the Creative Commons Attribution 4.0 License.
the Creative Commons Attribution 4.0 License.
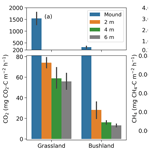
Carbon dioxide and methane fluxes from mounds of African fungus-growing termites
Risto Vesala
Petri Rönnholm
Laura Arppe
Petra Manninen
Markus Jylhä
Jouko Rikkinen
Petri Pellikka
Janne Rinne
Termites play an essential role in decomposing dead plant material in tropical ecosystems and are thus major sources of gaseous C emissions in many environments. In African savannas, fungus-growing termites are among the ecologically most influential termite species. We studied the gas exchange from mounds of two closely related fungus-growing species (Macrotermes subhyalinus and M. michaelseni, respectively) in two habitats representing different vegetation types (grassland, bushland) together with soil fluxes around the mounds. The fluxes from active termite mounds varied from 120 to 2100 mg CO2–C m−2 h−1 for carbon dioxide (CO2) and from 0.06 to 3.7 mg CH4–C m−2 h−1 for methane (CH4) fluxes. Mound CO2 fluxes varied seasonally with a 64 % decrease and 41 % increase in the fluxes from the dry to wet season at the grassland and bushland sites, respectively. During the wet season, the CO2 fluxes were significantly correlated with termite mound volume. The diurnal measurements from two M. michaelseni mounds suggest that the gas fluxes peak during the daytime, possibly reflecting changes in mound internal air circulation. Soil fluxes of both CO2 and CH4 were enhanced at up to 2 m distance from the mounds compared to the local soil respiration, indicating that, in addition to mound ventilation structures, a small proportion of the metabolic gases produced also leave the nest via surrounding soils.
- Article
(3686 KB) - Full-text XML
-
Supplement
(917 KB) - BibTeX
- EndNote
Termites are major degraders of dead organic plant matter in many tropical and subtropical ecosystems (Bignell and Eggleton, 2000; Jouquet et al., 2011), also making them a significant source of atmospheric carbon dioxide (CO2) and methane (CH4) (Zimmerman et al., 1982; Khalil et a., 1990). Especially in African dry savannas, the fungus-growing species comprise the most common and ecologically important termite group that may recycle a quarter of total dead plant matter and even 90 % of the woody litter in some arid ecosystems (Collins, 1981; Buxton, 1981). Fungus-growing termites cultivate symbiotic termite fungi (Termitomyces spp., Basidiomycota) in fungus combs, which are specialized structures within underground nest chambers. The combs are built from feces of termite workers and consist of largely undigested plant matter, initially ingested by foraging termites, that the Termitomyces further degrades (Rouland-Lefèvre, 2000; Vesala et al., 2022a). Eventually, termite hosts get their energy and nutrition from the degraded plant matter and/or the fungal mycelium (Sieber and Leuthold, 1981; Hyodo et al., 2003; Vesala et al., 2019a).
Fungus cultivation by termites is always associated with a complex caste system including numerous workers, soldiers and reproductive individuals, and the total number of termites within a colony typically ranges from hundreds of thousands to up to a few million individuals (Darlington, 1986, 1990). Such large insect populations are notable sources of CO2 already in themselves, but, within nests of fungus-growing termites, a major proportion of the nest CO2 emission is produced by the extensive fungus gardens whose total biomass in mature termite colonies may exceed 20 kg (Darlington, 1986; Darlington et al., 1997; Noirot and Darlington, 2000). Due to their methanogenic gut symbionts, termites are also among the major sources of atmospheric CH4 although estimates about their contribution to the global CH4 budget vary highly (currently considered to represent 1 %–4 % of the natural sources) due to uncertainties in methane-related processes and global termite biomass (Sanderson, 1996; Kirschke et al., 2013; Saunois et al., 2020). Methane can also be oxidized by soil methanotrophic microbes occurring within and around termite nests (Chiri et al., 2020). It has been evaluated that methane-oxidizing bacteria can mitigate even half of the methane produced in nests of some mound-building termite species (Sugimoto et al., 1998; Nauer et al., 2018).
Maintenance of large termite populations and the extensive fungal gardens necessitates effective gas exchange and stable conditions within the nest interior. Termites of the genera Macrotermes and Odontotermes build aboveground soil mounds to stabilize temperature, maintain humidity and enhance gas exchange (Noirot and Darlington, 2000; Korb, 2003, 2011). Mound structure can vary a lot depending on termite species and environmental conditions (Korb and Linsenmair, 1998; Korb, 2003, 2011). Even closely related species may rely on fundamentally different mechanisms of nest ventilation, as in cases of Kenyan Macrotermes michaelseni and Macrotermes subhyalinus, which build closed and open mounds, respectively (Darlington 1984, 1985). The open M. subhyalinus mounds have multiple large ventilation shafts at different elevations on the mound surface that promote the wind-induced Venturi effect, resulting in airflow through the shafts that ventilate the underground nest (Weir, 1973; Korb, 2011). The closed mounds of M. michaelseni lack these openings, and, instead, the air is circulated internally within the mound until the gas exchange between the nest interior and ambient air takes place through the porous mound surface (Noirot and Darlington, 2000; Ocko et al., 2017). Direct measurements of air velocities and temperatures within closed mounds have shown that air moves in convective cells following a diurnally oscillating thermal schedule (King et al., 2015; Ocko et al., 2017).
Despite the well-recognized importance of termite mounds as significant sources of greenhouse gases, field studies quantifying gas fluxes from termite mounds are rare and often limited to species that build relatively small mounds. Previous CO2 flux measurements from termite mounds have ranged from 40 to 2200 mg CO2–C m−2 h−1 (van Asperen et al., 2021; Jamali et al., 2013; Brümmer et al., 2009). For wood-feeding termites in Australia, the seasonal variability in CO2 and CH4 was high with larger fluxes occurring during the wet season (Jamali et al., 2013). For soil- and wood-feeding termites, the relationship between mound CO2 and CH4 fluxes has been species-specific (Jamali et al., 2013). With the assistance of Termitomyces symbionts, fungus-growing termites can utilize widely different types of plant matter including grasses, leaf litter and dead wood (Collins, 1981; Lepage, 1981a), and the proportion of utilized food sources may vary both geographically and seasonally (Boutton et al., 1983; Lepage et al., 1993; Vesala et al., 2022b). Alternative food sources may also differ in their nutritional value with potential consequences for the gas fluxes (Zhou et al., 2023). For example, a lower nitrogen content associated with grass-based compared to woody plant-based fungus combs found in Vesala et al. (2022b) could necessitate relatively high CO2 and CH4 emissions, as more carbon needs to be eliminated to gain sufficient protein from the N-deficient diet (Higashi et al., 1992; Eggleton and Tayasu, 2001). Whether such factors could affect the mound gas fluxes is currently not known.
In this study, we used the static chamber method to investigate CO2 and CH4 fluxes originating from mounds of two common species of fungus-growing termites, M. michaelseni and M. subhyalinus, in southern Kenya during periods in the dry and the wet season. As, in the area, both species inhabit a wide range of different environments, termite mounds were measured in two contrasting habitats (open grassland and dense bushland). Distribution of the two species is not completely overlapping, and, thus, in our sampling M. michaelseni was dominant at the grassland and M. subhyalinus at the bushland site. In Kenyan grasslands, M. michaelseni mounds are surrounded by dense networks of foraging tunnels with several openings to soil surface per square meter (Lepage, 1981a; Darlington, 1982) that could also increase soil CO2 and CH4 fluxes in mound proximity. Thus, in addition to direct gas fluxes from the mounds, we also measured soil CO2 and CH4 fluxes around the mounds. The study objectives were (1) to quantify the gas fluxes from the fungus-growing termite mounds with variable mound volumes and from the soil around the mounds, (2) to study the interactions between the fluxes and different environmental variables, and (3) to observe the diurnal and seasonal variation in mound fluxes.
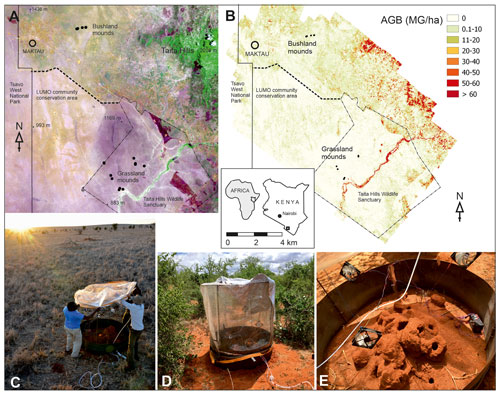
Figure 1Locations of the measured termite mounds (dots) at two study sites and the Maktau weather station shown in (a) Sentinel-2A satellite imagery (acquired 20 December 2020; magenta color indicate grasslands, while brownish colors indicate bushlands) and (b) an aboveground biomass map (Amara et al., 2020; Pellikka et al., 2018). Chamber measurement at the grassland (c) and the bushland site (d). Metal collar and wire frame of the measurement chamber around an open M. subhyalinus mound (e). Copernicus Open Access Hub (ESA, 2015). Photo credits: Petri Pellikka (c) and Markus Jylhä (d, e).
Table 1Characteristics of the measured Macrotermes termite mounds. R is the average mound radius, H is height and Vm is calculated aboveground mound volume (see Sect. 2.2). The status (active or dead) of the colonies was determined after the second measurement campaign by excavation.
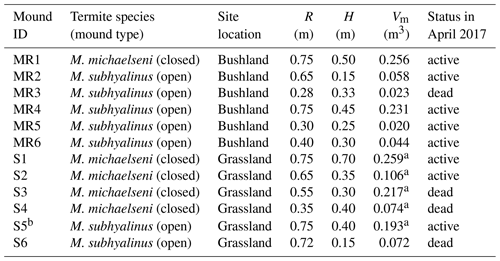
a These values were estimated using a photogrammetric technique. b The gas fluxes of this mound were not estimated due to nonlinear concentration time series (Fig. S2).
2.1 Site description
The chamber measurements were conducted in the lowland areas surrounding the Taita Hills, Kenya (Fig. 1). The mean annual rainfall is 433 mm based on measurements at the Maktau weather station (Räsänen et al., 2020). Termite mounds were measured at bushland and grassland locations that are 15 km apart. The bushland is characterized by over 50 % of thorny shrubs and small Acacia spp. and Commiphora spp. trees (Wachiye et al., 2020). All termite mounds measured in bushland were located in shaded locations. The grassland site is located at a private game sanctuary for wildlife conservation. The vegetation is characterized by common savanna grasses such as Chloris roxburghiana and Cenchrus ciliaris and scattered trees (e.g., Acacia tortilis, Albizia anthelmintica, Balanites aegyptiaca). The majority of bushland mounds were open mounds built by M. subhyalinus, whereas most of the grassland mounds were closed type and built by M. michaelseni (Table 1, Fig. S1 in the Supplement). The grassland ecosystem is assumed to be a result of decades-long wildlife conservation, which has caused a high population of mega-herbivores, namely elephants, which forage for leaves of the trees and bushes, thus destroying the tree cover. The tree cover and aboveground biomass are much higher in the bushland areas, which are not inhabited by elephants, as shown in Fig. 1b (Amara et al., 2020; Pellikka et al., 2018).
2.2 Estimation of mound volume
Volumes of the grassland mounds S1–S5 (Table 1) were measured photogrammetrically by taking a large number of digital images from different positions around the mound (method described in Vesala et al., 2019b). To increase the number of observations, these data were supplemented with five additional comparably sized mounds measured earlier in a close-by area (Vesala et al., 2019b). Linear regression with zero intercept was fitted between the equivalent cone volume and photogrammetric mound volume (Fig. 2). This relationship was used to estimate the volume at the bushland site where the photogrammetric method was not feasible due to the surrounding dense vegetation. The equivalent cone volume was calculated as
where R is the average of north–south and west–east measured radii and H is height. The aboveground termite mound volume (Vm) was 0.87⋅Vcone for mounds that were not sampled using the photogrammetric method. The aboveground termite mound volumes ranged from 0.3 % to 10 % of the total chamber volume.
2.3 Gas flux measurements from termite mounds and soil
CO2 and CH4 fluxes were measured using a static chamber method in November 2016 (end of the long dry season) and April 2017 (wet season) (Table S2). The chamber collar was installed before each measurement, and surrounding sand was applied to insulate the collar. The chamber closure time was 15 min, and each mound was measured three times with 5 min breaks in between the repetitions. The mean and standard deviation of the flux were calculated from the three repeated measurements. Daytime flux measurements were made between 10:00 and 16:00 (all times in this paper are given in local time, GMT+3). To study diurnal variation in gas fluxes, the mounds S1 and S2 were measured during at least three different time periods from the evening until the next day with three repeated measurements each time. Mound S1 was measured on 15 November 2016, and mound S2 was measured on 7 November 2016.
The size of the measurement chamber was a compromise between practicality and the typical size of termite mounds in the area. The radius of the chamber was 0.75 m and height was 1.5 m, still excluding the vast majority of Macrotermes mounds occurring in the area, which are larger on average. The metal collar height was 40 cm. Four upright-positioned steel rods and an attaching circular steel frame were used to support the cylindrical shape of the custom-made polyvinyl-chloride sheet. A rubber band was used to seal the polyvinyl-chloride sheet against the collar. Three people were needed to open and close the chamber. The gas analyzer (LGR Ultraportable Greenhouse Gas Analyzer Model 915-0011) was connected to the collar using Swagelok fittings. Chamber air was sampled continuously into the gas analyzer and circulated back to the chamber. The air was sampled inside the chamber using a 2.5 m long Teflon tube which had small holes along its length. The sampling line was attached in a spiral shape by supporting it from the middle points of the supporting steel rods, and it was connected to the inlet of the gas analyzer at the inside wall of the collar. The air inside the chamber was mixed using three 12 cm fans that were hanging in the middle point of the chamber. The gas analyzer recorded concentration every second. Temperature and relative humidity inside the chamber were measured using a Campbell CS215 T–RH sensor attached to the collar. A cellphone timing application (Time Meter) was used to record the precise start and end times of each measurement because the gas analyzer produces one continuous measurement file per day. Also, the manual soil moisture and temperature measurements were noted in the timing application. The notes were exported to a CSV file, which was parsed into numerical form. This procedure enabled us to automate flux calculation from raw data and minimize note-taking errors.
After termite mound measurement, the inlet lines were connected to a small opaque soil chamber (radius 7 cm, height 22 cm) to measure soil CO2 and CH4 fluxes around the termite mound. The soil gas flux measurements were made once for two opposing directions at 2, 4 and 6 m distance from the perimeter of the mound. The soil chamber was placed on patches of bare soil to minimize the effect of vegetation. The sampling time was 2 min. One CH4 flux measurement around S1 mound at 6 m distance during the wet season was discarded because the flux was over 1000 times higher than the mean of other measurements around the mound (most likely due to an underground termite nest).
Methane and carbon dioxide fluxes were calculated as
where for termite mound measurements and V=0.0034 m3 for the soil measurements, Vc=2.65 m3 is the termite chamber volume, P is pressure (Pa), A is the area of the chamber (m2), R is the gas constant (8.314 J mol−1 K−1) and T is the air temperature in kelvins. The slope of concentration increase (µmol mol−1 s−1) was determined from a linear regression of the 1 Hz concentration measurements.
2.4 Environmental measurements
The soil moisture and temperature were measured manually near the mound during each chamber measurement. A handheld soil moisture probe, the Campbell Scientific HydroSense II, was used to measure surface soil moisture. Soil temperature was measured using a stainless-steel temperature probe. Termite nest temperature measurements were used from an earlier study (Vesala et al., 2019b), where small temperature sensors (iButton Thermochron DS1922L) were placed next to the first fungus combs that were encountered by digging a small hole to the mound. Nest temperature measurements made at the grassland location in 2015 from large open and closed mounds (IDs TS56 and TS19 in Vesala et al., 2019b) were used. These nest temperatures were compared to continuous soil temperature measurements at the Maktau weather station. The weather station soil temperature (Campbell Scientific CS650) probe measured at 30 cm depth inside a fenced area.
The CO2 and CH4 fluxes measured for each mound were combined with aboveground biomass (AGB) data, assessed using airborne laser scanning and field measurements (Amara et al., 2020; Pellikka et al., 2018). This combination aimed to investigate the working hypothesis that fluxes might be related to the C:N ratios of termite diets that correlate negatively with vegetation density in the area (Vesala et al., 2022b).
2.5 Statistical analysis
Simple linear regression was used to test the statistical relationship between the measured fluxes and explanatory variables. The relationship between fluxes and mound volume was tested separately during the dry season (n=11) and wet season (n=7). The relationship of the environmental variables to the fluxes and to the ratio of CH4 to CO2 flux was tested using all the measurements (n=18). The mound flux–AGB relationship was tested with simple linear regression during the dry season (n=11). Statistical analyses were done using the SciPy Python package (Virtanen et al., 2020).
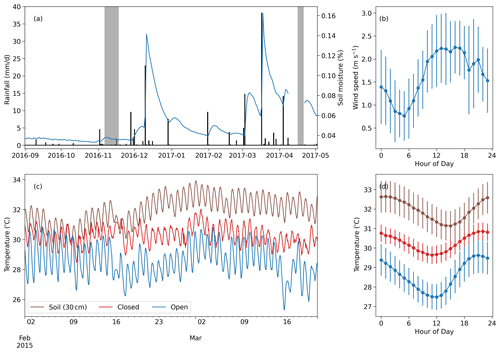
Figure 3Meteorological data from the study area. (a) Time series of daily rainfall (black bars) and daily mean soil moisture at 10 cm depth (blue line). The gray blocks indicate the days of the two measurement campaigns. (b) Diurnal mean and standard deviation of the wind speed during the first measurement campaign. (c) Soil temperature at 30 cm depth compared with nest temperatures within an open (TS56) and a closed (TS19) termite mound at the grassland site (measured in 2015 during a period of no rainfall; Vesala et al., 2019b). (d) Diurnal mean of the soil and nest temperatures during the time period presented in (c). The error bars indicate 1 standard deviation.
3.1 Environmental conditions
Total precipitation before the first measurement campaign was 10 mm from September to early November (Fig. 3), meaning that the short rainy season had not yet started. The precipitation between the campaigns was 153 mm, corresponding to a typical dry-year amount. The annual mean precipitation during this period between the campaigns was 287 mm, with 154 mm standard deviation calculated from 6 years of precipitation measurements at the weather station. The surface soil moisture was twice as high during the second campaign compared to the first campaign (Fig. 3a). The wind speed had a consistent trend during the measurement campaign days, showing a minimum around 06:00 and a maximum during the early afternoon. Revisiting the data collected in Vesala et al. (2019b) shows that termite mounds in the area have a consistent diurnal temperature rhythm, with the maximum nest temperature being always around 20:00, whereas the soil temperature at 30 cm depth has a maximum around midnight.
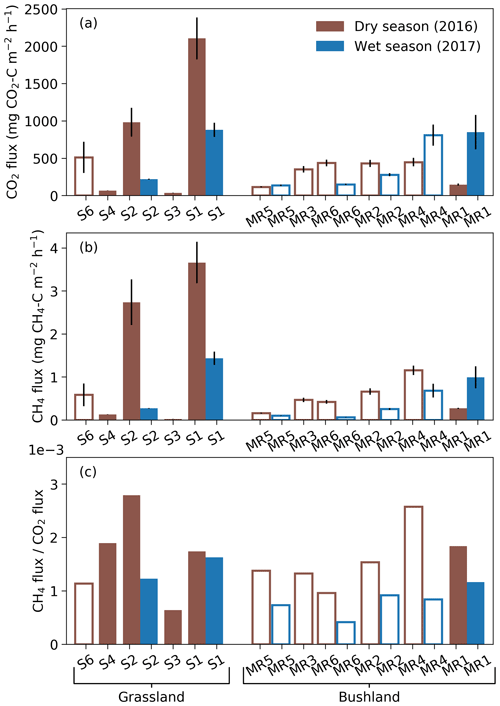
Figure 4Mean termite mound CO2 (a) and CH4 (b) fluxes and their relationship, i.e., CH4-to-CO2 flux ratios (c), measured from grassland and bushland sites during dry and wet seasons. Each mound was sampled once during each season by three repeated measurements. All measurements shown in this figure took place between 10:00 and 16:00. Error bars in (a) and (b) indicate standard errors. Hollow bars represent open and solid bars closed mounds. Termite colonies that were found to be dead in April 2017 (S3, S4, S6 and MR3) were left out from the wet-season measurements. The mounds are presented in order of increasing volume for each site.
3.2 Mound CO2 and CH4 fluxes
The mean CO2 fluxes ranged from 35 to 2100 mg CO2–C m−2 h−1 for closed M. michaelseni mounds and from 120 to 800 mg CO2–C m−2 h−1 for open M. subhyalinus mounds (Fig. 4). The mean CO2 flux was 1000 mg CO2–C m−2 h−1 for the grassland site and 380 mg CO2–C m−2 h−1 for the bushland site from the active mounds listed in Table 1. The largest CO2 fluxes were measured during the dry season at the grassland site. The site level mean CO2 flux decreased 64 % at the grassland and increased 41 % at the bushland from the dry to wet season. The lowest CO2 fluxes were registered from the grassland mounds S3 and S4, which turned out to be dead when excavated after the second measurement campaign (Table 1).
The mean CH4 fluxes ranged from 0.02 to 3.7 mg CH4–C m−2 h−1 for closed M. michaelseni mounds and 0.06 to 1.2 mg CH4–C m−2 h−1 for open M. subhyalinus mounds. The ratio of mound CH4 flux to mound CO2 flux ranged from to , with no clear difference between termite species or mound volume. The mean ratio was for grassland and for bushland sites. The ratio of CH4 flux to CO2 flux reduced by 36 % at the grassland and 49 % at the bushland site from dry to wet season. The dry-season mound CO2 and CH4 fluxes were not significantly correlated with the AGB (Fig. S3).
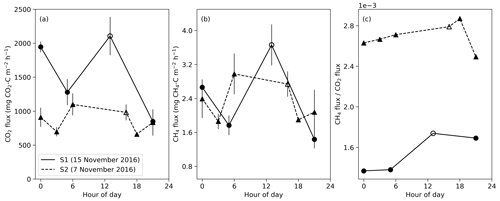
Figure 5Diurnal variation in CO2 and CH4 fluxes during the 2016 dry season for closed mounds S1 (measured 15 November) and S2 (measured 7 November). Error bars indicate the standard error calculated from three repeated measurements. The hollow symbols indicate daytime measurements that were done 9 d before nighttime measurements for S1 and 1 d before for S2.
Based on diurnal measurements in two closed mounds, the largest CO2 flux was measured around noon at the mound S1 (Fig. 5). However, the CO2 flux also increased at midnight for both S1 and S2 mounds. For both mounds, the difference between the maximum nighttime CO2 flux compared with the daytime maximum was less than 2 standard errors. For the S1 mound, the maximum flux value was 1.4 times the daily average for CO2 and 1.5 times that for CH4. The nighttime peak in the CO2 flux coincides with the maximum soil temperature at 30 cm depth at midnight (Fig. 3d). The diurnal cycle of the ratio of CH4 to CO2 flux showed one peak around noon for the S1 mound and at 18:00 for S2, which was not sampled at noon. These peaks are due to the increase in CH4 flux in proportion to CO2 flux during the daytime.
3.3 Drivers of mound CO2 and CH4 fluxes
The mean mound CO2 flux was significantly correlated with the termite mound volume during the wet season (R2=0.96, p value < 0.01; Fig. 6b), while the relation was not statistically significant during the dry season (R2=0.12, p value = 0.30; Fig. 6a). The CO2 flux and CH4 flux were not explained by surface soil moisture or soil temperature during the dry or wet season (Fig. S4). The soil moisture values were low for most mounds due to low precipitation amounts during the wet season. The mean CO2 and CH4 fluxes were linearly related (Fig. 6c). The ratio of CH4 and CO2 fluxes had no significant correlation with environmental variables and only a weak correlation with the magnitude of the CH4 flux (R2=0.34, p value = 0.01). The CH4 flux was significantly correlated with the mound volume during the wet season (R2=0.85, p value < 0.01; Fig. 6e). The standard deviation of the CO2 flux increased linearly with the mean flux magnitude (Fig. 6f).
3.4 Soil CO2 and CH4 fluxes around termite mounds
The soil CO2 and CH4 fluxes around the mounds were sampled to determine the effect of termites on soil fluxes around the mound. The site mean soil CO2 and CH4 were always higher at a 2 m distance compared to 4 and 6 m distances from the perimeter of the mound (Fig. 7 and Table 2). The mean soil CO2 flux at the bushland was only 29 % of the mean soil flux at the grassland. The dry-season means of all soil CO2 flux measurements were 62 and 27 mg CO2–C m−2 h−1 at 2 and 4 to 6 m distance (Table 2). The difference between soil CO2 flux at 2 and 4 to 6 m distance from the mound was less pronounced during the wet season. The mean mound CO2 fluxes were a factor of 25 and 6 higher in the dry and wet seasons, respectively, in comparison to the 4 to 6 m distance soil CO2 fluxes (Table 2). The dry-season means of all soil CH4 flux measurements were 0.07 and 0.02 mg CH4–C m−2 h−1 at 2 and 4 to 6 m distance (Table 2). The dry-season mean soil CH4 flux was positive at the grassland and bushland sites, whereas the mean wet-season flux was nearly zero with most fluxes being negative (Figs. 7, S5 and S6). The soil CH4 fluxes at a 2 m distance from the mound are much higher in proportion to fluxes at a 4 m distance at the grassland site compared to the bushland site (Fig. 7).
4.1 Mound CO2 and CH4 fluxes
The measured CO2 fluxes (from 35 to 2100 mg CO2–C m−2 h−1) were in a similar range to that reported in two earlier comparable studies, where chamber measurements were used to study greenhouse gas emissions from termite mounds in Australia (up to 1600 mg CO2–C m−2 h−1; Jamali et al., 2013) and Amazonia (190–2200 mg CO2–C m−2 h−1; van Asperen et al., 2021). Methane fluxes ranged from 0.02 to 3.7 mg CH4–C m−2 h−1 and were 2–20 times lower than those reported in earlier studies (0.4–6 mg CH4–C m−2 h−1; van Asperen et al., 2021; Jamali et al., 2013). In most fungus-growing species studied so far, workers have exhibited relatively high CH4 production rates (Rouland et al., 1993; Zhou et al., 2023). Thus, the lower CH4 emissions, in relation to CO2, observed in this study compared to mounds of previously studied termite species (which have all been termite types other than fungus-growing) could be attributed to the fact that a large proportion of the total CO2 in our measurements originated from the aerobic fungus gardens which do not produce CH4 (Darlington et al., 1997). Our biased sampling design, with one termite species dominating the bushland and the other the grassland site, does not allow us to reliably compare the two termite species. However, the relatively similar gas fluxes of the two species or mound types (Figs. 4 and 6) imply that they do not exhibit major differences in terms of CO2 or CH4 emissions. This result is also highly expected as the two termite species are closely related (Bagine et al., 1994; Vesala et al., 2017), have very similar population sizes and compositions (Darlington, 1984), and also share their dietary niches (Vesala et al., 2022b). The highest fluxes of both gases were registered from two closed mounds of M. michaelseni in the grassland. It is important to note that we did not obtain any information about the population sizes, and, thus, these two M. michaelseni mounds may simply have housed larger termite populations with correspondingly larger fungus gardens than the studied M. subhyalinus mounds.
As found by previous studies, the mound outer dimensions of both Macrotermes species correlate positively with the size of the termite population and the total biomass of fungus combs (Darlington and Dransfield, 1987; Darlington, 1990). Thus, the fluxes of metabolic gases should logically also show correlation with the mound volume. However, considering the high variation associated with the fluxes, it seems obvious that if the sizes of the studied mounds are too close to each other, the relationships between the mound volume and gas fluxes are difficult to detect. In this study, the mound volumes ranged from 0.023 to 0.259 m3 (Table 1), including notably more size variance than the earlier comparable studies (0.02 to 0.077 m3) (Jamali et al., 2013; van Asperen et al., 2021). We found that the measured CO2 fluxes were linearly correlated with the termite mound volume during the wet season (Fig. 6b) but that the relationship was more indistinct during the dry season (Fig. 6a). Determining the relationship between the mound volume and gas fluxes more reliably would require measurements from still much larger mounds, which however is problematic due to technical limitations. In East Africa, average volumes of Macrotermes mounds range from 2 to 3 m3 depending on the geographical area, and the maximum volumes can exceed 12 m3 (Pomeroy, 1977). Also in our study area, M. subhyalinus mounds with volumes of up to 6 m3 have been recorded (Vesala et al., 2019b), which is more than 20 times the volume of the largest mound measured here. Thus, the reported average flux values in this study are representative of the smallest Macrotermes mounds, but when scaling the results to the level of larger Macrotermes mounds (or calculating area-based estimates), the effect of mound volumes should be considered to avoid serious underestimations.
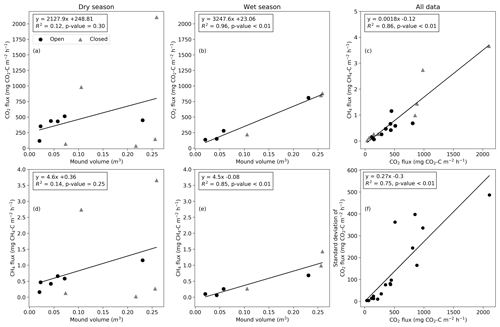
Figure 6The relationship between the mound volume and mean CO2 and CH4 fluxes during dry and wet seasons (a–b, d–e). (c) The relationship between CH4 and CO2 fluxes. Circles indicate open mounds, and triangles indicate closed mounds. (f) The relationship between the standard deviation and mean of the CO2 flux calculated from three repeated measurements.
The mounds at the grassland and bushland sites had contrasting seasonality of CO2 fluxes with a 64 % decrease at the grassland and 41 % increase at the bushland site from the dry to wet season (Fig. 4). This seasonal change is smaller than what has been measured for grass- and wood-feeding termites in Australia, which had a 90 % decrease in CO2 flux from the wet to dry season (Jamali et al., 2013). For fungus-growing termites, intra-annual variation can be caused by the annually produced brood of alates that, in Kenyan M. michaelseni, reaches its maximum biomass just before swarming in October–November (Darlington, 1986). Correspondingly, storage of fungus combs is typically at its largest soon after the long rains in May–June, after which it starts to decline (Lepage, 1981b). Such seasonal changes in biomasses of different nest components could cause intra-annual variation in gas fluxes. Additionally, differences in food quality could potentially affect nest CO2 emissions. Within our study area, fungus combs of grassland mounds had significantly lower nitrogen contents than those situated in more wooded habitats, suggesting that, on average, grass-based diets have lower nutritional value than wood-based diets (Vesala et al., 2022b). Such differences in food nutritional quality may reflect nest CO2 fluxes as more carbohydrates need to be eliminated to accumulate sufficient proteins from low-nitrogen food sources compared to those with a higher nitrogen content (Higashi et al., 1992). Most likely, food nutritional quality also changes during the year, with the changes possibly being more dramatic in grasslands, which have fewer and more contested food sources available for termites than bushlands with their more abundant and diverse food sources. Such local differences in food availability and quality could potentially lead to different seasonality in nest living biomass, which might explain the contrasting seasonal changes observed in CO2 fluxes between the two habitats and the higher intra-annual variance in gas fluxes observed in grassland compared to those in bushland mounds. We note that our seasonal sampling was limited to one measurement period during the dry and wet season, which does not capture the seasonal variability throughout the whole year.
There was a clear decrease in CH4-to-CO2 flux ratios from dry-season to wet-season measurements at both study sites. This difference might be partially explained by the smaller biomass of fungus gardens (which produce only CO2) in relation to that of termites (which produce both CO2 and CH4) during the dry season than during wet season (Darlington, 1986). Another explanation could be linked to the activity of methanotrophic bacteria within mound structures and/or surrounding soils that have been reported to consume a notable proportion of CH4 produced in the nests of several mound-building termite species (Sugimoto et al., 1998; Nauer et al., 2018). Although the humidity within termite nests remains at a constantly high level throughout the year (Lüscher, 1961; Agarwal, 1980), water content in surrounding soils and mound soil structures can decrease notably during periods with low precipitation (Jamali et al., 2011; Chen et al., 2019) (Fig. 3a). Dry soil conditions during periods with low precipitation most likely limit microbial activity (including the methane-oxidizing bacteria) within termite mound walls and surrounding soils, whereas increases in soil water content after rains could oppositely lead to increased microbial activity and enhanced methanotrophy. This result is also in congruence with our measurements of nest internal gas concentrations within M. michaelseni mounds, which tended to have higher CH4-to-CO2 concentration ratios during the driest period compared to seasons with higher soil moisture (Vesala et al., 2023).
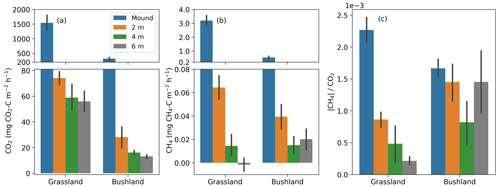
Figure 7(a–b) Mean CO2 and CH4 fluxes from termite mounds and surrounding soils during the dry season. Soil fluxes were always measured on two opposite sides of the mounds at distances of 2, 4 and 6 m from the mound perimeter (three repeated measurements at each specific location). Mean values and standard errors are calculated from all (dry-season) measurements at the site. (c) The ratio of the absolute value of CH4 and CO2 flux.
Four mounds that were measured during the first campaign were determined to be dead at the end of the second campaign (Table 1). The exceptionally low CO2 fluxes measured from the mounds S3 and S4, however, strongly suggest that these two mounds were already dead, or at least seriously weakened, during the first measurement campaign. An average lifespan of a Macrotermes bellicosus colony is thought to be around 4 years, whereas the mounds that they build persist typically for much longer (Pomeroy, 1976). However, a recent long-term monitoring study in Namibia found that 57 % of initially active M. michaelseni mounds were still active after 12 years (Wildermuth et al., 2022), demonstrating that some colonies can live much longer. These authors also found that small mounds were more likely to be dead than large mounds after 12 years, showing that large well-established colonies are less vulnerable to disruptions. Hence, dead Macrotermes mounds are a common feature in many landscapes, and for example, in many places within our study area, their number exceeds that of the active mounds (Vesala et al., 2017). Thus, determination of the proportion of active mounds in a landscape is a necessary precondition for ecosystem-level termite flux estimations for all such termite species that build persistent soil mounds.
4.2 Diurnal variation
Diurnal variations in CO2 and CH4 fluxes were measured in two closed M. michaelseni mounds over a period of 1 d. The afternoon peaks were on average 46 % and 57 % higher compared to the daily averages for CO2 and CH4 fluxes, respectively (Fig. 5). Our observations are in congruence with the nest internal CO2 concentration measurements by Ocko et al. (2017), who found that CO2 levels within M. michaelseni nests in Namibia peaked in the early afternoon, whereas concentrations at nighttime and especially soon after sunrise were somewhat lower. A corresponding trend has also been documented from outflow chimneys of Kenyan Macrotermes jeanneli, which had the highest CO2 concentrations at 15:00 and the lowest around 03:00–06:00 (Darlington et al., 1997). The daily maximum CO2 flux in the early afternoon found in this study (Fig. 5) agrees well with these earlier data, suggesting that the internal CO2 concentrations within M. michaelseni mounds show similar diurnal patterns to the mound CO2 fluxes. A positive relationship between the nest internal gases and mound fluxes has been demonstrated for a few mound-building termites (Khalil et al., 1990; Jamali et al., 2013). In agreement with our CH4 measurements, a similar peak in CH4 fluxes soon after midday was documented by Jamali et al. (2011) from mounds of three Australian termite species.
The lower CO2 and CH4 fluxes during the nighttime compared to daytime fluxes are probably mostly associated with the diurnal movements of termites: after sunset a proportion of termite workers and soldiers leave the nest due to foraging activities, thus decreasing the CO2 and CH4 production within the nest. During the night, termites also open the numerous foraging passages that directly connect the nest interior space to the soil surface near the mound (Lepage, 1981a; Darlington, 1982). This might lead to enhanced nest ventilation and could additionally affect gas fluxes originating from the mound. In addition to the afternoon flux peaks, we registered another peak in both CO2 and CH4 fluxes at midnight, which was not documented in earlier studies (Fig. 5). However, more measurements would be needed to understand the drivers of diurnal variation because we measured only two mounds in one location during the dry season. It would be especially useful to continuously measure the mound internal gas concentrations together with the mound fluxes.
In addition to the daily cycles of CO2 and CH4 fluxes, the relationship between CO2 and CH4 showed a clear diurnal pattern. The mound CH4-to-CO2 flux ratio was higher during the daytime than during the nighttime measurements (Fig. 5c). This result also supports the hypothesis that the termite population within the mound is smaller during the nighttime than during the daytime due to foraging activity. The metabolism of termites (together with gut microbiota) produces both CH4 and CO2, but a major proportion of CO2 originates from the fungal gardens, which are sessile and do not produce CH4 (Darlington et al., 1997). Thus any changes in termite biomass within the nest should inevitably reflect the mound CH4-to-CO2 flux ratios.
After all, the diurnal changes in gas fluxes measured in this study were relatively small. This underlines the fact that plant biomass degradation within mounds of fungus-growing termites is a continuously ongoing process and shows only minor diurnal changes. Therefore, a large proportion of termites also stay constantly in the nest, taking care of the fungus gardens, and thus the diurnal biomass changes within the nest are relatively small. The stable diurnal flux variability is in line with the stable humidity and temperature conditions within mounds of fungus-growing termites. Constant temperature and high humidity are of key importance for the fungal Termitomyces symbionts, which have a relatively narrow range of optimal microclimatic conditions (Lüscher, 1961; Thomas, 1981; Wood and Thomas, 1989).
4.3 Soil CO2 and CH4 fluxes around the mounds
The soil CO2 and CH4 fluxes were always higher close to the mound (at 2 m distance from the mound perimeter) compared to further distances (4 to 6 m from the mound perimeter) (Fig. 7). The mean soil CO2 flux from 4 to 6 m distance was 27 mg CO2–C m−2 h−1 during the dry season, which is comparable to the measured soil CO2 flux seasonal minimum of 20 mg CO2–C m−2 h−1 at the grassland site (Wachiye et al., 2020). Correspondingly, the mean soil CH4 fluxes at 4 to 6 m distances from the mounds were 0.020 and −0.004 mg CH4–C m−2 h−1 in the dry and wet seasons, respectively (Table 2), which is similar to an earlier field study where the soil CH4 fluxes were slightly negative with no seasonality at the grassland site (Wachiye et al., 2020). Our results thus demonstrate that, although the majority of the gas exchange between termite nests and ambient air takes place through the mound structures, a small proportion of gases also leave the nest via adjacent soils at up to 2 m distance. These results differ from the earlier measurements in soil-feeding termites in the Amazon, which showed enhanced soil fluxes around the mound only up to 0.5 m from the perimeter of the mound (van Asperen et al., 2021). The difference can be largely explained by the much larger size of the measured mounds in our study, but it most likely also reflects some fundamental differences between the mounds of soil-feeding and fungus-growing termites. While within soil-feeding species the mound itself acts as a nest for the colony, mounds built by especially Macrotermes michaelseni are more or less empty of insects and the actual nests are largely located underground below the mounds (Noirot and Darlington, 2000; Korb, 2011). Thus, the actual nests of fungus-growing termites may be much larger and extend to a somewhat wider area than their aboveground mounds. Macrotermes termites also have an extensive network of foraging tunnels surrounding their nest, with the largest radial tunnels having widths of 4–7 cm (Darlington, 1982). Such structures increase soil porosity around the mounds, enhancing water infiltration (Jouquet et al., 2011) and, obviously, also enabling some gas exchange to occur through the soil.
Soil CH4 fluxes around the mounds were systematically lower during the wet than during the dry season at all three distances (Table 2, Figs. S5 and S6). As the CH4 fluxes from the soils unaffected by termite mounds are shown to be slightly negative in grasslands of the same ecosystem (Wachiye et al., 2020), we are confident that termites were the main source of the soil CH4 fluxes detected in the current study. Seasonal changes in soil fluxes could thus be caused by changes in either the number of termites or their CH4 production rates or methane oxidation. During the rainy seasons, termite foraging activity is at its lowest (Lepage, 1981a), which could potentially decrease CH4 fluxes in mound surroundings as the foragers move from foraging tunnels towards the nests. This should, however, concurrently increase the CH4 fluxes from the mounds, which was not observed. Instead, we also found the mound CH4 fluxes to be lower during the rainy season than during the dry season. Thus, we suspect that, similarly to that discussed above in the case of mound fluxes, the soil CH4 fluxes near the mounds are largely regulated by CH4 oxidation within the soils surrounding the mounds, which could be enhanced by the increased soil water content after rains (Fig. 3a).
The CO2 and CH4 fluxes from fungus-growing termite mounds show diurnal and seasonal variation, albeit lower in magnitude than their wood-, grass- and soil-feeding counterparts. The likely reason for this stability is the temperature and humidity control needed for the fungal symbiont. The CO2 and CH4 fluxes scale with mound volume over a large volume range during the wet season. The soil flux measurements around the mound also show that the soil CO2 and CH4 fluxes are significantly affected by the termites at a 2 m distance from the perimeter of the mound. The fungus-growing termite mounds have lower CH4 fluxes per unit of CO2 than soil- and grass-feeding termites, which is due to the plant matter decomposition by Termitomyces fungi that do not emit CH4. For upscaling the greenhouse gas fluxes originating from mounds of fungus-growing termites, the work highlights the need to include the mound volume in the models, as well as to consider the potentially high proportion of inactive termite mounds in the landscape.
The data used in this study are available online: https://doi.org/10.6084/m9.figshare.21739484.v1 (Räsänen et al., 2022).
The supplement related to this article is available online at: https://doi.org/10.5194/bg-20-4029-2023-supplement.
MR, PM, MJ, PP and JR conducted the measurements; MR, RV, PR, LA, PP and JR designed the analysis; all authors contributed to the final version of the manuscript.
The contact author has declared that none of the authors has any competing interests.
Publisher’s note: Copernicus Publications remains neutral with regard to jurisdictional claims in published maps and institutional affiliations.
We thank Venla Pellikka, Mwadime Mjomba, Muhia Gicheru and Darius Kimuzi from Taita Research Station of the University of Helsinki and Remig Righa Mjomba, Wilson Mkala and Tobius Mwamburi from Taita Hills Wildlife Sanctuary for support during the fieldwork campaign. The research permit from the National Commission for Science, Technology and Innovation, Kenya (permit no. NCST/RCD/17/012/33), is appreciated.
This research has been supported by the Academy of Finland (grant nos. 318645 and 333868). Open-access funding was provided by the Helsinki University Library.
This paper was edited by Erika Buscardo and reviewed by Christian Brümmer and two anonymous referees.
Agarwal, V. B.: Temperature and relative humidity inside the mound of Odontotermes obesus (Rambur) (Isoptera: Termitidae), Proc. Anim. Sci., 89, 91–99, https://doi.org/10.1007/BF03179148, 1980.
Amara, E., Adhikari, H., Heiskanen, J., Siljander, M., Munyao, M., Omondi, P., and Pellikka, P.: Aboveground Biomass Distribution in a Multi-Use Savannah Landscape in Southeastern Kenya: Impact of Land Use and Fences, Land, 9, 381, https://doi.org/10.3390/land9100381, 2020.
Bagine, R., Brandl, R., and Kaib, M.: Species Delimitation in Macrotermes (Isoptera: Macrotermitidae): Evidence from Epicuticular Hydrocarbons, Morphology, and Ecology, Ann. Entom. Soc. Am., 87, 498–506, 1994.
Bignell, D. E. and Eggleton, P.: Termites in ecosystems, in Termites: Evolution, Sociality, Symbioses and Ecology, edited by: Abe, T., Bignell, D. E., and Higashi, M., Springer, 363–387, 2000.
Boutton, T. W., Arshad, M. A., and Tieszen, L. L.: Stable isotope analysis of termite food habits in East African grasslands, Oecologia, 59, 1–6, https://doi.org/10.1007/BF00388065, 1983.
Brümmer, C., Papen, H., Wassmann, R., and Brüggemann, N.: Fluxes of CH4 and CO2 from soil and termite mounds in south Sudanian savanna of Burkina Faso (West Africa), Global Biogeochem. Cy., 23, 1, https://doi.org/10.1029/2008GB003237, 2009.
Buxton, R. D.: Termites and the turnover of dead wood in an arid tropical environment, Oecologia, 51, 379–384, https://doi.org/10.1007/BF00540909, 1981.
Chen, C., Wu, J., Zhu, X., Jiang, X., Liu, W., Zeng, H., and Meng, F.-R.: Hydrological characteristics and functions of termite mounds in areas with clear dry and rainy seasons, Agriculture, Ecosyst. Environ., 277, 25–35, https://doi.org/10.1016/j.agee.2019.03.001, 2019.
Chiri, E., Greening, C., Lappan, R., Waite, D. W., Jirapanjawat, T., Dong, X., Arndt, S. K., and Nauer, P. A.: Termite mounds contain soil-derived methanotroph communities kinetically adapted to elevated methane concentrations, ISME J., 14, 2715–2731, https://doi.org/10.1038/s41396-020-0722-3, 2020.
Collins, N. M.: The role of termites in the decomposition of wood and leaf litter in the Southern Guinea savanna of Nigeria, Oecologia, 51, 389–399, https://doi.org/10.1007/BF00540911, 1981.
Darlington, J. P. E. C.: The underground passages and storage pits used in foraging by a nest of the termite Macrotermes michaelseni in Kajiado, Kenya, J. Zoology, 198, 237–247, https://doi.org/10.1111/j.1469-7998.1982.tb02073.x, 1982.
Darlington, J. P. E. C.: A method for sampling the populations of large termite nests, Ann. Appl. Biol., 104, 427–436, 1984.
Darlington, J. P. E. C.: Structure of mature mounds of the termite Macrotermes michaelseni in Kenya, Int. J. Trop. Insect Sci., 6, 149–156, https://doi.org/10.1017/S1742758400006536, 1985.
Darlington, J. P. E. C.: Seasonality in mature nests of the termite Macrotermes michaelseni in Kenya, Insectes Sociaux, 33, 168–189, 1986.
Darlington, J. P. E. C.: Populations in nests of the termite Macrotermes subhyalinus in Kenya, Insectes Sociaux, 37, 158–168, https://doi.org/10.1007/BF02224028, 1990.
Darlington, J. P. E. C. and Dransfield, R. D.: Size relationships in nest populations and mound parameters in the termite Macrotermes michaelseni in Kenya, Insectes Sociaux, 34, 165–180, 1987.
Darlington, J. P. E. C., Zimmerman, P. R., Greenberg, J., Westberg, C., and Bakwin, P.: Production of metabolic gases by nests of the termite Macrotermes jeanneli in Kenya, J. Trop. Ecol., 13, 491–510, https://doi.org/10.1017/S0266467400010671, 1997.
Eggleton, P. and Tayasu, I.: Feeding groups, lifetypes and the global ecology of termites, Ecol. Res., 16, 941–960, https://doi.org/10.1046/j.1440-1703.2001.00444.x, 2001.
Higashi, M., Abe, T., and Burns, T. P.: Carbon-nitrogen balance and termite ecology, P. Roy. Soc. B-Bio., 249, 303–308, https://doi.org/10.1098/rspb.1992.0119, 1992.
Hyodo, F., Tayasu, I., Inoue, T., Azuma, J. I., Kudo, T., and Abe, T.: Differential role of symbiotic fungi in lignin degradation and food provision for fungus-growing termites (Macrotermitinae: Isoptera), Funct. Ecol., 17, 186–193, https://doi.org/10.1046/j.1365-2435.2003.00718.x, 2003.
Jamali, H., Livesley, S. J., Dawes, T. Z., Cook, G. D., Hutley, L. B., and Arndt, S. K.: Diurnal and seasonal variations in CH4 flux from termite mounds in tropical savannas of the Northern Territory, Australia, Agr. Forest Meteorol., 151, 1471–1479, https://doi.org/10.1016/j.agrformet.2010.06.009, 2011.
Jamali, H., Livesley, S. J., Hutley, L. B., Fest, B., and Arndt, S. K.: The relationships between termite mound emissions and internal concentration ratios are species specific, Biogeosciences, 10, 2229–2240, https://doi.org/10.5194/bg-10-2229-2013, 2013.
Jouquet, P., Traoré, S., Choosai, C., Hartmann, C., and Bignell, D.: Influence of termites on ecosystem functioning. Ecosystem services provided by termites, Europ. J. Soil Biol., 47, 215–222, https://doi.org/10.1016/j.ejsobi.2011.05.005, 2011.
Khalil, M. A. K., Rasmussen, R. A., French, J. R. J., and Holt, J. A.: The Influence of Termites on Atmospheric Trace Gases: CH4, CO2, CHC13, N2O, CO, H2, and Light Hydrocarbons, J. Geophys. Res.-Atmos., 95, 3619–3634, 1990.
King, H., Ocko, S., and Mahadevan, L.: Termite mounds harness diurnal temperature oscillations for ventilation, P. Natl. Acad. Sci. USA, 112, 11589–11593, https://doi.org/10.1073/pnas.1423242112, 2015.
Kirschke, S., Bousquet, P., Ciais, P., Saunois, M., Canadell, J. G. Dlugokencky, E. J. Bergamaschi, P. Bergmann, D., Blake, D. R., Bruhwiler, L., Cameron-Smith, P., Castaldi, S., Chevallier, F., Feng, L., Fraser, A., Heimann, M., Hodson, E. L., Houweling, S., Josse, B., Fraser, P. J., Krummel, P. B., Lamarque, J. F. Langenfelds, R. L., Le Quere, C., Naik, V., O'Doherty, S., Palmer, P. I., Pison, I., Plummer, D., Poulter, B., Prinn, R. G., Rigby, M., Ringeval, B., Santini, M., Schmidt, M., Shindell, D. T., Simpson, I. J., Spahni, R., Steele, L. P., Strode, S. A., Sudo, K., Szopa, S., van der Werf, G. R., Voulgarakis, A., van Weele, M., Weiss, R. F., Williams, J. E. and Zeng, G.: Three decades of global methane sources and sinks, Nat. Geosci., 6, 813–823, https://doi.org/10.1038/ngeo1955, 2013.
Korb, J. and Linsenmair, K. E.: The effects of temperature on the architecture and distribution of Macrotermes bellicosus (Isoptera, Macrotermitinae) mounds in different habitats of a West African Guinea savanna, Insectes Soc., 45, 51–65, https://doi.org/10.1007/s000400050068, 1998.
Korb, J.: Thermoregulation and ventilation of termite mounds, Naturwissenschaften, 90, 212–219, https://doi.org/10.1007/s00114-002-0401-4, 2003.
Korb, J.: Termite Mound Architecture, from Function to Construction, in Biology of Termites: A Modern Synthesis, edited by: Bignell, D. E., Roisin, Y., and Lo, N., Springer, Dordrecht, 349–373, 2011.
Lepage, M. G.: L'impact des populations récoltantes deMacrotermes michaelseni (Sjöstedt) (Isoptera: Macrotermitinae) dans un écosystème semi-aride (Kajiado-Kenya), I – L'activité de récolte et son déterminisme, Insectes Soc., 28, 297–308, 1981a.
Lepage, M. G.: L'impact des populations récoltantes de Macrotermes michaelseni (Sjöstedt) (Isoptera: Macrotermitinae) dans un écosystème semi-aride (Kajiado-Kenya), II - La nourriture récoltée, comparaison avec les grands herbivores, Insectes Soc., 28, 309–319, 1981b.
Lepage, M., Abbadie, L., and Mariotti, A.: Food Habits of Sympatric Termite Species (Isoptera, Macrotermitinae) as Determined by Stable Carbon Isotope Analysis in a Guinean Savanna (Lamto, Cote d'Ivoire), J. Trop. Ecol., 3, 303–311, 1993.
Lüscher, M.: Air-Conditioned Termite Nests, Sci. Am., 205, 138–145, https://doi.org/10.1038/scientificamerican0761-138, 1961.
Nauer, P. A., Hutley, L. B., and Arndt, S. K.: Termite mounds mitigate half of termite methane emissions, P. Natl. Acad. Sci. USA, 115, 13306–13311, https://doi.org/10.1073/pnas.1809790115, 2018.
Noirot, C. and Darlington, J. P. E. C.: Termite nests: Architecture, Regulation and Defence, in Termites: Evolution, Sociality, Symbioses, Ecology, edited by: Abe, T., Bignell, D. E., and Higashi, M., Springer, 121–139, 2000.
Ocko, S. A., King, H., Andreen, D., Bardunias, P., Turner, J. S., Soar, R. and Mahadevan, L.: Solar-powered ventilation of African termite mounds, J. Exp. Biol., 220, 3260–3269, https://doi.org/10.1242/jeb.160895, 2017.
Pellikka, P. K. E., Heikinheimo, V., Hietanen, J., Schäfer, E., Siljander, M., and Heiskanen, J.: Impact of land cover change on aboveground carbon stocks in Afromontane landscape in Kenya, Appl. Geogr., 94, 178–189, https://doi.org/10.1016/j.apgeog.2018.03.017, 2018.
Pomeroy, D. E.: Studies on a population of large termite mounds in Uganda, Ecol. Entomol., 1, 49–61, https://doi.org/10.1111/j.1365-2311.1976.tb01204.x, 1976.
Pomeroy, D. E.: The Distribution and Abundance of Large Termite Mounds in Uganda, J. Appl. Ecol., 14, 465–475, https://doi.org/10.2307/2402559, 1977.
Rouland-Lefèvre, C.: Symbiosis with fungi, in Termites: Evolution, Sociality, Symbioses and Ecology, 289–306, 2000.
Räsänen, M., Merbold, L., Vakkari, V., Aurela, M., Laakso, L., Beukes, J. P., Zyl, P. G. V., Josipovic, M., Feig, G., Pellikka, P., Rinne, J., and Katul, G. G.: Root-zone soil moisture variability across African savannas: From pulsed rainfall to land-cover switches, Ecohydrology, 13, e2213, https://doi.org/10.1002/eco.2213, 2020.
Räsänen, M., Vesala, R., Rönnholm, P., Arppe, L., Manninen, P., Jylhä, M., Rikkinen, J., Pellikka, P., and Rinne, J.: Dataset for “Influence of termite mound structure and habitat on the mound CO2 and CH4 fluxes for fungus-growing termites”, figshare [data set], https://doi.org/10.6084/m9.figshare.21739484.v1, 2022.
Sanderson, M. S.: Biomass of termites and their emissions of methane and carbon dioxide: A global database, Global Biogeochem. Cy., 10, 543–557, https://doi.org/10.1029/96GB01893, 1996.
Saunois, M., Stavert, A. R., Poulter, B., Bousquet, P., Canadell, J. G., Jackson, R. B., Raymond, P. A., Dlugokencky, E. J., Houweling, S., Patra, P. K., Ciais, P., Arora, V. K., Bastviken, D., Bergamaschi, P., Blake, D. R., Brailsford, G., Bruhwiler, L., Carlson, K. M., Carrol, M., Castaldi, S., Chandra, N., Crevoisier, C., Crill, P. M., Covey, K., Curry, C. L., Etiope, G., Frankenberg, C., Gedney, N., Hegglin, M. I., Höglund-Isaksson, L., Hugelius, G., Ishizawa, M., Ito, A., Janssens-Maenhout, G., Jensen, K. M., Joos, F., Kleinen, T., Krummel, P. B., Langenfelds, R. L., Laruelle, G. G., Liu, L., Machida, T., Maksyutov, S., McDonald, K. C., McNorton, J., Miller, P. A., Melton, J. R., Morino, I., Müller, J., Murguia-Flores, F., Naik, V., Niwa, Y., Noce, S., O'Doherty, S., Parker, R. J., Peng, C., Peng, S., Peters, G. P., Prigent, C., Prinn, R., Ramonet, M., Regnier, P., Riley, W. J., Rosentreter, J. A., Segers, A., Simpson, I. J., Shi, H., Smith, S. J., Steele, L. P., Thornton, B. F., Tian, H., Tohjima, Y., Tubiello, F. N., Tsuruta, A., Viovy, N., Voulgarakis, A., Weber, T. S., van Weele, M., van der Werf, G. R., Weiss, R. F., Worthy, D., Wunch, D., Yin, Y., Yoshida, Y., Zhang, W., Zhang, Z., Zhao, Y., Zheng, B., Zhu, Q., Zhu, Q., and Zhuang, Q.: The Global Methane Budget 2000–2017, Earth Syst. Sci. Data, 12, 1561–1623, https://doi.org/10.5194/essd-12-1561-2020, 2020.
Sieber, R. and Leuthold, R. H.: Behavioural elements and their meaning in incipient laboratory colonies of the fungus-growing Termite Macrotermes michaelseni (Isoptera: Macrotermitinae), Insectes Soc., 28, 371–382, https://doi.org/10.1007/BF02224194, 1981.
Sugimoto, A., Inoue, T., Kirtibutr, N., and Abe, T.: Methane oxidation by termite mounds estimated by the carbon isotopic composition of methane, Global Biogeochem. Cy., 12, 595–605, https://doi.org/10.1029/98GB02266, 1998.
Thomas, R. J.: Ecological studies on the symbiosis of Termitomyces Heim with Nigerian Macrotermitinae, University of London, PhD Thesis, https://qmro.qmul.ac.uk/xmlui/handle/123456789/1777 (last access: 28 September 2023), 1981.
Wachiye, S., Merbold, L., Vesala, T., Rinne, J., Räsänen, M., Leitner, S., and Pellikka, P.: Soil greenhouse gas emissions under different land-use types in savanna ecosystems of Kenya, Biogeosciences, 17, 2149–2167, https://doi.org/10.5194/bg-17-2149-2020, 2020.
van Asperen, H., Alves-Oliveira, J. R., Warneke, T., Forsberg, B., de Araújo, A. C., and Notholt, J.: The role of termite CH4 emissions on the ecosystem scale: a case study in the Amazon rainforest, Biogeosciences, 18, 2609–2625, https://doi.org/10.5194/bg-18-2609-2021, 2021.
Vesala, R., Niskanen, T., Liimatainen, K., Boga, H., Pellikka, P., and Rikkinen, J.: Diversity of fungus-growing termites (Macrotermes) and their fungal symbionts (Termitomyces) in the semiarid Tsavo Ecosystem, Kenya, Biotropica, 49, 402–412, https://doi.org/10.1111/btp.12422, 2017.
Vesala, R., Arppe, L., and Rikkinen, J.: Caste-specific nutritional differences define carbon and nitrogen fluxes within symbiotic food webs in African termite mounds, Sci. Rep., 9, 16611–16698, https://doi.org/10.1038/s41598-019-53153-x, 2019a.
Vesala, R., Harjuntausta, A., Hakkarainen, A., Rönnholm, P., Pellikka, P., and Rikkinen, J.: Termite mound architecture regulates nest temperature and correlates with species identities of symbiotic fungi, Peer J., 6, e6237, https://doi.org/10.7717/peerj.6237, 2019b.
Vesala, R., Arppe, L., and Rikkinen, J.: Termitomyces fungus combs – formation, structure and functional aspects, in Microbial Symbionts: Functions and Molecular Interactions on Host, edited by: Dhanasekaran, D., Academic Press., ISBN 9780323993340, 2022a.
Vesala, R., Rikkinen, A., Pellikka, P., Rikkinen, J., and Arppe, L.: You eat what you find – local patterns in vegetation structure control diets of African fungus-growing termites, Ecol. Evol., 12, e8566, https://doi.org/10.1002/ece3.8566, 2022b.
Virtanen, P., Gommers, R., Oliphant, T. E., Haberland, M., Reddy, T., Cournapeau, D., Burovski, E., Peterson, P., Weckesser, W., Bright, J., van der Walt, S. J., Brett, M., Wilson, J., Millman, K. J., Mayorov, N., Nelson, A. R. J., Jones, E., Kern, R., Larson, E., Carey, C. J., Polat, İ., Feng, Y., Moore, E. W., VanderPlas, J., Laxalde, D., Perktold, J., Cimrman, R., Henriksen, I., Quintero, E. A., Harris, C. R., Archibald, A. M., Ribeiro, A. H., Pedregosa, F., van Mulbregt, P., and SciPy 1.0 Contributors: SciPy 1.0: fundamental algorithms for scientific computing in Python, Nat. Methods, 17, 261–272, https://doi.org/10.1038/s41592-019-0686-2, 2020.
Weir, J. S.: Air Flow, Evaporation and Mineral Accumulation in Mounds of Macrotermes subhyalinus (Rambur), J. Anim. Ecol., 42, 509–520, https://doi.org/10.2307/3120, 1973.
Wildermuth, B., Oldeland, J., Arning, C., Gunter, F., Strohbach, B. and Juergens, N.: Spatial patterns and life histories of Macrotermes michaelseni termite mounds reflect intraspecific competition: Insights of a temporal comparison spanning 12 years, Ecography, 9, e06306, https://doi.org/10.1111/ecog.06306, 2022.
Wood, T. G. and Thomas, R. J.: The mutualistic association between Macrotermitinae and Termitomyces, in: Insect-fungus interactions, edited by: Wilding, N., Collins, N. M., Hammond, P. M., and Webber, J. F., Academic Press., 69–92, 1989.
Zhou, Y., Staver, A. C., and Davies, A. B.: Species-level termite methane production rates, Ecology, 104, e3905, https://doi.org/10.1002/ecy.3905, 2023.
Zimmerman, P. R., Greenberg, J. P., Wandiga, S. O., and Crutzen, P. J.: Termites: A Potentially Large Source of Atmospheric Methane, Carbon Dioxide, and Molecular Hydrogen, Science, 218, 563–565, 1982.