the Creative Commons Attribution 4.0 License.
the Creative Commons Attribution 4.0 License.
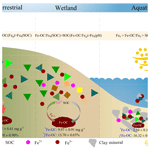
Ecosystem-specific patterns and drivers of global reactive iron mineral-associated organic carbon
Bo Zhao
Amin Dou
Zhiwei Zhang
Zhenyu Chen
Wenbo Sun
Yanli Feng
Xiaojuan Wang
Reactive iron (Fe) oxides are vital for long-term soil or sediment organic carbon (SOC) storage. However, the patterns and drivers of Fe-associated organic carbon (Fe-OC) over global geographic scales under various ecosystem types remain controversial. Here, we provided a systematic assessment of the distribution patterns and determinants of Fe-OC content and its contribution to SOC (fFe-OC) by assembling a global dataset comprising 862 observations from 325 sites in distinct ecosystems. We found that Fe-OC content across global ecosystems ranged from 0 to 83.3 g kg−1 (fFe-OC ranged from 0 % to 82.4 %), reflecting the high variability of the Fe-OC pool. Fe-OC contents varied with ecosystem type being greater in wetlands with a high molar ratio of Fe-OC dithionite-extractable Fe (Fed) compared with marine and terrestrial ecosystems. Furthermore, fFe-OC in wetlands was significantly lower than that in other ecosystems due to rich organic carbon (OC). In contrast with climate variables and soil pH, the random forest modeling and multivariate analysis showed that the Fe-OC : Fed and SOC were the predominant predictors of Fe-OC content and fFe-OC in wetlands and terrestrial ecosystems, whereas Fed content was a primary driver in marine ecosystems. Based on upper estimates of global SOC storage in various ecosystem types, we further estimated that 83.84 ± 3.8, 172.45 ± 8.74, and 24.48 ± 0.87 Pg of SOC were preserved by association with Fe oxides in wetland, terrestrial, and marine ecosystems, respectively. Taken together, our findings highlighted the importance of reactive Fe oxides in global SOC preservation, and their controlling factors were ecosystem specific.
- Article
(4749 KB) - Full-text XML
-
Supplement
(531 KB) - BibTeX
- EndNote
The global soil (sediment) organic carbon (SOC) cycle has become one of the hotspots in biogeochemical and global climate change research (Lal, 2004a; Crowther et al., 2016). Organic carbon (OC) sequestration is a significant ecosystem service (such as climate mitigation, soil fertility, and ecosystem stability) provided by terrestrial, wetland, and marine ecosystems. Accumulating evidence has shown that the reactive mineral matrix plays a critical role in sequestering and stabilizing SOC (Kramer and Chadwick, 2018; Ye et al., 2022). OC has a strong affinity for reactive Fe (hydr)oxides (Longman et al., 2022), and the resulting Fe and OC association by adsorption or coprecipitation is thought to promote OC long-term preservation in soils and sediments (Schmidt et al., 2011; Hemingway et al., 2019). Therefore, a systematic understanding of the patterns and drivers of Fe-associated OC (Fe-OC) is pivotal for accurately predicting SOC dynamics and reducing model uncertainties in forecasting carbon–climate feedback at the global scale.
In comparison to other metal minerals, Fe (hydr)oxides, one of the most prevalent reactive minerals, have larger specific surface areas, a higher OC affinity, and a greater potential to retain SOC (Guggenberger and Kaiser, 2003; Eusterhues et al., 2005; Kaiser et al., 2007). A growing body of studies has suggested that Fe (hydr)oxides play a fundamental role in stabilizing SOC in sediment and soil (Yu et al., 2021). Recently, Fe-OC has been extracted and quantified through the bicarbonate–citrate–dithionite (BCD) method and was estimated to constitute 21.5 % (Lalonde et al., 2012), 4.7 %–37.8 % (Zhao et al., 2016; Fang et al., 2019; Zong et al., 2021), and 3.4 %–11.8 % (Huang et al., 2021; Wang et al., 2021) of SOC in marine, terrestrial (i.e., forests, grasslands, farmland), and wetland (i.e., coastal, peatland, and lake wetlands) ecosystems, respectively. The Fe-OC content and contribution (fFe-OC) vary with ecosystem type. Marine sediments are the largest OC sink on Earth and are crucial to the global carbon cycle. Reactive Fe minerals can protect and bury large amounts of SOC within marine sediments, constituting a “rusty sink” (Lalonde et al., 2012). The fFe-OC in marine sediments is significantly lower than that in offshore estuarine sediments due to differences in sediment mineralogy, reactive Fe source, and organic matter composition (Longman et al., 2022). It is well-known that wetland ecosystems possess an extremely high rate of OC sequestration (McLeod et al., 2011; Hopkinson et al., 2012). Compared with terrestrial and marine ecosystems, wetland soils or sediments are periodically submerged due to (semi)diurnal tidal cycles or fluctuations in the water table (Yu et al., 2021). Thus, in wetland environments, Fe (hydr)oxides are repeatedly formed and destroyed as a result of periodical redox-induced changes in (Patzner et al., 2020), which is thought to weaken the interaction between Fe and OC (Huang and Hall, 2017; LaCroix et al., 2019; Anthony and Silver, 2020). However, Wang et al. (2017) proposed an important “iron gate” mechanism in OC-rich wetlands (Wang et al., 2017) and showed that the fFe-OC in wetlands and uplands is equally important (Wang et al., 2017). Thus, a systematic analysis of Fe-OC content and fFe-OC in terrestrial, wetland, and marine ecosystems at the global scale can provide evidence for the importance of reactive Fe minerals in global climate change.
Recently, some studies have found that the Fe-OC content and fFe-OC are mainly controlled by soil properties (Grybos et al., 2009; Ye et al., 2022), organic matter composition (Fisher et al., 2020), and climate (or latitude) (Kramer and Chadwick, 2018). For instance, Fe-OC increases with increasing latitude, mean annual precipitation (MAP), SOC content, and potential evapotranspiration (Zhao et al., 2016; Kramer and Chadwick, 2018), but it decreases with increasing soil pH at the continental scale (Ye et al., 2022). However, Fe-OC content and fFe-OC in farmland soils are not related to latitude, mean annual temperature (MAT), and MAP but are related to SOC content (Wan et al., 2019). In peatlands, Huang et al. (2021) found that Fe-OC content is positively correlated with the SOC content, C:N, and MAT but not with MAP at the regional scale (Huang et al., 2021). However, Fe-OC in coastal wetlands was positively correlated with amorphous Fe content and clay content but negatively correlated with soil pH and phenol oxidase activity (Bai et al., 2021). In marine sediments, Fe-OC content may be mainly responsible for SOC content and organic matter functional groups (especially carboxyl content) (Wang et al., 2019; Fisher et al., 2020). Additionally, according to Kramer and Chadwick (2018), fFe-OC in humid climate forest regions was much higher than that in semiarid and arid regions, confirming the natural linkages between fFe-OC and climate (Kramer and Chadwick, 2018). Fe-OC content is also influenced by the bonding mechanism of Fe and OC (Wagai and Mayer, 2007; Faust et al., 2021). The bonding mechanism between Fe and OC is determined by the Fe-OC dithionite-extractable Fe (Fed) molar ratio (Faust et al., 2021; Wang et al., 2021), with less than 1 indicating an Fe-OC bonding form of monolayer surface sorption and greater than 6 indicating a bonding mechanism dominated by coprecipitation (Wagai and Mayer, 2007; Lalonde et al., 2012). Generally, the OC content of the complexes obtained by coprecipitation is much higher than that of adsorption (Chen et al., 2014), which may also explain the wide variations in Fe-OC and fFe-OC. Thus, uncovering the factors controlling Fe-OC formation or association at the global scale is a prerequisite for predicting the size of the OC pool and its feedback on global climate change. However, the determinants of Fe-OC associations remain unknown globally, and only two studies on Fe-OC have been undertaken at continental scale, which focus on the relationships of Fe-OC and soil pH (Ye et al., 2022) and MAP and potential evapotranspiration (Kramer and Chadwick, 2018). These studies overlooked the influence of climate and soil properties (such as soil pH, Fed, Fe-OC : Fed, clay content) in controlling Fe-OC and fFe-OC in wetland and marine ecosystems. Furthermore, they have not yet explored the relationship between these key factors and Fe-OC and fFe-OC across global ecosystem types. A deeper understanding of these limitations in terrestrial, wetland, and marine ecosystems will allow us to draw clear conclusions regarding global patterns and drivers of Fe-OC.
In this study, we provide a comprehensive analysis of the spatial variability and characteristics of Fe-OC among terrestrial, wetland, and marine ecosystems and its governing factors globally. Specifically, we analyzed data from 862 observations from 46 published papers and the National Ecological Observatory Network (NEON) to explore (i) the importance of Fe-OC to SOC storage in wetland and marine ecosystems and its level compared with terrestrial ecosystems; (ii) whether the distribution patterns (i.e., spatial variability) of Fe-OC and the relationships between key factors and Fe-OC differ among ecosystem types; and (iii) the bonding mechanism of reactive Fe and OC in different ecosystem types, i.e., adsorption or coprecipitation.
2.1 Study selection
The ecosystem types included terrestrial, wetland, and marine systems in this study. We conducted extensive literature searches on the Web of Science (https://www.webofscience.com/2021, last access: October 2022) and the China National Knowledge Resource Integrated databases and searched for relevant research published from 2010 to August 2022. The appropriate studies were identified by the following search terms: (“reactive mineral” OR “iron”) AND (“bound” OR “associated” OR “stabilization” OR “interaction” OR “sequestration”) AND (“organic carbon”) (Fig. S1 in the Supplement). The following criteria must be met for inclusion in this study: (a) soil samples at 0–100 cm depth must be collected from in situ observation data of wetlands (i.e., peatland, bog, fen, deltaic, lake wetland, mangrove wetland, and estuary wetland), forests (i.e., evergreen forest and deciduous forest), grasslands (i.e., temperate grasslands and alpine grasslands), farmland (i.e., paddy field and crop), and marine ecosystems (i.e., marine and river sediments); (b) the contents of Fe-OC and Fed must be measured using the BCD method in bulk soil; and (c) Fe-OC and the Fe-OC Fed molar ratio must be provided or could be calculated from the publications. In total, we compiled 862 data records from 46 published papers along with 42 additional data collected from NEON. The dataset included 325 sites, with latitudes between 25.22∘ S and 81.75∘ N and longitudes between 156.4∘ W and 174.4∘ E (Fig. 1). We also collected global data on SOC stocks in terrestrial, wetland, and marine ecosystems, respectively, which will allow us to further estimate Fe-OC stocks in different ecosystems.
2.2 Data assembly and collection
Data from published articles and NEON were assembled to construct the Fe-OC dataset. Site-specific data such as ecosystem type, MAP, MAT, latitude, longitude, clay, soil pH, SOC, Fe-OC, Fed, Fe-OC Fed molar ratio, and fFe-OC (calculated using the following equation: fFe-OC (%) = Fe-OC SOC × 100 %) were collected from each published paper; other details are shown in Fig. S1. If the MAT and MAP are not reported, the data for each site shall be obtained from the WorldClim database (http://www.worldclim.org, last access: April 2022). The data obtained from the WorldClim database are the average climate data from 1970 to 2000. All original data and average data were taken from the published articles' text, graphs, and tables. When data were presented graphically, the numerical data were digitized and extracted with the GetData Graph Digitizer (v4.4, http://www.getdata-graph-digitizer.com, last access: December 2022).
2.3 Statistical analysis
All data analyses were conducted using the R platform (v 4.1.2; https://www.r-project.org/, last access: December 2022). We used the Shapiro–Wilk test to determine the homogeneity of variances and the normal distribution of the data before using parametric methods. We used the Kruskal–Wallis test to determine significant differences among different ecosystems.
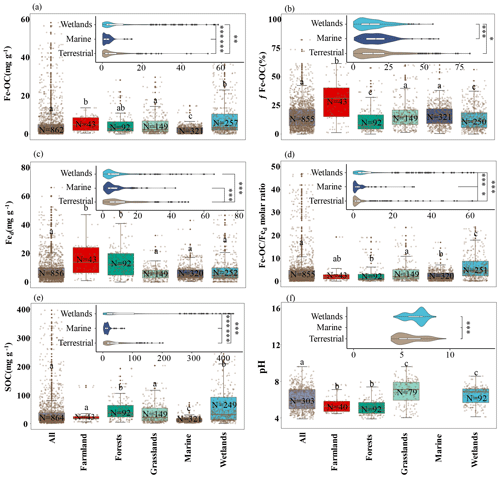
Figure 2The Fe-OC content (a), fFe-OC (b), soil pH (c), Fed content (d), SOC content (e), and Fe-OC Fed molar ratio (f) in different ecosystems are shown in the box plot. Solid dots indicate outliers, and imaginary points represent observations. Box edges are upper and lower quartiles, central lines are median value, and whiskers represent standard error. The differences among terrestrial, wetland, and marine ecosystems are illustrated (, , ).
Hedges' g, a bias-corrected standardized mean difference, was used to measure effect size to account for the bias of ecosystem-scale Fe-OC associated with small sample sizes (Chien and Krumins, 2022; Smale et al., 2020). Based on ecosystem types, all data were divided into terrestrial, marine, and wetland ecosystems, and the data were averaged separately for each ecosystem representing “control”. The sample sizes of individual cases (i.e., a single published article) represent “treatment”. The standardized mean difference between the control and treatment was measured by the pooled variance (Chien and Krumins, 2022). We used the package “metafor” in R (v 4.1.2; https://www.r-project.org/, last access: December 2022) to generate forest plots for every ecosystem by using a random effects model (Fig. S2). We calculated the total observed change (I2) and used the heterogeneity test (Q) to verify the heterogeneity of the collected data, and an I2 value higher than 75 % or p<0.05 indicates substantial heterogeneity (Meisner et al., 2014). We performed Spearman's correlation analyses to evaluate the relationship between environmental variables (SOC, MAT, MAP, clay, soil pH, Fe-OC : Fed, Fed, and latitude) and Fe-OC and fFe-OC. The linear (“lm” function in R) fitting was demonstrated to analyze the relationships between environmental variables and Fe-OC and fFe-OC. The significant correlation was considered at p<0.05. To test the relative importance of these drivers, a random forest (RF) analysis (Breiman, 2001) was performed according to the protocol described by Delgado-Baquerizo et al. (2016). For the RF analyses, the climate variables (MAT, MAP), soil properties (SOC, clay, soil pH, Fe-OC : Fed, and Fed), and geographical location (i.e., latitude) were included as predictors and the Fe-OC and fFe-OC changes and dynamics as the response variables. The significance of the models and cross-validated R2 values was evaluated with 500 permutations of the response variables with the “A3” R package. Similarly, using the “rfPermute” package for R (p<0.05), the importance of each predictor on the response variables was evaluated.
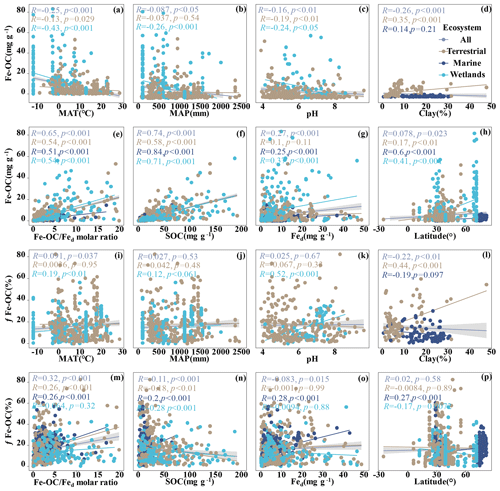
Figure 3Relationships between Fe-OC, fFe-OC, soil properties (soil pH, Fed, Fe-OC Fed molar ratio, SOC, clay), climate variables (MAT, MAP), and latitude across global ecosystem types. The line represents the line of best fit for each ecosystem, and the shaded area indicates the 95 % confidence interval for the global dataset. In marine ecosystems, the climate variables (MAT, MAP) and soil pH are not shown due to limited data.
3.1 Fe-associated OC and its related indicators across ecosystem types
Across global ecosystem types (i.e., terrestrial, wetland, and marine ecosystems), Fe-OC content (n=862) and fFe-OC (n=855) varied significantly and ranged from 0 to 83.3 mg g−1 (mean: 5.62 ± 0.32 mg g−1) and 0 %–82.4 % (mean: 16.03 ± 0.41 %), respectively (Fig. 2a, b). The contents of Fe-OC in terrestrial, marine, and wetland ecosystems were 5.42 ± 0.41 mg g−1 (fFe-OC: 17.76 ± 0.90 %), 2.34 ± 0.12 mg g−1 (fFe-OC: 16.32 ± 0.58 %), and 9.97 ± 0.91 mg g−1 (fFe-OC: 13.70 ± 0.63 %), respectively, with significant differences among ecosystem types (p<0.05; Fig. 2a). Correspondingly, the contribution of Fe-OC to SOC (fFe-OC) was significantly different among different ecosystem types (p<0.05; Fig. 2b). Meanwhile, Hedges' g unbiased standardized mean difference showed that small sample sizes at local scale (i.e., single published articles) had obvious distinct effect sizes for ecosystem-scale Fe-OC (I2<75 % or p<0.05), especially for marine ecosystems (Fig. S2). Fed contents (n=856) ranged from 0.03 to 245 mg g−1 (mean: 9.43 ± 0.53 mg g−1; Fig. 2c); that is, Fed varied 8167-fold, which was significantly higher in terrestrial ecosystems than in wetland and marine ecosystems (p<0.05; Fig. 2c). The Fe-OC Fed molar ratio (n=855) ranged from 0 to 331.68 (mean: 8.40 ± 0.85) at the global scale, and its mean value was significantly higher in wetlands than in terrestrial systems, while the minimum value was found in marine systems (p<0.05; Fig. 2d). SOC contents (n=854) ranged from 0.3 to 423.74 mg g−1 (mean: 43.28 ± 2.52 mg g−1), which had similar changes with the Fe-OC contents among ecosystem types (p<0.05; Fig. 2e). Taken together, the Fe-OC, SOC, and Fe-OC Fed molar ratio were significantly higher in wetlands, with the lowest values in marine ecosystems across global ecosystem types.
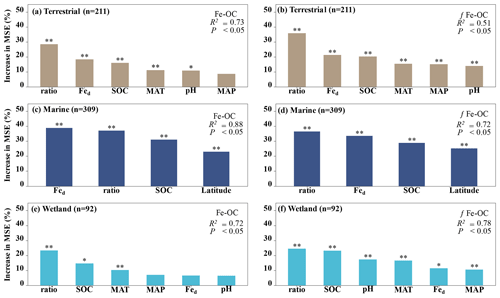
Figure 4The relative importance of climate variables (MAT, MAP), soil properties (SOC, soil pH, Fe-OC : Fed, and Fed), and geographical location (i.e., latitude) for Fe-OC and fFe-OC in terrestrial (a, b), marine (c, d), and wetland (e, f) ecosystems by random forest (RF) analysis. The mean square error (MSE) is used to estimate the importance of these predictors, with higher MSE values indicating more important predictors. In marine ecosystems, the climate variables (MAT, MAP) and soil pH are not shown due to limited data. Ratio: Fe-OC Fed molar ratio. Asterisks show significant differences: and .
3.2 Effect of environmental factors on Fe-OC and fFe-OC across ecosystem types
We analyzed their relationships with climate variables and soil properties to better understand the potential effect factors behind the observed variance in Fe-OC contents and fFe-OC among ecosystem types (Fig. 3). Among them, in wetland ecosystems, Fe-OC content showed a negative correlation with MAT (, p<0.001; Fig. 3a) and MAP (, p<0.001; Fig. 3b), while fFe-OC was positively correlated with the climate variables (MAT, MAP) (Fig. 3i, j). The Fe-OC content decreased significantly with increasing soil pH in wetlands (, p<0.01; Fig. 3c) and terrestrial systems (, p<0.05; Fig. 3c), but fFe-OC increased with increasing soil pH (R=0.52, p<0.001; Fig. 3k) in wetlands. Across the ecosystem types, Fed contents showed positive correlations with Fe-OC (R= 0.25, p<0.001; Fig. 3g) and fFe-OC (R=0.28, p<0.001; Fig. 3o) only in marine ecosystems. Moreover, Fe-OC increases significantly with Fed contents (R=0.35, p<0.001; Fig. 3g) in wetlands, but fFe-OC does not; however, Fed content has no relationship with Fe-OC and fFe-OC in terrestrial ecosystems. The molar ratio of Fe-OC Fed was positively correlated with Fe-OC and fFe-OC in other ecosystems, except for fFe-OC which was not correlated with the molar ratios in wetlands (Fig. 3e, m). Fe-OC contents increased significantly, but fFe-OC (except marine) decreased with increasing SOC contents in all ecosystems (Fig. 3f, n). At continental scales, Fe-OC content (R=0.35, p<0.001; Fig. 3d) and fFe-OC (R=0.44, p<0.001; Fig. 3l) were positively related to clay content. Latitudinal patterns in Fe-OC content and fFe-OC were observed across global ecosystem types (Fig. 3h, p). Taken together, Fe-OC contents are significantly correlated with both SOC and the Fe-OC Fed molar ratio, which may be important predictors of Fe-OC in global ecosystems.
Moreover, according to RF analysis, the Fe-OC Fed molar ratio and SOC and Fed contents were found to be the most important variables for predicting the Fe-OC content and fFe-OC across global ecosystem types (Fig. 4). Different controlling factors on Fe-OC content and fFe-OC were operational among ecosystem types. At continental scales, the Fe-OC Fed molar ratio was a central driver of the Fe-OC content and fFe-OC, and the contents of SOC and Fed had a more significant influence than the soil pH and climate variables (MAT, MAP) (Fig. 4a, b). The largest influence on Fe-OC content and fFe-OC in marine ecosystems was in the order of Fed > Fe-OC : Fd > SOC > latitude (Fig. 4c, d). In wetlands, the Fe-OC Fed molar ratio was the main driver of Fe-OC, whereas SOC had a more significant role than Fed and soil pH (Fig. 5e). For fFe-OC, the largest influence was in the range of SOC > Fe-OC : Fd > pH > MAT > MAP > Fed (Fig. 4f). The role of Fed content in controlling Fe-OC content and fFe-OC was greater in marine systems than in terrestrial and wetland systems. These results revealed that drivers of both Fe-OC content and fFe-OC were ecosystem specific. The climate predictors accounted for relatively small percentages in all ecosystems. Collectively, Fe-OC : Fd, SOC, and Fed were all selected by RF analysis as important predictors of changes in Fe-OC content and fFe-OC, which agreed with the results of our Spearman's correlation analyses (Fig. 3).
3.3 The vital role of Fe-OC : Fed in controlling Fe OC interactions
At the continental scale, the proportions of Fe-OC Fed molar ratios less than 1 (<1), between 1 and 6 (1–6), and higher than 6 (>6) were 33.10 %, 47.89 %, and 19.01 %, respectively (Fig. 5). Moreover, we found that the proportions of 1–6 were larger in grasslands and farmland than in forests, but the proportions of >6 in grasslands were higher. In marine ecosystems, the proportion of Fe-OC : Fed<1 (31.0 %) is lower than that of 1–6 (63.75 %), and the proportion of >6 (5.31 %) is the smallest. However, the proportion of Fe-OC : Fed>6 (39.44 %) in wetlands was significantly higher than that in other ecosystems (19.01 % and 5.31 %, respectively), but the proportion of <1 (13.55 %) was lower.
Consistent with our expectation, the molar ratio was significantly positively correlated with Fe-OC and SOC contents but negatively correlated with Fed in all ecosystem types (Fig. 6). Moreover, the results showed that MAT and MAP are also major negative regulators of the molar ratio dynamics at the continental scale, while soil pH is the negative regulator in wetland (Fig. 6a, c).
4.1 Reactive Fe promotes SOC preservation at the global scale
In contrast to previous studies (Kramer and Chadwick, 2018; Yu et al., 2021; Ye et al., 2022), our findings suggested that a comprehensive analysis of global patterns of Fe-OC associations across ecosystem types, particularly in wetland and marine ecosystems, can bridge the knowledge gap in understanding the importance of global SOC preservation by reactive Fe. Generally, mineral-associated organic carbon is the dominant SOC pool in soil systems with a proportion of approximately 50 %–80 % of SOC (Cotrufo et al., 2019). As an important component of reactive minerals, Fe (hydr)oxides play a fundamental role in the formation and dynamics of mineral-associated organic carbon (Lalonde et al., 2012). Our findings showed that the average content of Fe-OC was 5.63 ± 0.32 mg g−1 soil (n=862), and the proportion (fFe-OC) of Fe-OC in total SOC was 16.03 ± 0.41 % (n=855) across global ecosystems (Fig. 2a, b), indicating that Fe-OC is essential to the persistence of SOC. Consistent with our expectation, the fFe-OC of wetlands is significantly lower than that of marine and terrestrial systems. At the continental scale, the mean fFe-OC was 17.75 ± 0.90 % (0 %–82.36 %, n=284), which was consistent with findings from Tibetan alpine meadows (15.8 ± 12.0 %) (Fang et al., 2019) but was lower than those for continental-scale forests, such as moist forests (25.3 %–49.8 %) and wet forests (47.1 %–64.1 %) (Zhao et al., 2016; Kramer and Chadwick, 2018). According to upper estimates of global terrestrial SOC storage (971 Pg) (including forest (383 Pg), grassland (423 Pg), and farmland (165 Pg)) (Carter et al., 2000; Lal, 2004b; Pan et al., 2011; Prentice et al., 2001), we estimated that 172.45 ± 8.74 Pg of SOC was bound to Fe oxides in terrestrial ecosystems. Meanwhile, we predicted that 49.02 ± 5.24 Pg (12.80 ± 1.37 %), 74.28 ± 4.95 Pg (17.56 ± 1.17 %), and 28.41 ± 4.34 Pg (17.22 ± 2.63 %) of SOC were associated with Fe oxides in forests, grasslands, and farmlands, respectively. In contrast to terrestrial ecosystems, evidence of interactions between Fe and SOC in marine sediments has been reported more often (Berner, 1970), but the potentially importance of reactive Fe for SOC preservation has only recently been recognized in marine sediments (Lalonde et al., 2012). Recently, an accumulating body of studies has shown that reactive Fe has a strong affinity for SOC, forming stable Fe-OC complexes that can persist for thousands of years in marine sediments, serving as a rusty sink for marine sedimentary carbon (Lalonde et al., 2012; Faust et al., 2021). Our findings suggested that fFe-OC in global marine sediments ranged widely from 0.51 % to 60.3 %, with a mean of 16.32 ± 0.58 %. These values are consistent with published estimates for the East China Sea (13.2 ± 8.9 %) (Ma et al., 2018), Bohai Sea (11.5 ± 8.3 %) (Wang et al., 2019), River Delta (8.1 %–20.2 %) (Shields et al., 2016), Barents Sea (10 %–20 %) (Faust et al., 2021), and global marine surface sediments (21.8 ± 8.6 %) (Lalonde et al., 2012). Based on model-predicted global marine sedimentary OC stocks (150 Pg) (Hedges and Keil, 1995), we further estimated that 24.48 ± 0.87 Pg of the marine sedimentary OC was directly associated with Fe oxides which was comparable to the results of previous study (19–45 Pg OC) (Lalonde et al., 2012). Wetland ecosystems, however, frequently experience seawater flooding, atmosphere exposure, and/or disruption of the hydrological balance due to (semi)diurnal tidal cycles or water table drawdown, in contrast to terrestrial and marine systems (Huang and Hall, 2017; Patzner et al., 2020). Fe-OC associations are weakened with the reductive breakdown of Fe(III) (hydr)oxides driven by periodic soil redox processes (Patzner et al., 2020). Although wetlands store 20 %–30 % of the Earth's soil carbon (∼2500 Pg) (Roulet, 2000; Bridgham et al., 2006), the importance of Fe-OC in wetland soils or sediments remains controversial. In global wetlands, we found that the absolute content of Fe-OC was significantly higher than that in terrestrial and marine ecosystems, whereas the opposite was true for fFe-OC which was significantly lower in wetlands. Our findings in wetlands were also consistent with those of Ye et al. (2022) at continental scales (13.6 ± 1.0 %; Ye et al., 2022) and regional-scale wetlands (16.1 ± 1.4 %) (Wang et al., 2021) but were higher than those for specific peatland ecosystems (3.42 ± 1.32 %) (Huang et al., 2021). Compared with coastal wetlands (for instance, mangrove wetland and tidal wetland) (Bai et al., 2021; Zhao et al., 2022), inland wetlands (for instance, alpine wetland and peatland) have lower fFe-OC (Wang et al., 2017; Huang et al., 2021), which may lead to significantly lower fFe-OC in global wetlands. Therefore, the significance of reactive Fe minerals for SOC sequestration in global wetlands may be underestimated based on peatland fFe-OC (Huang et al., 2022). Here, based on global wetland fFe-OC and total SOC stocks (612 Pg) (Yu et al., 2010), we predicted that 83.84 ± 3.86 Pg of SOC was preserved by binding to Fe oxides. Collectively, these findings confirmed the fundamental role of reactive Fe minerals for OC sequestration and conservation in global ecosystems.
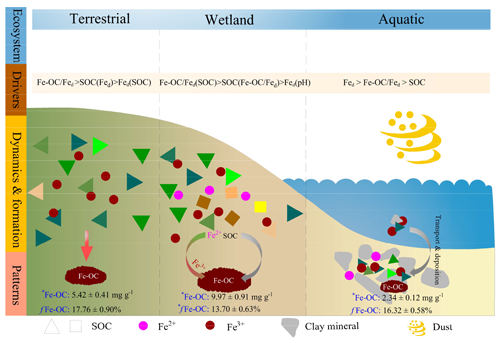
Figure 7Schematic representation of drivers, dynamics, and patterns of Fe-OC associations in different ecosystem types on global scale. Wetland ecosystem included coastal wetlands and inland wetlands, and aquatic ecosystem mainly refers to marine and freshwater ecosystems, but the data of freshwater systems in this study are scarce and dominated by marine systems. Data are averages of different ecosystem types. Different colored triangles and squares represent SOC molecular diversity. There was a lower SOC molecular diversity and concomitant lower contents of Fe-OC (e.g., terrestrial and aquatic ecosystems), whereas higher diversity increases the Fe-OC contents (e.g., wetlands). Meanwhile, there was a significant difference in the proportion of Fe-OC in total SOC (fFe-OC). The asterisk (∗) indicates significant differences.
Two possible mechanisms may explain the higher Fe-OC content in wetlands than in other ecosystems. First, the molar ratios of Fe-OC : Fed were significantly higher in wetlands than in terrestrial and marine ecosystems (p<0.05; Fig. 2d), suggesting that in wetlands reactive Fe is more effective in OC binding (Wagai and Mayer, 2007; Riedel et al., 2013). Numerous studies have shown that the Fe-OC : Fed acts as an indicator of Fe OC interaction types (Lalonde et al., 2012; Wang et al., 2017), with <1 suggesting that the Fe-OC bonding form is dominated by simple mono-layer adsorption, while higher molar ratios (>6) indicate coprecipitation (Wagai and Mayer, 2007; Faust et al., 2021). Thus, compared with other ecosystems, in wetlands coprecipitation played a more significant role in the binding or association of Fe-OC. Second, the SOC content in wetlands was significantly higher than that in terrestrial and marine ecosystems (p<0.05; Fig. 2e), and it is generally believed that the SOC in wetlands has various chemical bonds or chemical compositions (Wang et al., 2017; Coward et al., 2018). Thus, the high SOC content in wetlands could be responsible for the predominance of Fe(II) with a strong OC-complexation capacity (Jones et al., 2015; Bhattacharyya et al., 2018; Patzner et al., 2022), especially the enrichment of phenolic compounds (Freeman et al., 2001), ultimately promoting the Fe-OC association (Riedel et al., 2013; Coward et al., 2018).
4.2 Ecosystem-specific relationships of Fe-OC associations with key factors
The role of soil pH, SOC, Fed, Fe-OC : Fed, MAT, and MAP in controlling Fe-OC contents and fFe-OC among ecosystem types was thoroughly analyzed. A compilation of global datasets including terrestrial, wetland, and marine ecosystems demonstrated that Fe-OC content and fFe-OC are strongly coupled to both the Fe-OC : Fed molar ratio and SOC content ( Fig. 3e, f, m, n), indicating that the two variables are important determinants of Fe-OC content and fFe-OC. The results from the RF models also revealed that Fe-OC : Fed molar ratio, SOC content, and Fed content were important predictors of Fe-OC and fFe-OC across ecosystem types (Fig. 4). Collectively, these findings suggested a generic dependency of Fe-OC and fFe-OC on the Fe-OC : Fed molar ratio and SOC, regardless of their ecosystem types. Former studies on the response of Fe-OC to climate variables and soil properties only concentrated on the continental scale and specific ecosystems with limited data (Ye et al., 2022), making it challenging to reach definitive conclusions. Kramer and Chadwick (2018) concluded that continental-scale Fe-OC variation depended on MAP and potential evapotranspiration but overlooked the role of soil properties (Kramer and Chadwick, 2018). Our findings further showed that the soils with higher MAP were linked with lower soil pH (Fig. 6), which had a positive effect on Fe-OC contents at the continental scale (Fig. 3c), and these results are in line with Ye et al. (2022). Furthermore, we found that Fe-OC content was primarily controlled by the Fe-OC : Fed molar ratio at the continental scale and wetlands (Fig. 7). Given the strong affinity of OC with [Fe(III)] (hydr)oxides, we speculated that an increase in Fed content would lead to higher Fe-OC content, assuming sufficient SOC was present (Ma et al., 2018; Wang et al., 2019). Although reactive Fe plays a fundamental role in OC binding, its content is not related to Fe-OC content in specific terrestrial ecosystems such as the Qinghai–Tibet Plateau and regional-scale forests (Mu et al., 2016; Zhao et al., 2016). Our study, for the first time, illustrated the crucial role of Fed in controlling Fe-OC contents and fFe-OC in global marine ecosystems (Figs. 3g and 4c). Previous findings indicated that increased terrigenous reactive Fe inputs contributed to higher Fe-OC contents (Ma et al., 2018; Wang et al., 2019). Therefore, sedimentary Fed content was the controlling factor of Fe-OC associations in marine ecosystems. The findings of Faust et al. (2021), however, who showed that a higher Fed content does not always enhance Fe-OC associations in Arctic marine sediments, were in contrast to our findings (Faust et al., 2021). The differences between our results and those of Arctic marine sediments may be mainly related to the scale of the study. Nevertheless, the bonding mechanism of Fe and OC (adsorption vs. coprecipitation) is a predominant driver of fFe-OC in wetlands and terrestrial ecosystems, as illustrated by the RF analysis and a good linear correlation. Given that the Fe and OC interactions are substantially controlled by Fe redox processes (Riedel et al., 2013; Adhikari et al., 2016), we posited that the contents and proportions of Fe-OC are governed mainly by Fe redox cycling and associated bonding mechanisms with the exception of the marine ecosystems. The results of this study suggested that future climate warming may increase the proportions of Fe-OC in the total SOC, especially in wetlands (Fig. 3i, j), even though additional research is necessary to fully understand the effects of climate changes on Fe-OC at the global scale.
4.3 Potential bonding mechanism between Fe and OC across ecosystem types
Adsorption and coprecipitation are well-known to be important and well-documented processes for the association of OC and reactive Fe (Lalonde et al., 2012; Chen et al., 2014). Reactive Fe can act as a sorbent of OC to adsorb large amounts of OC to mineral surfaces due to its ubiquity in the environment, high-surface area, and small particle size (Kaiser and Guggenberger, 2003). Riedel et al. (2013) showed that coprecipitated Fe-OC complexes form when reduced Fe is oxidized in the presence of dissolved OC at the oxic–anoxic interface and present a high Fe-OC : Fed molar ratio (Riedel et al., 2013). The Fe-OC : Fed molar ratio can be used as an indicator for the bonding mechanism between Fe and OC (Lalonde et al., 2012; Peter and Sobek, 2018; Faust et al., 2021; Wang et al., 2021), with <1 indicating simple mono-layer sorption and >6 indicating coprecipitation (Tipping et al., 2002; Wagai and Mayer, 2007). Our findings suggested that the average Fe-OC : Fed molar ratio was 10.50 ± 1.91 at the continental scale. However, we could see that the Fe-OC : Fed molar ratios (mean 70.18 ± 13.82; range 2.58–331.68) were much higher in permafrost regions of the Tibetan Plateau than in other specific terrestrial ecosystems, resulting from relatively high Fe-OC and low Fed. (Mu et al., 2016). In view of the very high molar ratio, coprecipitation is the dominant bonding mechanism of OC and Fe, which contributes to fFe-OC reaching 59.5 % (average 19.5 ± 12.3 %) in Fe-poor (range 0.03–2.68 mg g−1 soil) permafrost soils of the Tibetan Plateau (Mu et al., 2016). If the permafrost region of the Tibetan Plateau is excluded, the Fe-OC : Fed molar ratio in global terrestrial ecosystems was only 3.74 ± 0.47, indicating that coprecipitation will become a less important bonding mechanism. Recently, a regional-scale survey including typical grasslands, shrublands, and forests by Wang et al. (2021) reported that the average Fe-OC : Fed molar ratio was 3.0 ± 0.5 (Wang et al., 2021), which lends further credence to the findings mentioned above. The average Fe-OC : Fed molar ratio was 2.56 ± 0.19 (n=320) in global marine ecosystems, similar to that of the Bohai Sea (1.59 ± 1.37) (Wang et al., 2019), Southern Yellow Sea (1.68 ± 1.80) (Ma et al., 2018), East China Sea (1.53 ± 1.28) (Ma et al., 2018), and Barents Sea (2.56 ± 1.76) (Faust et al., 2021), but was much lower than the previous average of global oceans (6.10 ± 7.5) (Lalonde et al., 2012), Arctic shelf (Salvadó et al., 2015), and River Delta (Shields et al., 2016) (Table S1 in the Supplement). Moreover, in wetlands, the molar ratios of Fe-OC : Fed were higher (13.47 ± 1.81) than those in terrestrial and marine ecosystems. These results were in accordance with previous findings in regional-scale wetlands (12.78 ± 2.43) (Wang et al., 2021) and coastal wetlands (11.0 ± 4.5) (Bai et al., 2021) but higher than those in peatlands (mean 6.53) (Huang et al., 2021) (Table S1). This suggested that the interaction between OC and Fe in wetland ecosystems is mainly dominated by coprecipitation at the global scale, usually with a molar ratio of >6. Overall, across the global ecosystem types, the average proportion of Fe-OC : Fed>1 ranged from 60 % to 80 % (Fig. 5), which indicated the importance of both adsorption and coprecipitation interactions. Furthermore, we found that SOC content could enhance the molar ratio of Fe-OC : Fed by positively regulating Fe-OC content. At the continental scale, climate variables (MAT, MAP) can negatively regulate the molar ratio by changing the Fed content (Fig. 6a), while in wetlands soil pH changes the Fe-OC content and then negatively regulates the molar ratio (Fig. 6c). Despite the molar ratio being widely used as an important indicator of the bonding mechanism of Fe and OC, recent studies have shown that only a portion of reactive Fe (25.7 %–62.6 %) was directly associated with OC (Barber et al., 2017). Thus, using the raw Fe-OC : Fe molar ratio may result in an underestimation of the actual molar ratio due to the existence of OC-free Fed (Wang et al., 2019; Faust et al., 2021). At neutral to alkaline pH, associated with arid and semiarid soils, the association of reactive Fe and OC is limited (Sowers et al., 2018a, b), while calcium (Ca) is especially important in OC binding via Ca bridging (Sowers et al., 2018a; Wang et al., 2021). Wang et al. (2021) provided direct evidence that the Fe-OC determined by the classic BCD method contained Ca-bound OC, accounting for approximately 24 % of Fe-OC (Wang et al., 2021), and the Fe-OC : Fed molar ratio might, therefore, be overestimated, for example, in the permafrost regions of the Tibetan Plateau (soil pH 8.01–9.52) (Mu et al., 2016). Therefore, to draw a valid inference on the bonding mechanisms of OC and reactive Fe, further work is necessary to unravel the complex mechanisms.
To our knowledge, this is the first study to reveal the patterns and drivers of Fe-OC across global ecosystems (Fig. 7). More importantly, our global-scale results showed that Fe-OC was an important fraction of SOC at the continental scale, in wetlands, and in marine ecosystems. Our findings highlighted that some drivers for Fe-OC associations are valid globally, but those ecosystem-specific predictors should also be uncovered. Correlation analysis and RF modeling indicated that the Fe-OC : Fed molar ratio and SOC were the predominant predictors of Fe-OC and fFe-OC compared with climate variables and soil pH in global ecosystems. The Fe-OC : Fed molar ratio was the predominant driver of Fe-OC at the continental scale and in wetlands, whereas Fed content was a good predictor in the global marine ecosystem, improving our ability to predict Fe-OC variations among ecosystem types. Moreover, in global wetlands, the fractions of Fe-OC in total SOC may be increasing in response to climate warming. As an indicator of the Fe and OC bonding mechanism, the molar ratio between 1–6 (<1 for adsorption, >6 for coprecipitation) in global ecosystems exceeds 60 %, highlighting the importance of the interactions of both adsorption and coprecipitation. Compared with terrestrial and marine ecosystems, coprecipitation plays a more important role in wetlands due to the high molar ratio. Our findings provide direct evidence that reactive Fe minerals are a dominant natural mechanism for long-term SOC storage in global ecosystems.
The raw data that support the findings of this study are available upon request.
The supplement related to this article is available online at: https://doi.org/10.5194/bg-20-4761-2023-supplement.
QW designed the study; BZ, AMD, ZWZ, ZYC, WBS, and YLF collected the data from various databases; BZ and QW performed the data analysis and prepared the manuscript with contributions from all co-authors; All authors contributed to the review and editing of the manuscript.
The contact author has declared that none of the authors has any competing interests.
Publisher’s note: Copernicus Publications remains neutral with regard to jurisdictional claims made in the text, published maps, institutional affiliations, or any other geographical representation in this paper. While Copernicus Publications makes every effort to include appropriate place names, the final responsibility lies with the authors.
We are very grateful to all the researchers whose data were compiled in this study.
This research has been supported by the National Natural Science Foundation of China (grant no. 32101333), the Fundamental Research Funds for the Central Universities (grant no. lzujbky-2023-ct04), the Key Talent Project of Gansu Province (grant no. 838002), and the Natural Science Foundation of Gansu Province (grant no. 23JRRA1052).
This paper was edited by Luo Yu and reviewed by two anonymous referees.
Adhikari, D., Poulson, S. R., Sumaila, S., Dynes, J. J., McBeth, J. M., and Yang, Y.: Asynchronous reductive release of iron and organic carbon from hematite–humic acid complexes, Chem. Geol., 430, 13–20, https://doi.org/10.1016/j.chemgeo.2016.03.013, 2016.
Anthony, T. L. and Silver, W. L.: Mineralogical associations with soil carbon in managed wetland soils, Global Change Biol., 26, 6555–6567, https://doi.org/10.1111/gcb.15309, 2020.
Bai, J., Luo, M., Yang, Y., Xiao, S., Zhai, Z., and Huang, J.: Iron-bound carbon increases along a freshwater-oligohaline gradient in a subtropical tidal wetland, Soil Biol. Biochem., 154, 108128, https://doi.org/10.1016/j.soilbio.2020.108128, 2021.
Barber, A., Brandes, J., Leri, A., Lalonde, K., Balind, K., Wirick, S., Wang, J., and Gelinas, Y.: Preservation of organic matter in marine sediments by inner-sphere interactions with reactive iron, Sci. Rep.-UK, 7, 366, https://doi.org/10.1038/s41598-017-00494-0, 2017.
Berner, R. A.: Sedimentary pyrite formation, Am. J. Sci., 268, 1–23, https://doi.org/10.2475/ ajs.268.1.1, 1970.
Bhattacharyya, A., Campbell, A. N., Tfaily, M. M., Lin, Y., Kukkadapu, R. K., Silver, W. L., Nico, P. S., and Pett-Ridge, J.: Redox fluctuations control the coupled cycling of iron and carbon in tropical forest soils, Environ. Sci. Technol., 52, 14129–14139, https://doi.org/10.1021/acs.est.8b03408, 2018.
Bridgham, S. D., Megonigal, J. P., Keller, J. K., Bliss, N. B., and Trettin, C.: The carbon balance of North American wetlands, Wetlands, 26, 889–916, https://doi.org/10.1023/A:1010933404324, 2006.
Chen, C., Dynes, J. J., Wang, J., and Sparks, D. L.: Properties of Fe-organic matter associations via coprecipitation versus adsorption, Environ. Sci. Technol., 48, 13751–13759, https://doi.org/10.1021/es503669u, 2014.
Chien, S. C. and Krumins, J. A.: Natural versus urban global soil organic carbon stocks: A meta-analysis, Sci. Total Environ., 807, 150999, https://doi.org/10.1016/j.scitotenv.2021.150999, 2022.
Cotrufo, M. F., Ranalli, M. G., Haddix, M. L., Six, J., and Lugato, E.: Soil carbon storage informed by particulate and mineral-associated organic matter, Nat. Geosci., 12, 989–994, https://doi.org/10.1038/s41561-019-0484-6, 2019.
Coward, E. K., Thompson, A., and Plante, A. F.: Contrasting Fe speciation in two humid forest soils: Insight into organomineral associations in redox-active environments, Geochim. Cosmochim. Ac., 238, 68–84, https://doi.org/10.1016/j.gca.2018.07.007, 2018.
Crowther, T. W., Todd-Brown, K. E., Rowe, C. W., Wieder, W. R., Carey, J. C., Machmuller, M. B., Snoek, B. L., Fang, S., Zhou, G., Allison, S. D., Blair, J. M., Bridgham, S. D., Burton, A. J., Carrillo, Y., Reich, P. B., Clark, J. S., Classen, A. T., Dijkstra, F. A., Elberling, B., Emmett, B. A., Estiarte, M., Frey, S. D., Guo, J., Harte, J., Jiang, L., Johnson, B. R., Kröel-Dulay, G., Larsen, K. S., Laudon, H., Lavallee, J. M., Luo, Y., Lupascu, M., Ma, L. N., Marhan, S., Michelsen, A., Mohan, J., Niu, S., Pendall, E., Peñuelas, J., Pfeifer-Meister, L., Poll, C., Reinsch, S., Reynolds, L. L., Schmidt, I. K., Sistla, S., Sokol, N. W., Templer, P. H., Treseder, K. K., Welker J. M., and Bradford M. A.: Quantifying global soil carbon losses in response to warming, Nature, 540, 104–108, https://doi.org/10.1038/nature20150, 2016.
Delgado-Baquerizo, M., Grinyer, J., Reich, P. B., and Singh, B. K.: Relative importance of soil properties and microbial community for soil functionality: insights from a microbial swap experiment, Funct. Ecol., 30, 1862–1873, https://doi.org/10.1111/1365-2435.12674, 2016.
Eusterhues, K., Rumpel, C., and Kogel-Knabner, I.: Organo-mineral associations in sandy acid forest soils: importance of specific surface area, iron oxides and micropores, Eur. J. Soil Sci., 56, 753–763, https://doi.org/10.1111/j.1365-2389.2005.00710.x, 2005.
Fang, K., Qin, S., Chen, L., Zhang, Q., and Yang, Y.: mineral controls on soil organic carbon stock across tibetan alpine grasslands, J. Geophys. Res-Biogeo., 124, 247–259, https://doi.org/10.1029/2018JG004782, 2019.
Faust, J. C., Tessin, A., Fisher, B. J., Zindorf, M., Papadaki, S., Hendry, K. R., Doyle, K. A., and Marz, C.: Millennial scale persistence of organic carbon bound to iron in Arctic marine sediments, Nat. Commun., 12, 275, https://doi.org/10.1038/s41467-020-20550-0, 2021.
Fisher, B. J., Moore, O. W., Faust, J. C., Peacock, C. L., and März, C.: Experimental evaluation of the extractability of iron bound organic carbon in sediments as a function of carboxyl content, Chem. Geol., 556, 119853, https://doi.org/10.1016/j.chemgeo.2020.119853, 2020.
Freeman, C., Ostle, N., and Kang, H.: An enzymic “latch” on a global carbon store, Nature, 409, 149–149, https://doi.org/10.1038/35051650, 2001.
Grybos, M., Davranche, M., Gruau, G., Petitjean, P., and Pédrot, M.: Increasing pH drives organic matter solubilization from wetland soils under reducing conditions, Geoderma, 154, 13–19, https://doi.org/10.1016/j.geoderma.2009.09.001, 2009.
Guggenberger, G. and Kaiser, K.: Dissolved organic matter in soil: challenging the paradigm of sorptive preservation, Geoderma, 113, 293–310, https://doi.org/10.1016/S0016-7061(02)00366-X,2003.
Hemingway, J. D., Rothman, D. H., Grant, K. E., Rosengard, S. Z., Eglinton, T. I., Derry, L. A., and Galy, V. V.: Mineral protection regulates long-term global preservation of natural organic carbon, Nature, 570, 228–231, https://doi.org/10.1038/s41586-019-1280-6, 2019.
Hopkinson, C. S., Cai, W. J., and Hu, X.: Carbon sequestration in wetland dominated coastal systems – a global sink of rapidly diminishing magnitude, Curr. Opin. Env. Sust., 4, 186–194, https://doi.org/10.1016/j.cosust.2012.03.005, 2012.
Huang, W. and Hall, S. J.: Elevated moisture stimulates carbon loss from mineral soils by releasing protected organic matter, Nat. Commun., 8, 1–10, https://doi.org/10.1038/s41467-017-01998-z, 2017.
Huang, X., Liu, X., Liu, J., and Chen, H.: Iron-bound organic carbon and their determinants in peatlands of China, Geoderma, 391, 114974, https://doi.org/10.1016/j.geoderma.2021, 2021.
Jones, A. M., Griffin, P. J., and Waite, T. D.: Ferrous iron oxidation by molecular oxygen under acidic conditions: The effect of citrate, EDTA and fulvic acid, Geochim. Cosmochim. Ac., 160, 117–131, https://doi.org/10.1016/j.gca.2015.03.026, 2015.
Kaiser, K. and Guggenberger, G.: Mineral surfaces and soil organic matter, Eur. J. Soil Sci., 54, 219–236, https://doi.org/10.1046/j.1365-2389.2003.00544.x, 2003.
Kaiser, K., Mikutta, R., and Guggenberger, G.: Increased stability of organic matter sorbed to ferrihydrite and goethite on aging, Soil Sci. Soc. Am. J., 71, 711–719, https://doi.org/10.2136/sssaj2006.0189, 2007.
Kramer, M. G. and Chadwick, O. A.: Climate-driven thresholds in reactive mineral retention of soil carbon at the global scale, Nat. Clim. Change, 8, 1104–1108, https://doi.org/10.1038/s41558-018-0341-4, 2018.
LaCroix, R. E., Tfaily, M. M., McCreight, M., Jones, M. E., Spokas, L., and Keiluweit, M.: Shifting mineral and redox controls on carbon cycling in seasonally flooded mineral soils, Biogeosciences, 16, 2573–2589, https://doi.org/10.5194/bg-16-2573-2019, 2019.
Lal, R.: Soil carbon sequestration impacts on global climate change and food security, Science, 304, 1623–1627, https://doi.org/10.1126/science.1097396, 2004a.
Lal, R.: Soil carbon sequestration to mitigate climate change, Geoderma, 123, 1–22, https://doi.org/10.1016/j.geoderma.2004.01.032, 2004b.
Lalonde, K., Mucci, A., Ouellet, A., and Gelinas, Y.: Preservation of organic matter in sediments promoted by iron, Nature, 483, 198–200, https://doi.org/10.1038/nature10855, 2012.
Longman, J., Faust, J. C., Bryce, C., Homoky, W. B., and März, C.: Organic carbon burial with reactive iron across global environments, Global Biogeochem. Cy., 36, e2022GB007447, https://doi.org/10.1029/2022gb007447, 2022.
Ma, W. W., Zhu, M. X., Yang, G. P., and Li, T.: Iron geochemistry and organic carbon preservation by iron (oxyhydr) oxides in surface sediments of the East China Sea and the south Yellow Sea, J. Mar. Syst., 178, 62–74, https://doi.org/10.1016/j.jmarsys.2017.10.009, 2018.
McLeod, E., Chmura, G. L., Bouillon, S., Salm, R., Björk, M., Duarte, C. M., Lovelock, C. E., Schlesinger, W. H., and Silliman, B. R.: A blueprint for blue carbon: toward an improved understanding of the role of vegetated coastal habitats in sequestering CO2, Front. Ecol. Environ., 9, 552–560, https://doi.org/10.1890/110004, 2011.
Meisner, A., Gera Hol, W. H., de Boer, W., Krumins, J. A., Wardle, D. A., and van der Putten, W. H.: Plant–soil feedbacks of exotic plant species across life forms: a meta-analysis, Biol. Invasions, 16, 2551–2561, https://doi.org/10.1007/s10530-014-0685-2, 2014.
Mu, C. C., Zhang, T. J., Zhao, Q., Guo, H., Zhong, W., Su, H., and Wu, Q. B.: Soil organic carbon stabilization by iron in permafrost regions of the Qinghai-Tibet Plateau, Geophys. Res. Lett., 43, 10, 286–294, https://doi.org/10.1002/2016GL070071, 2016.
Pan, Y. D., Birdsey, R. A., Fang, J. Y., Houghton, R., Kauppi, P. E., Kurz, W. A., Phillips, O. L., Shvidenko, A., Lewis, S. L., Canadell, J. G., Ciais, P., Jackson, R. B., Pacala, S. W., McGuire, A. D., Piao, S. L., Rautiainen, A., Sitch, S., Hayes, D.: A large and persistent carbon sink in the world's forests, Science, 333, 988–993, https://doi.org/10.1126/ science.120160, 2011.
Patzner, M. S., Mueller, C. W., Malusova, M., Baur, M., Nikeleit, V., Scholten, T., Hoeschen, C., Byrne, J. M., Borch, T., and Kappler, A.: Iron mineral dissolution releases iron and associated organic carbon during permafrost thaw, Nat. Commun., 11, 1–11, https://doi.org/10.1038/s41467-020-20102-6, 2020.
Patzner, M. S., Kainz, N., Lundin, E., Barczok, M., Smith, C., Herndon, E., Kinsman-Costello, L., Fischer, S., Straub, D., Kleindienst, S., Kappler, A., and Bryce, C.: Seasonal fuctuations in iron cycling in thawing permafrost peatlands, seasonal fluctuations in iron cycling in thawing permafrost peatlands, Environ. Sci. Technol., 56, 4620–4631, https://doi.org/10.1021/acs.est.1c06937, 2022.
Peter, S. and Sobek, S.: High variability in iron-bound organic carbon among five boreal lake sediments, Biogeochemistry, 139, 19–29, https://doi.org/10.1007/s10533-018-0456-8, 2018.
Riedel, T., Zak, D., Biester, H., and Dittmar, T.: Iron traps terrestrially derived dissolved organic matter at redox interfaces, P. Natl. Acad. Sci. USA, 110, 10101–10105, https://doi.org/10.1073/pnas.1221487110, 2013.
Roulet, N. T.: Peatlands, carbon storage, greenhouse gases, and the Kyoto Protocol: Prospects and significance for Canada, Wetlands, 20, 605–615, https://doi.org/10.1672/0277-5212(2000)020[0605:PCSGGA]2.0.CO;2, 2000.
Salvadó, J. A., Tesi, T., Andersson, A., Ingri, J., Dudarev, O. V., Semiletov, I. P., and Gustafsson, Ö.: Organic carbon remobilized from thawing permafrost is resequestered by reactive iron on the Eurasian Arctic Shelf, Geophys. Res. Lett., 42, 8122–8130, https://doi.org/10.1002/2015GL066058, 2015.
Schmidt, M. W., Torn, M.S., Abiven, S., Dittmar, T., Guggenberger, G., Janssens, I. A., Kleber, M., Kogel-Knabner, I., Lehmann, J., Manning, D. A., Nannipieri, P., Rasse, D. P., Weiner, S., and Trumbore, S. E.: Persistence of soil organic matter as an ecosystem property, Nature, 478, 49–56, https://doi.org/10.1038/nature10386, 2011.
Shields, M. R., Bianchi, T. S., Gélinas, Y., Allison, M. A., and Twilley, R. R.: Enhanced terrestrial carbon preservation promoted by reactive iron in deltaic sediments, Geophys. Res. Lett., 43, 1149–1157, https://doi.org/10.1002/2015GL067388, 2016.
Smale, D. A., Wernberg, T., Oliver, E. C. J., Thomsen, M., Harvey, B. P., and Straub, S. C., Burrows, M. T., Alexander, L. V., Benthuysen, J. A., Donat, M, Feng, G. M., Hobday, A. J., Holbrook, N. J., Perkins-Kirkpatrick, S. E., Scannell, H. A., Gupta, A. S., Payne, B. L., and Moore, P. J.: Marine heatwaves threaten global biodiversity and the provision of ecosystem services, Nat. Clim. Change, 9, 306–312, https://doi.org/10.1038/s41558-019-0412-1, 2019.
Sowers, T. D., Adhikari, D., Wang, J., Yang, Y., and Sparks, D. L.: Spatial associations and chemical composition of organic carbon sequestered in Fe, Ca, and organic carbon ternary systems, Environ. Sci. Technol., 52, 6936–6944, https://doi.org/10.1021/acs.est.8b01158, 2018a.
Sowers, T. D., Stuckey, J. W., and Sparks, D. L.: The synergistic effect of calcium on organic carbon sequestration to ferrihydrite, Geochem. T., 19, 4, https://doi.org/10.1186/s12932-018-0049-4, 2018b.
Tipping, E., Rey-Castro, C., Bryan, S. E., and Hamilton-Taylor, J.: Al(III) and Fe(III) binding by humic substances in freshwaters, and implications for trace metal speciation, Geochim. Cosmochim. Ac., 66, 3211–3224, https://doi.org/10.1016/S0016-7037(02)00930-4, 2002.
Wagai, R. and Mayer, L. M.: Sorptive stabilization of organic matter in soils by hydrous iron oxides, Geochim. Cosmochim. Ac., 71, 25–35, https://doi.org/10.1016/j.gca.2006.08.047, 2007.
Wan, D., Ye, T. H., Lu, Y., Chen, W. L., Cai, P., Huang, Q. Y.: Iron oxides selectively stabilize plant-derived polysaccharides and aliphatic compounds in agricultural soils, Eur. J. Soil Sci., 70, 1153–1163, https://doi.org/10.1111/ejss.12827, 2019.
Wang, D., Zhu, M. X., Yang, G. P., and Ma, W. W.: Reactive iron and iron-bound organic carbon in surface sediments of the river-dominated Bohai Sea (China) versus the Southern Yellow Sea, J. Geophys. Res-Biogeo., 124, 79–98, https://doi.org/10.1029/2018JG004722, 2019.
Wang, S., Jia, Y., Liu, T., Wang, Y., Liu, Z., and Feng, X.: Delineating the role of calcium in the large-scale distribution of metal-bound organic carbon in soils, Geophys. Res. Lett., 48, e2021GL092391, https://doi.org/10.1029/2021GL092391, 2021.
Wang, Y., Wang, H., He, J. S., and Feng, X.: Iron-mediated soil carbon response to water-table decline in an alpine wetland, Nat. Commun., 8, 1–9, https://doi.org/10.1038/ncomms15972, 2017.
Ye, C., Huang, W., Hall, S. J., and Hu, S.: Association of organic carbon with reactive iron oxides driven by soil ph at the global scale, Global Biogeochem. Cy., 36, e2021GB007128, https://doi.org/10.1029/2021GB007128, 2022.
Yu, C., Xie, S., Song, Z., Xia, S., and Åström, M. E.: Biogeochemical cycling of iron (hydr-) oxides and its impact on organic carbon turnover in coastal wetlands: A global synthesis and perspective, Earth-Sci. Rev., 218, 103658, https://doi.org/10.1016/j.earscirev.2021.103658, 2021.
Zhao, B., Jia, Y., Wu, S., Wei, L., Li, J., Hong, H., Yan, C., Williams, M. A., and Wang, Q.: Preservation of soil organic carbon in coastal wetlands promoted by glomalin–iron–organic carbon ternary system, Limnol. Oceanogr., 67, 180–192, https://doi.org/10.1002/lno.12238, 2022.
Zhao, Q., Poulson, S. R., Obrist, D., Sumaila, S., Dynes, J. J., McBeth, J. M., and Yang, Y.: Iron-bound organic carbon in forest soils: quantification and characterization, Biogeosciences, 13, 4777–4788, https://doi.org/10.5194/bg-13-4777-2016, 2016.
Zong, M., Lin, C., Li, S., Li, H., Duan, C., Peng, C., Guo, Y. M., and An, R.: Tillage activates iron to prevent soil organic carbon loss following forest conversion to cornfields in tropical acidic red soils, Sci. Total Environ., 761, 143253, https://doi.org/10.1016/j.scitotenv.2020.143253, 2021.