the Creative Commons Attribution 4.0 License.
the Creative Commons Attribution 4.0 License.
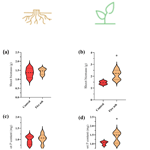
Direct foliar phosphorus uptake from wildfire ash
Daniel Palchan
Avner Gross
Atmospheric particles originating from combustion byproducts (burned biomass or wildfire ash) are highly enriched in nutrients such as P, K, Ca, Mg, Fe, Mn, and Zn. Over long timescales, deposited wildfire ash particles contribute to soil fertility by replenishing soil nutrient reservoirs. However, the immediate nutritional effects of freshly deposited fire ash on plants are mostly unknown. Here, we study the influence of fire ash on plant nutrition by applying ash separately on a plant's foliage or to its substrate around the roots. We conducted experiments on chickpea model plants under ambient and elevated CO2 levels, which reflect current and future climate scenarios. We found that plants can utilize fire ash P and Ni through their leaves by direct nutrient uptake from particles captured on their foliage but not via their roots, both under ambient and elevated CO2 levels. These results indicate that over a short timescale, plants effectively uptake P from fire ash only via the foliage rather than the root pathway, which is possibly due to low bioavailability or limited contact between fire ash particles and the roots. According to many previous studies, elevated levels of CO2 will reduce the ionome of plants due to the partial inhibition of the key root uptake mechanism, thus increasing the significance of foliar nutrient uptake in a future climate. Furthermore, the role of fire ash is expected to increase in the future world, thus giving a competitive advantage to plants that can utilize fire ash P from the foliar pathway, as fire ash P is a particularly efficient and important source of P.
- Article
(1764 KB) - Full-text XML
- Companion paper
-
Supplement
(539 KB) - BibTeX
- EndNote
Atmospheric particles are a major source of macro- and micronutrients such as phosphorus (P), iron (Fe), magnesium (Mg), calcium (Ca), potassium (K), manganese (Mn), zinc (Zn), and nickel (Ni) that are essential for terrestrial and marine ecosystems (Mikhailova et al., 2019; Aciego et al., 2017; Chadwick et al., 1999; Goll et al., 2022; Gross et al., 2015; Palchan et al., 2018). In terrestrial ecosystems, particularly the P-depleted Amazonian rainforest, the aeolian contribution of P is of special importance. The depletion of P in the Amazon is driven by processes such as leaching and plant uptake, coupled with the rapid fixation of P to soil minerals. Globally, P deficiency is prevalent due to insufficient P in the bedrock or slow weathering (Aciego et al., 2017), high leaching, or increased plant uptake (Vitousek et al., 2010; Gross et al., 2016; Lynch, 2011; Cunha et al., 2022; Hou et al., 2020). Combustion byproducts from biomass burning (fire ash) are among the most dominant atmospheric particles with global amounts of 750 Tg and can be deposited locally or transported remotely and supply nutrients even between continents (Barkley et al., 2019). More than 50 % of the fire ash particles are generated via high grassland and savanna fires in Africa, whereas the rest are emitted from the savannas in the Amazonian region or various drylands (Bauters et al., 2021; Yadav and Devi, 2019). For example, Barkley et al. (2019) have shown that African fire ash is wind-transported and supplies up to half of the P deposited annually in the Amazon basin. Bauters et al. (2021) showed that biomass burning in Africa is a major contributor of P to nearby equatorial forests by atmospheric deposition. According to their findings, the actual deposition of fire ash is higher over the forest because of the canopy complexity of the trees, pointing out the importance of the canopy trapping as a pathway for nutrient input into forest ecosystems. However, while the long-term impact of fire ash P reservoirs is well documented, the direct impact of freshly deposited fire ash on plant nutrition has been overlooked so far. Recent studies have shown that atmospheric deposition of desert dust has a direct and immediate impact on plant's nutrition by foliar P uptake from particles that are captured on their leaves (Gross et al., 2021; Starr et al., 2023; Lokshin et al., 2024) even though their P is mostly found in biologically unavailable forms (Gross et al., 2015; Longo et al., 2014). These studies suggest that plants facilitate P uptake from dust by enhancing the dissolution of insoluble P-bearing minerals via the exudation of P-solubilizing organic acids and the acidification of the leaf surface area.
The nutritional impact of fire ash may be even higher than that of dust and volcanic ash, as the concentration of P in fire ash particles can reach up to 10 000 ppm, which is 5–10 times larger than in desert dust (Tan and Lagerkvist, 2011; Tiwari et al., 2022; Barkley et al., 2019). The knowledge regarding the bioavailability and chemical speciation of P in fire ash is still poor; yet, solubility is supposedly significantly higher than that of desert dust and volcanic ash, owing to P-releasing chemical reactions that occur in high temperatures during biomass burning such as the high-melting crystalline phases (calcium/magnesium/potassium phosphates) during combustion (Tan and Lagerkvist, 2011; Tiwari et al., 2022; Myriokefalitakis et al., 2016; Anderson et al., 2010).
The contribution of fire ash as a nutrient supplier is expected to further increase under almost all future climate scenarios, as increased temperatures and drought frequency intensify fire weather conditions, which, in turn, escalate the number of fires and their intensities (Liu et al., 2014, 2010; Pausas and Keeley, 2021; Canadell et al., 2021). Thus, understanding the actual nutritional impact of fire ash on plants becomes even more relevant. Furthermore, elevated levels of CO2 generate a phenomenon known as the “CO2 fertilization effect”, which accelerates primary biomass production because of the increase in CO2 assimilation by plants (Loladze, 2002; Myers et al., 2014; Gojon et al., 2022). The larger biomass of plants increases their demand for P and other nutrients to sustain the stoichiometric imbalance caused by CO2 fertilization, making alternative nutrient sources such as fire ash more important.
A recent study that examined the impact of desert dust and volcanic ash on plants' nutrition emphasized the dominant role of the foliar nutrient uptake pathway under conditions of elevated levels of CO2 (eCO2), which are projected to reduce plant nutrient uptake from the root systems, causing a reduction in plant nutrient status (Lokshin et al., 2024). Other studies documented that plants that grow under eCO2 increase their efflux rates of soluble sugars, carboxylates, and organic acids such as oxalate and malate that promote the dissolution of nutrient-bearing minerals (Dong et al., 2021).
Here, we aim to study the immediate (i.e., several weeks') impact of fire ash on plant nutrition under current and future CO2 levels. We used chickpeas as our model plants since these plants have a unique set of properties that enhance the dissolution of nutrients from leaves or roots, such as high secretion of organic acids that lower the pH of their leaf or soil surroundings and a high concentration of trichomes on leaves that help them retain particles on their surfaces (Gross et al., 2021; Starr et al., 2023; Yoshida et al., 1997). The plants were grown under eCO2 and ambient CO2 (aCO2) conditions in a greenhouse. The plants were supplied with fire ash as their sole P source, which was applied both directly to the foliage and to the substrate around the root system, to assess the P-acquisition pathway that plants utilize from fire ash deposition. Additionally, we compare the impact of fire ash particles on plant nutrition to that of other atmospheric particles such as desert dust and volcanic ash. To further understand the impact of eCO2 conditions on plants' ability to uptake nutrients, we grew chickpea plants under eCO2, assessing the influence of fire ash P by applying it to the foliage and the substrate around the root system of the plants.
2.1 Plant material
The experiments were performed on chickpea plant that served as our model plant in this research (Cicer arietinum `Zehavit', a commercial Israeli kabuli cultivar). We used chickpea as the model plant because previous works had shown that chickpea positively responds to a foliar application of desert dust and volcanic ash (Gross et al., 2021; Lokshin et al., 2024). The plants were grown at the Gilat Research Center in southern Israel (31°210′ N, 34°420′ E) in a fully controlled glasshouse with an inner division into two rooms that are 2 × 4 m in size and 4 m tall. In both rooms, the temperature was fixed at 25±3 °C, with a relative humidity of 50 %–60 %. The rooms were equipped with a computer-controlled CO2 supply system (Emproco Ltd.; Ashkelon, Israel) that automatically adjusted the CO2 concentrations in the rooms. One room had CO2 levels of 400 ppm (aCO2), and the other room had CO2 levels of 850 ppm (eCO2) to simulate current and future earth CO2 levels based on a high-emission scenario (“business as usual”, SSP 8.5). Initially, the seeds per pot were sown in 54 pots filled with inert soilless media (Perlite 206; particle size of 0.075–1.5 mm; Agrekal; HaBonim, Israel). After germination, plants were thinned to one plant per pot. All the pots were supplied with a nutrition solution (fertigation) containing the following elements: N (50 mg L−1), P (3.5 mg L−1), K (50 mg L−1), Ca (40 mg L−1) Mg (10 mg L−1), Fe (0.8 mg L−1), Mn (0.4 mg L−1), Zn (0.2 mg L−1), B (0.4 mg L−1), Cu (0.3 mg L−1), and Mo (0.2 mg L−1). The mineral concentrations were achieved by proportionally dissolving NH4NO3, KH2PO4, KNO3, MgSO4, and NaNO3. The micronutrients were supplied in EDTA (ethylenediaminetetraacetic acid) chelates as commercial liquid fertilizer (Koratin; ICL Ltd). The location of each pot within the glasshouse was randomized at the beginning and changed every 2 weeks over the course of the experiment. The plants were drip-irrigated four times per day for 5 min via an automated irrigation system from the germination stage. At 14 d after germination (DAG), when plants were in early vegetative stages (two or three developed leaves), we changed the nutrient media for 42 of the pots to P-deficient media (P concentration of 0.1 mg L−1), with similar concentrations for the other elements (−P). Visible P deficiency symptoms (e.g., a chlorosis of mature leaves, a slight symptoms of necrotic leaf tips, and an overall decrease in biomass accumulation) were observed after 35 DAG. At this stage, 12 −P plants were applied with fire ash on the foliage (−P + leaf ash) and 12 into the substrate near the roots (−P + ash to the substrate), while the rest served as control group (−P or +P). Overall, each group had six repetitions, resulting in a total of 54 pots grouped as follows: +P (control group), −P (control group), −P + leaf ash, and −P + ash to the substrate.
2.2 Fire ash type
The fire ash in this study was produced by burning branches and leaves from five trees: Hyphaene thebaica, Olea europaea, Albizia lebbeck, Cupressus, and Pinus. Samples of Hyphaene thebaica, Olea europaea, and Albizia lebbeck were collected from the campus of Ben-Gurion University (31°15.680′ N, 34°47.964′ E). The fire ash that was added to the plants was produced by burning branches and needles of coniferous trees (Cupressus and Pinus) in a controlled bonfire setting. These branches and needles were collected from trees that grow in the Neve Shalom Forest, Israel (31°821′ N, 34°987′ E). Later, the ash was burned again in a furnace at 550 °C for 2 h to achieve complete combustion of the organic material. In the real world, fire ash particles exhibit a wide range of burning completeness and temperatures, along with various types of organic matter, including stems, branches, leaves, fruits, and needles. In our attempt to describe and quantify the phenomenon and recognize the variability in real-world conditions, we wanted to establish a “perfect” set of conditions. Complete combustion of organic material results in the production and oxidation of volatiles or gases such as CO2, carbon monoxide (CO), methane (CH4), or nitrogen dioxide (NO2), with only mineral residue remaining (i.e., “mineral ash”; Bodí et al., 2014). The ash was processed through a set of sieves to achieve a particle size smaller than 63 µm, the size subject to wind dispersion (Guieu et al., 2010). The chemical and mineralogical properties, as well as P concentrations of our fire ash samples, resemble reported values in the literature (Bigio and Angert, 2019; Tan and Lagerkvist, 2011; Tiwari et al., 2022).
2.3 Fire ash chemical and mineralogical analysis
To validate the typical chemical, mineralogical, and elemental composition of our fire ash samples and ensure comparability with other studies, we conducted X-ray diffraction (XRD) and X-ray fluorescence (XRF) as explained in the following. Mineralogical analysis of the fire ash was performed with XRD on 2 g of the fire ash using a Panalytical Empyrean powder diffractometer equipped with a position-sensitive X'Celerator detector. The data were collected in 2 h geometry using Cu Kα radiation (k=1.54178 Å) at 40 kV and 30 mA. Scans were run over ca. 15 min over a 2 h range between 5 and 65°, with an approximate step size of 0.033°. The phase analysis and quantification were performed using Match! v2.1.1 powder XRD analysis software with the PDF-2 crystallographic library (released in the year 2002) and the WinPlotr (September 2018 version) graphic tool for powder diffraction. We based the phase quantification on the relative intensity ratio method, which is determined by maximum intensity peaks relative to the corundum (Al2O3) maximum intensity peak (Hubbard and Snyder, 1988), using published intensity values for all identified phases. Elemental analysis was performed on 1 g of dried powder using XRF spectrometer (Panalytical Axios X-ray system) with a single goniometer-based measuring channel consisting of a Super Sharp X-ray tube with a rhodium anode operated at up to 60 kV and up to 50 mA at a maximum power level of 1 kW. The WDXRF used a beryllium (75 mm) anode tube window with different analyzing crystals (LiF(200) crystal, PE-002 crystal, Ge(111) crystal, PX1 synthetic multilayer monochromator, and PX7 synthetic multilayer monochromator) to analyze the range of elements. The instrument is equipped with two types of detectors: a flow detector for longer wavelengths and a scintillation detector for shorter wavelengths. The chemical and mineralogical properties of the fire ash are presented in Tables S1 and S2 in the Supplement. The total P and the total elemental analysis in fire ash were measured using inductively coupled plasma mass spectrometry (0.625 %). We performed a modified Hedley scheme to assess P fractionation in the four fire ash samples following a modified Hedley scheme (Mirabello et al., 2013; Gross et al., 2016). Briefly, P was sequentially extracted from 0.1 g of fire ash in four steps. First, the fire ash P was extracted with 30 mL of 0.5 M NaHCO3 (HCO3-P, which reflects the dissolved and labile P that is considered to have high bioavailability). In the second step, dust P was extracted with 1 M NaOH (NaOH-P, considered P that is loosely sorbed to Fe or Al oxides). In the third step, P was extracted with 1 M HCl (HCl-P; considered Ca–P complexes). The P in the extracts was measured using the molybdenum blue method (Murphy and Riley, 1962) in a microplate photometer (Multiskan Sky; Thermo Scientific). The chemical and mineralogical properties of the fire ash analogue are presented in Tables S1 and S4 in the Supplement.
2.4 Fire ash application
Fire ash was applied manually to the plants in two separate applications – either on the foliage or on the roots. The first fire ash application was made after the first visual deficiency signs occurred at 35 DAG. Since there are limited data on the actual deposition of fire ash on terrestrial ecosystems, we followed the application doses of Gross et al. (2021). Briefly, Gross et al. mimicked the yearly average amounts of dust deposition (January to April) in the western Negev region, Israel (Offer and Goossens, 2001; Uni and Katra, 2017), where the application dose was set to the equivalent value of 30 g m−2 (a typical dust deposition level during the major growth period). While this amount does not accurately represent the actual deposition of fire ash, it allows for a comparison of the impact of the two different atmospheric particles. The leaf area was determined in parallel trials in which the chickpea was grown in similar growing conditions. Based on this, 1.5 g of fire ash was applied on each application (between 35 and 45 DAG), resulting in a total of 3 g of ash per plant. The application of fire ash particles was performed in the following manner: we placed the ash particles in a sieve with a 63 µm mesh size and spread the ash by gently shaking the sieve above each plant, while some of the particles were spilled around the plants during the process. Prior to the application, the pots' surfaces were covered with nylon to prevent the settling of ash particles to the root system. Afterwards, the plants were left undisturbed with the ash particles settled on their foliage. The same amount of ash that was applied to the foliage was applied to the substrate around the root area. Afterward, ash particles were gently mixed around the roots to enhance the physical contact between the roots and the particles, thereby increasing the chances of having a more significant impact. All the surfaces of all the pots (−P foliar ash, −P root ash, −P, and +P) were covered with nylon to equalize plants' conditions and minimize the effects of unrelated processes.
2.5 Plant biomass and elemental analysis
The plants were harvested 10 d after the last ash application, which was at 55 DAG. Afterwards, we followed a cleaning method used in the study of Gross et al. (2021). Briefly, the plants were rinsed in tap water and 0.1 M HCl and three times in distilled water to remove any remaining ash residue. Then the plants were dried in an oven at 65 °C for 72 h and their dry weight was determined. Given that plant growth and alterations in plant ionome are evident through an increase in biomass, our examination focused on the above-ground part of the plants (shoot), including the stem, branches, and foliage. Sample preparation for mineral analysis was conducted as follows: the dry plants were ground to a fine powder in a stainless-steel ball mill (Retsch MM400; Germany). Elemental analysis was performed by burning 1 g of the plant material at 550 °C for 4 h to eliminate the organic material. The plant material treated with fire ash was subsequently dissolved using 1 ml of concentrated HNO3. The solution was diluted with double-distilled water (DDW) to achieve a 1 : 100 dilution. The obtained solution was then measured by the Agilent 8900 inductive coupled plasma mass spectrometer at Hebrew University (ICP-MS). Prior to analysis, the ICP-MS was calibrated with a series of multi-element standard solutions (1 pg mL−1–100 ng mL−1 Merck ME VI) and standards of major metals (300 ng mL−1–3 mg mL−1). An internal standard (50 ng mL−1 Sc and 5 ng mL−1 Re and Rh) was added to every standard and sample for drift correction. Standard reference solutions (USGS SRS T-207 and T-209) were examined at the beginning and end of the calibration to determine accuracy. The calculated accuracies for the major and trace elements are 3 % and 2 %, respectively. Biomass and elemental properties of the control plants, root and foliage-treated plants, and fire ash particles are given in Tables S2–S4.
2.6 Leaf pH and fire ash holding capacity
The pH was measured by manually attaching a portable pH electrode designed for flat surfaces (Eutech pH 150 P17/B-NS Epoxy, a pH electrode with a flat head for cream samples and surfaces that is refillable) onto the surface of three or four leaves from each plant. pH recordings were taken once a week between the beginning of a P-deficient nutrition until the day before the termination of the experiment (Gross et al., 2021; Starr et al., 2023; Yoshida et al., 1997) (Table S5). The measurement of leaf surface pH was made on P-deficient and P-sufficient plants but not the fire ash treated plants because the presence of the material on the leaf surface interferes with the physical contact between the flat surface of the pH electrode and the leaf itself.
Fire ash holding capacity is a measure for the maximal mass of ash that can be held on the surface of a leaf after the application of an excessive dose of ash and the removal of the free particles. This value was achieved by manually dispersing 1 g of ash on the adaxial surface of 0.5 g of fresh leaves, which were detached at the end of the experiment from 12 specially grown P-deficient and P-sufficient plants that did not receive the ash treatment (additional plants). These plants were taken to the Climate change research lab at Ben-Gurion University to perform the holding capacity analysis. After fire ash application, the leaves were gently shaken for 10 s and weighed. The differences between the weights before and after the ash application represent the leaf holding capacity (Gajbhiye et al., 2016).
2.7 Statistical analysis
Treatment comparisons for all measured parameters were tested using post hoc Tukey honest significant difference (HSD) tests (P<0.05). The significant differences are denoted using different letters in the figures. The standard errors in the mean in the vertical bars (in the figures) were calculated using GraphPad Prism version 9.0.0.
3.1 Fractionation of P in fire ash samples
Most of the P in fire ash produced from four different tree types was found in the HCl fraction (ranging from 59 %–80 %). The fraction of NaHCO3-P ranged from 18 %–39 %. The NaOH-P fraction ranged from 1 %–2 %. The average total P ranged from 5382 to 7323 mg g−1 (Fig. 1).
3.2 Shoot biomass and total P under aCO2
Changes in P concentration were not observed due to P starvation. Thus, in this case, presenting changes in terms of total P rather than concentration is the correct way to show the foliar P uptake (as well as under eCO2). Plants that received foliar application of fire ash showed a significant increase in their biomass and P content (Fig. 2b, d). Control plants had a dry biomass of 1.36 g, while the foliar-treated plants had a dry biomass of 2.14 g, indicating an increase of 57.0 %. Similarly, the P content increased from 0.96 to 1.45 mg, representing a growth of 50.3 %. No increases in biomass or P content were observed in plants that received fire ash in the substrate (Fig. 2a, c).
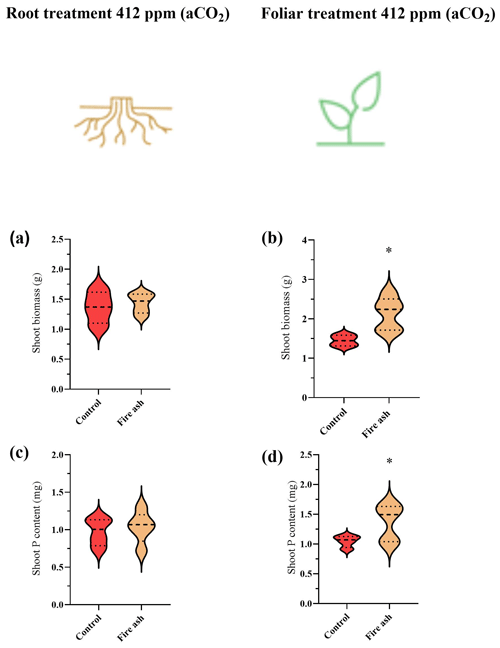
Figure 2(a–d) The biomass and P content in chickpea plants that were grown under aCO2 levels, after the application of fire ash in the substrate or to the foliage. Control plants with no ash application are colored in red, while plants treated with fire ash are indicated by a bright brown color. (a, b) Shoot biomass of plants that had fire ash applied to their roots or their leaves. (c, d) P content of plants that were applied with fire ash on their roots or their leaves. Asterisks represent statistically significant differences between bars (P<0.05; Tukey test). Error bars represent standard deviations (n=5). The results are presented in a violin plot, where the dashed line represents the median and thin dotted lines indicate quartiles.
3.3 Shoot biomass and total P under eCO2
Plants that received a foliar application of fire ash show a significant increase in their biomass and P content (Fig. 3b, d). Control plants had a dry biomass of 1.50 g, while the foliar-treated plants had a dry biomass of 1.84 g, representing an increase of 23.2 %. Additionally, the P content rose from 1.03 to 1.17 mg, reflecting an increase of 12.6 %. No increases in biomass or P content were observed in plants that received fire ash in the substrate (Fig. 3a, c).
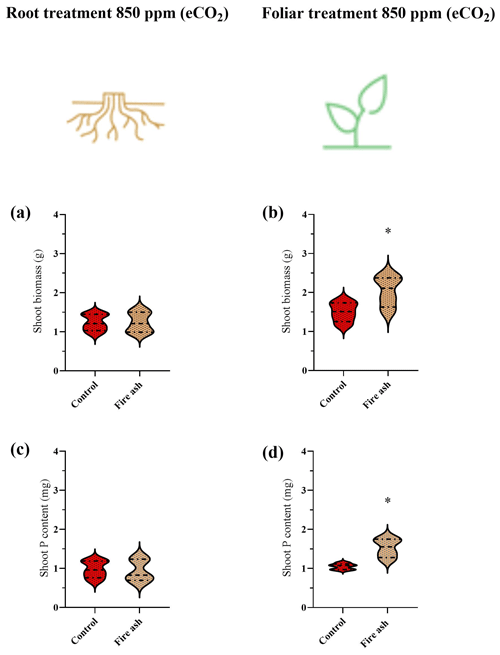
Figure 3(a–d) The biomass and P content in chickpea plants that were grown under eCO2 levels, after the application of fire ash in the substrate or to the foliage. Control plants with no ash application are colored in red, and plants that had fire ash applied to them are colored in bright brown. (a, b) Shoot biomass of plants that were treated with fire ash on their roots or their leaves. (c, d) P content of plants that were treated with fire ash on their roots or their leaves. Asterisks represent statistically significant differences between bars (P<0.05; Tukey test), n=5. The results are presented in a violin plot, where the dashed line represents the median and thin dotted lines indicate quartiles.
3.4 Shoot nutrient status under eCO2 conditions
To quantify the impact of elevated eCO2 conditions on the nutrient status of plant samples, we conducted a comparison between the ionome of chickpea plants grown under aCO2 and those grown under eCO2 levels for plants both treated and untreated with foliar fire ash. The comparison was conducted as follows: the average value of each nutrient in plants grown under aCO2 was calculated, and then each nutrient in individual chickpea plants grown under eCO2 levels was expressed as a ratio relative to the average under aCO2 conditions (eCO2 planteach individual plant aCO2 plantaverage of all the control plants). We observed that eCO2 conditions led to a reduction in the concentrations of Mg, K, Ca, Mn, Zn, Cu, Fe, and Ni, ranging from 28.7 % (Fe) to 90 % (Ni). The plants treated with foliar fire ash indicate a significant increase in the concentration of Ni, offsetting the reduction in the control untreated plants that was seen under eCO2 from −90 % to −60 %, representing a 33 % increase. No significant changes were observed for other nutrients (Fig. 4).
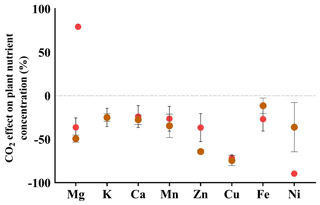
Figure 4The percentage (%) change in the nutrient concentration of plants that were grown under eCO2 conditions in comparison to the plants that were grown under aCO2 conditions. Red circles represent untreated control plants. Brown circles represent plants that received fire ash through foliar application. The dashed line represents the average values of the control plants grown under aCO2 levels; the values below the dashed line indicate a decline in the respective nutrient under eCO2 levels. Error bars represent standard deviations (n=5).
3.5 Leaf surface properties
Leaf surface pH ranged from 1 to 1.3, with an average value of 1.18 (Table S5). Fire ash holding capacity ranged from 0.07 to 0.17 g, with an average value of 0.13 g (presented in Table S6). Fire ash holding capacity values were at the same order of magnitude as the values of desert dust and fire ash that were reported in Lokshin et al. (2024).
4.1 XRD, XRF, and total P
The XRF, XRD, and total P values of the fire ash samples in our study are within the same order of magnitude as those found in the ash of Mediterranean savanna forests (Sánchez-García et al., 2023). Also, the fractionation of P in the ashes aligns with fractionation reported in other studies (Wu et al., 2023). The results demonstrate fire ash exhibit a higher concentration of total P in comparison to soils, mineral dust, or volcanic ash (Ranatunga et al., 2009; Tiwari et al., 2022; Starr et al., 2023), with a significant percentage being in soluble form (18 %–39 %). Yet, the majority of fire ash P is not readily soluble and is found in biologically unavailable form.
4.2 P uptake from fire ash under aCO2 levels
Foliar application of fire ash under ambient CO2 levels increased the chickpea biomass and total P content compared to untreated control plants, demonstrating that foliar uptake of P from fire ash has a direct nutritional impact on plants. The average P concentration of both the control and treated plants ranged between 600 and 800 µg g−1 (Tables S3 and S4), indicating P starvation (Gross et al., 2021). Therefore, we assume that all additional P absorbed via the foliar pathway has been directed towards gaining biomass and boosting photosynthesis, maintaining the P concentration at the same level. Chickpea plants were more responsive to fire ash in comparison to experiments with desert dust and volcanic ash from Lokshin et al. (2024), demonstrating the importance of fire ash P for plant growth. However, because there was no nutritional impact when fire ash was deposited in the substrate area near the roots, we conclude the nutritional impact occurred exclusively through the foliar pathway, even though the concentration and solubilities of P and other nutrients in fire ash are considered higher in comparison to desert dust and volcanic ash (Bigio and Angert, 2019; Gross et al., 2015; Tan and Lagerkvist, 2011; Tiwari et al., 2022). The low bioavailability of fire ash P for root uptake implies that fire ash P is not fully soluble in the rhizosphere and may be loosely attached to Ca, Fe, or Al that prevents its utilization (Masto et al., 2013; Qian et al., 2009; Tan and Lagerkvist, 2011; Santín et al., 2018). Another reason for the roots' impairment in P uptake might be the insufficient physical contact between fire ash particles and the roots, even though the tested plants exhibited an extensive root system, with an average root-to-shoot ratio of 50 : 50; the pH of the substrate was around 7, in the range of most alkaline soils. In contrast, plants' foliage has a greater surface area that increases the direct physical contact between fire ash particles and plant tissues, thus creating suitable conditions for their partial solubilization. These results suggest that solubility tests examined by chemical extractions do not necessarily reflect actual biological availability and emphasize the importance of fertilization experiments with plants. It is difficult to compare our results with other studies because direct foliar uptake of P from fire ash particles has been overlooked until now. As far as we know, our results represent the first experiment in which fire ash particles were directly added to plants, thus definitively challenging the common perception that P is solely taken up through the roots from the soil, even in the case of atmospheric particle deposition.
4.3 Foliar nutrient uptake mechanism
Gross et al. (2021) and Lokshin et al. (2024) have studied foliar nutrient uptake mechanisms and pointed out that the presence of trichomes facilitates the adhesion of dust captured on leaf surface and promotes the release of P solubilizing metabolites, such as malic, citric, and oxalic acids. In addition to the natural secretion of organic acids, the increased secretion may be enhanced as a result of the breakage of trichomes, following the application of atmospheric particles on plants foliage. As in previous studies, we also measured a highly acidic leaf surface environment (average pH value of 1.15) and a high fire ash holding capacity (average value of 15 %), thus supporting the previous assertions that a low pH and a high holding capacity may help facilitate P uptake on plant leaves. The combination of leaf surface acidification, secretion of organic acids, and additional exudations combined with an increased trichomes density enhances capture and holding of particles, which in turn facilitates the foliar nutrient uptake in chickpea (chickpea leaves' holding capacity of fire ash particles is presented in Table S6).
4.4 Foliar nutrient uptake under eCO2 levels
In accordance with previous studies, growing chickpea plants under eCO2 levels drove a significant reduction in nutrient status in comparison to plants that were grown under aCO2 levels despite receiving the same fertigation solution. The nutritional decline under eCO2 mostly occurs due to the “dilution” effect, where accumulation of carbon (C) exceeds that of mineral nutrients, and, more importantly, because of a result of a partial inhibition of key root uptake mechanisms (Myers et al., 2014; Loladze, 2002; Gojon et al., 2022). In our experiment, the control group was grown under eCO2 conditions and did not exhibit an increase in biomass. This indicates that the dilution effect is not responsible for the nutrient reduction observed in our study and suggests that the primary reason for the nutritional reduction is likely the downregulation of the transfer from the roots to shoot or root uptake. As a result of the foliar application of fire ash particles, the nutritional reduction under eCO2 was partially offset, leading to an increase in plants' Ni concentrations (Fig. 3). Yet, the nutritional contribution of foliar fire ash under eCO2 levels was less prominent compared to the foliar application of desert dust and volcanic ash, which, in addition to Ni, also increased the concentration of Fe (Lokshin et al., 2024). We anticipated that the foliage would absorb additional nutrients such as K, Ca, and Zn, which are present in higher concentrations in fire ash compared to desert dust and volcanic ash (Table S2). The absence of an impact of fire ash on the chickpea Fe concentration contrasts with previous laboratory studies that demonstrated substantially greater Fe solubilities in combustion-derived aerosols compared to crystalline minerals (Schroth et al., 2009; Fu et al., 2012). One reason for the low response to fire ash Fe may be related to incomplete combustion, which determines the bioavailability of mineral nutrients in fire ash that is mediated by combustion temperature and the presence of oxygen (Tan and Lagerkvist, 2011). In addition, natural forest fires exhibit varying combustion conditions, adding to the complexity of projecting the actual nutrient bioavailability of wildfires (Bodí et al., 2014). Thus, the actual availability of fire ash nutrients such as Fe, P, and others warrants further research based on laboratory and field fertilization experiments at different combustion conditions.
4.5 Broader aspect
We recognize that in other plants, the phenomenon of foliar uptake might be less pronounced (Starr et al., 2023; Gross et al., 2021). In our opinion, selecting a suitable plant was essential to effectively demonstrate it. In a broader context, numerous articles have documented the contribution of wildfire ash to soil fertility through long-term processes, despite the fact that fire ash P contains a significant proportion of labile P (Bauters et al., 2021; Barkley et al., 2019; Bodí et al., 2014). The current perception dictates that fire ash nutrients are not immediately available to plants but rather interact with soil components and bind to minerals in the soil (Tiwari et al., 2022; Chadwick et al., 1999; Okin et al., 2004). Our results demonstrated that in the time frame of a few weeks, the contribution of fire ash through the roots is negligible. In contrast, fire ash has an immediate nutritional significance to plants via foliar nutrient uptake pathway. It happens due to the foliar secretion of organic acids, such as oxalic and malic, which facilitate the dissolution of fire ash P. Bauters et al. (2021) emphasized that the canopy of the trees in African tropical forests captures P from biomass-burning byproducts, which, upon settling on the ground with rain, contributed P to the nutrition of trees as was evident by larger and denser trees growth. We postulate that at least part of that fire ash P was taken up directly by the foliage. In a future world where the quantity and intensity of fires are expected to rise alongside the increasing demand for P due to the CO2 fertilization effect, the contribution of fire ash to natural ecological systems will increase. The fact that the foliar uptake mechanism remains generally unaffected under eCO2 suggests that this pathway may become crucial. Plants exhibiting the foliar nutrient uptake trait are more likely to benefit from fire ash fertilization in a future world. Another important contribution of fire ash P deposition is its potential to alleviate the ecological stoichiometric imbalance resulting from anthropogenic nitrogen (N) pollution in soils, which is expected to grow in the next few decades (Liu et al., 2013) and disrupt plants and the ecosystem's function. Thus, fire ash may play a larger role in a world that shifts from N to P limitation (Du et al., 2020). Currently, biogeochemical models do not incorporate foliar P uptake from fire ash and other atmospheric particles such as desert dust and volcanic ash. Given the substantial contribution of foliar uptake to plant nutrition from atmospheric deposition, it is imperative to include this process in global carbon and vegetation models. Such models should take into account the canopy surface areas, canopy roughness, soil fertility, and geographical distribution of atmospheric particles sources and sinks. This inclusion will lead to a more comprehensive description of the global P cycle.
We have conducted controlled experiments where we have grown chickpea plants under ambient and elevated CO2 conditions. We fertilized the plants separately on foliage and roots with fresh fire ash and harvested the plants after 4 weeks. We then studied the plants' elemental composition and reached the following conclusions:
-
Freshly deposited fire ash has a direct impact on plant P nutrition only through the foliar nutrient uptake pathway and not via the root system.
-
The root acquisition of freshly deposited fire ash P and other nutrients is limited despite the high solubility of fire ash P.
-
In general, P uptake from fire ash was higher than that of other natural atmospheric particles such as desert dust and volcanic ash.
-
Foliar nutrient uptake from fire ash is sustained even under elevated CO2 conditions, implying that the significance of foliar nutrient uptake from fire ash will increase in future climates. This is expected as the ionome of plants is anticipated to decrease, primarily due to the partial downregulation of the roots' nutrient uptake mechanism.
Source data for the main text, methods, and supplementary information are provided in the tables of the Supplement.
The supplement related to this article is available online at: https://doi.org/10.5194/bg-21-2355-2024-supplement.
AL performed the growing experiments and sample preparations and wrote the article along with input from all authors. DP and AG conceived and supervised the entire project.
The contact author has declared that none of the authors has any competing interests.
Publisher’s note: Copernicus Publications remains neutral with regard to jurisdictional claims made in the text, published maps, institutional affiliations, or any other geographical representation in this paper. While Copernicus Publications makes every effort to include appropriate place names, the final responsibility lies with the authors.
Anton Lokshin is grateful for the financial support provided by the Keren Kayemeth LeIsrael-Jewish National Fund (KKL-JNF) climate team doctoral fellowship. We thank Yigal Erel and Ofir Tirosh for their support with inductively coupled plasma mass spectrometry analyses. The project was funded by the Israeli Science Foundation (ISF), grant number 144/19.
This research has been supported by the Keren Kayemeth LeIsrael-Jewish National Fund (KKL-JNF) climate team doctoral fellowship and the Israeli Science Foundation (ISF) (grant no. 144/19).
This paper was edited by Sara Vicca and reviewed by two anonymous referees.
Aciego, S. M., Riebe, C. S., Hart, S. C., Blakowski, M. A., Carey, C. J., Aarons, S. M., Dove, N. C., Botthoff, J. K., Sims, K. W. W., and Aronson, E. L.: Dust outpaces bedrock in nutrient supply to montane forest ecosystems, Nat. Commun., 8, 14800, https://doi.org/10.1038/ncomms14800, 2017.
Anderson, L. D., Faul, K. L., and Paytan, A.: Phosphorus associations in aerosols: What can they tell us about P bioavailability?, Mar. Chem., 120, 44–56, 2010.
Barkley, A. E., Prospero, J. M., Mahowald, N., Hamilton, D. S., Popendorf, K. J., Oehlert, A. M., Pourmand, A., Gatineau, A., Panechou-Pulcherie, K., Blackwelder, P., and Gaston, C. J.: African biomass burning is a substantial source of phosphorus deposition to the Amazon, Tropical Atlantic Ocean, and Southern Ocean, P. Natl. Acad. Sci. USA, 116, 16216–16221, https://doi.org/10.1073/pnas.1906091116, 2019.
Bauters, M., Drake, T. W., Wagner, S., Baumgartner, S., Makelele, I. A., Bodé, S., Verheyen, K., Verbeeck, H., Ewango, C., Cizungu, L., Van Oost, K., and Boeckx, P.: Fire-derived phosphorus fertilization of African tropical forests, Nat. Commun., 12, 5129, https://doi.org/10.1038/s41467-021-25428-3, 2021.
Bigio, L. and Angert, A.: Oxygen Isotope Signatures of Phosphate in Wildfire Ash, ACS Earth Space Chem., 3, 760–769, https://doi.org/10.1021/acsearthspacechem.8b00216, 2019.
Bodí, M. B., Martin, D. A., Balfour, V. N., Santín, C., Doerr, S. H., Pereira, P., Cerdà, A., and Mataix-Solera, J.: Wildland fire ash: Production, composition and eco-hydro-geomorphic effects, 130, 103–127, https://doi.org/10.1016/j.earscirev.2013.12.007, 2014.
Canadell, J. G. and Jackson, R. B. (Eds.): Ecosystem collapse and climate change, Cham, Switzerland, Springer, 2021.
Chadwick, O. A., Derry, L. A., Vitousek, P. M., Huebert, B. J., and Hedin, L. O.: Changing sources of nutrients during four million years of ecosystem development, Nature, 397, 491–497, https://doi.org/10.1038/17276, 1999.
Cunha, H. F. V., Andersen, K. M., Lugli, L. F., Santana, F. D., Aleixo, I. F., Moraes, A. M., Garcia, S., Di Ponzio, R., Mendoza, E. O., Brum, B., Rosa, J. S., Cordeiro, A. L., Portela, B. T. T., Ribeiro, G., Coelho, S. D., de Souza, S. T., Silva, L. S., Antonieto, F., Pires, M., Salomão, A. C., Miron, A. C., de Assis, R. L., Domingues, T. F., Aragão, L. E. O. C., Meir, P., Camargo, J. L., Manzi, A. O., Nagy, L., Mercado, L. M., Hartley, I. P., and Quesada, C. A.: Direct evidence for phosphorus limitation on Amazon forest productivity, Nature, 608, 558–562, https://doi.org/10.1038/s41586-022-05085-2, 2022.
Dong, J., Hunt, J., Delhaize, E., Zheng, S. J., Jin, C. W., and Tang, C.: Impacts of elevated CO2 on plant resistance to nutrient deficiency and toxic ions via root exudates: A review, Sci. Total Environ., 754, 142434, https://doi.org/10.1016/j.scitotenv.2020.142434, 2021.
Du, E., Terrer, C., Pellegrini, A. F. A., Ahlström, A., van Lissa, C. J., Zhao, X., Xia, N., Wu, X., and Jackson, R. B.: Global patterns of terrestrial nitrogen and phosphorus limitation, Nat. Geosci., 13, 221–226, https://doi.org/10.1038/s41561-019-0530-4, 2020.
Fu, H., Lin, J., Shang, G., Dong, W., Grassian, V. H., Carmichael, G. R., Li, Y., and Chen, J.: Solubility of iron from combustion source particles in acidic media linked to iron speciation, Environ. Sci. Technol., 46, 11119–11127, https://doi.org/10.1021/es302558m, 2012.
Gajbhiye, T., Kim, K. H., Pandey, S. K., and Brown, R. J.: Foliar transfer of dust and heavy metals on roadside plants in a subtropical environment, Asian J. Atmos. Environ., 10, 137–145, 2016.
Gojon, A., Cassan, O., Bach, L., Lejay, L., and Martin, A.: The decline of plant mineral nutrition under rising CO2: physiological and molecular aspects of a bad deal, Trends Plant Sci., 28, 185–198, https://doi.org/10.1016/j.tplants.2022.09.002, 2022.
Goll, D. S., Bauters, M., Zhang, H., Ciais, P., Balkanski, Y., Wang, R., and Verbeeck, H.: Atmospheric phosphorus deposition amplifies carbon sinks in simulations of a tropical forest in Central Africa, New Phytol., 237, 2054–2068, https://doi.org/10.1111/nph.18535, 2022.
Gross, A., Goren, T., Pio, C., Cardoso, J., Tirosh, O., Todd, M. C., Rosenfeld, D., Weiner, T., Custódio, D., and Angert, A.: Variability in sources and concentrations of saharan dust phosphorus over the Atlantic Ocean, Environ. Sci. Tech. Lett., 2, 31–37, https://doi.org/10.1021/ez500399z, 2015.
Gross, A., Turner, B. L., Goren, T., Berry, A., and Angert, A.: Tracing the Sources of Atmospheric Phosphorus Deposition to a Tropical Rain Forest in Panama Using Stable Oxygen Isotopes, Environ. Sci. Technol., 50, 1147–1156, https://doi.org/10.1021/acs.est.5b04936, 2016.
Gross, A., Tiwari, S., Shtein, I., and Erel, R.: Direct foliar uptake of phosphorus from desert dust, New Phytol., 230, 2213–2225, https://doi.org/10.1111/nph.17344, 2021.
Guieu, C., Dulac, F., Desboeufs, K., Wagener, T., Pulido-Villena, E., Grisoni, J.-M., Louis, F., Ridame, C., Blain, S., Brunet, C., Bon Nguyen, E., Tran, S., Labiadh, M., and Dominici, J.-M.: Large clean mesocosms and simulated dust deposition: a new methodology to investigate responses of marine oligotrophic ecosystems to atmospheric inputs, Biogeosciences, 7, 2765–2784, https://doi.org/10.5194/bg-7-2765-2010, 2010.
Hou, E., Luo, Y., Kuang, Y., Chen, C., Lu, X., Jiang, L., Luo, X., and Wen, D.: Global meta-analysis shows pervasive phosphorus limitation of aboveground plant production in natural terrestrial ecosystems, Nat. Commun., 11, 637, https://doi.org/10.1038/s41467-020-14492-w, 2020.
Hubbard, C. R. and Snyder, R. L.: RIR-measurement and use in quantitative XRD, Powder Diffraction, 3, 74–77, 1988.
Liu, X., Zhang, Y., Han, W., Tang, A., Shen, J., Cui, Z., Vitousek, P., Erisman, J. W., Goulding, K., Christie, P., Fangmeier, A., and Zhang, F.: Enhanced nitrogen deposition over China, Nature, 494, 459–462, https://doi.org/10.1038/nature11917, 2013.
Liu, Y., Stanturf, J., and Goodrick, S.: Trends in global wildfire potential in a changing climate, Forest Ecol. Manage., 259, 685–697, https://doi.org/10.1016/j.foreco.2009.09.002, 2010.
Liu, Y., Goodrick, S., and Heilman, W.: Wildland fire emissions, carbon, and climate: Wildfire-climate interactions, Forest Ecol. Manage., 317, 80–96, https://doi.org/10.1016/j.foreco.2013.02.020, 2014.
Lokshin, A., Palchan, D., and Gross, A.: Foliar nutrient uptake from dust sustains plant nutrition, The New Phytologist, in review, 2024.
Loladze, I.: Rising atmospheric CO2 and human nutrition: Toward globally imbalanced plant stoichiometry?, Trend. Ecol. Evol., 17, 457–461, https://doi.org/10.1016/S0169-5347(02)02587-9, 2002.
Longo, A. F., Ingall, E. D., Diaz, J. M., et al.: P‐NEXFS analysis of aerosol phosphorus delivered to the Mediterranean Sea, Geophys. Res. Lett., 41, 4043–4049, 2014.
Lynch, J. P.: Root phenes for enhanced soil exploration and phosphorus acquisition: Tools for future crops, Plant Physiol., 156, 1041–1049, https://doi.org/10.1104/pp.111.175414, 2011.
Masto, R. E., Mahato, M., Selvi, V. A., and Ram, L. C.: The effect of fly ash application on phosphorus availability in an acid soil, Energ. Source Part A, 35, 2274–2283, https://doi.org/10.1080/15567036.2010.533337, 2013.
Mikhailova, E. A., Post, G. C., Cope, M. P., Post, C. J., Schlautman, M. A., and Zhang, L.: Quantifying and mapping atmospheric potassium deposition for soil ecosystem services assessment in the United States, Front. Environ. Sci., 7, 74, https://doi.org/10.3389/fenvs.2019.00074, 2019.
Mirabello, M. J., Yavitt, J. B., Garcia, M., Harms, K. E., Turner, B. L., and Wright, S. J.: Soil phosphorus responses to chronic nutrient fertilisation and seasonal drought in a humid lowland forest, Panama, Soil Res., 51, 215–221, 2013.
Murphy, J. A. M. E. S. and Riley, J. P.: A modified single solution method for the determination of phosphate in natural waters, Anal. Chim. Ac., 27, 31–36, 1962.
Myers, S. S., Zanobetti, A., Kloog, I., Huybers, P., Leakey, A. D. B., Bloom, A. J., Carlisle, E., Dietterich, L. H., Fitzgerald, G., Hasegawa, T., Holbrook, N. M., Nelson, R. L., Ottman, M. J., Raboy, V., Sakai, H., Sartor, K. A., Schwartz, J., Seneweera, S., Tausz, M., and Usui, Y.: Increasing CO2 threatens human nutrition, Nature, 510, 139–142, https://doi.org/10.1038/nature13179, 2014.
Myriokefalitakis, S., Nenes, A., Baker, A. R., Mihalopoulos, N., and Kanakidou, M.: Bioavailable atmospheric phosphorous supply to the global ocean: a 3-D global modeling study, Biogeosciences, 13, 6519–6543, https://doi.org/10.5194/bg-13-6519-2016, 2016.
Offer, Z. Y. and Goossens, D.: Ten years of aeolian dust dynamics in a desert region (Negev desert, Israel): Analysis of airborne dust concentration, dust accumulation and the high-magnitude dust events, J. Arid. Environ., 47, 211–249, https://doi.org/10.1006/jare.2000.0706, 2001.
Okin, G. S., Mahowald, N., Chadwick, O. A., and Artaxo, P.: Impact of desert dust on the biogeochemistry of phosphorus in terrestrial ecosystems, Global Biogeochem. Cy., 18, GB2005, https://doi.org/10.1029/2003GB002145, 2004.
Palchan, D., Stein, M., Goldstein, S. L., Almogi-Labin, A., Tirosh, O., and Erel, Y.: Synoptic conditions of fine-particle transport to the last interglacial Red Sea-Dead Sea from Nd-Sr compositions of sediment cores, Quaternary Sci. Rev., 179, 123–136, https://doi.org/10.1016/j.quascirev.2017.09.004, 2018.
Pausas, J. G. and Keeley, J. E.: Wildfires and global change, Front. Environ., 19, 387–395, 2021.
Qian, Y., Miao, S. L., Gu, B., and Li, Y. C.: Effects of Burn Temperature on Ash Nutrient Forms and Availability from Cattail (Typha domingensis) and Sawgrass (Cladium jamaicense) in the Florida Everglades, J. Environ. Qual., 38, 451–464, https://doi.org/10.2134/jeq2008.0126, 2009.
Ranatunga, T. D., Taylor, R. W., Bhat, K. N., Reddy, S. S., Senwo, Z. N., and Jackson, B.: Inorganic phosphorus forms in soufrière hills volcanic ash and volcanic ash-derived soil, Soil Sci., 174, 430–438, https://doi.org/10.1097/SS.0b013e3181b6deab, 2009.
Sánchez-García, C., Santín, C., Neris, J., Sigmund, G., Otero, X. L., Manley, J., González-Rodríguez, G., Belcher, C. M., Cerdà, A., Marcotte, A. L., Murphy, S. F., Rhoades, C. C., Sheridan, G., Strydom, T., Robichaud, P. R., and Doerr, S. H.: Chemical characteristics of wildfire ash across the globe and their environmental and socio-economic implications, Environ. Int., 178, 108065, https://doi.org/10.1016/j.envint.2023.108065, 2023.
Santín, C., Otero, X. L., Doerr, S. H., and Chafer, C. J.: Impact of a moderate/high-severity prescribed eucalypt forest fire on soil phosphorous stocks and partitioning, Sci. Total Environ., 621, 1103–1114, https://doi.org/10.1016/j.scitotenv.2017.10.116, 2018.
Schroth, A. W., Crusius, J., Sholkovitz, E. R., and Bostick, B. C.: Iron solubility driven by speciation in dust sources to the ocean, Nat. Geosci., 2, 337–340, https://doi.org/10.1038/ngeo501, 2009.
Starr, M., Klein, T., and Gross, A.: Direct foliar acquisition of desert dust phosphorus fertilizes forest trees despite reducing photosynthesis, Tree Physiol., 43, 794–804, https://doi.org/10.1093/treephys/tpad012, 2023.
Tan, Z. and Lagerkvist, A.: Phosphorus recovery from the biomass ash: A review, Renew. Sust. Energ. Rev., 15, 3588–3602, https://doi.org/10.1016/j.rser.2011.05.016, 2011.
Tiwari, S., Erel, R., and Gross, A.: Chemical processes in receiving soils accelerate solubilisation of phosphorus from desert dust and fire ash, Eur. J. Soil Sci., 73, e13270, https://doi.org/10.1111/ejss.13270, 2022.
Uni, D. and Katra, I.: Airborne dust absorption by semi-arid forests reduces PM pollution in nearby urban environments, Sci. Total Environ., 598, 984–992, https://doi.org/10.1016/j.scitotenv.2017.04.162, 2017.
Vitousek, P. M., Porder, S., Houlton, B. Z., and Chadwick, O. A.: Terrestrial phosphorus limitation: Mechanisms, implications, and nitrogen-phosphorus interactions, Ecol. Appl., 20, 5–15, https://doi.org/10.1890/08-0127.1, 2010.
Wu, Y., Pae, L. M., Gu, C., and Huang, R.: Phosphorus Chemistry in Plant Ash: Examining the Variation across Plant Species and Compartments, ACS Earth Space Chem., 7, 2205–2213, https://doi.org/10.1021/acsearthspacechem.3c00145, 2023.
Yadav, I. C. and Devi, N. L.: Biomass burning, regional air quality, and climate change, in: Encyclopedia of Environmental Health, Elsevier, 386–391, https://doi.org/10.1016/B978-0-12-409548-9.11022-X, 2019.
Yoshida, M., Cowgill, S. E., and Wightman, J. A.: Roles of oxalic and malic acids in Chickpea trichome exudate in host-plant resistance to Helicoverpa armigera, J. Chem. Ecol., 23, 1195–1210, https://doi.org/10.1023/B:JOEC.0000006395.45516.e8, 1997.