest tree species, New Phytol., 232, 148–161, https://doi.org/10.1111/nph.17584, 2021.
Vargas G., G., Pérez-Aviles, D., Raczka, N., Pereira-Arias, D., Tijerín-Triviño, J., Medvigy, D., Waring, B. G., Morrisey, E., Brzostek, E., and Powers, J. S.: Throughfall exclusion and fertilization effects on tropical dry forest tree plantations, a large-scale experiment, Dryad [data set], https://doi.org/10.5061/dryad.5x69p8d6r, 2023.
Wang, R., Goll, D., Balkanski, Y., Hauglustaine, D., Boucher, O., Ciais, P., Janssens, I., Penuelas, J., Guenet, B., Sardans, J., Bopp, L., Vuichard, N., Zhou, F., Li, B., Piao, S., Peng, S., Huang, Y., and Tao, S.: Global forest carbon uptake due to nitrogen and phosphorus deposition from 1850 to 2100, Glob. Change Biol., 23, 4854–4872, https://doi.org/10.1111/gcb.13766, 2017.
Waring, B. G., Gei, M. G., Rosenthal, L., and Powers, J. S.: Plant–microbe interactions along a gradient of soil fertility in tropical dry forest, J. Trop. Ecol., 32, 314–323, https://doi.org/10.1017/S0266467416000286, 2016.
Waring, B. G., Pérez-Aviles, D., Murray, J. G., and Powers, J. S.: Plant community responses to stand-level nutrient fertilization in a secondary tropical dry forest, Ecology, 100, e02691, https://doi.org/10.1002/ecy.2691, 2019.
Waring, B. G., Guzman, M. E. D., Du, D. V., Dupuy, J. M., Gei, M., Gutknecht, J., Hulshof, C., Jelinski, N., Margenot, A. J., Medvigy, D., Pizano, C., Salgado-Negret, B., Schwartz, N. B., Trierweiler, A. M., Bloem, S. J. V., Vargas G., G., and Powers, J. S.: Soil biogeochemistry across Central and South American tropical dry forests, Ecol. Monogr., 91, e01453, https://doi.org/10.1002/ecm.1453, 2021.
Werden, L. K., Becknell, J. M., and Powers, J. S.: Edaphic factors, successional status and functional traits drive habitat associations of trees in naturally regenerating tropical dry forests, Funct. Ecol., 32, 2766–2776, https://doi.org/10.1111/1365-2435.13206, 2018a.
Werden, L. K., Alvarado, P., Zarges, S., Calderón M., E., Schilling, E. M., Gutiérrez L., M., and Powers, J. S.: Using soil amendments and plant functional traits to select native tropical dry forest species for the restoration of degraded Vertisols, J. Appl. Ecol., 55, 1019–1028, https://doi.org/10.1111/1365-2664.12998, 2018b.
Werden, L. K., Calderón-Morales, E., J, P. A., L, M. G., Nedveck, D. A., and Powers, J. S.: Using large-scale tropical dry forest restoration to test successional theory, Ecol. Appl., 30, e02116, https://doi.org/10.1002/eap.2116, 2020.
Wickham, H., Averick, M., Bryan, J., Chang, W., McGowan, L. D., François, R., Grolemund, G., Hayes, A., Henry, L., Hester, J., Kuhn, M., Pedersen, T. L., Miller, E., Bache, S. M., Müller, K., Ooms, J., Robinson, D., Seidel, D. P., Spinu, V., Takahashi, K., Vaughan, D., Wilke, C., Woo, K., and Yutani, H.: Welcome to the Tidyverse, Journal of Open Source Software, 4, 1686, https://doi.org/10.21105/joss.01686, 2019.
Witt, C., Gaunt, J. L., Galicia, C. C., Ottow, J. C. G., and Neue, H.-U.: A rapid chloroform-fumigation extraction method for measuring soil microbial biomass carbon and nitrogen in flooded rice soils, Biol. Fert. Soils, 30, 510–519, https://doi.org/10.1007/s003740050030, 2000.
Wood, S. N.: Stable and Efficient Multiple Smoothing Parameter Estimation for Generalized Additive Models, J. Am. Stat. Assoc., 99, 673–686, https://doi.org/10.1198/016214504000000980, 2004.
Wood, S. N.: Fast stable restricted maximum likelihood and marginal likelihood estimation of semiparametric generalized linear models, J. R. Stat. Soc. B, 73, 3–36, https://doi.org/10.1111/j.1467-9868.2010.00749.x, 2011.
Wright, S. J.: Tropical forests in a changing environment, Trends Ecol. Evol., 20, 553–560, https://doi.org/10.1016/j.tree.2005.07.009, 2005.
Wright, S. J., Yavitt, J. B., Wurzburger, N., Turner, B. L., Tanner, E. V. J., Sayer, E. J., Santiago, L. S., Kaspari, M., Hedin, L. O., Harms, K. E., Garcia, M. N., and Corre, M. D.: Potassium, phosphorus, or nitrogen limit root allocation, tree growth, or litter production in a lowland tropical forest, Ecology, 92, 1616–1625, https://doi.org/10.1890/10-1558.1, 2011.
Wright, S. J., Turner, B. L., Yavitt, J. B., Harms, K. E., Kaspari, M., Tanner, E. V. J., Bujan, J., Griffin, E. A., Mayor, J. R., Pasquini, S. C., Sheldrake, M., and Garcia, M. N.: Plant responses to fertilization experiments in lowland, species-rich, tropical forests, Ecology, 99, 1129–1138, https://doi.org/10.1002/ecy.2193, 2018.
Wurzburger, N. and Miniat, C. F.: Drought enhances symbiotic dinitrogen fixation and competitive ability of a temperate forest tree, Oecologia, 174, 1117–1126, https://doi.org/10.1007/s00442-013-2851-0, 2014.
Xu, X., Medvigy, D., Powers, J. S., Becknell, J. M., and Guan, K.: Diversity in plant hydraulic traits explains seasonal and inter-annual variations of vegetation dynamics in seasonally dry tropical forests, New Phytol., 212, 80–95, https://doi.org/10.1111/nph.14009, 2016.