the Creative Commons Attribution 4.0 License.
the Creative Commons Attribution 4.0 License.
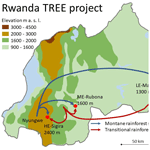
Thermophilisation of Afromontane forest stands demonstrated in an elevation gradient experiment
Bonaventure Ntirugulirwa
Etienne Zibera
Nkuba Epaphrodite
Aloysie Manishimwe
Donat Nsabimana
Johan Uddling
The response of tropical trees and tree communities to climate change is crucial for the carbon storage and biodiversity of the terrestrial biosphere. Trees in tropical montane rain forests (TMFs) are considered particularly vulnerable to climate change, but this hypothesis remains poorly evaluated due to data scarcity. To reduce the knowledge gap in the response of TMF trees to warming, we established a field experiment along a 1300–2400 m elevation gradient as a proxy for warming in Rwanda. Seedling-size trees of 20 species native to montane forests in eastern and central Africa were planted in multi-species plots at three sites along the gradient. They have overlapping distributions but primarily occur in either transitional rain forests (∼ 1600–2000 ) or mid-elevation TMFs (∼ 2000–3000 ), with both early- (ES) and late-successional (LS) species represented in each elevation origin group. Tree growth (diameter and height) and survival were monitored regularly over 2 years. We found that ES species, especially from lower elevations, grew faster at warmer sites, while several of the LS species, especially from higher elevations, did not respond or grew slower. Moreover, a warmer climate increased tree mortality in LS species, but not much in ES species. ES species with transitional rain forest origin strongly increased proportional to stand basal area at warmer sites, while TMF species declined, suggesting that lower-elevation ES species will have an advantage over higher-elevation species in a warming climate. The risk of higher-elevation and LS species of becoming outcompeted by lower-elevation and ES species due to a thermophilisation response in a warmer climate has important implications for biodiversity and carbon storage of Afromontane forests.
- Article
(2105 KB) - Full-text XML
-
Supplement
(3422 KB) - BibTeX
- EndNote
The global average temperature has already increased by 1.09 ∘C from the period 1850–1900 to 2020 and is projected to further increase by the end of this century to between 1.4 and 4.4 ∘C, depending on CO2 emission scenario, SSP1-1.9 and SSP5-8.5, respectively (Arias et al., 2021). Although warming in the tropical region is predicted to be less than in the boreal region (Arias et al., 2021), the warming sensitivity of tropical trees may be larger since they are adapted to a thermally stable environment (Doughty and Goulden, 2008; Ghalambor et al., 2006; Janzen, 1967) and may have a lower capacity to acclimate to increased temperature compared to plants from more seasonal regions (Crous et al., 2022; Cunningham and Read, 2002; Way and Oren, 2010).
Forest monitoring data have shown that increasingly warm and dry conditions during recent decades have caused a decline in the Amazon carbon sink (Brienen et al., 2015). More recently, there have been indications of this occurring also in the Congo basin (Hubau et al., 2020). On top of these long-term trends, pronounced negative effects of warm and dry conditions during El Niño years caused negative effects on tree growth in Central America, Amazonia, western and central Africa, and south-western Asia as well as increased tree mortality in Amazonia (Clark et al., 2003; Lewis et al., 2011; Rifai et al., 2018). Both heat and drought contribute to these trends and patterns (Jung et al., 2017), and the two stress factors likely interact such that heat (and the associated increase in water vapour pressure deficit, VPD) increases the severity of drought stress and vice versa (Killi et al., 2017; Zhao et al., 2013; Bauman et al., 2021, 2022; Humphrey et al., 2021). However, due in part to common co-variation in heat and drought, the direct effect of warming on tropical forests remains unclear (Docherty et al., 2022; Piao et al., 2020; Wang et al., 2014; Zhou et al., 2013). While negative effects of decreasing precipitation have been demonstrated in stand-scale throughfall exclusion experiments (da Costa et al., 2010; Estiarte et al., 2016; Meir et al., 2014; Nepstad et al., 2007; Rowland et al., 2021), large-scale-warming field experiments are lacking. This severely hampers our ability to predict the fate of the tropical carbon sink in a warming world.
Global meta-analyses of experimental data with trees indicate that warming usually has positive effects on leaf net photosynthesis and growth in cooler biomes but negative effects in the tropics (Crous et al., 2022; Lin et al., 2010; Way and Oren, 2010). While warming has an overall negative effect on tropical tree growth across studies (Way and Oren, 2010), it should be emphasised that this is not always the case. Large variability across studies and species has been observed, from positive to negative effects (e.g. Slot and Winter, 2018; Dusenge et al., 2021; Wittemann et al., 2022). This may reflect large variation in species origin temperature range and how this compares to experimentally applied temperature treatments, with positive effects being more likely if the origin climate is rather cool, and the warming treatment is modest.
Tropical montane rain forests (TMFs) are considered particularly vulnerable to changes in the environment, but our knowledge on how they will respond to climate change is currently very limited (Boehmer, 2011; Salinas et al., 2021; Tovar et al., 2022). TMFs occur on all continents within the tropical biome. The lower elevation limit varies widely between and within continents, but using a general lower limit of 1000 , the cover has been estimated to be 8 % of the total tropical forest area (Spracklen and Righelato, 2014). TMFs provide important ecosystem services, such as regulation of regional climate and hydrology (Alamgir et al., 2016; Martínez et al., 2009; Soh et al., 2019); storage of surprisingly large amounts of carbon, especially on the African continent (Cuni-Sanchez et al., 2021; Nyirambangutse et al., 2017; Okello et al., 2022); and hosting of high levels of biodiversity and endemism (Kessler and Kluge, 2008). Warming may have smaller direct negative effects on higher-elevation species growing in cooler TMF environments (at their current distribution) than on lower-elevation species which may already grow near or at their thermal optimum (Feeley et al., 2020). On the other hand, a warmer climate can make lower-elevation species more competitive at higher elevations, over time shifting species distributions upwards at the expense of current higher-elevation species. Moreover, a warmer climate may shift the competitive balance between species with overlapping distributions to the disadvantage of those with ranges centred at higher, cooler elevations. Such a shift has recently been observed in Andean montane forests (Duque et al., 2015; Fadrique et al., 2018). The tree community composition shift with warming observed in these studies was caused primarily by increased mortality in higher-elevation species, leading to increasing relative abundance of species with ranges centred at lower elevations, so-called “thermophilisation”. These findings show that there is considerable variation in warming sensitivity among co-existing species and that this is linked to species distribution range and thermal niche. The underlying mechanisms behind the variation are not well understood, but a recent study on four species from contrasting elevation origins indicates that species differences in physiological temperature sensitivities play a role (Wittemann et al., 2022). Such species differences may be related to different growth strategies and the associated traits.
Tree species dominating in secondary and primary forests are examples of species groups with different growth strategies, i.e. early- and late-successional species, respectively. The early-successional species are acquisitive and fast-growing, with low wood density, while late-successional species are more conservative and slow-growing, with higher wood density. Secondary forests have progressively become more common at the expense of primary forests due to land-use changes and now represents > 50 % of all tropical forests (Chazdon et al., 2009). However, it remains uncertain if the early-successional species are winners or losers in a warming climate and thus if climate change will amplify the expansion of secondary forests or not. There are experimental indications that photosynthesis and growth respond more negatively to warming in late-successional species (Carter et al., 2020; Cheesman and Winter, 2013a; Dusenge et al., 2021; Mujawamariya et al., 2023; Slot and Winter, 2018; Tarvainen et al., 2022; Vårhammar et al., 2015). However, these studies included only few species representing early- and late-successional strategies, used artificial heating in chambers/infrared heaters, or only looked at physiology. Field monitoring in a TMF in Kenya showed that the effects of high temperature on tree stem growth were more negative in late- compared to early- and mid-successional species (Gliniars et al., 2013). In contrast, early-successional species have been found to be more susceptible to climate-induced mortality compared to late-successional species (Aleixo et al., 2019; Bauman et al., 2022; Elias et al., 2020; Feng et al., 2017; Herrera-Ramírez et al., 2021). A possible explanation of these discrepancies might be that early-successional species are more drought-sensitive (Elias et al., 2020), while late-successional species are more heat-sensitive.
Knowledge about climate and climate change responses of different tropical tree species is also crucial for tree plantation efforts. Historically, exotic species have been preferred in most tropical areas (Sands, 2013). However, many current plantation programmes aim to increase the use of native tree species to diversify and enhance ecosystem services (Bremer and Farley, 2010; Galhena et al., 2013; Thomas et al., 2014), resilience to different stress factors (Aguirre-Gutiérrez et al., 2022; Brasier, 2008; Woodcock et al., 2017), and biodiversity (Brockerhoff et al., 2008; Stephens and Wagner, 2007). Moreover, plantations with a mixture of native species may be hydrologically preferable for nearby agriculture compared to monocultures with fast-growing exotic species (Sena et al., 2014). However, the current ambitions to promote the use of native tree species in tropical plantations is hampered by limited knowledge of their climate sensitivity, leading to large risks of failure if species are planted in the wrong place or without consideration of likely climate change during the coming decades (Aitken et al., 2008; Forrester et al., 2005; Thomas et al., 2014).
Elevation gradients offer the potential to study temperature responses of plants and ecosystems under ecologically realistic conditions (Körner, 2007; Malhi et al., 2010; Tito et al., 2020). However, most studies conducted along tropical elevation gradients have focused on characterising differences in plant and ecosystem traits of existing natural vegetation, with different species or ecotypes being present at different elevations (Báez et al., 2015; Cuesta et al., 2017; Fadrique et al., 2018; Malizia et al., 2020; Mujawamariya et al., 2018). While such studies are relevant for exploring how climate shapes plant communities and ecosystems in the long term, they say little about the responses of trees under ongoing rapid climate change. Studies that integrate elevation gradient approaches with experimental manipulations have been suggested to bridge the gap between large-scale observational studies and smaller-scale controlled experiments with limited ecological realism (Sundqvist et al., 2013). A powerful but underused approach is to use multi-species plantations with genetically similar plant material established at different elevations. If tree species are planted in mixed stands, such studies also offer the possibility of exploring climate change effects on tree stand composition. That is the approach taken in this study.
We investigated the growth and mortality responses of 20 tree species originating from two elevation ranges of African upland forests (montane and transitional rain forests having their mid-point of origin above and below 2000 , respectively). Seedling sized trees were planted in large and mixed multi-species plantations along an elevation gradient ranging from 2400 down to 1300 in Rwanda. The elevation gradient was used as a proxy for possible future warming, and irrigation was applied to compensate for variation in rainfall along the gradient. The overall aim of the study was to determine the effect of a warmer climate on tree growth and mortality of tropical upland tree species with contrasting successional strategies and origin climate. Since this is a stand-level study, we could also assess warming impacts on tree stand composition within the experimental sites. Our specific aims were to test the following hypotheses:
- H1.
Tree growth responses to a warmer climate are more positive (or less negative) in early- than in late-successional tree species.
- H2.
The warmer climate at lower elevation decreases tree growth in species with their central distribution in montane rain forests (> 2000 ), while it stimulates growth of species predominantly occurring in transitional rain forests (< 2000 ).
- H3.
Mortality increases in a warmer climate, and this is more pronounced in high-elevation and late-successional compared to lower-elevation and early-successional tree species.
- H4.
Interspecific variation in growth and mortality responses leads to altered tree community composition in a warmer climate, favouring lower-elevation and early-successional tree species.
Table 1Weather and soil characteristics of the sites along the elevation gradient. Weather data show mean ± standard deviation for 2 years, from 1 February 2018 to 31 January 2020. Soil T and SWC are measured at 10–20 cm depth on six plots, and other soil data variables represent a mean of 0–30 cm depth of three blocks at each site. Wind, soil T, and SWC were only measured during the 2019/20 period. HE, high elevation; ME, mid-elevation; LE, low elevation.
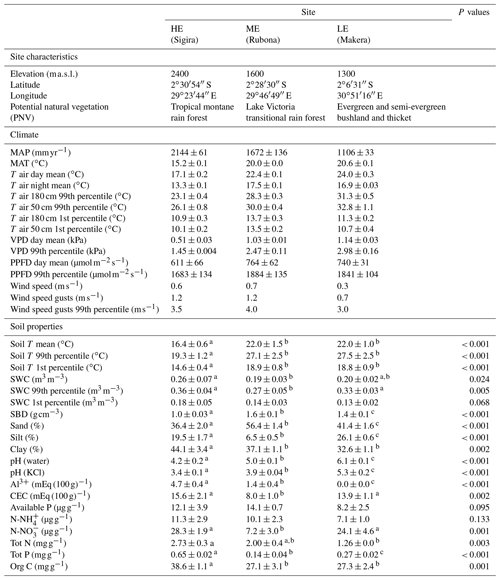
PNV, defined by Kindt et al. (2014); MAP, mean annual precipitation; MAT, mean annual temperature; T air day, day temperature (PPFD > 2 ); T night, night temperature (PPFD < 2 ); VPD, vapour pressure deficit; PPFD, photosynthetic photon flux density; T soil, soil temperature at 10–20 cm depth; SWC, soil water content at 10–20 cm depth; SBD, soil bulk density; CEC, cation exchange capacity; Tot N and P, total nitrogen and phosphorus concentration; Org C, organic carbon concentration. Different letters after values indicate significant differences (P<0.05) between sites.
2.1 Experimental sites
Growth and mortality of tropical trees were studied within the TRopical Elevation Experiment in Rwanda (Rwanda TREE; see http://www.rwandatree.com, last access: 12 December 2023) over 2 years after planting (planting period was January 2018 to December 2019). Mixed plantations with 20 tropical tree species native to eastern and central Africa were established at three sites along an elevation gradient from 2400 to 1300 (Table 1, Fig. S1 in the Supplement). From the highest to the lowest elevation, over a distance of ca. 170 km, the mean annual temperature (MAT) increased by 5.4 ∘C, while mean annual precipitation (MAP) decreased by approximately 50 %. The high-elevation (HE) site (2400 ) is located at Sigira in the district of Nyamagabe (2∘30′54′′ S, 29∘23′44′′ E) in close proximity to the plantation buffer zone surrounding Nyungwe National Park (NNP), a TMF in the south-western part of Rwanda. The mid-elevation (ME) site (1600 ) is located at Rubona agricultural research station in the district of Huye (2∘28′30′′ S, 29∘46′49′′ E), ca. 43 km south-east from the Sigira site. The low-elevation (LE) site (1300 ) is located at Ibanda Makera in the district of Kirehe (2∘6′31′′ S, 30∘51′16′′ E), ca. 126 km north-east of the Rubona site, in the eastern part of Rwanda, near the border with Tanzania. The sites were located in different zones of potential natural vegetation (Kindt et al., 2014): HE in the Afromontane tropical rain forest, referred to as the TMF or montane forest zone; ME in the Lake Victoria transitional rain forests, referred to as LVTFs or transitional forests; and LE in the evergreen and semi-evergreen bushland and thicket (Table 1, Fig. S1). Although many of the selected species are distributed in both montane and transitional forests, the HE site (Sigira) is considered as the control site in this experiment since today's remaining natural forests are predominantly montane, and all species except one can be found at > 2000 m elevation (Fig. 1). Furthermore 18 out of 20 species used in this experiment naturally grow in the neighbouring NNP, ranging from 2950 down to 1600 (Fisher and Killmann, 2008; Nyirambangutse et al., 2017). With the HE site as a control, the ME (Rubona) and LE (Makera) sites represent two different warming scenarios.
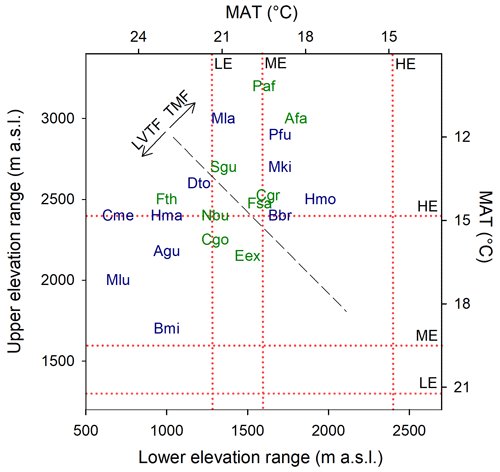
Figure 1Commonly observed natural elevation ranges for the species used in the experiment (Bloesch et al., 2009; Fischer and Killmann, 2008; Kindt et al., 2014; Nyirambangutse et al., 2017). The mean annual temperature (MAT) scale was developed from a linear regression (R2 = 0.95) of MAT vs. elevation, using data from eight locations in Rwanda. The diagonal dashed black line separates the species originating from the tropical montane forest (TMF) and the Lake Victoria transitional forest (LVTF). The elevation of the experimental sites (high – HE/Sigira; mid – ME/Rubona; low – LE/Makera) are indicated by the dotted red lines. Species codes in blue and green are early- and late-successional species, respectively. For full species names, see Table 2.
2.2 Experimental design and plot preparation
Before establishment of plantations, all vegetation consisting of bushes and trees was cleared, and large roots were removed. At each site, 18 plots with an area of 15 m × 15 m, spaced by 2.5 m paths, were established on a 50 m × 102.5 m planimetric area. Within each plot, 20 different species with a replication of 5 (i.e. 100 trees per plot) were planted with 1.5 m × 1.5 m spacing and randomised positioning of each individual tree. The total number of trees at each site was 1800 (i.e. 18 plots × 100 trees). The 18 plots allowed for a full factorial experimental design, with three water levels and two fertility levels and a replication of three plots for each of the six treatment combinations. Before mid-July 2019, all trees at all sites received water when needed, irrespective of the subsequent planned water treatment. During the first dry period in July–August 2018 all trees were manually irrigated, while all plants were exposed to the dry period from mid-July to the end of August 2019. The water and nutrient treatments started in September and November 2019, respectively. No significant (P > 0.05) effect on diameter and height growth of the treatments was observed during this period, likely because September to December is within the rainy season. We therefore use averages of all plots in this study. Site maps with experimental design are presented in Figs. S2–S4 in the Supplement.
Table 2Taxonomy of species, their functional type, and ecological and geographical distribution. TMF, tropical montane forest (≳ 2000 ); LVTF, Lake Victoria transitional forest (∼ 1600–2000 ), ES, early-successional; LS, late-successional. The distribution is classified into western (W), central (C), eastern (E), and southern (S) Africa.
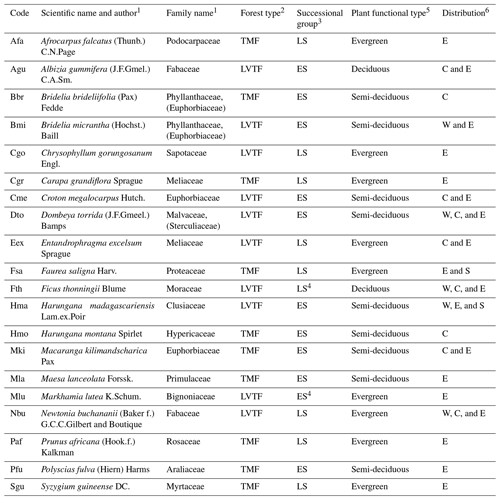
1 Taxonomy information from World Flora Online (http://www.worldfloraonline.org (last access: 26 September 2023). Family names are given according to the Angiosperm Phylogeny Group, APG III, system, and when applicable earlier family names are given in brackets). 2 forest types follow the potential natural vegetation by Kindt et al. (2014). 3 sources of successional group classification are given in the Table S4. 4 the classification of Fth and Mlu into distinct successional groups is ambiguous, but according to our assessment they belong mostly to the LS and ES groups, respectively (see Table S4), and will be treated as such. 5 semi-deciduous species drop a variable amount of leaves mainly depending on drought period severity but are rarely completely defoliated. 6 according to our definition, central and eastern Africa distribution areas overlap in Rwanda, and thus trees with both C and E distributions will be found there, but only the main distribution areas are given.
2.3 Plant material
The tree species included in the experiment were selected to represent important and common species in two eastern and central African forest types: montane forest (TMF > 2000 ) and transitional forest (LVTF ∼ 1600–2000 ); see Fig. 1 for species elevation ranges. From each forest type, species representing both early-succession (ES) and late-succession (LS) strategies were selected with the following number of species per combination: five ES–TMF, five LS–TMF, six ES–LVTF, and four LS–LVTF (Table 2). Note that from the literature, the classification Ficus thonninghii and Markhamia lutea into distinct successional groups is ambiguous (Tables 2 and S4 in the Supplement), but according to our assessment they belong mostly to late- and early-successional groups, respectively, and are treated as such here. The species represent a mix of mostly evergreen and semi-deciduous trees, but also two deciduous species were included (Table 2).
The trees were propagated from seeds, cuttings, or naturally generated seedlings, depending on species-specific difficulties of propagation, in poly-pots in a nursery at Rubona research station (near the ME site) in 2017. The germplasms were collected from Nyungwe and Ndiza montane forests (high elevation) or Rubona research station and Ruhande arboretum, located in the transitional forest vegetation zone (mid-elevation), depending on forest type of origin. After 6 to 12 months in the nursery and having reached a height of ∼ 5–75 cm, depending on species, each plant was randomly assigned to a site, plot, and plot position (as constrained by the experimental design) and transplanted to the experimental plots within a period of approximately 1 month at the turn of the year from 2017 to 2018. Details of the propagation and initial sizes of the trees are given in Tables S1–S3 in the Supplement.
2.4 Environmental conditions
Weather stations were installed in an open space next to the plantations at all Rwanda TREE sites, equipped with sensors for measurements of temperature, relative humidity, solar radiation, precipitation, and wind direction and speed (VP-3/VP4, QSO-S PAR, ECRN-100, and Davis cup anemometer, respectively, from Meter Group Inc., Pullman, WA, USA) connected to data logger (Em50G, Meter Group Inc.). In addition, soil temperature and soil water content sensors (Teros 12, Meter Group Inc.) were installed in the centre of six plots connected to a data logger (Em60G, Meter Group Inc.). The recording of most parameters started late January 2018, while wind and soil measurements started late August 2018 (Table 1). All parameters were recorded every 30 min. The MATs measured from 1 February 2018 to 31 January 2020 at the HE site (Sigira), ME site (Rubona), and LE site (Makera) were 15.2, 20.0, and 20.6 ∘C, respectively. The daytime and extreme temperatures (expressed as the 99th percentile) were 17.1 and 23.1 ∘C at the HE site, 22.4 and 28.4 ∘C at the ME site, and 24.0 and 31.2 ∘C at the LE site. The larger difference in the daytime and extreme temperatures compared to MAT between the ME and LE sites was mainly due to a larger diurnal range and lower nighttime temperatures at the LE site. Also, MAP differed between sites, decreasing progressively from Sigira (2144 mm) to Rubona (1672 mm) and Makera (1106 mm; Table 1). However, the relative seasonal distribution of precipitation was similar at all sites, with the highest rainfall in March–May and a dry period in June–August. Solar radiation was similar at the ME and LE sites, while the HE site received on average less radiation, probably due to higher cloudiness as only small differences in monthly maximum radiation were observed between the sites (not shown). Differences in high light climate may influence species with early- and late-successional strategies differently (Ntawuhiganayo et al., 2020), but we believe that the on average 8.5 % lower high radiation at the HE site compared to the LE site likely has very limited effects. Soil temperatures were closely related to the site MAT, although they were probably also affected by radiation and canopy cover. Soil water content (SWC) was similar at the LE and ME sites in spite of differences in MAP and soil texture (Table 1). The mean SWC at the HE site was substantially higher compared to the two other sites, probably because of both higher MAP, higher water-holding capacity due to higher soil clay content (Table 1), and lower evapotranspiration due to lower air temperature.
2.5 Soil characteristics
Before planting the trees, in November 2017 soil samples were collected at 0–10 and 20–30 cm in the centre of each plot using soil sample rings (∅ 53 mm, Ejkelkamp soil and water, Giesbeek, the Netherlands). Soil bulk density (SBD), , , and available P were analysed from composite samples of two adjacent plots, while the remaining soil parameters were analysed from composite samples of six plots. All values are presented as averages of 0–30 cm depth and as the mean and standard deviation of three samples per site (each combining six plots), representing three blocks per site (Table 1). Oven-dried (70 ∘C) soil samples of known volume were used to determine SBD. Samples for analysis of total N and P were pre-ground using a mortar and pestle and further ground to a fine powder with a ball mill (model MM 301, Retsch, Haan, Germany). N and P concentrations were determined by dry combustion using an elemental analyser (EA 1108, Fison Instruments, Rodano, Italy) and inductively coupled plasma mass spectrometry (AQ250, ACME Analytical Laboratories, Vancouver, BC, Canada), respectively. For all other parameters, fresh soil samples were sent for analysis to the Soil and Plant Analytical Laboratory at the Rwanda Agriculture and Animal Resource Development Board (RAB) at Rubona research station, Rwanda.
The main soil texture differences between sites were a larger proportion of sand and less silt at Rubona (53 %–62 % and 5 %–9 %, respectively) compared to the soil at the Sigira and Makera sites (35 %–45 % and 15 %–27 %, respectively), while the clay content was relatively high at all sites (30 %–50 %). The soil pH (water) was 4.2 at the HE site and increased by approximately one unit for each step down the elevation gradient, which explains the decline in Al3+ with decreasing elevation but does not affect the cation exchange capacity (CEC). Soil fertility expressed as total N and P content tended to decline with decreasing elevation, partly explained by higher organic content at the HE site compared to the other sites. However, the available N (, ) and P were not significantly affected by elevation, but was lower at the ME site.
2.6 Growth and mortality monitoring
The stem height (h) and diameter (D) of each tree were measured trimonthly during the first 2 years, starting at planting (December 2017/January 2018) and resulting in eight censuses. The measurement of D was taken using callipers, while h was measured with a measuring stick or, for larger trees, a telescopic measuring pole. For species having several stems, only the main stem (tallest) was measured regularly and presented in this study, but we also report the average number of living stems for each species observed during the seventh census (late September to early October 2019). At each census, dead trees or trees with reduced vitality were recorded. The D measurements of trees with a h below and above 250 cm diameter were done at the stem base (∼ 5 cm above ground, Dbase) and at breast height diameter (Dbh), respectively. To compare the diameter of all trees irrespective of h, parallel measurements of Dbh and Dbase were made during two consecutive censuses after the trees had reached a h of 250 cm as well as after 3 years. These data were used to develop species-specific functions to estimate the Dbase for all trees on which Dbase was not measured and at all census occasions using the following equation:
where k and a are species-specific constants determined from the change in ratio with h. Note that when roots were causing stem irregularities (e.g. swelling), the Dbase was measured above 5 cm from the ground. As quality assurance, all D and h data were plotted against time to identify outliers and missing values which were replaced by interpolated values. The interpolated values corresponded to < 1.5 % of the Dbase and < 0.5 % of the h values out of 43 200 recordings for each variable.
2.7 Relative growth rate of Dbase and h
The relative growth rates (RGRs) of both Dbase and h of the trees were calculated using the standard RGR equation:
where X1 and X2 corresponds to Dbase or h of the beginning and end, respectively, of each trimonthly census time interval (t1 and t2, respectively). Since RGR varies with tree size (Fig. S5 in the Supplement) the values were size-standardised to be able to make size-independent RGR comparisons of different species and sites. This was done for one small and one large tree size category, by averaging the RGR for Dbase within ranges of 10–25 and 50–75 mm (D-RGRD10–25 and D-RGRD50–75) and for h within ranges of 75–100 and 250–300 cm (H-RGRH75–100 and H-RGRH250–300), respectively.
2.8 Tree stand composition
The site effect on tree stand composition was analysed by comparing the basal area (BA) of the four species groups, ES–TMF, ES–LVTF, LS–TMF, and LS–LVTF. The BA is a stand-level measure defined as the sum of cross-sectional stem area (Ac, calculated from Dbase values) of living individuals expressed per ground area. The BA of each species group was calculated as follows:
where Ap is the plot area (225 m2), and n and d are the numbers of living and dead individuals per plot, respectively, within each species group. The term is to normalise for initial difference in number of individuals (i.e. number of species × 5 replicates per plot) between species groups (Table 2) but also to include both living and dead trees in the effect on BA. Absolute (cm2 m−2) and fractional (percent of plot area) BA for each species group are presented as BAabs and BAfrac, respectively. The influence of mortality on BA for the four species groups was analysed by comparing the result from Eq. (3) with and without the term d. Although this will not consider the dynamic effect of neighbouring trees exploring the space of the dead trees and thus potentially having larger Dbase growth, it will give a reasonable estimate of the importance of mortality for BA of different species groups. Note that the BAabs and BAfrac of the four species groups represented approximately 25 % each at the beginning of the experiment.
2.9 Statistics
The site and species effects on Dbase, h (eighth census), number of stems per individual (seventh census), D-RGRD10–25, D-RGRD10–25, H-RGRH75–100, and H-RGRH250–300 were analysed using a two-way ANOVA with site and species as fixed factors. The site and species group effects on BAabs and BAfrac were analysed using a two-way split plot (split between the four species groups within each plot) ANOVA with species groups as within- and sites as between-subject factors. In the case of significant site–species/species group interactions, the site effect was analysed separately for each species/species group using a one-way ANOVA followed by Tukey's post hoc test. This latter type of analysis was also made for soil variables. All effects were analysed by using plot-level means (n=18 or less when applicable per site). A test of normality (Shapiro–Wilk) was performed to test the distribution of data, and Levene's test was performed for homogeneity of variance. Normality was mostly obtained for analysis of individual species and sites. Homogeneity of variance was mostly obtained for analysis of individual species and species groups (for BA) but not when all species or species groups were analysed together. Effects were considered statistically significant at P < 0.05 if homogeneity of variance was obtained and at P < 0.01 when homogeneity was not obtained. Furthermore, to reduce the potential bias caused by non-homogeneity, species with an unequal replication of RGR data at the plot level (ratio of > 1.5 of highest and lowest number of plot replicates between sites) were not included in the statistical analysis. This reduced the number of species included in the standardised RGR analysis and is denoted “not applicable” (n.a.) in the presentation of statistical results. Greenhouse–Geisser correction was used to adjust degrees of freedom (df's) for within-subject factors violating the sphericity assumption according to Mauchly's test. A generalised linear regression model with Poisson distribution was used to analyse the site and species effect on tree mortality using records from individual trees. As the site–species interaction was significant, the site effect for each species was analysed by paired comparison of the site using the same method. To reduce the effects of multiple analysis of site effects the significant P values were reduced to < 0.01. All statistical tests were made by using the SPSS 28.0 software package (SPSS, Inc., Chicago, IL, USA).
Table 3Results from two-way ANOVA for site and species (Sp.) effects on stem diameter at the base (Dbase), stem height, number of stems per individual (no. of stems), absolute basal area (BA) of species categories, and standardised relative growth rates (RGR) of Dbase at a Dbase of 10–25 mm (D-RGRD10–25) and 50–75 mm (D-RGRD50–75) and height at a height of 75–100 cm (H-RGRH75–100) and 250–300 cm (H-RGRH250–300) as well as Poisson regression for tree mortality. The ANOVA was based on plot averages, while the Poisson regression was based on individual trees. Species that did not meet the criterion for balanced number of plot replicates (i.e. at least one individual on each of ≥ 12 plots per site) or had zero mortality were not included in the analysis. No. sp., number of species; n.a., not applicable. See Figs. 2 and 3 for post hoc tests.
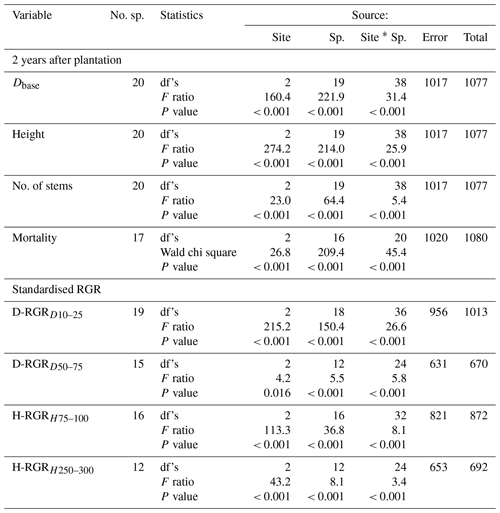
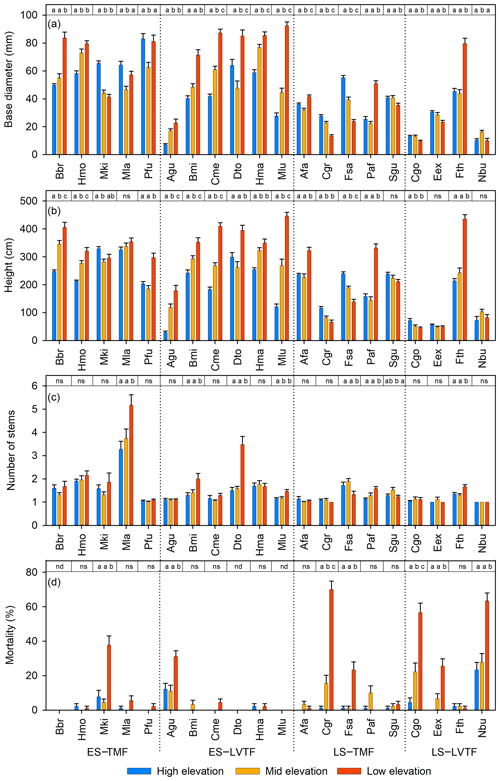
Figure 2(a) Stem height, (b) stem diameter at base, (c) number of stems from ground per individual, and (d) mortality in percent of planted individuals 2 years after plantation (except number of stems recorded 21 months after plantation) of each species and site (high, middle, and low elevation). Each bar shows plot level mean with standard error. For tree individuals with several stems, only the tallest stem was measured for diameter and height on each individual tree (a, b). Full species names are given in Table 2. Species are grouped into combinations of early-successional (ES), late-successional (LS), tropical montane rain forest (TMF), and Lake Victoria transitional rain forest (LVTF). The significances of the site effects for each species are reported at the top of each panel. Tukey's post hoc test was used for diameter, height, and number of stems, while paired Poisson regressions were used for mortality. Different letters indicate significant differences (P < 0.05, except for mortality, where P < 0.01 was used); ns, not significant; nd, no dead individuals (and thus no variance analysis could be conducted) (d). For statistical details, see Table S4.
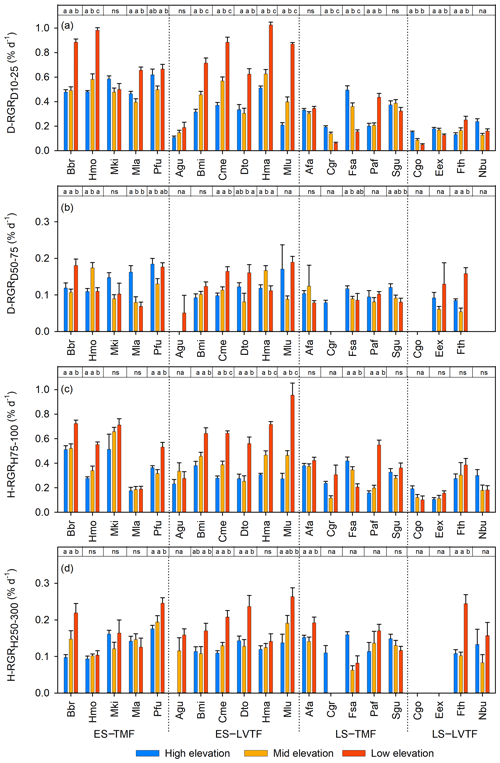
Figure 3Standardised relative growth rates (RGRs) of stem diameter at base (Dbase) at a Dbase of (a) 10–25 mm (D-RGRD10–25) and (b) 50–75 mm (D-RGRD10–25) and of height at a height of (c) 75–100 cm (H-RGRH75–100) and (d) 250–300 cm (H-RGRH250–300) of each species and site (high, middle, and low elevation). Each bar shows plot level mean with standard error. Full species names are given in Table 2. Species are grouped into combinations of early-successional (ES), late-successional (LS), tropical montane rain forest (TMF), and Lake Victoria transitional rain forest (LVTF). The results of the post hoc test (Tukey's) for site effects for each species are reported at the top of each panel. Different letters indicate significant differences (P < 0.05); ns, not significant; na, not applicable for the statistical analysis due to too few replicates within the standardised diameter or height intervals. For statistical details, see Table S4, and for standardised RGR details, see Fig. S5.
3.1 Overall effects of high temperature on growth and mortality
Both site and species identity affected tree growth, expressed as absolute and relative stem D and h increments (Table 3, Figs. 2a, b and 3). Tree mortality also differed between sites and species (Fig. 2d). However, the responses to a warmer climate (i.e. site) were species-specific, as shown by the highly significant (P < 0.001) interactions between species and sites for all analysed growth-related variables (Table 3). In the following, we address how these species differences relate to contrasting successional strategy (early- vs. late-successional) and climate of species origin (montane vs. transitional forests).
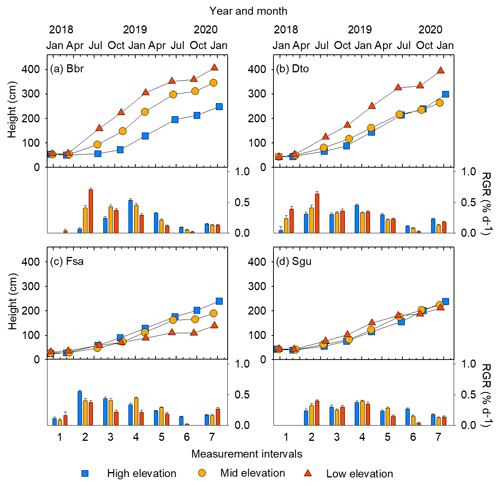
Figure 4Examples of changes in stem height and relative growth rate (RGR) over 2 years, shown for four species at three sites of different elevation: (a) Bridelia brideliifolia (Bbr), (b) Dombeya torrida (Dto), (c) Faurea saligna (Fsa), (d) Syzygium guineense (Sgu). See Figs. S6 and S7 for growth trajectories of all species.
3.2 Growth of stem base diameter and height
The most typical growth curve patterns are illustrated for stem h in Fig. 4, starting with a lag phase after planting, followed by faster growth rates that decline with increasing size. As a result of species–site interactions the following growth trajectories were identified: (i) increasing growth with warming at both ME and LE sites or only at the LE site compared to the HE site (Fig. 4a and b), (ii) decreasing growth with warming (Fig. 4c), and (iii) no effect on growth by warming (Fig. 4d). For a comprehensive view of growth trajectories of both Dbase and h of all species, see Figs. S6 and S7 in the Supplement. For early-successional species, warmer growth temperature significantly increased Dbase and h growth in 8 out of 11 () and species, respectively, but the response differed between elevation origin groups (Fig. 2a and b, Table S5 in the Supplement). A warmer climate significantly increased stem D and h in all early-successional species () species of transitional forest origin, while early-successional species of montane forest origin exhibited increased, decreased, or unchanged stem D and h with warming. In late-successional species, stem D and h increased at warmer sites only in species, while others significantly decreased or were unaffected. In general, the late-successional species grew slower compared to the early-successional species at all sites, and the responses to warming in late-successional species did not depend on elevation origin. The effects of warm climate on stem D and h were strongly linked, except for Albizia gummifera, which deviated substantially from the 1:1 line (Fig. 5). On average, Dbase and h after 2 years for early-successional species increased by 12 % and 43 % at the ME and LE sites, respectively, compared to the HE site, while corresponding values were −8 % and +11 % for late-successional species, −7 % and +10 % for TMF species, and +23 % and +59 % for transitional forest species.
3.3 Number of stems
For translation into stem volume production, the multistem behaviour is also important. The individuals of most tree species had one or two stems (Fig. 2c). Eight species (five late- and three early-successional species) have, on average, approximately one stem per tree at all sites. In species, trees at warmer sites had a significantly higher average number of stems than at the coolest site (Fig. 2c). These six species belong to all four groups of successional and elevation origin combinations, but the increase at warm sites was particularly large in the two early-successional species, Maesa lanceolata and Dombeya torrida. Noteworthy is that Dbase and h of M. lanceolate did not respond to warming; instead, warming increased wood volume through a significant increase in the number of stems, meaning that standing stock of 10 out of 11 early-successional species responded positively to warming. Only the Faurea saligna (late-successional and montane forest origin species) had a lower average number of stems at the warmest site.
3.4 Standardised RGR of stem diameter and height
Warmer growth temperature significantly stimulated the standardised RGR for smaller trees (Dbase of 10–25 mm and h of 75–100 cm) of both stem Dbase () and h () in early-successional species, respectively, which is similar to the effect on Dbase and h after 2 years (cf. Figs. 2a, b and 3a, c). As expected, the standardised RGR was generally lower for the late-successional species, and very few of these species grew faster at warmer sites. Instead, most of them grew slower or were not significantly affected. However, note that one and two out of nine late-successional species did not qualify for statistical analysis of D-RGR and H-RGR, respectively, due to low replication as a result of small sizes and high mortality (Table S5). Taken together, the similarities between warming effects on standing stock after 2 years and RGR indicate that the different initial sizes of the trees at planting (Tables S2 and S3) do not appear to have had a systematic effect on the warming responses.
For larger trees, fewer individuals reached the size ranges (Dbase of 50–75 mm and h of 250–300 cm), which resulted in fewer plot replicates and fewer species that could be included in the analysis than for the smaller trees (Fig. 3b and d, Table S5). However, the main picture is that both D-RGR and H-RGR for larger trees were lower than for smaller trees and that fewer large early-successional trees were significantly stimulated by warming. This resulted in smaller differences between early- and late-successional species for larger trees compared to smaller trees, regarding both overall RGR and the warming responses. This may partly be influenced by an effect of the seasonal drought period in 2019, when no irrigation was applied in line with the design, causing a particularly negative effect on the larger trees (usually early-successional) t the warmer sites with higher VPD and lower soil water content (Table 1), combined with possible higher hydraulic vulnerability among early-successional trees.
3.5 Tree mortality
Warmer growth temperature increased tree mortality, especially at the warmest site (Fig. 2d). Mortality > 10 % over the 2-year period was found in 7 species: late and early-successional species. High mortality was equally common in species of both montane () and transitional () forest origin. The highest mortality was observed in the three late-successional species Carapa grandiflora, Chrysophyllum gorungosanum, and Newtonia buchananii (70 %, 62 %, and 58 %, respectively, at the warmest site). Moreover, late-successional species with high mortality (> 20 %) usually () had lower Dbase and D-RGR at warmer sites (cf. Figs. 2a, d and 3a). The largest part of the mortality at the warmest site (76 %) was recorded between July 2018 and June 2019 (Table S6 in the Supplement). High mortality may partly be linked to small initial plant size, especially for the two early-successional species A. gummifera and Macaranga kilimandscharica, which were substantially smaller at planting compared to other species (Tables S2 and S3). However, within species, it was predominantly not the small trees that died (data not shown). Furthermore, one of the late-successional species with the highest warming-induced mortality (C. grandiflora) did not have smaller initial plant size than most other species in the experiment, so size is likely not the main explanation here. Another potential cause of the high mortality at the LE site is species-specific difficulties for plant establishment in high temperatures. However, it should be noted that only six trees were recorded dead after the first 3 critical months. Five of these were replaced by new individuals from the nursery and were not included in the mortality data (Table S6). The fact that species with high mortality also responded negatively to warming in terms of growth rather suggests that species heat sensitivity was the main cause of warming-induced mortality.
3.6 Tree stand composition
Tree stand composition, expressed as both BAabs and BAfrac of the four species groups (ES–TMF, ES–LVTF, LS–TMF, LS–LVTF) and resulting from changes in both Dbase growth and mortality, was significantly affected by the site (Tables 4 and 5, Fig. 6). The Dbase growth contributed most to the site mean BA, while mortality affected BA by only 3.4 % and 4.5 % at HE and ME sites, respectively, but by 17 % at the LE site (data not shown). BAabs of transitional forest species was significantly stimulated at the LE compared to the HE site, while TMF species were unaffected or showed decreased values (Fig. 6a). The BAabs values of the different species groups at the ME site were similar to either the LE (LS–TMF) or the HE sites (the other three groups). For BAfrac, values increased at warmer sites in both early- and late-successional species of transitional forest origin, while they decreased for both early- and late-successional species of montane forest origin. All sites were significantly different from each other within each species groups, except for HE and ME sites in the late-successional/transitional forest group (Fig. 6b). With these contrasting responses of different species groups, there was a marked difference in tree stand composition between the sites (Fig. 7). Compared to the coolest site, stands in a warmer climate had smaller BA fractions of montane forest species and larger fractions of transitional forest species. The differences were particularly large for the early-successional/transitional forest and the late-successional/montane forest groups, where the BA fractions were almost doubled or halved, respectively.
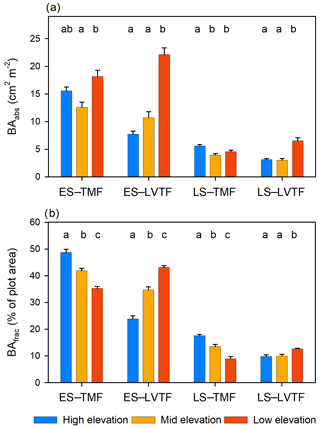
Figure 6(a) Absolute and (b) fractional basal area (BAabs and BAfrac, respectively) after 2 years per species category of early-successional (ES) and late-successional (LS) species from the tropical montane rain forest (TMF) and Lake Victoria transitional rain forest (LVTF) from three sites at different elevations. Both BAabs and BAfrac were standardised to compensate for the somewhat different initial number of individuals between the species groups (see Eq. 3). Each bar shows plot level mean (n=18) with standard error. Different letters above each species category indicate a significant difference (P < 0.05) between sites.
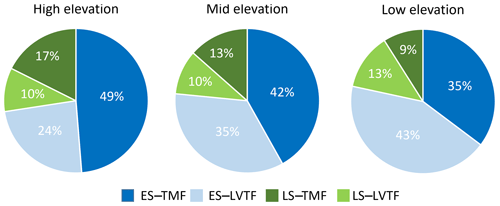
Figure 7Fractions of basal area (BAfrac) 2 years after planting for four combinations of early-successional (ES) and late-successional (LS) species from the tropical montane rain forest (TMF) and Lake Victoria transitional rain forest (LVTF) grown at three sites at different elevations. BAfrac was standardised to compensate for the somewhat different initial number of individuals between the species groups (see Eq. 3).
Table 4Two-way split plot ANOVA for site and species (Sp.) group effects on absolute (abs) and fractional (frac) basal area (BA) of four species groups (ES–TMF, ES–LVTF, LS–TMF, LS–LVTF). Greenhouse–Geisser correction was used to calculate the degrees of freedom (df's) for within subjects as the assumption of sphericity was violated. Note that between-subject analysis was not applicable for BAfrac as the sum value is 100 % for each plot.
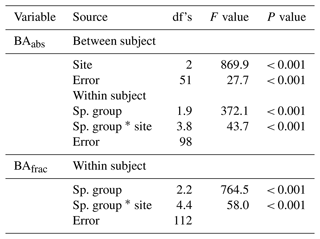
We used an experimental elevation gradient approach to explore the temperature responses of the growth and mortality of a broad range of tropical tree species with contrasting successional strategies and elevation ranges of origin in the Rwanda TREE project. Current knowledge of the temperature sensitivity of tropical tree growth is based primarily on either monitoring in intact forests (e.g. Bennett et al., 2021; Clark et al., 2003, 2010; Damptey et al., 2021; Herrera-Ramírez et al., 2021; Hubau et al., 2020; McDowell et al., 2018; Slot et al., 2021; Sullivan et al., 2020) or controlled warming experiments with small plants grown in chambers (e.g. Cheesman and Winter, 2013a; Cheesman and Winter, 2013b; Slot and Winter, 2018; Wittemann et al., 2022). The semi-controlled experimental approach of Rwanda TREE bridges the gap between these two types of studies by using large plantations with the same species and genotypes grown at three sites spanning 5.4 ∘C in MAT, with irrigation to compensate for site differences in precipitation. Moreover, by using mixed multi-species tree communities, we could assess the implications of interspecific variation in temperature responses for tree stand composition in a warmer climate. This has, to our knowledge, never been explored in an experimental and tropical setting before.
4.1 Warming effects on early- and late-successional species
Warmer climate stimulated the standing stock of stem-volume-related variables (Dbase, h, and number of stems) after 2 years in 10 out of 11 early-successional species, while responses of late-successional species were mixed, including increases, decreases, and no significant changes (Fig. 2). Similar patterns were observed for standardised RGR of both Dbase and h (Fig. 3, Table S5). These results support our first hypothesis (H1), stating that the growth of early-successional species respond more positively to warming than late-successional tree species. They are in accordance with results from a study with potted seedlings grown in the same soil at the three Rwanda TREE sites, where warming strongly stimulated biomass growth in the early-successional species Harungana montana but not in the late-successional species Syzygium guineense (Dusenge et al., 2021). This suggests that the site effects and successional group difference observed here were caused by climate responses rather than by site differences in soil conditions (Table 1). Moreover, other studies on photosynthesis of trees in Rwanda showed stronger negative effects of high temperature and warming in late-successional compared to early-successional tree species (Vårhammar et al., 2015; Tarvainen et al., 2022; Mujawamariya et al., 2023). The finding of contrasting warming responses of early- and late-successional species is also in line with results for photosynthesis and growth in warming experiments with tropical tree seedlings conducted in indoor climate-controlled chambers (Slot and Winter, 2018) as well as in field chambers (Cheesman and Winter 2013b). Finally, our results are consistent with field observations of negative effects of temperature on stem growth being stronger in late- compared to early- and mid-successional species in a TMF in Kenya (Gliniars et al., 2013). Several lines of evidence thus indicate a shift in competitive balance favouring early- over late-successional species in a warmer climate.
The loss of competitiveness of late- compared to early-successional species at warmer sites may in part be linked to the high sun exposure of plants in this study. When plants were small, especially during the first year of the experiment, they were growing under open conditions. This may have been stressful for late-successional species due to their generally lower tolerance to high light intensities, as observed in previous studies (Poorter, 1999; Veenendaal et al., 1996). However, it is likely not high solar radiation per se that is harmful for many late-successional species at the warmer sites in our experiment since peak and mean solar radiation was actually slightly lower at the LE compared to the ME site, while growth of several species was significantly lower at the LE compared to the ME site. An indirect effect of heat is more likely, such that the lower inherent stomatal conductance of late-compared to early-successional species (especially at the LE site; Mujawamariya et al., 2023) leads to higher leaf temperatures and stronger physiological heat stress under warm conditions. This has indeed been observed in previous studies in Rwanda (Vårhammar et al., 2015; Ntawuhiganayo et al., 2020; Tarvainen et al., 2022; Mujawamariya et al., 2023). The negative effect of a warmer climate observed in some late-successional species in this experiment may therefore be stronger than effects that would have occurred under understorey conditions. However, while late-successional species as young trees often grow in the understorey, they also frequently occur in forest gaps in TMFs (data from Nyirambangutse et al., 2017). The lower competitiveness of sun-exposed late-successional seedlings in a warmer climate thus implies an amplified disadvantage at early stand developmental stages compared to early-successional species. Since early stand development is a crucial phase for tree stand composition, this handicap of young late-successional seedlings is likely to endure into later stages.
4.2 Warming effects on species of different-elevation origins
The finding that tree growth of many species is stimulated by warmer climate is contrary to field observations that warm conditions decrease tropical tree growth (Clark et al., 2003, 2010 and 2013; Dong et al., 2012; Feeley et al., 2007; Way and Oren, 2010; Vlam et al., 2014; Hubau et al., 2020). However, this study is on montane and highland tree species that naturally grow in rather cool conditions, with prevailing mean annual temperatures in the middle of the species elevation ranges of approximately 15 to 21 ∘C (Fig. 1). It is therefore not surprising that tree growth in many species was stimulated at the warmer sites compared to the coolest site, provided that we irrigated during the first 1.5 years to avoid drought stress.
The stimulation of growth rate at warmer sites was especially high for the group of early-successional species with a transitional forest origin (+39 % and +140 % at the ME and LE sites, respectively, compared to the HE site; Fig. 3a). Thus, at least for early-successional species, results supported the latter part of H2, stating that warmer climate stimulates growth of species from lower elevation of origin. There was also partial support for the first part of H2, stating that warming decreased tree growth in species from higher elevation of origin (TMF) as warming had negative effects on tree growth in 5 out of 10 TMF species. Our results are in line with findings in a controlled chamber warming experiment with seedlings of four of the species used in this study, where the lowest-elevation species was better at physiologically acclimating and growing at high growth temperatures than the highest-elevation species (Wittemann et al., 2022). The differences in warming response of species with different elevation origins (transitional vs. montane forests) also corroborates the observation of thermophilisation in Andean tropical forests, where tree species with their distribution ranges centred at lower elevation had gained in relative abundance as climate got warmer over time, at the expense of higher-elevation species (Duque et al., 2015; Fadrique et al., 2018). The present study and the chamber warming study on Rwandan tree species (Wittemann et al., 2022) suggest that warming-induced declines in abundance of higher-elevation species are underpinned by lower capacity of leaf physiology and tree growth processes to handle, or benefit from, warming.
4.3 Warming effect on tree mortality
Warmer climate increased tree mortality in late-successional species, but not often or much in early-successional species (Fig. 2d). However, there was no clear difference between montane and transitional forest species (Fig. 2d), and thus H3 was only partly supported. The lack of expected difference between montane and transitional forest species conflicts with the observations that thermophilisation is driven by higher mortality of species with higher elevation ranges (Duque et al., 2015). It may be that the limited number of species in this study has masked possible influences of species origin or that these will show up at later stages in the experiment.
The findings of highest mortality in especially the slow-growing late-successional species (cf. Fig. 2a, b, and d) are in line with Laurance et al. (2004a), who observed increased mortality among slow- compared to fast-growing tree species in an analysis of growth measurements made in eighteen 1 ha plots over up to 3 decades in the Amazon rain forest. However, opposite to these results, more recent long-term studies of mortality in 24 Australian plots and 189 Amazonian plots with tropical forest concluded that fast-growing species were at the highest risk under increasing drought and high VPD, respectively (Bauman et al., 2022; Esquivel-Muelbert et al., 2020). Moreover, studies in Amazonia and Borneo rain forests observed especially high mortality among early-successional tree species during hot and dry conditions in El Niño years (Aleixo et al., 2019; Slik, 2004). Interestingly, the early-successional species with high mortality in Slik (2004) belonged to the genus Macaranga (seven species), which was the same genus as one of the two early-successional species with high mortality in our experiment (Fig. 2d).
The contrasting results of our study and some of the previous observational studies may be a consequence of early-successional species being more sensitive to drought, while late-successional species are more sensitive to high temperature and VPD, particularly at young ages. Indeed, most of the mortality in the observational studies, especially of genera affiliated with wetter climatic regimes, was attributed to drought/water stress (Esquivel-Muelbert et al., 2017). If considering only the effect of warming when there is no water limitation, as in our irrigated study, then early-successional species might be protected against heat stress by transpiratory cooling, while late-successional species (with lower transpiration) experience stronger physiological heat stress. This was indicated in a previous Rwandan common garden study with seedlings (Vårhammar et al., 2015) as well as in a recent Rwanda TREE study exploring upper heat tolerance in tropical tree species (Tarvainen et al., 2022). However, the reliance on transpiratory cooling to prevent overheating in early-successional species may not be a feasible strategy during drought, possibly contributing to the above-mentioned reports of high mortality in early-successional species.
The higher mortality in early-successional species in monitoring studies may be likely linked to higher hydraulic vulnerability compared to late-successional species (Apgaua et al., 2015; Eller et al., 2018), as well as to their demography with higher recruitment rate and shorter life span (Laurance et al., 2004b; Nyirambangutse et al., 2017). These effects may be mainly expressed by taller mature trees, which may contribute to explaining the difference between our results and long-term observational studies (Bauman et al., 2022; Esquivel-Muelbert et al., 2020). Thus, we cannot exclude that the mortality pattern among successional groups may change with increasing age and size of the trees. If late-successional trees mainly respond negatively to warming at young ages, the recruitment rate may still be inhibited, causing at least a transitional shift in species composition. Consequently, the succession from secondary to primary forest will be slower, with implications for both large-scale carbon storage (across stands at different successional stages) and how fast the final goals of forest restoration can be achieved. Recovery of species composition is already a slow successional process (longer than a century), which may thus be even slower in a warmer climate (Poorter et al., 2021).
4.4 Tree stand composition
We showed that early-successional species with transitional rain forest origin strongly increased their total and fractional basal area at warmer sites, while montane forest species declined (Fig. 7), supporting H4. These results are in line with the thermophilisation observations in Andean tropical forests along elevation gradients, where tree communities have experienced directional shifts in composition towards greater relative abundances of species with ranges centred at lower, warmer elevations during recent decades (Duque et al., 2015; Fadrique et al., 2018). This neotropical thermophilisation was caused primarily by increased mortality of more cool-adapted and heat-sensitive species (with higher elevation ranges), while the tree stand composition shift at warmer sites observed in this study was mostly explained by positive growth responses of species with lower elevation ranges. Contributions by mortality were small since it was the highest in species with small trees that do not contribute much to basal area. However, since late-successional trees grow slower but in the long term grow taller (Laurance et al., 2004b; Nyirambangutse et al., 2017), similar species-specific mortality rates in a mature forest would have had a much larger influence on BA. At later stand development, it is likely that the increased competitiveness and growth of lower-elevation-origin species in a warmer climate will lead to increased warming-induced mortality of higher-elevation species also in the Rwanda TREE plantations when competition for resources increases (Lohbeck et al., 2013). It is also possible that the observed growth reduction in higher-elevation late-successional species at warmer sites is an indication of coming mortality (Cailleret et al., 2017; Esquivel-Muelbert et al., 2020). However, there are several climate-related pathways towards mortality (Gora and Esquivel-Muelbert, 2021; Zuleta et al., 2022), and future research should try to disentangle the mortality mechanisms of different climate variables and how they interact with different groups of species.
Like in studies of leaf morphology (Manishimwe et al., 2022) and photosynthesis (Dusenge et al., 2021; Tarvainen et al., 2022; Wittemann et al., 2022; Mujawamariya et al., 2023) in the Rwanda TREE project, the tree growth response to warming was highly species-specific. This points out the importance of including several species representing different ecological niches when assessing warming responses of highly diverse tropical forests. We showed that a warmer climate increased tree growth in most early-successional species, while responses of late-successional species were more variable. Growth stimulation in early-successional species was stronger in species originating from transitional rain forest compared to higher-elevation montane forest. Several of the late-successional species had both decreased growth and increased mortality at warmer sites. At the stand level, the contribution of transitional forest species to stand basal area increased at the expense of montane forest species at lower elevation and warmer climate and thus demonstrated a thermophilisation response. We conclude that many tropical highland and montane species show potential growth stimulation in a warmer climate, while other species are likely to be threatened by projected increases in temperature. In particular, higher-elevation and late-successional species are at risk of becoming outcompeted by lower-elevation and early-successional species. This may challenge local and regional biodiversity in African high-elevation forests, where the level of endemism usually peaks above 2000 (Platts et al., 2013; Kessler and Kluge, 2008; Richter, 2008). While lower-elevation species may move uphill under warming, montane species have limited options with shrinking thermally suitable areas at increasing elevation and nowhere to go when reaching the top of the mountain. If our results for young trees influence stand community composition at later-successional stages, the increased mortality of late-successional species in combination with increased growth of early-successional species will likely result in faster carbon turnover but lower carbon storage of tropical forests (Nyirambangutse et al., 2017; Aleixo et al., 2019).
The successful establishment of mixed plantations in this experiment demonstrates that it is not necessary to wait to plant late-successional species until early-successional species have established and formed closed canopies. Simultaneous plantation of carefully selected climate- and site-adapted early- and late-successional species may thus speed up forest restoration considerably. Our results contribute to reducing the current uncertainty in native tree species restoration strategies (Aitken et al., 2008; Forrester et al., 2005; Thomas et al., 2014). They also help to improve the ability to model tree demography of Afromontane forests under climate change (Pugh et al., 2020), facilitating better predictions to support forest-based climate change mitigation and adaptation in this region.
The data that support the findings of this study are available from the corresponding author upon reasonable request.
The supplement related to this article is available online at: https://doi.org/10.5194/bg-20-5125-2023-supplement.
BN, GW, JU, and DN planned and designed the experiment; BN, EZ, NE, and AM conducted field measurements; EZ, BN, and GW compiled and analysed the data; BN, GW, and JU wrote the manuscript with contributions from the other authors. All authors read and approved the final manuscript.
The contact author has declared that none of the authors has any competing interests.
Publisher's note: Copernicus Publications remains neutral with regard to jurisdictional claims made in the text, published maps, institutional affiliations, or any other geographical representation in this paper. While Copernicus Publications makes every effort to include appropriate place names, the final responsibility lies with the authors.
This article is part of the special issue “Ecosystem experiments as a window to future carbon, water, and nutrient cycling in terrestrial ecosystems”. It is not associated with a conference.
We are grateful to the Rwanda Agriculture and Animal Resources Development Board (RAB) for providing land for the field plots and to the Rwanda Development Board (RDB) for providing access permits to seed collection in Nyungwe National Park. Furthermore, we are grateful for all the support from the driver and the field staff at the Rwanda TREE sites.
This study was funded by the Swedish Research Council (VR; grant nos. 2015-03338, 2018-04669, and 2021-05265) and the Swedish Research Council for Environment, Agricultural Sciences and Spatial Planning (Formas; grant nos. 2015-1458 and 2020-01497). JU and GW were also supported by the Swedish strategic research area “Biodiversity and Ecosystem services in a Changing Climate” (BECC; http://www.becc.lu.se/, last access: 12 December 2023).
The article processing charges for this open-access publication were covered by the Gothenburg University Library.
This paper was edited by Victor Rolo and reviewed by two anonymous referees.
Aguirre-Gutiérrez, J., Berenguer, E., Oliveras Menor, I., Bauman, D., Corral-Rivas, J. J., Nava-Miranda, M. G., Both, S., Ndong, J. E., Ondo, F. E., Bengone, N. N., Mihinhou, V., Dalling, J. W., Heineman, K., Figueiredo, A., González-M, R., Norden, N., Hurtado-M, A. B., González, D., Salgado-Negret, B., Reis, S. M., de Seixas, M. M. M., Farfan-Rios, W., Shenkin, A., Riutta, T., Girardin, C. A. J., Moore, S., Abernethy, K., Asner, G. P., Bentley, L. P., Burslem, D. F. R. P., Cernusak, L. A., Enquist, B. J., Ewers, R. M., Ferreira, J., Jeffery, K. J., Joly, C. A., Marimon, B., Martin, R. E., Morandi, P. S., Phillips, O. L., Bennett, A. C., Lewis, S. L., Quesada, C. A., Marimon, B. S., Kissling, W. D., Silman, M., Teh, Y. A., White, L. J .T., Salinas, N., Coomes, D. A., Barlow, J., Adu-Bredu, S., and Malhi, Y.: Functional susceptibility of tropical forests to climate change, Nat. Ecol. Evol., 6, 878–889, https://doi.org/10.1038/s41559-022-01747-6, 2022.
Aitken, S. N., Yeaman, S., Holliday, J. A., Wang, T., and Curtis-McLane, S.: Adaptation, migration or extirpation: climate change outcomes for tree populations, Evol. Appl., 1, 95–111, https://doi.org/10.1111/j.1752-4571.2007.00013.x, 2008.
Alamgir, M., Turton, S. M., Macgregor, C. J., and Pert, P. L.: Assessing regulating and provisioning ecosystem services in a contrasting tropical forest landscape, Ecol. Indic., 64, 319–334, https://doi.org/10.1016/j.ecolind.2016.01.016, 2016.
Aleixo, I., Norris, D., Hemerik, L., Barbosa, A., Prata, E., Costa, F., and Poorter, L.: Amazonian rainforest tree mortality driven by climate and functional traits, Nat. Clim. Change, 9, 384–388, https://doi.org/10.1038/s41558-019-0458-0, 2019.
Apgaua, D. M. G., Ishida, F. Y., Tng, D. Y. P., Laidlaw, M. J., Santos, R. M., Rumman, R., Eamus, D., Holtum, J. A. M., and Laurance, S. G. W.: Functional traits and water transport strategies in lowland tropical rainforest trees, PLOS ONE, 10, e0130799, https://doi.org/10.1371/journal.pone.0130799, 2015.
Arias, P. A., Bellouin, N., Coppola, E., Jones, R. G., Krinner, G., Marotzke, J., Naik, V., Palmer, M. D., Plattner, G.-K., Rogelj, J., Rojas, M., Sillmann, J., Storelvmo, T., Thorne, P. W., Trewin, B., Rao, K. A., Adhikary, B., Allan, R. P., Armour, K., Bala, G., Barimalala, R., Berger, S., Canadell, J. G., Cassou, C. , Cherchi, A., Collins, W., Collins, W. D., Connors, S. L., Corti, S., Cruz, F., Dentener, F. J., Dereczynski, C., Di Luca, A., Diongue Niang, A., Doblas-Reyes, F. J., Dosio, A., Douville, H., Engelbrecht, F., Eyring, V., Fischer, E., Forster, P., Fox-Kemper, B., Fuglestvedt, J. S., Fyfe, J. C., Gillett, L. Goldfarb, N. P., Gorodetskaya, I., Gutierrez, J. M., Hamdi, R., Hawkins, E., Hewitt, H. T., Hope, P. ., Islam, A. S., Jones, C., Kaufman, D. S.., Kopp, D. S., Kosaka, Y., Kossin, J., Krakovska, S., Lee, J.-Y., Li, J., Mauritsen, T., Maycock, T. K., Meinshausen, M., Min, S.-K., Monteiro, P. M. S., Ngo-Duc, T., Otto, F., Pinto, I., Pirani, A., Raghavan, K., Ranasinghe, R., Ruane, A. C., Ruiz, L., Sallée, J.-B., Samset, B. H., Sathyendranath, S., Seneviratne, S. I., Sörensson, A. A., Szopa, S., Takayabu, I., Tréguier, A.-M., van den Hurk, B., Vautard, R., von Schuckmann, K., Zaehle, S., Zhang, X., and Zickfeld, K.: Technical summary, in: Climate change 2021: The physical science basis. Contribution of working group I to the sixth assessment report of the Intergovernmental Panel on Climate Change, edited by: Masson-Delmotte, V., Zhai, P., Pirani, A., Connors, S. L., Péan, C., Berger, S., Caud, N., Chen, Y., Goldfarb, L., Gomis, M. I., Huang, M., Leitzell, K., Lonnoy, E., Matthews, J. B. R., Maycock, T. K., Waterfield, T., Yelekçi, O., Yu, R., and Zhou, B., Cambridge University Press, Cambridge, United Kingdom and New York, NY, USA, 33-144, https://doi.org/10.1017/9781009157896.002, 2021.
Báez, S., Malizia, A., Carilla, J., Blundo, C., Aguilar, M., Aguirre, N., Aquirre, Z., Álvarez, E., Cuesta, F., Duque, Á., Farfán-Ríos, W., García-Cabrera, K., Grau, R., Homeier, J., Linares-Palomino, R., Malizia, L. R., Cruz, O. M., Osinaga, O., Phillips, O. L., Reynel, C., Silman, M. R., and Feeley, K. J.: Large-scale patterns of turnover and basal area change in Andean forests, PLOS ONE, 10, e0126594, 1–14, https://doi.org/10.1371/journal.pone.0126594, 2015.
Bauman, D., Fortunel, C., Cernusak, L. A., Bentley, L. P., McMahon, S. M., Rifai, S. W., Aguirre-Gutierrez, J., Oliveras, I., Bradford, M., Laurance, S. G. W., Delhaye, G., Hutchinson, M .F., Dempsey, R., McNellis, B. E., Santos-Andrade, P. E., Ninantay-Rivera, H. R., Paucar, J. R. C., Phillips, O. L., and Malhi, Y.: Tropical tree growth sensitivity to climate is driven by species intrinsic growth rate and leaf traits, Global Change Biol., 28, 1414–1432, https://doi.org/10.1111/gcb.15982, 2021.
Bauman, D., Fortunel, C., Delhaye, G., Malhi, Y., Cernusak, L. A., Bentley, L. P., Rifai, S. W. Aguirre-Gutierrez, J., Oliveras, I., Phillips, O. L., McNellis, B. E., Bradford, M., Laurance, S. G. W. Hutchinson, M. F., Dempsey, R., Santos-Andrade, P. E., Ninantay-Rivera, H. R., Paucar, J. C. R., and McMahon, S. M.: Tropical tree mortality has increased with rising atmospheric water stress, Nature, 608, 528–533, https://doi.org/10.1038/s41586-022-04737-7, 2022.
Bennett, A. C., Dargie, G. C., Cuni-Sanchez, A., Mukendi, J. T., Hubau, W., Mukinzi, J. M., Phillips, O. L., Malhi, Y., Sullivan, M. J. P., Cooper, D. L. M., Adu-Bredu, S., Affum-Baffoe, K., Amani, C. A., Banin, L. F., Beeckman, H., Begne, S. K., Bocko, Y. E., Boeckx, P., Bogaert, J., Brncic, T., Chezeaux, E., Clark, C. J., Daniels, A. K., de Haulleville, T., Kamdem, M. N. D., Doucet, J. L., Ondo, F. E., Ewango, C. E. N., Feldpausch, T. R., Foli, E. G., Gonmadje, C., Hall, J. S., Hardy, O. J., Harris, D. J., Ifo, S. A., Jeffery, K. J., Kearsley, E., Leal, M., Levesley, A., Makana, J. R. Lukasu, F. M., Medjibe, V. P., Mihindu, V., Moore, S., Begone, N. N., Pickavance, G. C., Poulsen, J. R., Reitsma, J., Sonke, B., Sunderland, T. C. H., Taedoumg, H., Talbot, J., Tuagben, D. S., Umunay, P. M., Verbeeck, H., Vleminckx, J., White, L. J. T., Woell, H., Woods, J. T., Zemagho, L., and Lewis, S. L.: Resistance of African tropical forests to an extreme climate anomaly, P. Natl. Acad. Sci. USA, 118, 1–12, https://doi.org/10.1073/pnas.2003169118, 2021.
Bloesch, U., Troupin, G., and Derungs, N.: Les plantes ligneuses du Rwanda: flore, écologie et usage, Shaker Verlag, Aachen, Germany, 757 pp., 2009.
Boehmer, H. J.: Vulnerability of tropical montane rain forest ecosystems due to climate change, in: Coping with Global Environmental Change, Disasters and Security – Threats, Challenges, Vulnerabilities and Risks, edited by: Brauch, H. G., Oswald Spring, Ú., Mesjasz, C., Grin, J., Kameri-Mbote, P., Chourou, B., Dunay, P., and Birkmann, J., Springer-Verlag Berlin Heidelberg, 789–802, https://doi.org/10.1007/978-3-642-17776-7_46, 2011.
Brasier, C. M.: The biosecurity threat to the UK and global environment from international trade in plants, Plant Path., 57, 792–808, https://doi.org/10.1111/j.1365-3059.2008.01886.x, 2008.
Bremer, L. L. and Farley, K. A.: Does plantation forestry restore biodiversity or create green deserts? A synthesis of the effects of land-use transitions on plant species richness, Biodivers. Conserv., 19, 3893–3915, https://doi.org/10.1007/s10531-010-9936-4, 2010.
Brienen, R. J. W., Phillips, O. L., Feldpausch, T. R., Gloor, E., Baker, T. R., Lloyd, J., Lopez-Gonzalez, G., Monteagudo-Mendoza, A., Malhi, Y., Lewis, S. L., Martinez, R. V., Alexiades, M., Davila, E. A., Alvarez-Loayza, P., Andrade, A., Aragao, L., Araujo-Murakami, A., Arets, E., Arroyo, L., Aymard, G. A., Banki, O. S., Baraloto, C., Barroso, J., Bonal, D., Boot, R. G. A., Camargo, J. L. C., Castilho, C. V., Chama, V., Chao, K. J., Chave, J., Comiskey, J. A., Valverde, F. C., da Costa, L., de Oliveira, E. A., Di Fiore, A., Erwin, T. L., Fauset, S., Forsthofer, M., Galbraith, D. R., Grahame, E. S., Groot, N., Herault, B., Higuchi, N., Coronado, E. N. H., Keeling, H., Killeen, T. J., Laurance, W. F., Laurance, S., Licona, J., Magnussen, W. E., Marimon, B. S., Marimon, B. H., Mendoza, C., Neill, D. A., Nogueira, E. M., Nunez, P., Camacho, N. C. P., Parada, A., Pardo-Molina, G., Peacock, J., Pena-Claros, M., Pickavance, G. C., Pitman, N. C. A., Poorter, L., Prieto, A., Quesada, C. A., Ramirez, F., Ramirez-Angulo, H., Restrepo, Z., Roopsind, A., Rudas, A., Salomao, R. P., Schwarz, M., Silva, N., Silva-Espejo, J. E., Silveira, M., Stropp, J., Talbot, J., ter Steege, H., Teran-Aguilar, J., Terborgh, J., Thomas-Caesar, R., Toledo, M., Torello-Raventos, M., Umetsu, R. K., Van der Heijden, G. M. F., Van der Hout, P., Vieira, I. C. G., Vieira, S. A., Vilanova, E., Vos, V. A., and Zagt, R. J.: Long-term decline of the Amazon carbon sink, Nature, 519, 344–348, https://doi.org/10.1038/nature14283, 2015.
Brockerhoff, E. G., Jactel, H., Parrotta, J. A., Quine, C. P., and Sayer, J.: Plantation forests and biodiversity: Oxymoron or opportunity?, Biodivers. Conserv., 17, 925–951, https://doi.org/10.1007/s10531-008-9380-x, 2008.
Cailleret, M., Jansen, S., Robert, E. M. R., Desoto, L., Aakala, T., Antos, J. A., Beikircher, B., Bigler, C., Bugmann, H., Caccianiga, M., Cada, V., Camarero, J. J., Cherubini, P., Cochard, H., Coyea, M. R. Cufar, K., Das, A. J., Davi, H., Delzon, S., Dorman, M., Gea-Izquierdo, G., Gillner, S., Haavik, L. J., Hartmann, H., Heres, A. M., Hultine, K. R.,Janda, P. Kane, J. M. Kharuk, V. I., Kitzberger, T., Klein, T., Kramer, K., Lens, F., Levanic, T., Calderon, J. C. L., Lloret, F., Lobodo-Vale, R., Lombardi, F., Rodriguez, R. L., Makinen, H. Mayr, S., Meszaros, I., Metsaranta, J. M., Minunno, F., Oberhuber, W., Papadopoulos, A., Peltoniemi, M., Petritan, A. M.,Rohner, B., Sanguesa-Barreda, G., Sarris, D., Smith, J. M., Stan, A. B., Sterck, F., Stojanovic, D. B., Suarez, M. L., Svoboda, M., Tognetti, R., Torres-Ruiz, J. M., Trotsiuk, V., Villalba, R., Vodde, F., Westwood, A. R., Wyckoff, P. H., Zafirov, N., and Martinez-Vilalta, J.: A synthesis of radial growth patterns preceding tree mortality, Global Change Biol., 23, 1675–1690, https://doi.org/10.1111/gcb.13535, 2017.
Carter, K. R., Wood, T. E., Reed, S. C., Schwartz, E. C., Reinsel, M. B., Yang, X., and Cavaleri, M. A.: Photosynthetic and Respiratory Acclimation of Understory Shrubs in Response to in situ Experimental Warming of a Wet Tropical Forest, Front. For. Glob., 3, 576320, 1–20, https://doi.org/10.3389/ffgc.2020.576320, 2020.
Chazdon, R. L., Peres, C. A., Dent, D., Sheil, D., Lugo, A. E., Lamb, D., and Miller, S. E.: The potential for species conservation in tropical secondary forests, Conserv. Biol., 23, 1406–1417, https://doi.org/10.1111/j.1523-1739.2009.01338.x, 2009.
Cheesman, A. W. and Winter, K.: Growth response and acclimation of CO2 exchange characteristics to elevated temperatures in tropical tree seedlings, J. Exp. Bot., 64, 3817–3828, https://doi.org/10.1093/jxb/ert211, 2013a.
Cheesman, A. W. and Winter, K.: Elevated night-time temperatures increase growth in seedlings of two tropical pioneer tree species, New Phytol., 197, 1185–1192, https://doi.org/10.1111/nph.12098, 2013b.
Clark, D. A., Piper, S. C., Keeling, C. D., and Clark, D. B.: Tropical rain forest tree growth and atmospheric carbon dynamics linked to interannual temperature variation during 1984–2000, P. Natl. Acad. Sci. USA, 100, 5852–5857, https://doi.org/10.1073/pnas.0935903100, 2003.
Clark, D. A., Clark, D. B., and Oberbauer, S. F.: Field-quantified responses of tropical rainforest aboveground productivity to increasing CO2 and climatic stress, 1997–2009, J. Geophys. Res.-Biogeo., 118, 783–794, https://doi.org/10.1002/jgrg.20067, 2013.
Clark, D. B., Clark, D. A., and Oberbauer, S. F.: Annual wood production in a tropical rain forest in NE Costa Rica linked to climatic variation but not to increasing CO2, Global Change Biol., 16, 747–759, https://doi.org/10.1111/j.1365-2486.2009.02004.x, 2010.
Crous, K. Y., Uddling, J., and De Kauwe, M. G.: Temperature responses of photosynthesis and respiration in evergreen trees from boreal to tropical latitudes, New Phytol., 234, 353–374, https://doi.org/10.1111/nph.17951, 2022.
Cuesta, F., Muriel, P., Llambí, L. D., Halloy, S., Aguirre, N., Beck, S., Carilla, J., Meneses, R. I., Cuello, S., Grau, A., Gámez, L. E., Irazábal, J., Jácome, J., Jaramillo, R., Ramírez, L., Samaniego, N., Suárez-Duque, D., Thompson, N., Tupayachi, A., Vinas, P., Yager, K., Becerra, M. T., Pauli, H., and Gosling, W. D.: Latitudinal and altitudinal patterns of plant community diversity on mountain summits across the tropical Andes, Ecography, 40, 1381–1394, https://doi.org/10.1111/ecog.02567, 2017.
Cuni-Sanchez, A., Sullivan, M. J. P., Platts, P. J., Lewis, S. L., Marchant, R., Imani, G., Hubau, W., Abiem, I., Adhikari, H., Albrecht, T., Altman, J., Amani, C., Aneseyee, A. B., Avitabile, V., Banin, L., Batumike, R., Bauters, M., Beeckman, H., Begne, S. K., Bennett, A., Bitariho, R., Boeckx, P., Bogaert, J., Bräuning, A., Bulonvu, F., Burgess, N., Calders, K., Chapman, C., Chapman, H., Comiskey, J., de Haulleville, T., Decuyper, M., DeVries, B., Dolezal, J, Droissart, V., Ewango, C., Feyera, S., Gebrekristos, A., Gereau, R., Gilpin, M., Hakizimana, D., Hall, J., Hamilton, A., Hardy, O., Hart, T., Heiskanen, J., Hemp, A., Herold, M., Hiltner, U., Horak, D., Kamdem, M-N., Kayijamahe, C., Kenfack, D., Kinyanjui, M., Klein, J., Lisingo, J., Lovett, J., Lung, M., Makana, J-R., Malhi, Y., Marshall, A., Martin, E., Mitchard, E., Morel, A., Mukendi, J. T., Timberlake, J., Nchu, F., Nyirambangutse, B., Okello, J., Peh, K., Petri, P., Phillips, O., Plumptre, A., Qie, L., Rovero, F., Sainge, M., Schmitt, C, Sedlacek, O., Ngute, A., Sheil, D., Sheleme, D., Simegn, T., Simo-Droissart, M., Sonke, B., Soromessa, T., Sunderland, T., Svoboda, M., Taedoumg, H., Taplin, J., Taylor, D., Thomas, S., Tuagben, D., Umunay, P., Uzabaho, E., Verbeeck, H., Vleminckx, J., Wallin, G., Wheeler, C., Willcock, S., Woods, J., and Zibera, E.: High aboveground carbon stock of African tropical montane forests, Nature, 596, 536–542, https://doi.org/10.1038/s41586-021-03728-4, 2021.
Cunningham, S. and Read, J.: Comparison of temperate and tropical rainforest tree species: Growth responses to temperature, Oecologia, 133, 112–119, https://doi.org/10.1007/s00442-002-1034-1, 2002.
da Costa, A. C. L., Galbraith, D., Almeida, S., Portela, B. T. T., da Costa, M., de Athaydes Silva Junior, J., Braga, A. P., de Gonçalves, P. H. L., de Oliveira, A. A., Fisher, R., Phillips, O. L., Metcalfe, D. B., Levy, P., and Meir, P.: Effect of 7 yr of experimental drought on vegetation dynamics and biomass storage of an eastern Amazonian rainforest, New Phytol., 187, 579–591, https://doi.org/10.1111/j.1469-8137.2010.03309.x, 2010.
Damptey, F. G., Adofo, E., Duah-Gyamfi, A., Adusu, D., and Opuni-Frimpong, E.: Logging effects on seedling regeneration and diversity in a tropical moist semi-deciduous forest in Ghana, Geol. Ecol. Landsc., 1–12, https://doi.org/10.1080/24749508.2021.1952769, 2021.
Docherty, E. M., Gloor, E., Sponchiado, D., Gilpin, M., Pinto, C. A. D., Junior, H. M., Coughlin, I., Ferreira, L., Junior, J. A. S., da Costa, A. C. L.: Long-term drought effects on the thermal sensitivity of Amazon forest trees, Plant Cell Environ., 46, 185–198, https://doi.org/10.1111/pce.14465, 2022.
Dong, S. X., Davies, S. J., Ashton, P. S., Bunyavejchewin, S., Nur Supardi, M. N., Kassim, A. R., Tan, S., and Moorcroft, P. R.: Variability in solar radiation and temperature explains observed patterns and trends in tree growth rates across four tropical forests, P. Roy. Soc. B-Biol Sci., 279, 3923–3931, https://doi.org/10.1098/rspb.2012.1124, 2012.
Doughty, C. E. and Goulden, M. L.: Are tropical forests near a high temperature threshold?, J. Geophys. Res.-Biogeo., 114, 1–12, https://doi.org/10.1029/2007JG000632, 2008.
Duque, A., Stevenson, P. R., and Feeley, K. J.: Thermophilization of adult and juvenile tree communities in the northern tropical Andes, P. Natl. Acad. Sci. USA, 112, 10744–10749, https://doi.org/10.1073/pnas.1506570112, 2015.
Dusenge, E.M, Wittemann, M., Mujawamariya, M., Ntawuhiganayo, E. B., Zibera, E., Ntirugulirwa, E., Way, D., Nsabimana, D., Uddling, J., and Wallin, G.: Limited thermal acclimation of photosynthesis in tropical montane tree species, Global Change Biol., 27, 4860–4878, https://doi.org/10.1111/gcb.15790, 2021.
Elias, F., Ferreira, J., Lennox, G. D., Berenguer, E., Ferreira, S., Schwartz, G., and Barlow, J.: Assessing the growth and climate sensitivity of secondary forests in highly deforested Amazonian landscapes, Ecology, 101, e02954, https://doi.org/10.1002/ecy.2954, 2020.
Eller, C. B., Barros, F., de, V., Bittencourt, P. R. L., Rowland, L., Mencuccini, M., and Oliveira, R. S.: Xylem hydraulic safety and construction costs determine tropical tree growth, Plant Cell Environ., 41, 548–562, https://doi.org/10.1111/pce.13106, 2018.
Esquivel-Muelbert, A., Galbraith, D., Dexter, K. G., Baker, T. R. Lewis, S. L., Meir, P., Rowland, L., da Costa, A. C. L. Nepstad, D., and Phillips, O. L.: Biogeographic distributions of neotropical trees reflect their directly measured drought tolerances, Sci. Rep., 7, 8334, 1–11, https://doi.org/10.1038/s41598-017-08105-8, 2017.
Esquivel-Muelbert, A., Phillips, O. L., Brienen, R. J. W., Fauset, S., Sullivan, M. J. P., Baker, T. R., Chao, K. J., Feldpausch, T. R., Gloor, E., Higuchi, N., Houwing-Duistermaat, J., Lloyd, J., Liu, H. Y., Malhi, Y., Marimon, B., Marimon, B., Monteagudo-Mendoza, A., Poorter, L., Silveira, M., Torre, E. V., Davila, E. A., Pasquel, J. D., Almeida, E., Loayza, P. A., Andrade, A., Aragao, L., Araujo-Murakami, A., Arets, E., Arroyo, L., Aymard, G. A., Baisie, M., Baraloto, C., Camargo, P. B., Barroso, J., Blanc, L., Bonal, D., Bongers, F., Boot, R., Brown, F., Burban, B., Camargo, J. L., Castro, W., Moscoso, V. C., Chave, J., Comiskey, J., Valverde, F. C., da Costa, A. L., Cardozo, N. D., Di Fiore, A., Dourdain, A., Erwin, T., Llampazo, G. F., Vieira, I. C. G., Herrera, R., Honorio Coronado, E., Huamantupa-Chuquimaco, I., Jimenez-Rojas, E., Killeen, T., Laurance, S., Laurance, W., Levesley, A., Lewis, S. L., Ladvocat, K., Lopez-Gonzalez, G., Lovejoy, T., Meir, P., Mendoza, C., Morandi, P., Neill, D., Nogueira Lima, A. J., Vargas, P. N., de Oliveira, E. A., Camacho, N. P., Pardo, G., Peacock, J., Pena-Claros, M., Penuela-Mora, M. C., Pickavance, G., Pipoly, J., Pitman, N., Prieto, A., Pugh, T. A. M., Quesada, C., Ramirez-Angulo, H., de Almeida Reis, S. M., Rejou-Machain, M., Correa, Z. R., Bayona, L. R., Rudas, A., Salomao, R., Serrano, J., Espejo, J. S., Silva, N., Singh, J., Stahl, C., Stropp, J., Swamy, V., Talbot, J., ter Steege, H., Terborgh, J., Thomas, R., Toledo, M., Torres-Lezama, A., Gamarra, L. V., van der Heijden, G., van der Meer, P., van der Hout, P., Martinez, R. V., Vieira, S. A., Cayo, J. V., Vos, V., Zagt, R., Zuidema, P., and Galbraith, D.: Tree mode of death and mortality risk factors across Amazon forests, Nat. Commun., 11, 5515, https://doi.org/10.1038/s41467-020-18996-3, 2020.
Estiarte, M., Vicca, S., Peñuelas, J., Bahn, M., Beier, C., Emmett, B. A., Fay, P. A., Hanson, P. J., Hasibeder, R., Kigel, J., Kröel-Dulay, G., Larsen, K. S., Lellei-Kovács, E., Limousin, J. M., Ogaya, R., Ourcival, J. M., Reinsch, S., Sala, O. E., Schmidt, I. K., Sternberg, M., Tielborger, K., Tietema, A., and Janssens, I. A.: Few multiyear precipitation-reduction experiments find a shift in the productivity-precipitation relationship, Global Change Biol., 22, 2570–2581, https://doi.org/10.1111/gcb.13269, 2016.
Fadrique, B., Báez, S., Duque, Á., Malizia, A., Blundo, C., Carilla, J., Osinaga-Acosta, O., Malizia, L., Silman, M., Farfán-Ríos, W., Malhi, Y., Young, K. R., Cuesta C, F., Homeier, J., Peralvo, M., Pinto, E., Jadan, O., Aguirre, N., Aguirre, Z., and Feeley, K. J.: Widespread but heterogeneous responses of Andean forests to climate change, Nature, 564, 207–212, https://doi.org/10.1038/s41586-018-0715-9, 2018.
Feeley, K. J., Joseph Wright, S., Nur Supardi, M. N., Kassim, A. R., and Davies, S. J.: Decelerating growth in tropical forest trees, Ecol. Lett., 10, 461–469, https://doi.org/10.1111/j.1461-0248.2007.01033.x, 2007.
Feeley, K., Martinez-Villa, J., Perez, T., Duque, A. S., Gonzalez, D. T., and Duque, A.: The thermal tolerances, distributions, and performances of tropical montane tree species, Front. For. Glob. Change, 3, 1–11, https://doi.org/10.3389/ffgc.2020.00025, 2020.
Feng, X., Uriarte, M., González, G., Reed, S., Thompson, J., Zimmerman, J. K., and Murphy, L.: Improving predictions of tropical forest response to climate change through integration of field studies and ecosystem modelling, Global Change Biol., 24, e213–e232, https://doi.org/10.1111/gcb.13863, 2017.
Fischer, E. and Killmann, D.: Illustrated field guide to the Plants of Nyungwe national park Rwanda, Koblenze Geographical Colloquia, Series Biogeographical Monographs, Mainz, Universität Koblenz Landau, Germany, 771 pp, 2008.
Forrester, D. I., Bauhus, J., and Cowie, A. L.: On the success and failure of mixed-species tree plantations: Lessons learned from a model system of Eucalyptus globulus and Acacia mearnsii, Forest Ecol. Manag., 209, 147–155, https://doi.org/10.1016/j.foreco.2005.01.012, 2005.
Galhena, D., Freed, R., and Maredia, K. M.: Home gardens: a promising approach to enhance household food security and wellbeing, Agric. Food Secur., 2, 1–13, https://doi.org/10.1186/2048-7010-2-8, 2013.
Ghalambor, C. K., Huey, R. B., Martin, P. R., Tewksbury, J. J., and Wang, G.: Are mountain passes higher in the tropics? Janzen's hypothesis revisited, Integr. Comp. Biol., 46, 5–17, https://doi.org/10.1093/icb/icj003, 2006.
Gliniars, R., Becker, G. S., Braun, D., and Dalitz, H.: Monthly stem increment in relation to climatic variables during 7 years in an East African rainforest, Trees-Struc. Funct., 27, 1129–1138, https://doi.org/10.1007/s00468-013-0863-3, 2013.
Gora, E. M. and Esquivel-Muelbert, A.: Implications of size-dependent tree mortality for tropical forest carbon dynamics, Nat. Plants, 7, 384–391, https://doi.org/10.1038/s41477-021-00879-0, 2021.
Herrera-Ramírez, D., Sierra, C. A., Römermann, C., Muhr, J., Trumbore, S., Silvério, D., Brando, P. M., and Hartmann, H.: Starch and lipid storage strategies in tropical trees relate to growth and mortality, New Phytol., 230, 139–154, https://doi.org/10.1111/nph.17239, 2021.
Hubau, W., Lewis, S. L., Phillips, O. L., Affum-Baffoe, K., Beeckman, H., Cuni-Sanchez, A., Daniels, A. K., Ewango, C. E. N., Fauset, S., Mukinzi, J. M., Sheil, D., Sonke, B., Sullivan, M. J. P., Sunderland, T. C. H., Taedoumg, H., Thomas, S. C., White, L. J. T., Abernethy, K. A., Adu-Bredu, S., Amani, C. A., Baker, T. R., Banin, L. F., Baya, F., Begne, S. K., Bennett, A. C., Benedet, F., Bitariho, R., Bocko, Y. E., Boeckx, P., Boundja, P., Brienen, R. J. W., Brncic, T., Chezeaux, E., Chuyong, G. B., Clark, C. J., Collins, M., Comiskey, J. A., Coomes, D. A., Dargie, G. C., de Haulleville, T., Kamdem, M. N. D., Doucet, J. L., Esquivel-Muelbert, A., Feldpausch, T. R., Fofanah, A., Foli, E. G., Gilpin, M., Gloor, E., Gonmadje, C., Gourlet-Fleury, S., Hall, J. S., Hamilton, A. C., Harris, D. J., Hart, T. B., Hockemba, M. B. N., Hladik, A., Ifo, S. A., Jeffery, K. J., Jucker, T., Yakusu, E. K., Kearsley, E., Kenfack, D., Koch, A., Leal, M. E., Levesley, A., Lindsell, J. A., Lisingo, J., Lopez-Gonzalez, G., Lovett, J. C., Makana, J. R., Malhi, Y., Marshall, A. R., Martin, J., Martin, E. H., Mbayu, F. M., Medjibe, V. P., Mihindou, V., Mitchard, E. T. A., Moore, S., Munishi, P. K. T., Bengone, N. N., Ojo, L., Ondo, F. E., Peh, K. S. H., Pickavance, G. C., Poulsen, A. D., Poulsen, J. R., Qie, L., Reitsma, J., Rovero, F., Swaine, M. D., Talbot, J., Taplin, J., Taylor, D. M., Thomas, D. W., Toirambe, B., Mukendi, J. T., Tuagben, D., Umunay, P. M., van der Heijden, G. M. F., Verbeeck, H., Vleminckx, J., Willcock, S., Woll, H., Woods, J. T., and Zemagho, L.: Asynchronous carbon sink saturation in African and Amazonian tropical forests, Nature, 579, 80–87, https://doi.org/10.1038/s41586-020-2035-0, 2020.
Humphrey, V., Berg, A., Ciais, P., Gentine, P., Jung, M., Reichstein, M., Seneviratne, S. I., and Frankenberg, C.: Soil moisture-atmosphere feedback dominates land carbon uptake variability, Nature, 592, 65–69, https://doi.org/10.1038/s41586-021-03325-5, 2021.
Janzen, D. H.: Why Mountain Passes are Higher in the Tropics, Am. Nat., 101, 233–249, 1967.
Jung, M., Reichstein, M., Schwalm, C. R., Huntingford, C., Sitch, S., Ahlström, A., Arneth, A., Camps-Valls, G., Ciais, P., Friedlingstein, P., Gans, F., Ichii, K., Jain, A. K., Kato, E., Papale, D., Poulter, B., Raduly, B., Rödenbeck, C., Tramontana, G., Viovy, N., Wang, Y. P., Weber, U., Zaehle, S., and Zeng, N.: Compensatory water effects link yearly global land CO2 sink changes to temperature, Nature, 541, 516–520, https://doi.org/10.1038/nature20780, 2017.
Kessler, M. and Kluge, J.: Diversity and endemism in tropical montane forests – from patterns to processes, in: The Tropical Mountain Forest – Patterns and Processes in a Biodiversity Hotspot, Biodiversity and Ecology Series 2, edited by: Gradstein, S. R., Homeier, J., and Gansert, D., Universitätsverlag Göttingen, Göttingen, 35–50, 2008.
Killi, D., Bussotti, F., Raschi, A., and Haworth, M.: Adaptation to high temperature mitigates the impact of water deficit during combined heat and drought stress in C3 sunflower and C4 maize varieties with contrasting drought tolerance, Physiol. Plantarum, 159, 130–147, https://doi.org/10.1111/ppl.12490, 2017.
Kindt, R., van Breugel, P., Lillesø, J.-P. B., Minani, V., Ruffo, C. K., Gapusi, J., Jamnadass, R., and Graudal, L.: Potential natural vegetation of eastern Africa, Volume 9, Atlas and tree species composition for Rwanda, Department of Geosciences and Natural Resource Management, University of Copenhagen, Copenhagen, Denmark, 93 pp, 2014.
Körner, C.: The use of “altitude” in ecological research, Trends Ecol. Evol., 22, 569–574, https://doi.org/10.1016/j.tree.2007.09.006, 2007.
Laurance, W. F., Oliveira, A. A., Laurance, S. G., Condit, R., Nascimento, H. E. M., Sanchez-Thorin, A. C., Lovejoy, T. E., Andrade, A., D'Angelo, S., Riberio, J. E., and Dick, C. W.: Pervasive alteration of tree communities in undisturbed Amazonian forests, Nature, 428, 171–175, https://doi.org/10.1111/j.1744-7429.2005.00021.x, 2004a.
Laurance, W. F., Nascimento, H. E. M., Laurance, S. G., Condit, R., D'Angelo, S., and Andrade, A.: Inferred longevity of Amazonian rainforest trees based on a long-term demographic study, Forest Ecol. Manag., 190, 131–143, https://doi.org/10.1016/j.foreco.2003.09.011, 2004b.
Lewis, S. L., Brando, P. M., Phillips, O. L., Van Der Heijden, G. M. F., and Nepstad, D.: The 2010 Amazon drought, Science, 331, 554, https://doi.org/10.1126/science.1200807, 2011.
Lin, D., Xia, J., and Wan, S.: Climate warming and biomass accumulation of terrestrial plants: A meta-analysis, New Phytol., 188, 187–198, https://doi.org/10.1111/j.1469-8137.2010.03347.x, 2010.
Lohbeck, M., Poorter, L., Martinez-Ramos, M., Rodriguez-Velazquez, J., van Breugel, M., and Bongers, F.: Changing drivers of species dominance during tropical forest succession, Funct. Ecol., 28, 1052–1058, https://doi.org/10.1111/1365-2435.12240, 2013.
Malhi, Y., Silman, M., Salinas, N., Bush, M., Meir, P., and Saatchi, S.: Introduction: Elevation gradients in the tropics: Laboratories for ecosystem ecology and global change research, Global Change Biol., 16, 3171–3175, https://doi.org/10.1111/j.1365-2486.2010.02323.x, 2010.
Malizia, A., Blundo, C., Carilla, J., Acosta, O. O., Cuesta, F., Duque, A., Aguirre, N., Aguirre, Z., Ataroff, M., Baez, S., Calderon-Loor, M., Cayola, L., Cayuela, L., Ceballos, S., Cedillo, H., Rios, W. F., Feeley, K. J., Fuentes, A. F., Alvarez, L. E. G., Grau, R., Homeier, J., Jadan, O., Llambi, L. D., Rivera, M. I. L., Macia, M. J., Malhi, Y., Malizia, L., Peralvo, M., Pinto, E., Tello, S., Silman, M., and Young, K. R.: Elevation and latitude drives structure and tree species composition in Andean forests: Results from a large-scale plot network, PLOS ONE, 15, e0231553, 1–18, https://doi.org/10.1371/journal.pone.0231553, 2020.
Manishimwe, A., Ntirugulirwa, B., Zibera, E., Nyirambangutse, B., Mujawamariya, M., Dusenge M. E., Bizuru, E., Nsabimana, D., Uddling, J., and Wallin, G.: Warming responses of leaf morphology are highly variable among tropical tree species, Forests, 13, 219, 1–24, https://doi.org/10.3390/f13020219, 2022.
Martínez, M. L., Pérez-Maqueo, O., Vázquez, G., Castillo-Campos, G., García-Franco, J., Mehltreter, K., Equihua, M., and Landgrave, R.: Effects of land use change on biodiversity and ecosystem services in tropical montane cloud forests of Mexico, Forest Ecol. Manag., 258, 1856–1863, https://doi.org/10.1016/j.foreco.2009.02.023, 2009.
McDowell, N., Allen, C. D., Anderson-Teixeira, K., Brando, P., Brienen, R., Chambers, J., Christoffersen, B., Davies, S., Doughty, C., Duque, A., Espirito-Santo, F., Fisher, R., Fontes, C. G., Galbraith, D., Goodsman, D., Grossiord, C., Hartmann, H., Holm, J., Johnson, D. J., Kassim, A., Keller, M., Koven, C., Kueppers, L., Kumagai, T., Malhi, Y., McMahon, S. M., Mencuccini, M., Meir, P., Moorcroft, P., Muller-Landau, H. C., Phillips, O. L., Powell, T., Sierra, C. A., Sperry, J., Warren, J., Xu, C. G., and Xu, X. T.: Drivers and mechanisms of tree mortality in moist tropical forests, New Phytol., 219, 851–869, , https://doi.org/10.1111/nph.15027, 2018.
Meir, P., P. M. Brando, D. N., Vasconcelos, S., Costa, A. C. L., Davidson, E., Almeida, S., Fisher, R. A., Sotta, E. D., Zarin, D., and Cardinot, G.: The Effects of Drought on Amazonian Rain Forests, in: Amazonia and Global Change, edited by: Keller, M., Bustamante, M., Gash, J., and Dias, S., Geoph. Monog. Series, 186, 429–449, 2014.
Mujawamariya, M., Witteman, M., Dusenge, E. M., Manishimwe, A., Ntirugulirwa, B., Zibera, E., Nsabimana, D., Wallin, G., and Uddling, J.: Contrasting warming responses of photosynthesis in early-and late-successional tropical trees, Tree Physiol. 43, 1104–1117, https://doi.org/10.1093/treephys/tpad035, 2023.Nepstad, D. C., Tohver, I. M., David, R., Moutinho, P., and Cardinot, G.: Mortality of large trees and lianas following experimental drought in an amazon forest, Ecology, 88, 2259–2269, https://doi.org/10.1890/06-1046.1, 2007.
Mujawamariya, M., Manishimwe, A., Ntirugulirwa, B., Zibera, E., Ganszky D., Bahati, E. N., Nyirambangutse, B., Nsabimana, D., Wallin, G., and Uddling, J.: Climate Sensitivity of Tropical Trees Along an Elevation Gradient in Rwanda, Forests, 9, 647, https://doi.org/10.3390/f9100647, 2018.
Ntawuhiganayo, E. B., Uwizeye, F. K., Zibera, E., Dusenge, M. E., Ziegler, C., Ntirugulirwa B., Nsabimana, D., Wallin, G., and Uddling, J.: Traits controlling shade tolerance in tropical montane trees, Tree Physiol., 40, 183–197, https://doi.org/10.1093/treephys/tpz119, 2020.
Nyirambangutse, B., Zibera, E., Uwizeye, F. K., Nsabimana, D., Bizuru, E., Pleijel, H., Uddling, J., and Wallin, G.: Carbon stocks and dynamics at different successional stages in an Afromontane tropical forest, Biogeosciences, 14, 1285–1303, https://doi.org/10.5194/bg-14-1285-2017, 2017.
Okello, J., Bauters, M., Verbeeck, H., Kasenene, J., and Boeckx, P.: Aboveground carbon stocks, woody and litter productivity along an elevational gradient in the Rwenzori Mountains, Uganda, Biotropica, 54, 906–920, https://doi.org/10.1111/btp.13114, 2022.
Piao, S. L., Wang, X. H., Wang, K., Li, X. Y., Bastos, A., Canadell, J. G., Ciais, P., Friedlingstein, P., Sitch, S.: Interannual variation of terrestrial carbon cycle: Issues and perspectives, Global Change Biol., 26, 300–318, https://doi.org/10.1111/gcb.14884, 2020.
Platts, P. J., Gereau, R. E., Burgess, N. D., and Marchant, R.: Spatial heterogeneity of climate change in an Afromontane centre of endemism, Ecography, 36, 518–530, https://doi.org/10.1111/j.1600-0587.2012.07805.x, 2013.
Poorter, L.: Growth responses of 15 rain-forest tree species to a light gradient: the relative importance of morphological and physiological traits Functional, Ecology, 13, 396–410, https://doi.org/10.1046/j.1365-2435.1999.00332.x, 1999.
Poorter, L., Craven, D., Jakovac, C. C., van der Sande, M. T., Amissah, L., Bongers, F., Chazdon, R. L., Farrior, C. E., Kambach, S., Meave, J. A., Munoz, R., Norden, N., Ruger, N., van Breugel, M., Zambrano, A. M. A., Amani, B., Andrade, J. L., Brancalion, P. H. S., Broadbent, E. N., de Foresta, H., Dent, D. H., Derroire, G., DeWalt, S. J., Dupuy, J. M., Duran, S. M., Fantini, A. C., Finegan, B., Hernandez-Jaramillo, A., Hernandez-Stefanoni, J. L., Hietz, P., Junqueira, A. B., N'Dja, J. K., Letcher, S. G., Lohbeck, M., Lopez-Camacho, R., Martinez-Ramos, M., Melo, F. P. L., Mora, F., Muller, S. C., N'Guessan, A. E., Oberleitner, F., Ortiz-Malavassi, E., Perez-Garcia, E. A., Pinho, B. X., Piotto, D., Powers, J. S., Rodriguez-Buritica, S., Rozendaal, D. M. A., Ruiz, J., Tabarelli, M., Teixeira, H. M., Sampaio, E., van der Wal, H., Villa, P. M., Fernandes, G. W., Santos, B. A., Aguilar-Cano, J., de Almeida-Cortez, J. S., Alvarez-Davila, E., Arreola-Villa, F., Balvanera, P., Becknell, J. M., Cabral, G. A. L., Castellanos-Castro, C., de Jong, B. H. J., Nieto, J. E., Espirito-Santo, M. M., Fandino, M. C., Garcia, H., Garcia-Villalobos, D., Hall, J. S., Idarraga, A., Jimenez-Montoya, J., Kennard, D., Marin-Spiotta, E., Mesquita, R., Nunes, Y. R. F., Ochoa-Gaona, S., Pena-Claros, M., Perez-Cardenas, N., Rodriguez-Velazquez, J., Villanueva, L. S., Schwartz, N. B., Steininger, M. K., Veloso, M. D. M., Vester, H. F. M., Vieira, I. C. G., Williamson, G. B., Zanini, K., and Herault, B.: Multidimensional tropical forest recovery, Science, 374, 1370–1376, https://doi.org/10.1126/science.abh3629, 2021.
Pugh, T. A. M., Rademacher, T., Shafer, S. L., Steinkamp, J., Barichivich, J., Beckage, B., Haverd, V., Harper, A., Heinke, J., Nishina, K., Rammig, A., Sato, H., Arneth, A., Hantson, S., Hickler, T., Kautz, M., Quesada, B., Smith, B., and Thonicke, K.: Understanding the uncertainty in global forest carbon turnover, Biogeosciences, 17, 3961–3989, https://doi.org/10.5194/bg-17-3961-2020, 2020.
Richter, M.: Tropical mountain forests – distribution and general features, in: The Tropical Mountain Forest – Patterns and Processes in a Biodiversity Hotspot, Biodiversity and Ecology Series 2, edited by: Gradstein, S. R., Homeier, J., and Gansert, D., Universitätsverlag Göttingen, Göttingen, 7–24, 2008.
Rifai, S. W., Girardin, C. A. J., Berenguer, E., del Aguila-Pasquel, J., Dahlsjo, C. A. L., Doughty, C. E., Jeffery, K. J., Moore, S., Oliveras, I., Riutta, T., Rowland, L. M., Murakami, A. A., Addo-Danso, S. D., Brando, P., Burton, C., Ondo, F. E., Duah-Gyamfi, A., Amezquita, F. F., Freitag, R., Pacha, F. H., Huasco, W. H., Ibrahim, F., Mbou, A. T., Mihindou, V. M., Peixoto, K. S., Rocha, W., Rossi, L. C., Seixas, M., Silva-Espejo, J. E., Abernethy, K. A., Adu-Bredu, S., Barlow, J., da Costa, A. C. L., Marimon, B. S., Marimon, B., Meir, P., Metcalfe, D. B., Phillips, O. L., White, L. J. T., and Malhi, Y.: ENSO Drives interannual variation of forest woody growth across the tropics, Philos. T. R. Soc. B., 373, 20170410, https://doi.org/10.1098/rstb.2017.0410, 2018.
Rowland, L., Oliveira, R. S., Bittencourt, P. R. L., Giles, A. L., Coughlin, I., Costa, P. D., Domingues, T., Ferreira, L. V., Vasconcelos, S. S., Junior, J. A. S., Oliveira, A. A. R., da Costa, A. C. L., Meir, P., and Mencuccini, M.: Plant traits controlling growth change in response to a drier climate, New Phytol., 229, 1363–1374, https://doi.org/10.1111/nph.16972, 2021.
Salinas, N., Cosio, E. G., Silman, M., Meir, P., Nottingham, A. T., Roman-Cuesta, R. M., and Malhi, Y.: Editorial: Tropical Montane Forests in a Changing Environment, Front. Plant Sci., 12, 712748, https://doi.org/10.3389/fpls.2021.712748, 2021.
Sands, R.: Forestry in a global context, 2nd edn., New Zealand School of Forestry, University of Canterbury, New Zealand, 2013.
Sena, K., Barton, C., Angel, P., Agouridis, C., and Warner, R.: Influence of spoil type on chemistry and hydrology of interflow on a surface coal mine in the eastern us coalfield, Water Air Soil Poll., 225, 2171, https://doi.org/10.1007/s11270-014-2171-y, 2014.
Slik, J. W. F.: El Niño droughts and their effects on tree species composition and diversity in tropical rain forests, Oecologia, 141, 114–120, https://doi.org/10.1007/s00442-004-1635-y, 2004.
Slot, M. and Winter, K.: High tolerance of tropical sapling growth and gas exchange to moderate warming, Funct. Ecol., 32, 599–611, https://doi.org/10.1111/1365-2435.13001, 2018.
Slot, M., Cala, D., Aranda, J., Virgo, A., Michaletz, S. T., and Winter, K.: Leaf heat tolerance of 147 tropical forest species varies with elevation and leaf functional traits, but not with phylogeny, Plant Cell Environ., 44, 2414–2427, https://doi.org/10.1111/pce.14060, 2021.
Soh, M. C. K., Mitchell, N. J., Ridley, A. R., Butler, C. W., Puan, C. L., and Peh, K. S. H.: Impacts of Habitat Degradation on Tropical Montane Biodiversity and Ecosystem Services: A Systematic Map for Identifying Future Research Priorities, Front. For. Glob., 2, 1–18, https://doi.org/10.3389/ffgc.2019.00083, 2019.
Spracklen, D. V. and Righelato, R.: Tropical montane forests are a larger than expected global carbon store, Biogeosciences, 11, 2741–2754, https://doi.org/10.5194/bg-11-2741-2014, 2014.
Stephens, S. S. and Wagner, M. R.: Forest plantations and biodiversity: A fresh perspective, J. Forest., 105, 307–313, 2007.
Sullivan, M. J. P., Lewis, S. L., Affum-Baffoe, K., Castilho, C., Costa, F., Sanchez, A. C., Ewango, C. E. N., Hubau, W., Marimon, B., Monteagudo-Mendoza, A., Qie, L., Sonke, B., Martinez, R. V., Baker, T. R., Brienen, R. J. W., Feldpausch, T. R., Galbraith, D., Gloor, M., Malhi, Y., Aiba, S. I., Alexiades, M. N., Almeida, E. C., de Oliveira, E. A., Davila, E. A., Loayza, P. A., Andrade, A., Vieira, S. A., Aragao, L., Araujo-Murakami, A., Arets, E., Arroyo, L., Ashton, P., Aymard, C. G., Baccaro, F. B., Banin, L. F., Baraloto, C., Camargo, P. B., Barlow, J., Barroso, J., Bastin, J. F., Batterman, S. A., Beeckman, H., Begne, S. K., Bennett, A. C., Berenguer, E., Berry, N., Blanc, L., Boeckx, P., Bogaert, J., Bonal, D., Bongers, F., Bradford, M., Brearley, F. Q., Brncic, T., Brown, F., Burban, B., Camargo, J. L., Castro, W., Ceron, C., Ribeiro, S. C., Moscoso, V. C., Chave, J., Chezeaux, E., Clark, C. J., de Souza, F. C., Collins, M., Comiskey, J. A., Valverde, F. C., Medina, M. C., da Costa, L., Dancak, M., Dargie, G. C., Davies, S., Cardozo, N. D., de Haulleville, T., de Medeiros, M. B., Pasquel, J. D., Derroire, G., Di Fiore, A., Doucet, J. L., Dourdain, A., Droissart, V., Duque, L. F., Ekoungoulou, R., Elias, F., Erwin, T., Esquivel-Muelbert, A., Fauset, S., Ferreira, J., Llampazo, G. F., Foli, E., Ford, A., Gilpin, M., Hall, J. S., Hamer, K. C., Hamilton, A. C., Harris, D. J., Hart, T. B., Hedl, R., Herault, B., Herrera, R., Higuchi, N., Hladik, A., Coronado, E. H., Huamantupa-Chuquimaco, I., Huasco, W. H., Jeffery, K. J., Jimenez-Rojas, E., Kalamandeen, M., Djuikouo, M. N. K., Kearsley, E., Umetsu, R. K., Kho, L. K., Killeen, T., Kitayama, K., Klitgaard, B., Koch, A., Labriere, N., Laurance, W., Laurance, S., Leal, M. E., Levesley, A., Lima, A. J. N., Lisingo, J., Lopes, A. P., Lopez-Gonzalez, G., Lovejoy, T., Lovett, J. C., Lowe, R., Magnusson, W. E., Malumbres-Olarte, J., Manzatto, A. G., Marimon, B. H., Marshall, A. R., Marthews, T., Reis, S. M. D., Maycock, C., Melgaco, K., Mendoza, C., Metali, F., Mihindou, V., Milliken, W., Mitchard, E. T. A., Morandi, P. S., Mossman, H. L., Nagy, L., Nascimento, H., Neill, D., Nilus, R., Vargas, P. N., Palacios, W., Camacho, N. P., Peacock, J., Pendry, C., Mora, M. C. P., Pickavance, G. C., Pipoly, J., Pitman, N., Playfair, M., Poorter, L., Poulsen, J. R., Poulsen, A. D., Preziosi, R., Prieto, A., Primack, R. B., Ramirez-Angulo, H., Reitsma, J., Reejou-Meechain, M., Correa, Z. R., de Sousa, T. R., Bayona, L. R., Roopsind, A., Rudas, A., Rutishauser, E., Abu Salim, K., Salomao, R. P., Schietti, J., Sheil, D., Silva, R. C., Espejo, J. S., Valeria, C. S., Silveira, M., Simo-Droissart, M., Simon, M. F., Singh, J., Shareva, Y. C. S., Stahl, C., Stropp, J., Sukri, R., Sunderland, T., Svatek, M., Swaine, M. D., Swamy, V., Taedoumg, H., Talbot, J., Taplin, J., Taylor, D., ter Steege, H., Terborgh, J., Thomas, R., Thomas, S. C., Torres-Lezama, A., Umunay, P., Gamarra, L. V., van der Heijden, G., van der Hout, P., van der Meer, P., van Nieuwstadt, M., Verbeeck, H., Vernimmen, R., Vicentini, A., Vieira, I. C. G., Torre, E. V., Vleminckx, J., Vos, V., Wang, O., White, L. J. T., Willcock, S., Woods, J. T., Wortel, V., Young, K., Zagt, R., Zemagho, L., Zuidema, P. A., Zwerts, J. A., and Phillips, O. L.: Long-term thermal sensitivity of Earth's tropical forests, Science, 368, 869–874, https://doi.org/10.1126/science.aaw7578, 2020.
Sundqvist, M. K., Sanders, N. J., and Wardle, D. A.: Community and ecosystem responses to elevational gradients: Processes, mechanisms, and insights for global change, Annu. Rev. Ecol. Evol. S., 44, 261–280, https://doi.org/10.1146/annurev-ecolsys-110512-135750, 2013.
Tarvainen, L., Wittemann, M., Mujawamariya, M., Manishimwe, A., Zibera, E., Ntirugulirwa, B., Ract, C., Manzi, O. J. L., Andersson, M. X., Spetea, C., Nsabimana, D., Wallin, G., and Uddling, J.: Handling the heat – photosynthetic thermal stress in tropical trees, New Phytol., 233, 236–250, https://doi.org/10.1111/nph.17809, 2022.
Thomas, E., Jalonen, R., Loo, J., Boshier, D., Gallo, L., Cavers, S., Bordács, S., Smith, P., and Bozzano, M.: Genetic considerations in ecosystem restoration using native tree species, Forest Ecol. Manag., 333, 66–75, https://doi.org/10.1016/j.foreco.2014.07.015, 2014.
Tito, R., Vasconcelos, H. L., and Feeley, K. J.: Mountain Ecosystems as Natural Laboratories for Climate Change Experiments, Front. For. Glob., 3, 38, 1–8, https://doi.org/10.3389/ffgc.2020.00038, 2020.
Tovar, C., Carril, A. F., Gutierrez, A. G., Ahrends, A., Fita, L., Zaninelli, P., Flombaum, P., Abarzua, A. M., Alarcon, D., Aschero, V., Baez, S., Barros, A., Carilla, J., Ferrero, M. E., Flantua, S. G. A., Gonzales, P., Menendez, C. G., Perez-Escobar, O. A., Pauchard, A., Ruscica, R. C., Sarkinen, T., Sorensson, A. A., Srur, A., Villalba, R., and Hollingsworth, P. M.: Understanding climate change impacts on biome and plant distributions in the Andes: Challenges and opportunities, J. Biogeogr., 49, 1420–1442, https://doi.org/10.1111/jbi.14389, 2022.
Vårhammar, A., Wallin, G., Mclean, C. M., Dusenge, M. E., Medlyn, B. E., Hasper, T. B., Nsabimana, D., and Uddling, J.: Photosynthetic temperature responses of tree species in Rwanda: Evidence of pronounced negative effects of high temperature in montane rainforest climax species, New Phytol., 206, 1000–1012, https://doi.org/10.1111/nph.13291, 2015.
Veenendaal, E. M., Swaine, M. D., Lecha, R. T., Walsh, M. F., Abebrese, I. K., and Owusu-Afriyie, K.: Responses of West African forest tree seedlings to irradiance and soil fertility, Funct. Ecol., 10, 501–511, https://doi.org/10.2307/2389943, 1996.
Vlam, M., Baker, P. J., Bunyavejchewin, S., and Zuidema, P. A.: Temperature and rainfall strongly drive temporal growth variation in Asian tropical forest trees, Oecologia, 174, 1449–1461, https://doi.org/10.1007/s00442-013-2846-x, 2014.
Wang, X., Piao, S., Ciais, P., Friedlingstein, P., Myneni, R. B., Cox, P., Heimann, M., Miller, J., Peng, S., Wang, T., Yang, H., and Chen, A.: A two-fold increase of carbon cycle sensitivity to tropical temperature variations, Nature, 506, 212–215, https://doi.org/10.1038/nature12915, 2014.
Way, D. A. and Oren, R.: Differential responses to changes in growth temperature between trees from different functional groups and biomes: a review and synthesis of data, Tree Physiol., 30, 669–688, https://doi.org/10.1093/treephys/tpq015, 2010.
Wittemann, M., Andersson, M. X., Ntirugulirwa, B., Tarvainen, L., Wallin, G., and Uddling, J.: Temperature acclimation of net photosynthesis and its underlying component processes in four tropical tree species, Tree Physiol., 42, 1188–1202, https://doi.org/10.1093/treephys/tpac002, 2022.
Woodcock, P., Cottrell, J. E., Buggs, R. J. A., and Quine, C. P.: Mitigating pest and pathogen impacts using resistant trees: A framework and overview to inform development and deployment in Europe and North America, Forestry, 91, 1–16, https://doi.org/10.1093/forestry/cpx031, 2017.
Zhao, J., Hartmann, H., Trumbore, S., Ziegler, W., and Zhang, Y.: High temperature causes negative whole-plant carbon balance under mild drought, New Phytol., 200, 330–339, https://doi.org/10.1111/nph.12400, 2013.
Zhou, X., Fu, Y., Zhou, L., Li, B., and Luo, Y.: An imperative need for global change research in tropical forests, Tree Physiol., 33, 903–912, https://doi.org/10.1093/treephys/tpt064, 2013.
Zuleta, D., Arellano, G ., Muller-Landau, H. C., McMahon, S. M., Aguilar, S., Bunyavejchewin, S., Cardenas, D., Chang-Yang, C. H., Duque, A., Mitre, D., Nasardin, M., Perez, R., Sun, I. F., Yao, T. L., and Davies, S. J.: Individual tree damage dominates mortality risk factors across six tropical forests, New Phytol., 233, 705–721, https://doi.org/10.1111/nph.17832, 2022.