the Creative Commons Attribution 4.0 License.
the Creative Commons Attribution 4.0 License.
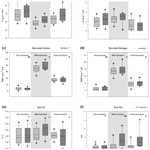
Long-term fertilization increases soil but not plant or microbial N in a Chihuahuan Desert grassland
Violeta Mendoza-Martinez
Scott L. Collins
Although the negative consequences of increased nitrogen (N) supply for plant communities and soil chemistry are well known, most studies have focused on mesic grasslands, and the fate of added N in arid and semi-arid ecosystems remains unclear. To study the impacts of long-term increased N deposition on ecosystem N pools, we sampled a 26-year-long fertilization (10 g N m−2 yr−1) experiment in the northern Chihuahuan Desert at the Sevilleta National Wildlife Refuge (SNWR) in New Mexico. To determine the fate of the added N, we measured multiple soil, microbial, and plant N pools in shallow soils at three time points across the 2020 growing season. We found small but significant increases with fertilization in soil-available NO-N and NH-N, yet the soil microbial and plant communities do not appear to be taking advantage of the increased N availability, with no changes in biomass or N content in either community. However, there were increases in total soil N with fertilization, suggesting increases in microbial or plant N earlier in the experiment. Ultimately, the majority of the N added in this multi-decadal experiment was not found in the shallow soil or the microbial or plant community and is likely to have been lost from the ecosystem entirely.
- Article
(1012 KB) - Full-text XML
- BibTeX
- EndNote
Agriculture and other anthropogenic activities have disrupted the nitrogen (N) cycle and increased N deposition in ecosystems worldwide (Vitousek et al., 1997). Reactive N released into the atmosphere has doubled over the last 50 years (Galloway et al., 2008), more than half of which has been attributed to crop production (Liu et al., 2022), and the southwestern United States has become a region of particular concern. Higher levels of atmospheric N deposition often increase plant biomass and alter soil chemistry in grasslands across the globe (Fay et al., 2015; Fenn et al., 2003a, b), but the effects of chronic N addition in arid ecosystems remain unclear. Although drylands are known to be primarily water-limited ecosystems (Noy-Meir et al., 1973; Collins et al., 2008), multiple studies hint that a sequential limitation by water then N may exist (e.g., Hall et al., 2011; Ladwig et al., 2012; Rao and Allen, 2010; Rao et al., 2011), and thus increased N deposition in these ecosystems may result in shifts in ecosystem nutrient availability and cycling, as well as changes in plant and microbial biomass (Chalcraft et al., 2008; Stevens et al., 2010; Wei et al., 2013). Drylands represent Earth's largest biome (Schimel, 2010), support nearly 40 % of the world's population (Adeel and Safriel, 2005), and are dominant drivers of interannual variability in the global terrestrial carbon (C) sink (Poulter et al., 2014; Ahlström et al., 2015). Therefore, understanding alterations to ecosystem processes caused by increased levels of N deposition is critical.
There are a number of different ultimate sinks for N added to ecosystems – changing soil nutrient pools, uptake by organisms, or potentially being lost from the system through leaching or volatilization (Peterjohn and Schlesinger, 1990; McCalley and Sparks, 2009; Brown et al., 2022; Püspök et al., 2022), with the ultimate destination of the added N dependent on seasonality of both N and water inputs, as well as potential seasonally dependent limiting factors for plants and microbes (Homyak et al., 2014). For example, nutrient cycling models indicate that N inputs of 20 to 35 kg ha−1 yr−1 in semi-arid ecosystems lead to higher concentrations of NO leaching beyond the primary rooting zone, a decrease in NH pools in soil, and increasing soil acidity (Fenn et al., 1996, 2003a, b). The decrease in NH pools may result from increases in net N mineralization and nitrification caused by N deposition, with the N cycle being NO-dominated (Risch et al., 2019; Krichels et al., 2022). Further, N may be lost as nitric oxide emissions, which have been found in semi-arid or arid ecosystems to be primarily re-emissions from atmospherically deposited N as a result of excess N deposition, especially following rewetting (Homyak et al., 2016).
Instead of being stored in soils or lost from the system, additional N in dryland ecosystems may be taken up by organisms including soil microbes for their metabolic functions, particularly during periods of higher water availability. Drylands are known for pulse-driven nutrient cycling, where biological activity and ecosystem processes are predominantly driven by precipitation and the lags between water pulses (Ogle and Reynolds, 2004; Schwinning and Sala, 2004; Collins et al., 2014). Studies, however, show a high variability in microbial responses to increased N deposition. On the one hand, small increases in N inputs can alleviate the limitation in the microbial pool, increasing soil organic matter (SOM) decomposition (Allison et al., 2009). In contrast, chronic N enrichment leads to altered microbial community composition (Chen et al., 2015; Widdig et al., 2020), a reduction of microbial biomass, and slower SOM decomposition (Frey et al., 2004; Forstner et al., 2019). These factors pose potential consequences for ecosystem nutrient cycling and climate regulation. Unfortunately, predictive models of ecosystem responses to N enrichment for other geographic regions are often not applicable to the semi-arid ecosystems of the southwestern US (Fenn et al., 2003a).
The plant community, in competition with soil microbes, may also be taking up added N. Although secondary to water limitations, desert plants often exhibit N deficiency (Peterjohn and Schlesinger, 1990; Hooper and Johnson, 1999; Elser et al., 2007; LeBauer and Treseder, 2008; Hall et al., 2011). Added N can stimulate plant growth (Báez et al., 2007) and increase biomass (Zeng et al., 2010) but can also cause significant losses in plant biodiversity (e.g., Sala et al., 2000; Fay et al., 2015; Midolo et al., 2019), with nitrophilic (Bobbink et al., 1988) and invasive (Brooks, 2003) plants often outcompeting native species (Tilman, 1987). Additionally, accumulation of N in the soil can increase soil acidification, which, in turn, can result in the loss of cations essential to plants (e.g., calcium) (Johnson et al., 1994) and increase the availability of toxic soil metals, such as aluminum (Lindsay and Walthall, 1995), further affecting productivity. These responses, however, seem to be highly variable within dryland ecosystems. While some studies report significant increases in net primary production (Luo et al., 2017), other studies suggest that plant species richness, foliar N concentrations, and percent cover exhibited limited to no response to increased N (McHugh et al., 2017). These inconsistencies highlight the need for further research to understand how chronic addition of high levels of N affects the nutrient dynamics of semi-arid ecosystems.
To study the impacts of long-term increases in N availability on the ecosystem N budget, we sampled soils from a long-term fertilization experiment established in 1995 at the Sevilleta National Wildlife Refuge (SNWR), central New Mexico, USA. We examined soil, microbial, and plant N pools to determine the allocation of additional N in response to 26 years of N fertilization in a native northern Chihuahuan Desert plant community across the growing season. We hypothesized that (1) fertilized plots would exhibit higher N content in all pools relative to controls and (2) that seasonal variation would play a bigger role in measured N than treatment.
2.1 Study site
The experiment was conducted at the Sevilleta National Wildlife Refuge (SNWR) located approximately 90 km south of Albuquerque, New Mexico, USA. Yearly temperatures range from 1.6 °C in January to 33.4 °C in June (Collins et al., 2008). This region receives an average of 234 mm of annual precipitation, with the majority falling as large monsoon events occurring from July to September (Gosz et al., 1995; Pennington and Collins, 2007). The SNWR is composed of several ecoregions, including the northernmost extent of Chihuahuan Desert grassland that intergrades into shortgrass steppe to the north and east of the refuge (Ladwig et al., 2012). This research was conducted in a transition zone between these two ecoregions, containing a mixture of dominant grasses, such as black grama grass (Bouteloua eriopoda), blue grama grass (Bouteloua gracilis), and other perennial C4 grasses. The soil at the research site is a Typic Calciorthid sandy loam (Johnson et al., 2003).
2.2 Experimental design
A total of 20 10 m × 5 m plots (10 control and 10 treatments) were established in 1995 to examine the impacts of N enrichment on aboveground and belowground processes (Johnson et al., 2003). Every year since their establishment, the 10 treatment plots have received 10 g N m−2 as NH4NO3 each June prior to the monsoon season to reflect the potential rates of atmospheric deposition near urban areas in the southwestern US; the remaining 10 have served as ambient controls (Fenn et al., 2003b; Ladwig et al., 2012). In June 2003, a management fire was allowed to burn through all the plots. Since fires are considered a natural part of this ecosystem and belowground processes in the area have been shown to be highly tolerant to fire (Brown and Collins, 2023; Vojdani et al., 2024), all 26 years of the experiment have been considered in this analysis.
2.3 Sampling protocol
Soils were collected on three sampling dates (Fig. 1): late June (pre-monsoon), early July (mid-monsoon), and early October 2020 (post-monsoon) after 26 years of N-addition treatments. At each plot, five soil cores were collected near the base of individual clumps of grass and/or other dominant plants when grasses were not present from 0–10 cm in depth using a 2.5 cm wide soil auger. Soil samples were homogenized, passed through a 2 mm sieve, and divided into subsamples for respective analyses. Additionally, following fertilizer application in late June, three soil samples were collected as described above, this time using a 15 cm diameter bucket auger at a depth of 20 cm to quantify total root standing crop biomass. In early October, leaf tissue samples from Mesa dropseed (Sporobolus flexuosus), the only grass common in all treatment and control plots, were harvested from each plot. Native to western North America, Mesa dropseed is a perennial grass that readily reproduces from seed and is an important source of forage in the region (Gibbens, 1991; Paulsen and Ares, 1962). Leaf tissue samples were dried, ground, and analyzed for % C and % N as described below.
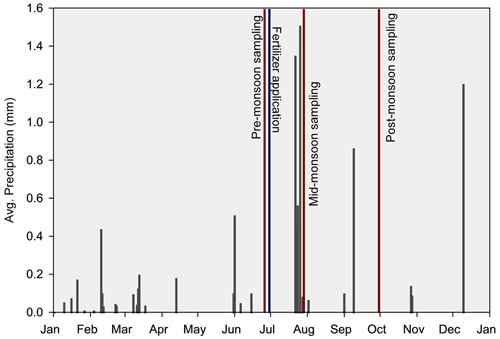
Figure 1Average daily precipitation (black bars), date of fertilizer application (blue line), and sampling dates (red lines) for the year 2020 in a 26-year southwestern US grassland fertilization experiment. Total yearly precipitation amount: 126.87 mm. Created using meteorological data from the Sevilleta National Wildlife Refuge, New Mexico (Moore and Hall, 2023).
2.4 Soil pH, salinity, and organic matter content
Soil pH and salinity were measured by combining a 15 g subsample of soil with 30 mL of deionized water, stirring, and leaving to rest for 30 min before measuring pH and salinity using a monitoring sonde. Soil salinity was recorded as a specific conductance (SPC) value. SOM was measured using the loss-of-ignition method (Rather, 1918). A subsample of 5 g of soil was dried at 60 °C for 48 h. Once dry, the sample was combusted in a muffle furnace at 375–450 °C for 16 h. The % SOM was calculated as the mass lost by ignition divided by the pre-ignition soil mass following Brown et al. (2022).
2.5 Soil N pools
One 5 g subsample from each plot was oven-dried at 65 °C for 48 h to calculate its soil to water ratio. For % C and % N, a subsample was dried, ground, cleared of carbonates using acid fumigation (Harris et al., 2001), and combusted to determine % C and % N using a dry combustion C and N analyzer (ElementarPyroCube, Ronkonkoma, NY, USA). A 5 g soil subsample per plot was extracted with 0.5 M K2SO4 for 2 h and vacuum-filtered through glass filter paper. Extracts were frozen at −20 °C until analyzed for nitrate (NO-N) and ammonium (NH-N) content, extractable organic C, and extractable total N (EOC and ETN, respectively). Both NO-N and NH-N were analyzed using colorimetric microplate assays (BioTEK Synergy HT microplate reader; BioTek Instruments Inc., Winooski, VT, USA). Soil NO-N was analyzed using a modified Griess reaction (Doane and Horwath, 2003) and NH-N using the Berlethot reaction protocol (Rhine et al., 1998). Soil EOC and ETN concentrations were determined in a minimum 10× dilution of the original extract using a Shimadzu EOC/TN analyzer with an autosampler (TOC-L CPH/CPN; Shimadzu Scientific Instruments Inc., Columbia, MD, USA). Extractable organic N (EON) was calculated as the difference between ETN and the sum of inorganic pools (NO-N and NH-N).
2.6 Microbial pools
Microbial biomass N (MBN) and C (MBC) were quantified using a modification of the chloroform fumigation–extraction technique (Brookes et al., 1985). One 5 g subsample of fresh soil was combined with 2 mL of ethanol-free chloroform and incubated at room temperature under a fume hood for 24 h in a stoppered 250 mL Erlenmeyer flask. Following incubation, flasks were vented and extracted with 0.5 M K2SO4 following the same procedure described in the previous section. Fumigated samples were analyzed for EOC and ETN; MBN and MBC were calculated as the difference between ETN and EOC concentrations extracted from fumigated and non-fumigated samples.
2.7 Species composition, aboveground biomass, and belowground biomass
Effects of treatments on plant species composition were outside of the scope of this experiment, but an earlier study on this experiment (Ladwig et al., 2012) includes information on species composition in these plots. During peak spring and fall growing seasons of each year, vegetation within four 1 m2 subplots in each treatment and control plot is measured, and the biomass of each species is determined allometrically from cover and height size classes. We used the aboveground biomass data collected in 2020 for this analysis (Baur et al., 2021).
To determine root biomass, triplicate soil cores described above were combined and homogenized before measuring total soil volume using a graduated cylinder. Roots were collected by sieving the samples with a 2 mm sieve and hand-picking roots with forceps. Extracted roots were then washed free of soil and dried at 65 °C for 48 h before mass was determined. Root biomass (mg cm−3) was calculated as root biomass per unit soil volume.
2.8 Calculated N content
Using the determined N concentration in each pool above, we estimated the N content per square meter to our sampling depth of 10 cm. All pools were estimated based on the October measurement, as this was the only sampling for which plant N was measured. Bulk density did not vary between treatments ( p=0.24), and we used the average bulk density of all plots (1.31 g cm−3) to determine N content in fertilized and unfertilized plots separately. The difference between these values was calculated by subtracting N of control plots from N of fertilized plots. N content in the plant pools is only a rough estimate, as we only measured leaf N concentration for a single species and did not measure root N concentration. Using just the N concentration of the measured species in each control and fertilized plot and the fall aboveground biomass values, we estimated the N content of the aboveground plants and then calculated a difference as above. For the root N content, we also used the N content of the aboveground tissue (this will be an overestimate) and calculated the N pool for the volume within the top 10 cm of soil using our root biomass measures.
2.9 Statistical analyses
Data underwent log10 transformation to achieve a higher approximation to normality for statistical analysis; data presented in figures are untransformed. Normality was tested using the Shapiro–Wilk test (p>0.05) in R. Soil N pools were analyzed as a two-way analysis of variance (ANOVA) using the “aov” function, with the factors of “treatment” and “sampling date”. All ANOVA assumptions were met, and sample sizes were equal between treatments. Statistical significance was determined at α<0.05. When statistical differences or interactions between factors were detected, Tukey tests were performed to find differences between specific groups using the “stats” R package (R Core Team, 2021). All analyses were performed using R 4.0.3 (R Core Team, 2021).
3.1 Soil pH, salinity, and organic matter content
We found that long-term N addition had few effects on the measured physical soil properties. Soils from both control and fertilized plots had similar pH (8.79 and 8.73, respectively) and soil organic matter content (1.05 % and 0.97 %, respectively), although fertilized plots had a higher salinity (106.51 µS cm−1 SPC, SE = 3.25) than control plots (93.00 µS cm−1 SPC, SE = 4.40) (Table 1).
3.2 Soil N pools
Soil total % N significantly increased with N addition and varied across the season (Fig. 3f, Table 1), while soil total % C showed no response to either treatment or sampling date (Fig. 3e, Table 1). There were no interactions between treatment and sampling date for either soil total % C or % N (Table 1). We found a strong effect of fertilization treatment on soil-available N, including higher NO-N (Fig. 2a, Table 1) and NH-N (Fig. 2b, Table 1) in fertilized plots. Both NO-N and NH-N also showed significant seasonal variation (Fig. 2, Table 1), and there was no interaction between sampling month and fertilization treatment for either variable (Table 1). EOC and ETN both showed significant increases with N addition and responses to sampling date with no interaction between the two (Fig. 3a, b, Table 1). However, neither MBC (Fig. 3c) nor MBN (Fig. 3d) responded to N treatment but did show significant seasonal variation (Table 1).
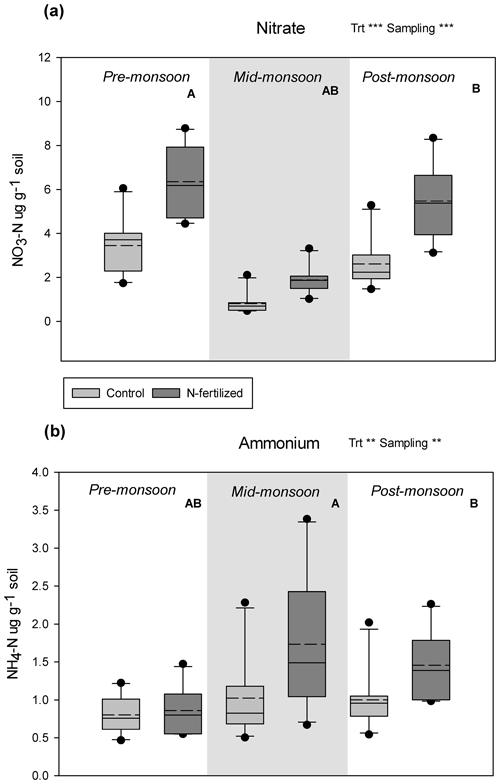
Figure 2Box plot, including the mean (dashed line, n=10) and extreme values (black dots) of inorganic N pools: soil nitrate (a) and soil ammonium (b) in a 26-year southwestern US grassland fertilization experiment. Overall significance for a two-way ANOVA with the main factors of treatment (Trt) and sampling date (Sampling) is shown (* p<0.05, p<0.01, p<0.001). Letters (A and B) indicate statistical difference between individual sampling dates (p<0.05). Original data are shown in the figure but statistics were performed for log-transformed data.
3.3 Aboveground and belowground biomass
We found no effects of fertilization on any of the plant variables measured. Aboveground biomass did not respond to fertilization treatments in the spring (, p=0.73), in the fall (, p=0.33), or as annual biomass measures (, p=0.26) (Baur et al., 2021). Similarly, neither plant % C (control 45.20 %; fertilized 45.12 %) nor % N (control 1.32 %; fertilized 1.44 %) showed responses to the N-addition treatment (Table 1). Root standing crop biomass (control 0.71 mg cm−3, fertilized 0.65 mg cm−3), as an estimate for belowground productivity, did not show a significant response to fertilization (, p=0.46).
Table 1Summary of two-way ANOVA for nutrient pools and soil properties across one growing season in a grassland fertilization experiment after 26 years (est. 1995) of annual N addition in the form of NH4NO3. Significant values (p<0.05) are in bold. df for all soil nutrient pool responses: treatment effect (1, 54), sampling date (2, 54), and the interaction of treatment and sampling date (1, 18).
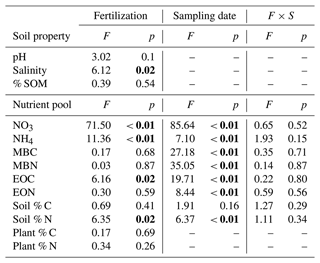
3.4 Calculated N content
In most cases the difference in N content between fertilized and unfertilized plots resulted in a positive number, indicating an accumulation of N in fertilized plots (Table 2). However, using our estimated N content of the aboveground plants, the difference between fertilized and non-fertilized plots was negative, indicating a negative effect of fertilization on plant N content (Table 2). We estimated no difference in the root N pool for the volume within the top 10 cm of soil (Table 2).
Table 2Summary of N pools in soil and plants during a single post-monsoon sampling period in a grassland fertilization experiment after 26 years (est. 1995) of yearly N addition in the form of NH4NO3. N in µg g−1 soil was measured on soil collected from the field and then converted to g N g−1 soil using the soil bulk density (1.31 g cm−3). Plant aboveground N pools were estimated using % N of a single species (Sporobolus flexuosus) collected from fertilized and unfertilized plots and calculated aboveground biomass. Belowground N pools were estimated using the measured aboveground N concentrations and measured belowground biomass to a 10 cm depth.
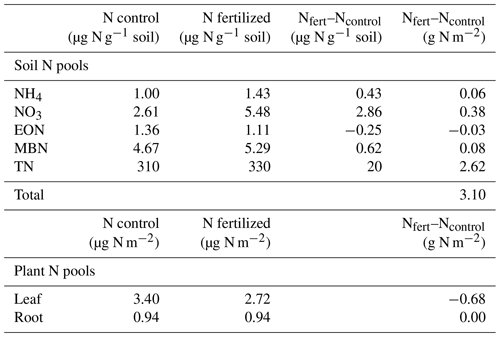
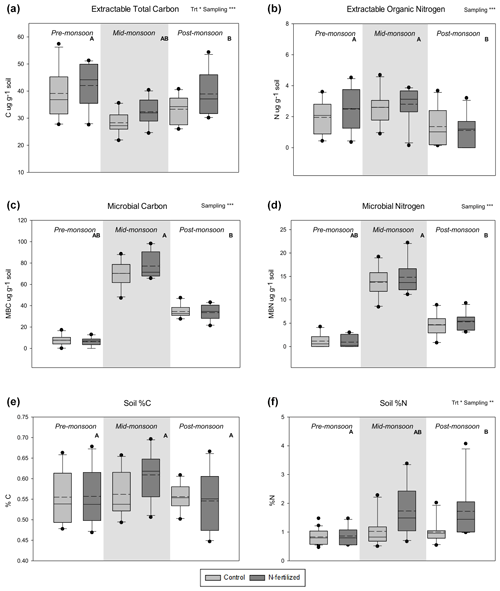
Figure 3Distribution of data based on a five-number summary – and mean (dashed line) – of different analyzed C and N pools: (a) extractable organic C, (b) extractable organic N, (c) microbial C, (d) microbial N, (e) soil percent C, and (f) soil percent N in a long-term fertilization experiment in the northern Chihuahuan Desert. Levels of overall significance for a two-way ANOVA with the main factors of treatment (Trt) and sampling date (Sampling) are shown (* p<0.05, p<0.01, p<0.001). Letters (A and B) indicate statistical difference between individual sampling dates (p<0.05). Original data are shown in the figure but statistics were performed for log-transformed data.
We examined the effects of increased N inputs on plant, soil, and microbial N pools in a Chihuahuan Desert grassland that has received annual additions of N for 26 years. We found small but significant increases with fertilization in most soil N pools, including available N (NO-N and NH-N) (Fig. 2) and soil total N (Soil % N, Fig. 3f), but not extractable organic N (EON, Fig. 3b). However, this did not translate to higher N pools within organisms in this system. Neither soil microbial C nor N significantly increased with fertilization (Fig. 3c, d), and there was no effect on aboveground or belowground plant biomass or N content in plant leaf tissue of a common grass, Sporobolus flexuosus. Thus, despite significant inputs of N into this system (260 g m−2 of N) there were only small increases in soil pools, and the soil microbes and plant communities do not appear to be taking advantage of increased N availability.
The N added to these plots did translate to increased inorganic N in the upper soil layers of fertilized plots. Nitrate and ammonium are the primary sources of N for both soil microbes and plants (Harrison et al., 2007), suggesting that annual fertilization increased N availability to these organisms. Using some back-of-the envelope calculations, we determined that the additional inorganic N available in these plots is less than 0.5 g m−2 (Table 2), which is less than 5 % of the 260 g added over the lifetime of the experiment. Our data are consistent with prior research demonstrating that semi-arid ecosystems have low N retention (Peterjohn and Schlesinger, 1990; McCalley and Sparks, 2009). For instance, 7 years of simulated N deposition at three semi-arid grasslands resulted in rapid but ephemeral changes in soil inorganic N (Phillips et al., 2021), suggesting that the additional N quickly left the system.
Despite the additional inorganic N added to the ecosystem, the vegetation did not detectably respond to fertilization with increased aboveground or belowground biomass or increased N content within that biomass. Although not significant, the average aboveground plant biomass was actually higher in control (256.8 g m−2) than fertilized plots (188.4 g m−2). We found similar % N in the leaf tissue of one common grass species; therefore, N content in aboveground tissue could not account for any of the additional N added to the fertilized plots. Belowground biomass in control (0.71 mg cm−3) and fertilized plots (0.65 mg cm−3) is nearly identical, and if we assume that the % N in the root tissue is similar between the two treatments, as it is for the aboveground tissue, then this would also not account for any of the “missing N”. Other semi-arid ecosystem N-addition experiments on the Colorado Plateau (Phillips et al., 2021) also reported no plant response to fertilization. Although measurements of fertilization effects on plant composition were outside the scope of this experiment, previous studies on these plots detected no plant response to fertilization except ephemeral increases in years with above-average precipitation (Ladwig et al., 2012), further supporting the claim that N limitation in semi-arid ecosystems is less prominent than water stress (Carpenter et al., 1990; Hooper and Johnson, 1999; Brooks, 2003; Rao and Allen, 2010; Rao et al., 2011; Wang and Wen, 2023; Brown and Collins, 2024). Indeed, water stress may actually lead to further nutrient stress – the mobility of free ions in the soil is highly dependent on water status, particularly NO3 (Plett et al., 2020). Moreover, a meta-analysis of dryland N-addition studies by Hooper and Johnson (1999) concluded that geographical and temporal variation in precipitation significantly increased absolute plant production and ecosystem resource use efficiency, emphasizing plant co-limitation by multiple resources and the tight link between species shifts, changing resource levels, and water availability.
Similar to the plant response, belowground microbial communities did not accumulate biomass C (MBC) nor N (MBN) in response to fertilization treatments. In contrast with these findings, a meta-analysis of 14 studies on N deposition in drylands by Sinsabaugh et al. (2015) showed soil microbial communities to be sensitive to even low rates of N deposition, with positive microbial biomass and metabolic responses. However, Sinsabaugh et al. (2015) described various shortcomings of their interpretations of soil microbial responses: dryland landscapes are highly heterogeneous, and N-addition studies are scarce and differ in doses and duration. Nevertheless, individual studies have reported that the composition and function of dryland microbiota are unresponsive to N additions such as the 2-year N-deposition experiment in semi-arid grasslands reported by McHugh et al. (2017) or even negative after 5 years of N addition (Li et al., 2010), suggesting contingent responses to similar N inputs across dryland ecosystems. Like plants, the unresponsiveness of the microbial community to additional N inputs may be attributed to a possible co-limitation by another resource, especially water or carbon. In a study in this same ecosystem, Brown et al. (2022) found that while nitrogen availability generally increases over the growing season, it especially increases following small rain events which stimulate microbial extracellular enzyme activities. In these ecosystems with low organic content in soils, soil microbes are often limited by C rather than N (Garcia et al., 1994) and N immobilization is hindered by the lack of available C to build biomass. Other nutrients which are also low in these soils with little organic matter, such as phosphorus, potassium, or even micronutrients, may also hinders the biota's capacity to take advantage of additional N (Ramirez et al., 2010; Fay et al., 2015; Radujković et al., 2021; Choi et al., 2022). We did not measure microbial efforts to acquire C, N, or P via extracellular enzymes and can only speculate on the potential limitation of soil microbes by other nutrients. Because there was no change in either MBC or MBN, there was also consequently no change after fertilization in microbial stoichiometry, which can sometimes be used to suggest microbial limitation by specific resources (Sinsabaugh and Shah, 2012).
Classic N-saturation models suggest that in an N-limited system, N added to the system will move from vegetation, to litter, to soil, and, given that enough N is added to the system, to N losses typically through leaching losses but potentially through denitrification and ammonia volatilization (Aber et al., 1998). Under this model, given the lack of accumulation in either plant or soil microbial N pools, this system could be considered N-saturated. However, N saturation models likely need to be modified for arid ecosystems (Lovett and Goodale, 2011; Homyak et al., 2014) where there may be asynchrony between N availability or deposition and plant demand (Ochoa-Hueso et al., 2011). For example, in this experiment the fertilization treatment is applied at the beginning of the monsoon season before peak periods of plant growth. In these arid systems, where biological activity is highly sensitive to fluctuations in water, N budgets may suggest N saturation despite the ecosystem remaining N-limited. Models developed explicitly for arid lands suggest that N saturation occurs as a simultaneous flow of N to four possible destinations: vegetation, detritus and SOM, leaching, or gaseous loss (Lovett and Goodale, 2011). Homyak et al. (2014) further developed this model to include C limitation by microbial communities resulting in low N immobilization and the asynchrony between N addition and plant N demands as addition factors which would determine the quantity of N lost through leaching or gaseous N loss (Homyak et al., 2014).
We suggest that although the dryland ecosystem in this study may become N-limited during periods of high rainfall or soil moisture, because the N added to this experiment happened before peak plant growth, it is likely being lost from the system through leaching during large rain events or volatilization when soils are hot and dry (McCalley and Sparks, 2009; Brown et al., 2022). Similar to our results, 8 years of simulated increases in N deposition in the Colorado Plateau led to some short-term but no long-lasting effects on an array of soil, plant, and microbial metrics, suggesting that serial or simultaneous limitation of multiple other resources may weaken the direct effects of higher N levels on ecosystem processes in drylands (Osborne et al., 2022). Given the limited amount of yearly precipitation received by our site, we suspected that the likelihood of rainfall percolating deep enough to cause nutrient leaching would be low. However, McHugh et al. (2017) reported resin capture results showing that N was moving down through the soil profile in a semi-arid grassland on the Colorado Plateau with similar annual precipitation, and nitrate in the subsoil of numerous arid regions indicates that leaching may indeed be common (Walvoord et al., 2003). Alternatively, N may have been lost from the system via volatilization, which has been described as a dominant mechanism for N loss from ecosystems of the desert southwest (Peterjohn and Schlesinger, 1990) and supported in several recent studies (Homyak et al., 2016; McHugh et al., 2017). In fact, rather than water stimulating periods of high plant N uptake as we predict, water pulses may actually stimulate gaseous N losses by denitrification in addition to higher N leaching (Yahdjian and Sala, 2010).
Even though we did not detect an increase in N accumulation by the plant or microbial community with fertilization, we still found an accumulation of organic N in the fertilized plot soils, with the majority of this found in the total N pool rather than extractable organic N. Despite a relatively small difference in the soil % N between control (0.031 %) and fertilized (0.033 %) plots, when accounting for the volume and mass of soil found in the top 10 cm of the soil, this would be an additional 2.6 g m−2 of N (Table 2) found in fertilized plots. Because the N added each year is in an inorganic form (NH4NO3), this increase in organic or total N over time suggests that the N was converted to an organic form (through uptake and assimilation of N into microbial and plant biomass, as observed by Harrison et al. 2007) and then deposited into the soil. This is supported by short-term studies which have reported only increases in plant N but no increases in soil N after 5 (Zeng et al., 2010) or 8 (Osborne et al., 2022) years of N addition, although other experiments with similar amounts of N added for 6 years were sufficient to increase total soil N content (Sha et al., 2021). Thus, over the duration of the experiment there were at least some years where there was higher plant or microbial biomass produced in the fertilized plots, such as when production increases during high precipitation years (Hall et al., 2011; Ladwig et al., 2012).
In agreement with our second hypothesis, the effect of seasonal variability on N pools was higher than differences between fertilization and control treatments. Sampling date effects were significant for every variable that was measured multiple times, except for soil % C, which is unlikely to change much seasonally but can be quite variable from year to year in response to precipitation variability (Hou et al., 2021). In general, drylands inherently have high temporal heterogeneity in rainfall, where large, infrequent precipitation events are often thought to regulate ecosystem structure and function (Noy-Meir, 1973), including fluctuations in C and nutrient availability (Collins et al., 2014; Schimel, 2018). In particular, NO and NH are known to show high intra-annual seasonality in many ecosystems, including drylands where N availability is often quite low to begin with (Zak et al., 1994) and linked to the timing of rainfall (Cui and Caldwell, 1997; Dijkstra et al., 2012; Brown et al., 2022). Surprisingly, the effect of fertilization did not vary between sampling dates for any of the variables, even when including dates both before and after fertilization. Shorter-term N-addition studies (McHugh et al., 2017; Phillips et al., 2021) have reported only transient increases immediately after fertilizer addition. Yet in this study these available forms of N still showed persistent, likely cumulative, effects a year after fertilization, consistent with limited uptake of available nutrients by microbial and plant communities. Persistent effects on variables such as total soil N are less unexpected because they typically vary little over the short term. Finally, this study only took place within a single year, but we would also expect high year-to-year variability in available resources and N pools. Fertilization in this experiment is timed to occur close to the beginning of monsoon rains, although because of the unpredictability of the rains, the time between fertilizer application and the monsoon shifts each year. Timing of N addition relative to precipitation can alter the ultimate effects of fertilizer addition in drylands (Stursova et al., 2006).
Ultimately, between the 0.5 g m−2 of additional inorganic N found in fertilized plots and the 2.6 g m−2 found in the soil total N pool, much of the additional 260 g m−2 of N added over the lifetime of the experiment remains unaccounted for. Thus, a significant portion of the added N has been lost from the system. While the route by which N left the system is unknown because N was not immobilized by plants or microbes, we can infer that the N has either reached into deeper soil profiles or was lost via gaseous losses, with the route of loss likely depending on time of year and timing of precipitation. We encourage studies with variation in both the application of nitrogen and the timing of measurements to assess potential for seasonal variation in the N-limitation status of this ecosystem. Together our results demonstrate that these nutrient-poor ecosystems have limited capacity to retain N even under high rates of N deposition.
The datasets generated during the current study are available on the EDI Data Portal (https://doi.org/10.6073/pasta/, Mendoza-Martinez et al., 2024).
All authors contributed to study conceptualization and methodology. Investigation was performed by VMM, with supervision by JRM and SLC. All authors participated in formal analyses. The writing (original draft preparation) was completed by VMM, and all authors participated in the writing (review and editing) process.
The contact author has declared that none of the authors has any competing interests.
Publisher's note: Copernicus Publications remains neutral with regard to jurisdictional claims made in the text, published maps, institutional affiliations, or any other geographical representation in this paper. While Copernicus Publications makes every effort to include appropriate place names, the final responsibility lies with the authors.
This article is part of the special issue “Ecosystem experiments as a window to future carbon, water, and nutrient cycling in terrestrial ecosystems”. It is not associated with a conference.
We thank the Sevilleta National Wildlife Refuge and US Fish and Wildlife Services for permitting this experiment, as well as the Sevilleta Long-term Ecological Research interns, field crew, and staff for its maintenance and continuation. Funding for Violeta Mendoza-Martinez was provided by University of Texas at El Paso (UTEP) through the Biology Undergraduate Research Scholars Program and the NSF through the ACSScellence Scholar Program at UTEP.
This research has been supported by the National Science Foundation (grant nos. DEB-1655499 and DBI-1950237).
This paper was edited by Karin Rebel and reviewed by Kristina Young and one anonymous referee.
Aber, J. D., McDowell, W., Nadelhoffer, K., Magill, A., Berntson, G., McNulty, S., Currie, W., Rustad, L., and Fernandez, I.: Forest Ecosystems Hypotheses revisited, Bioscience, 48, 921–934, 1998.
Adeel, Z. and Safriel, U.: Ecosystems and human well-being: Desertification Synthesis., 36 pp., ISBN 1-56973-590-5, 2005.
Ahlström, A., Raupach, M. R., Schurgers, G., Smith, B., Arneth, A., Jung, M., Reichstein, M., Canadell, J. G., Friedlingstein, P., Jain, A. K., Kato, E., Poulter, B., Sitch, S., Stocker, B. D., Viovy, N., Wang, Y. P., Wiltshire, A., Zaehle, S., and Zeng, N.: The dominant role of semi-arid ecosystems in the trend and variability of the land CO2 sink, Science, 348, 895–899, https://doi.org/10.1126/science.aaa1668, 2015.
Allison, S. D., LeBauer, D. S., Ofrecio, M. R., Reyes, R., Ta, A. M., and Tran, T. M.: Low levels of nitrogen addition stimulate decomposition by boreal forest fungi, Soil Biol. Biochem., 41, 293–302, https://doi.org/10.1016/j.soilbio.2008.10.032, 2009.
Báez, S., Fargione, J., Moore, D. I., Collins, S. L., and Gosz, J. R.: Atmospheric nitrogen deposition in the northern Chihuahuan desert: Temporal trends and potential consequences, J. Arid Environ., 68, 640–651, https://doi.org/10.1016/j.jaridenv.2006.06.011, 2007.
Baur, L., Collins, S. L., Muldavin, E., Rudgers, J. A., and Pockman, W. T.: Nitrogen Fertilization Experiment (NFert): Seasonal Biomass and Seasonal and Annual NPP Data at the Sevilleta National Wildlife Refuge, New Mexico ver 208430, Environmental Data Initiative [data set], https://doi.org/10.6073/pasta/01a70b97fec7b2b2f744c6e254ed9b97, 2021.
Bobbink, R., Bik, L., and Willems, J. H.: Effects of nitrogen fertilization on vegetation structure and dominance of Brachypodium pinnatum (L.) Beauv. in chalk grassland, Acta Bot. Neerl., 37, 231–242, https://doi.org/10.1111/j.1438-8677.1988.tb02132.x, 1988.
Brookes, P. C., Landman, A., Pruden, G., and Jenkinson, D. S.: Chloroform fumigation and the release of soil nitrogen: A rapid direct extraction method to measure microbial biomass nitrogen in soil, Soil Biol. Biochem., 17, 837–842, https://doi.org/10.1016/0038-0717(85)90144-0, 1985.
Brooks, M. L.: Effects of increased soil nitrogen on the dominance of alien annual plants in the Mojave Desert, J. Appl. Ecol., 40, 344–353, https://doi.org/10.1046/j.1365-2664.2003.00789.x, 2003.
Brown, R. F., Sala, O. E., Sinsabaugh, R. L., and Collins, S. L.: Temporal Effects of Monsoon Rainfall Pulses on Plant Available Nitrogen in a Chihuahuan Desert Grassland, J. Geophys. Res.-Biogeo., 127, e2022JG006938, https://doi.org/10.1029/2022JG006938, 2022.
Brown, R. F. and Collins S. L.: As above, not so below: Long-term dynamics of net primary production across a dryland transition zone, Glob. Change Biol., 29, 3941–3953, https://doi.org/10.1111/gcb.16744, 2023.
Brown, R. F. and Collins, S. L.: Revisiting the bucket model: Long-term effects of rainfall variability and nitrogen enrichment on net primary production in a desert grassland, J. Ecol., 112, 629–641, https://doi.org/10.1111/1365-2745.14258, 2024.
Carpenter, A. T., Moore, J. C., Redente, E. F., and Stark, J. C.: Plant community dynamics in a semi-arid ecosystem in relation to nutrient addition following a major disturbance, Plant Soil, 126, 91–99, https://doi.org/10.1007/BF00041373, 1990.
Chalcraft, D. R., Cox, S. B., Clark, C., Cleland, E. E., Suding, K. N., Weiher, E., and Pennington, D.: Scale-dependent responses of plant biodiversity to nitrogen enrichment, Ecology, 89, 2165–2171, https://doi.org/10.1890/07-0971.1, 2008.
Chen, D., Lan, Z., Hu, S., and Bai, Y.: Effects of nitrogen enrichment on belowground communities in grassland: Relative role of soil nitrogen availability vs. soil acidification, Soil Biol. Biochem., 89, 99–108, https://doi.org/10.1016/j.soilbio.2015.06.028, 2015.
Choi, R. T., Reed, S. C., and Tucker, C. L.: Multiple resource limitation of dryland soil microbial carbon cycling on the Colorado Plateau, Ecology, 103, 1–17, https://doi.org/10.1002/ecy.3671, 2022.
Collins, S. L., Belnap, J., Grimm, N. B., Rudgers, J. A., Dahm, C. N., D'Odorico, P., Litvak, M., Natvig, D. O., Peters, D. C., Pockman, W. T., Sinsabaugh, R. L., and Wolf, B. O.: A multiscale, hierarchical model of pulse dynamics in arid-land ecosystems, Annu. Rev. Ecol. Evol. Syst., 45, 397–419, https://doi.org/10.1146/annurev-ecolsys-120213-091650, 2014.
Collins, S. L., Sinsabaugh, R. L., Crenshaw, C., Green, L., Porras-Alfaro, A., Stursova, M., and Zeglin, L. H.: Pulse dynamics and microbial processes in aridland ecosystems, J. Ecol., 96, 413–420, https://doi.org/10.1111/j.1365-2745.2008.01362.x, 2008.
Cui, M. and Caldwell, M. M.: A large ephemeral release of nitrogen upon wetting of dry soil and corresponding root responses in the field, Plant Soil, 191, 291–299, https://doi.org/10.1023/A:1004290705961, 1997.
Dijkstra, F. A., Augustine, D. J., Brewer, P., Fischer, J. C. Von, Brewer, P., and Fischer, J. C. Von: Nitrogen cycling and water pulses in semiarid grasslands: are microbial and plant processes temporally asynchronous?, Oecologia, 170, 799–808, 2012.
Doane, T. A. and Horwáth, W. R.: Spectrophotometric Determination of Nitrate with a Single Reagent, Anal. Lett., 36, 2713–2722, https://doi.org/10.1081/AL-120024647, 2003.
Elser, J. J., Bracken, M. E. S., Cleland, E. E., Gruner, D. S., Harpole, W. S., Hillebrand, H., Ngai, J. T., Seabloom, E. W., Shurin, J. B., and Smith, J. E.: Global analysis of nitrogen and phosphorus limitation of primary producers in freshwater, marine and terrestrial ecosystems, Ecol. Lett., 10, 1135–1142, https://doi.org/10.1111/j.1461-0248.2007.01113.x, 2007.
Fay, P. A., Prober, S. M., Harpole, W. S., Knops, J. M. H., Bakker, J. D., Borer, E. T., Lind, E. M., MacDougall, A. S., Seabloom, E. W., Wragg, P. D., Adler, P. B., Blumenthal, D. M., Buckley, Y. M., Chu, C., Cleland, E. E., Collins, S. L., Davies, K. F., Du, G., Feng, X., Firn, J., Gruner, D. S., Hagenah, N., Hautier, Y., Heckman, R. W., Jin, V. L., Kirkman, K. P., Klein, J., Ladwig, L. M., Li, Q., McCulley, R. L., Melbourne, B. A., Mitchell, C. E., Moore, J. L., Morgan, J. W., Risch, A. C., Schütz, M., Stevens, C. J., Wedin, D. A., and Yang, L. H.: Grassland productivity limited by multiple nutrients, Nat. Plants, 1, 1–5, https://doi.org/10.1038/nplants.2015.80, 2015.
Fenn, M. E., Baron, J. S., Allen, E. B., Rueth, H. M., Nydick, K. R., Geiser, L., Bowman, W. D., Sickman, J. O., Meixner, T., Johnson, D. W., and Neitlich, P.: Ecological effects of nitrogen deposition in the western United States, Bioscience, 53, 404–420, https://doi.org/10.1641/0006-3568(2003)053[0404:EEONDI]2.0.CO;2, 2003a.
Fenn, M. E., Haeuber, R., Tonnesen, G. S., Baron, J. S., Grossman-Clarke, S., Hope, D., Jaffe, D. A., Copeland, S., Geiser, L., Rueth, H. M., and Sickman, J. O.: Nitrogen emissions, deposition, and monitoring in the western United States, Bioscience, 53, 391–403, https://doi.org/10.1641/0006-3568(2003)053[0391:NEDAMI]2.0.CO;2, 2003b.
Fenn, M. E., Poth, M. A., and Johnson, D. W.: Evidence for nitrogen saturation in the San Bernardino Mountains in southern California, Forest Ecol. Manag., 82, 211–230, https://doi.org/10.1016/0378-1127(95)03668-7, 1996.
Forstner, S. J., Wechselberger, V., Müller, S., Keibinger, K. M., Díaz-Pinés, E., Wanek, W., Scheppi, P., Hagedorn, F., Gundersen, P., Tatzber, M., Gerzabek, M. H., and Zechmeister-Boltenstern, S.: Vertical Redistribution of Soil Organic Carbon Pools After Twenty Years of Nitrogen Addition in Two Temperate Coniferous Forests, Ecosystems, 22, 379–400, https://doi.org/10.1007/s10021-018-0275-8, 2019.
Frey, S. D., Knorr, M., Parrent, J. L., and Simpson, R. T.: Chronic nitrogen enrichment affects the structure and function of the soil microbial community in temperate hardwood and pine forests, Forest Ecol. Manag., 196, 159–171, https://doi.org/10.1016/j.foreco.2004.03.018, 2004.
Galloway, J. N., Townsend, A. R., Erisman, J. W., Bekunda, M., Cai, Z., Freney, J. R., Martinelli, L. A., Seitzinger, S. P., and Sutton, M. A.: Transformation of the Nitrogen Cycle, Science, 320, 889–892, 2008.
Garcia, C., Hernandez, T., and Costa, F.: Microbial activity in soils under mediterranean environmental conditions, Soil Biol. Biochem., 26, 1185–1191, https://doi.org/10.1016/0038-0717(94)90142-2, 1994.
Gibbens, R. P.: Some Effects of Precipitation Patterns on Mesa Dropseed Phenology, J. Range Manag., 44, 86–90, https://doi.org/10.2307/4002646, 1991.
Gosz, J. R., Moore, D. I., Shore, G. A., Grover, H. D., Rison, W., and Rison, C.: Lightning estimates of precipitation location and quantity on the Sevilleta LTER, New Mexico, Ecol. Appl., 5, 1141–1150, https://doi.org/10.2307/2269361, 1995.
Hall, S. J., Sponseller, R. A., Grimm, N. B., Huber, D., Kaye, J. P., Clark, C., and Collins, S. L.: Ecosystem response to nutrient enrichment across an urban airshed in the Sonoran Desert, Ecol. Appl., 21, 640–660, https://doi.org/10.1890/10-0758.1, 2011.
Harris, D., Horwáth, W. R., and van Kessel, C.: Acid fumigation of soils to remove carbonates prior to total organic carbon or CARBON-13 isotopic analysis, Soil Sci. Soc. Am. J., 65, 1853–1856, https://doi.org/10.2136/sssaj2001.1853, 2001.
Harrison, K. A., Bol, R., and Bardgett, R. D.: Preferences for different nitrogen forms by coexisting plant species and soil microbes, Ecology, 88, 989–999, https://doi.org/10.1890/06-1018, 2007.
Homyak, P. M., Sickman, J. O., Miller, A. E., Melack, J. M., Meixner, T., and Schimel, J. P.: Assessing Nitrogen-Saturation in a Seasonally Dry Chaparral Watershed: Limitations of Traditional Indicators of N-Saturation, Ecosystems, 17, 1286–1305, https://doi.org/10.1007/s10021-014-9792-2, 2014.
Homyak, P. M., Blankinshipa, J. C., Marchus, K., Lucero, D. M., Sickman, J. O., and Schimel, J. P.: Aridity and plant uptake interact to make dryland soils hotspots for nitric oxide (NO) emissions, P. Natl. Acad. Sci. USA, 113, E2608–E2616, https://doi.org/10.1073/pnas.1520496113, 2016.
Hooper, D. U. and Johnson, L.: Nitrogen limitation in dryland ecosystems: Responses to geographical and temporal variation in precipitation, Biogeochemistry, 46, 247–293, https://doi.org/10.1007/BF01007582, 1999.
Hou, E., Rudgers, J. A., Collins, S. L., Litvak, M. E., White, C. S., Moore, D. I., and Luo, Y.: Sensitivity of soil organic matter to climate and fire in a desert grassland, Biogeochemistry, 156, 59–74, https://doi.org/10.1007/s10533-020-00713-3, 2021.
Johnson, A. H., Andersen, S. B., and Siccama, T. G.: Acid rain and soils of the Adirondacks. I. Changes in pH and available calcium, 1930–1984, Can. J. Forest Res., 24, 39–45, 1994.
Johnson, N. C., Rowland, D. L., Corkidi, L., Egerton-Warburton, L. M., and Allen, E. B.: Nitrogen enrichment alters mycorrhizal allocation at five mesic to semiarid grasslands, Ecology, 84, 1895–1908, https://doi.org/10.1890/0012-9658(2003)084[1895:NEAMAA]2.0.CO;2, 2003.
Krichels, A. H., Homyak, P. M., Aronson, E. L., Sickman, J. O., Botthoff, J., Shulman, H., Piper, S., Andrews, H. M., and Jenerette, G. D.: Rapid nitrate reduction produces pulsed NO and N2O emissions following wetting of dryland soils, Biogeochemistry, 158, 233–250, https://doi.org/10.1007/s10533-022-00896-x, 2022.
Ladwig, L. M., Collins, S. L., Swann, A. L., Xia, Y., Allen, M. F., and Allen, E. B.: Above- and belowground responses to nitrogen addition in a Chihuahuan Desert grassland, Oecologia, 169, 177–185, https://doi.org/10.1007/s00442-011-2173-z, 2012.
LeBauer, D. S. and Treseder, K. K.: Nitrogen limitation of net primary productivity in terrestrial ecosystems is globally distributed, Ecology, 89, 371–379, https://doi.org/10.1890/06-2057.1, 2008.
Li, L. J., Zeng, D. H., Yu, Z. Y., Fan, Z. P., and Mao, R.: Soil microbial properties under N and P additions in a semi-arid, sandy grassland, Biol. Fertil. Soils, 46, 653–658, https://doi.org/10.1007/s00374-010-0463-y, 2010.
Lindsay, W. L. and Walthall, P.: The Solubility of Aluminum in Soils, in: The Environmental Chemistry of Aluminum, edited by: Sposito, G., CRC Press, 333–362, 1995.
Liu, L., Xu, W., Lu, X., Zhong, B., Guo, Y., Lu, X., Zhao, Y., He, W., Wang, S., Zhang, X., Liu, X., and Vitousek, P.: Exploring global changes in agricultural ammonia emissions and their contribution to nitrogen deposition since 1980, P. Natl. Acad. Sci. USA, 119, 1–9, https://doi.org/10.1073/pnas.2121998119, 2022.
Lovett, G. M. and Goodale, C. L.: A New Conceptual Model of Nitrogen Saturation Based on Experimental Nitrogen Addition to an Oak Forest, Ecosystems, 14, 615–631, https://doi.org/10.1007/s10021-011-9432-z, 2011.
Luo, Q., Gong, J., Yang, L., Li, X., Pan, Y., Liu, M., Zhai, Z., and Baoyin, T. tao: Impacts of nitrogen addition on the carbon balance in a temperate semiarid grassland ecosystem, Biol. Fertil. Soils, 53, 911–927, https://doi.org/10.1007/s00374-017-1233-x, 2017.
McCalley, C. K. and Sparks, J. P.: Abiotic Gas Formation Drives Nitrogen Loss from a Desert Ecosystem, Science, 326, 837–840, https://doi.org/10.1126/science.1178984, 2009.
McHugh, T. A., Morrissey, E. M., Mueller, R. C., Gallegos-Graves, L. V., Kuske, C. R., and Reed, S. C.: Bacterial, fungal, and plant communities exhibit no biomass or compositional response to two years of simulated nitrogen deposition in a semiarid grassland, Environ. Microbiol., 19, 1600–1611, https://doi.org/10.1111/1462-2920.13678, 2017.
Mendoza-Martinez, V., Collins, S. L. and McLaren, J. R.: Nitrogen budget in a Chihuahuan desert grassland long-term nitrogen fertilization experiment at the Sevilleta National Wildlife Refuge, Environmental Data Initiative [data set], https://doi.org/10.6073/pasta/, 2024.
Midolo, G., Alkemade, R., Schipper, A. M., Benítez-López, A., Perring, M. P., and De Vries, W.: Impacts of nitrogen addition on plant species richness and abundance: A global meta-analysis, Global Ecol. Biogeogr., 28, 398–413, https://doi.org/10.1111/geb.12856, 2019.
Moore, D. I. and Hall, K. M.: Meteorology Data from the Sevilleta National Wildlife Refuge, New Mexico version 16, Environmental Data Initiative [data set], https://doi.org/10.6073/pasta/decdaa, 2023.
Noy-Meir, I.: Desert Ecosystems: Environment and Producers, Annu. Rev. Ecol. Syst., 4, 25–51, https://doi.org/10.1146/annurev.es.04.110173.000325, 1973.
Ochoa-Hueso, R., Allen, E. B., Branquinho, C., Cruz, C., Dias, T., Fenn, M. E., Manrique, E., Pérez-Corona, M. E., Sheppard, L. J., and Stock, W. D.: Nitrogen deposition effects on Mediterranean-type ecosystems: An ecological assessment, Environ. Pollut., 159, 2265–2279, https://doi.org/10.1016/j.envpol.2010.12.019, 2011.
Ogle, K. and Reynolds, J. F.: Plant responses to precipitation in desert ecosystems: Integrating functional types, pulses, thresholds, and delays, Oecologia, 141, 282–294, https://doi.org/10.1007/s00442-004-1507-5, 2004.
Osborne, B. B., Roybal, C. M., Reibold, R., Collier, C. D., Geiger, E., Phillips, M. L., Weintraub, M. N., and Reed, S. C.: Biogeochemical and ecosystem properties in three adjacent semi-arid grasslands are resistant to nitrogen deposition but sensitive to edaphic variability, J. Ecol., 110, 1615–1631, https://doi.org/10.1111/1365-2745.13896, 2022.
Paulsen Jr., H. A. and Ares, F. N.: Grazing values and management of black grama and tobosa grasslands and associated shrub ranges of the Southwest, USDA Forest Service, Tech. Bull., 1962.
Pennington, D. D. and Collins, S. L.: Response of an aridland ecosystem to interannual climate variability and prolonged drought, Landsc. Ecol., 22, 897–910, https://doi.org/10.1007/s10980-006-9071-5, 2007.
Peterjohn, W. T. and Schlesinger, W. H.: Nitrogen loss from deserts in the southwestern United States, Biogeochemistry, 10, 67–79, https://doi.org/10.1007/BF00000893, 1990.
Phillips, M. L., Winkler, D. E., Reibold, R. H., Osborne, B. B., and Reed, S. C.: Muted responses to chronic experimental nitrogen deposition on the Colorado Plateau, Oecologia, 195, 513–524, https://doi.org/10.1007/s00442-020-04841-3, 2021.
Plett, D. C., Ranathunge, K., Melino, V. J., Kuya, N., Uga, Y., and Kronzucker, H. J.: The intersection of nitrogen nutrition and water use in plants: New paths toward improved crop productivity, J. Exp. Bot., 71, 4452–4468, https://doi.org/10.1093/jxb/eraa049, 2020.
Poulter, B., Frank, D., Ciais, P., Myneni, R. B., Andela, N., Bi, J., Broquet, G., Canadell, J. G., Chevallier, F., Liu, Y. Y., Running, S. W., Sitch, S., and Van Der Werf, G. R.: Contribution of semi-arid ecosystems to interannual variability of the global carbon cycle, Nature, 509, 600–603, https://doi.org/10.1038/nature13376, 2014.
Püspök, J. F., Zhao, S., Calma, A. D., Vourlitis, G. L., Allison, S. D., Aronson, E. L., Schimel, J. P., Hanan, E. J., and Homyak, P. M.: Effects of experimental nitrogen deposition on soil organic carbon storage in Southern California drylands, Glob. Change Biol., 29, 1660–1679, https://doi.org/10.1111/gcb.16563, 2023.
Radujković, D., Verbruggen, E., Seabloom, E. W., Bahn, M., Biederman, L. A., Borer, E. T., Boughton, E. H., Catford, J. A., Campioli, M., Donohue, I., Ebeling, A., Eskelinen, A., Fay, P. A., Hansart, A., Knops, J. M. H., MacDougall, A. S., Ohlert, T., Olde Venterink, H., Raynaud, X., Risch, A. C., Roscher, C., Schütz, M., Silveira, M. L., Stevens, C. J., Van Sundert, K., Virtanen, R., Wardle, G. M., Wragg, P. D., and Vicca, S.: Soil properties as key predictors of global grassland production: Have we overlooked micronutrients?, Ecol. Lett., 24, 2713–2725, https://doi.org/10.1111/ele.13894, 2021.
Ramirez, K. S., Lauber, C. L., Knight, R., Bradford, M. A., and Fierer, N.: Consistent effects of nitrogen fertilization on soil bacterial communities in contrasting systems, Ecology, 91, 3463–3470, https://doi.org/10.1890/10-0426.1, 2010.
Rao, L. E. and Allen, E. B.: Combined effects of precipitation and nitrogen deposition on native and invasive winter annual production in California deserts, Oecologia, 162, 1035–1046, https://doi.org/10.1007/s00442-009-1516-5, 2010.
Rao, L. E., Steers, R. J., and Allen, E. B.: Effects of natural and anthropogenic gradients on native and exotic winter annuals in a southern California Desert, Plant Ecol., 212, 1079–1089, https://doi.org/10.1007/s11258-010-9888-5, 2011.
Rather, J. B.: An accurate loss-on-ignition method for the determination of organic matter in soils, J. Ind. Eng. Chem., 10, 439–442, 1918.
R Core Team: R: A language and environment for statistical computing. R Foundation for Statistical Computing, Vienna, Austria, https://www.R-project.org (last access: 30 May 2024), 2021.
Rhine, E. D., Mulvaney, R. L., Pratt, E. J., and Sims, G. K.: Improving the Berthelot Reaction for Determining Ammonium in Soil Extracts and Water, Soil Sci. Soc. Am. J., 62, 473–480 https://doi.org/10.2136/sssaj1998.03615995006200020026x, 1998.
Risch, A. C., Zimmermann, S., Ochoa-Hueso, R., Schütz, M., Frey, B., Firn, J. L., Fay, P. A., Hagedorn, F., Borer, E. T., Seabloom, E. W., Harpole, W. S., Knops, J. M. H., McCulley, R. L., Broadbent, A. A. D., Stevens, C. J., Silveira, M. L., Adler, P. B., Báez, S., Biederman, L. A., Blair, J. M., Brown, C. S., Caldeira, M. C., Collins, S. L., Daleo, P., di Virgilio, A., Ebeling, A., Eisenhauer, N., Esch, E., Eskelinen, A., Hagenah, N., Hautier, Y., Kirkman, K. P., MacDougall, A. S., Moore, J. L., Power, S. A., Prober, S. M., Roscher, C., Sankaran, M., Siebert, J., Speziale, K. L., Tognetti, P. M., Virtanen, R., Yahdjian, L., and Moser, B.: Soil net nitrogen mineralisation across global grasslands, Nat. Commun., 10, 1–10, https://doi.org/10.1038/s41467-019-12948-2, 2019.
Sala, O. E., Armesto, J. J., Berlow, E., Dirzo, R., Huber-sanwald, E., Huenneke, L. F., Jackson, R. B., Kinzig, A., Leemans, R., Lodge, D. M., Mooney, H. A., Poff, N. L., Sykes, M. T., Walker, B. H., Walker, M., and Wall, D. H.: Global Biodiversity Scenarios for the Year 2100, Science, 287, 1770–1774, 2000.
Schimel, D. S.: Drylands in the earth system, Science, 327, 418–419, https://doi.org/10.1126/science.1184946, 2010.
Schimel, J. P.: Life in dry soils: Effects of drought on soil microbial communities and processes, Annu. Rev. Ecol. Evol. Syst., 49, 409–432, https://doi.org/10.1146/annurev-ecolsys-110617-062614, 2018.
Schwinning, S. and Sala, O. E.: Hierarchy of responses to resource pulses in arid and semi-arid ecosystems, Oecologia, 141, 211–220, https://doi.org/10.1007/s00442-004-1520-8, 2004.
Sha, M., Xu, J., Zheng, Z., and Fa, K.: Enhanced atmospheric nitrogen deposition triggered little change in soil microbial diversity and structure in a desert ecosystem, Glob. Ecol. Conserv., 31, e01879, https://doi.org/10.1016/j.gecco.2021.e01879, 2021.
Sinsabaugh, R. L., Belnap, J., Rudgers, J., Kuske, C. R., Martinez, N., and Sandquist, D.: Soil microbial responses to nitrogen addition in arid ecosystems, Front. Microbiol., 6, 1–12, https://doi.org/10.3389/fmicb.2015.00819, 2015.
Sinsabaugh, R. L. and Shah, J. J. F.: Ecoenzymatic stoichiometry and ecological theory, Annu. Rev. Ecol. Evol. Syst., 43, 313–343, https://doi.org/10.1146/annurev-ecolsys-071112-124414, 2012.
Stevens, C. J., Dupr, C., Dorland, E., Gaudnik, C., Gowing, D. J. G., Bleeker, A., Diekmann, M., Alard, D., Bobbink, R., Fowler, D., Corcket, E., Mountford, J. O., Vandvik, V., Aarrestad, P. A., Muller, S., and Dise, N. B.: Nitrogen deposition threatens species richness of grasslands across Europe, Environ. Pollut., 158, 2940–2945, https://doi.org/10.1016/j.envpol.2010.06.006, 2010.
Stursova, M., Crenshaw, C. L., and Sinsabaugh, R. L.: Microbial Responses to Long-Term N Deposition in a Semiarid Grassland, Microb. Ecol., 51, 90–98, https://doi.org/10.1007/s00248-005-5156-y, 2006.
Tilman, D.: Secondary succession and the pattern of plant dominance along experimental nitrogen gradients., Ecol. Monogr., 57, 189–214, https://doi.org/10.2307/2937080, 1987.
Vitousek, P. M., Mooney, H. A., Lubchenco, J., and Melillo, J. M.: General – The Rocky Mountain Law Journal, Science, 277, 494–499, 1997.
Vojdani, A., Baur, L. E., Rudgers, J. A., and Collins, S. L. : Sensitivity of root production to long-term aridity under environmental perturbations in Chihuahuan Desert ecosystems, J. Ecol., https://doi.org/10.1111/1365-2745.14319, 2024.
Walvoord, M. A., Phillips, F. M., Stonestrom, D. A., Evans, R. D., Hartsough, P. C., Newman, B. D., and Striegl, R. G.: A Reservoir of Nitrate Beneath Desert Soils, Science, 302, 1021–1024, https://doi.org/10.1126/science.1086435, 2003.
Wang, J. and Wen, X.: Divergent responses of nitrogen availability to aridity in drylands, Plant Soil, 482, 111–125, https://doi.org/10.1007/s11104-022-05673-1, 2023.
Wei, C., Yu, Q., Bai, E., Lü, X., Li, Q., Xia, J., Kardol, P., Liang, W., Wang, Z., and Han, X.: Nitrogen deposition weakens plant-microbe interactions in grassland ecosystems, Glob. Change Biol., 19, 3688–3697, https://doi.org/10.1111/gcb.12348, 2013.
Widdig, M., Heintz-Buschart, A., Schleuss, P. M., Guhr, A., Borer, E. T., Seabloom, E. W., and Spohn, M.: Effects of nitrogen and phosphorus addition on microbial community composition and element cycling in a grassland soil, Soil Biol. Biochem., 151, 108041, https://doi.org/10.1016/j.soilbio.2020.108041, 2020.
Yahdjian, L. and Sala, O. E.: Size of precipitation pulses controls nitrogen transformation and losses in an arid Patagonian ecosystem, Ecosystems, 13, 575–585, https://doi.org/10.1007/s10021-010-9341-6, 2010.
Zak, D. R., Tilman, D., Parmenter, R. R., Rice, C. W., Fisher, F. M., Vose, J., Milchunas, D., and Martin, C. W.: Plant production and soil microorganisms in late-successional ecosystems: A continental-scale study, Ecology, 75, 2333–2347, https://doi.org/10.2307/1940888, 1994.
Zeng, D. H., Li, L. J., Fahey, T. J., Yu, Z. Y., Fan, Z. P., and Chen, F. S.: Effects of nitrogen addition on vegetation and ecosystem carbon in a semi-arid grassland, Biogeochemistry, 98, 185–193, https://doi.org/10.1007/s10533-009-9385-x, 2010.