the Creative Commons Attribution 4.0 License.
the Creative Commons Attribution 4.0 License.
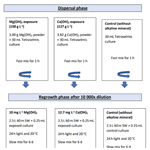
Ocean alkalinity enhancement impacts: regrowth of marine microalgae in alkaline mineral concentrations simulating the initial concentrations after ship-based dispersions
Stephanie Delacroix
Tor Jensen Nystuen
August E. Dessen Tobiesen
Andrew L. King
Erik Höglund
Increasing the marine carbon dioxide (CO2) absorption capacity by adding alkaline minerals into the world's oceans is a promising marine carbon dioxide removal (mCDR) approach to increase the ocean's CO2 storage potential and mitigate ocean acidification. Still, the biological impacts of dispersion of alkaline minerals need to be evaluated prior to its field deployment, especially the impacts of the initial discharge causing local and temporary extreme alkalinity/pH changes. In this study, the toxicity effect on marine microalgae of two commonly used alkaline minerals, calcium hydroxide (Ca(OH)2) and magnesium hydroxide (Mg(OH)2), was determined by adding the same equivalent molar concentration of hydroxyl ions. Cultures of marine green microalgae Tetraselmis suecica were exposed to Ca(OH)2 or Mg(OH)2, in concentrations mimicking the initial high concentrations following a dispersion scenario from a ship. A short-term exposure with high-alkaline mineral concentration called “dispersion phase” was followed by a dilution step and a “regrowth” phase over 6 d. There was no detectable effect of Mg(OH)2 treatment on algae growth either after the dispersion phase or during the regrowth phase, compared to control treatments. The Ca(OH)2 treatment resulted in very few living algal cells after the dispersion phase, but a similar growth rate was observed during the regrowth phase as was for the Mg(OH)2 and control treatments. Standardized whole effluent toxicity (WET) tests were carried out with a range of Mg(OH)2 concentrations using a sensitive marine diatom, Skeletonema costatum, which confirmed the relatively low toxicity effect of Mg(OH)2. Similar biological effects were observed on natural microalgae assemblages from a local seawater source when applying the same Mg(OH)2 concentration range and exposure time used in the WET tests. The results suggest that Mg(OH)2 is relatively safe compared to Ca(OH)2 with respect to marine microalgae.
- Article
(773 KB) - Full-text XML
- BibTeX
- EndNote
It is widely recognized that reducing carbon dioxide emissions is not sufficient to accomplish the goals of the Paris Agreement of 2015, to limit global warming and ocean acidification (Pathak et al., 2022). Accordingly, there is an urgent need for additional approaches to remove carbon dioxide. Many different marine dioxide carbon removal (mCDR) approaches are currently under evaluation (GESAMP, 2019), including artificial upwelling/downwelling, nutrient fertilization, deep sea storage, electrochemical ocean carbon dioxide removal, macroalgal/microalgal cultivation, marine ecosystem restoration, and ocean alkalinity enhancement (OAE). In general, the principle of some of these approaches is based on acceleration of the natural process of absorption and long-term storage of the excess atmospheric carbon dioxide by the ocean (Siegel et al., 2021; NASEM, 2021). Among them, OAE has been put forward as one of the most promising approaches, because the acidification remediation process itself triggers the reduction of the atmospheric carbon dioxide level (Renforth and Henderson, 2017). Hence, when the aqueous carbon dioxide deficit, generated by the addition of alkaline mineral, returns to the initial equilibrium with atmospheric carbon dioxide, the final pH still remains slightly higher than the initial pH, while calcite (most stable polymorph of calcium carbonate CaCO3) level and aragonite (crystal structure of calcium carbonate) saturation state are elevated. The aragonite saturation state is commonly used to track ocean acidification (Jiang et al., 2015). The most studied alkaline minerals for OAE approaches are limestone (CaCO3), olivine (Mg,Fe)2SiO4, sodium hydroxide (NaOH), and calcium hydroxide (Ca(OH)2) (DOSI, 2022). While the latter mineral has been evaluated for large-scale application on the Mediterranean Sea (Butenschön et al., 2021), a large-scale study involving field deployment of olivine in coastal waters off New York, USA, is currently being performed (Tollefson, 2023). Magnesium hydroxide has also recently been studied (Yang et al., 2023; Hartmann et al., 2023). Its relatively low water solubility allows it to be added in a larger amount without reaching harmful pH levels (Tollefson, 2023) and will potentially increase the durability of the alkalinization effect. Following this, in addition to raw material source scalability (Caserini et al., 2022), alkalinization efficiency and solubility are important criteria of OAEs (Hartmann et al., 2023; Ilyina et al., 2013). Moreover, the effects on the aquatic environment need to be considered, including the biological impact of the initial discharge of high-alkaline mineral concentrations upon dispersion causing local and temporary extreme alkalinity/pH changes. Accordingly, Bach et al. (2019) and Burns and Corbett (2020) pointed out that before approval of the alkaline mineral dispersion at global scale, a risk assessment of the toxicity effect of the alkaline minerals on marine organisms must be performed. Thus, it is crucial to consider not only the toxicity effect, if any, of the final low-alkaline mineral concentration after expected final dilution into the ocean, but also the potential initial toxicity effect of the initial hotspot discharge of the alkaline mineral on local organisms. These discharges upon dispersion might be local and temporary, but it is important to consider that they would be applied at a global scale. These local and temporary effects will potentially include increased cation levels (Mg2+ and Ca2+), increased bicarbonate and carbonate ions, pH increase, or decrease of dissolved carbon dioxide. Perturbations that potentially form impact hotspots, affecting phytoplankton species composition and growth, result in impacts higher up in the food chain (Bach et al., 2019). Biological impacts will strongly depend on the spatial and temporal scale of alkaline mineral dispersion, and studies must therefore use realistic alkaline mineral dispersion scenarios.
In this study, the biological impact of initial and temporary discharge of Mg(OH)2 concentrations expected from dispersion from a moving ship was compared to Ca(OH)2 on marine microalgae. This was done by exposing cultured Tetraselmis suecica to the above alkaline minerals. The toxicity of Mg(OH)2 was then further investigated by using a sensitive microalgal species, in a recognized and standardized whole effluent toxicity (WET) test with cultured diatom Skeletonema costatum. Additional experiments were performed for further toxicity assessment of Mg(OH)2 on a natural microalgal assemblage from local seawater.
The study was performed in three steps. In the first step, the toxicity effect was studied by exposing marine algae to alkaline minerals in successive concentrations mimicking dispersion from a moving ship. These experiments were carried out with Tetraselmis suecica, a standard test organism in toxicity studies (Ebenezer et al., 2016; Li et al., 2017; Seoane et al., 2014; Vagi et al., 2005). In the second step, toxicity effects of the alkaline minerals were verified by a standardized WET ecotoxicology assay with Skeletonema costatum, a more sensitive marine algal species (Petersen et al., 2014; Wee et al., 2016), by using the recognized 72 h growth inhibition test (ISO 10253, 2016). In the third step, the toxicity effect was studied by exposing a natural assemblage of marine algal species from the Oslofjord, Drøbak, Norway, to similar Mg(OH)2 concentrations used in the WET tests. All experiments were carried out in non-airtight containers to allow ambient CO2 to re-equilibrate with seawater used for the experiments.
2.1 Exposure of Tetraselmis suecica to simulated dispersion of alkaline minerals from a moving ship
The expected distribution of a slurry of Mg(OH)2 during its dispersion from the ship's discharge point on the surface of the oceans was determined utilizing computational fluid dynamic (CFD) models (FORCE Technology Inc., Denmark) and the Bottom RedOx Model (BROM) (Yakushev et al., 2017). In those models, both the forced and natural mixing effects of the Mg(OH)2 by the ship's propeller and physical oceanic processes (waves, convection, currents, etc.), respectively, in the ship's wake were simulated with different scenarios, including propeller motion, velocity of tangential ocean currents, Mg(OH)2 slurry discharge rate dissolution rate settling rate, ship size, and ship speed. Dilution was observed with an immediate minimum dilution rate of within 2 min after injection, followed by an additional minimum dilution rate of during the next 5 h and a final minimum dilution rate of during the following next 5 h. Moreover, the tonnage capacity and operating costs of a ship were also considered together with a final Mg(OH)2 concentration target of <1 mg L−1. Taken together, this suggested that the dispersion rate of 500 kg s−1 would be the most realistic applicable scenario. From this dispersion rate, it was concluded that marine organisms would be exposed to <100 g L−1 approximately for less than 1 h followed by a dilution to <10 mg L−1 over a period of 10 h.
To investigate biological impact of Mg(OH)2 and compare it with Ca(OH)2, cultures of Tetraselmis suecica were exposed to these three alkaline minerals during a simulated dispersion phase (as described above) followed by a regrowth phase (Fig. 1). In the dispersion phase, 30 mL of Tetraselmis suecica cultures (see further down), in exponential growth with a cell density range within 2.6×105–1.4×106 cells mL−1, was exposed to the alkaline minerals in 50 mL glass beakers with continuous mixing at approximately 300 rpm with a magnetic stirrer (VELP Scientifica) for 1 h. To achieve similar concentrations of hydroxide ions in the different alkaline mineral treatments, algae were exposed to either 100 g L−1 (or 1.7 M) of Mg(OH)2 or 127 g L−1 (or 1.7 M) of Ca(OH)2 (Fig. 1).
In the regrowth phase, a subsample from each exposure media was diluted by 10 000 in local seawater, and algal cell density was monitored for 6 d. The dilution was performed by mixing a 0.25 mL subsample to 2.5 L of ambient 60 m deep seawater from the Oslofjord (Fig. 1). The diluted subsamples were incubated in 3 L glass beakers in a 20 °C temperature-controlled climate room with 24 h light (2× 21 W Philips Pentura Mini) and continuous mixing with a magnetic stirrer (VELP Scientifica; 100 rpm approximately). The measured light intensity was within 20–60 µmol photons m−2 s−1. As the beakers were left uncovered, evaporated water volume was replaced every 24 h (except for week-end period) by an equivalent volume of ultrapure water. Effects of each alkaline mineral were investigated in triplicate, including both the exposure and regrowth phases, resulting in total of nine bioassays which were conducted in the laboratory facilities of the Norwegian Institute for Water Research (NIVA) in Oslo between November 2021 and January 2022. Each bioassay study was conducted with one or two alkaline minerals in parallel and repeated three times for each alkaline mineral with new cultures of Tetraselmis suecica, except for two of the NaOH studies, which were started on the same day from the same algal culture. In addition, control bioassays excluding the addition of alkaline minerals were performed in parallel with each alkaline mineral exposure, including a dispersal phase followed by a regrowth phase.
The ambient Oslofjord seawater was unfiltered and unsterilized water collected from 60 m depth just outside of NIVA's marine research station located at Drøbak, 40 km south of Oslo. The water quality of this seawater is stable year-round with a temperature of approximately 7 °C. This water is representative of ocean regions (i.e. rich in oxygen but poor in inorganic and organic contents), with 0.7 mg C L−1 of particulate organic carbon (POC), 1.1 mg C L−1 of dissolved organic carbon (DOC), 6 mg L−1 of total suspended solids (TSSs), and very low biological load with <1 cell mL−1 of algae and less than 500 CFU mL−1 of heterotrophic bacteria.
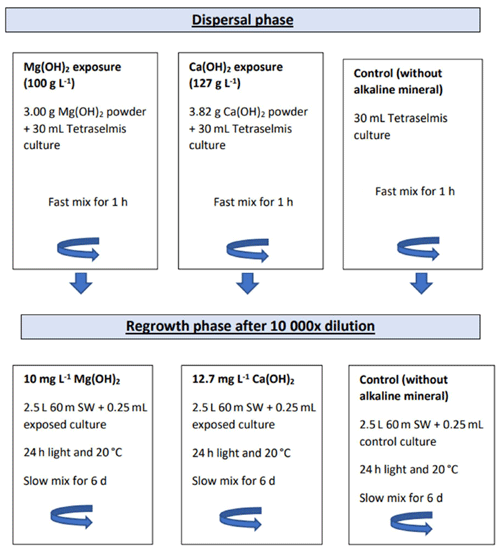
Figure 1Schematic illustration of the experimental set-up including the dispersion phase in 50 mL glass beakers followed by the dilution step and the regrowth phase of the exposed algal cells in 3 L glass beakers.
Before exposure, the algae were collected from 1 L laboratory cultures of Tetraselmis suecica (NIVA-3/10; Norwegian Institute for Water Research, Oslo, Norway). At first, a 50 mL algal culture was prepared by semi-static cultivation in a 100 mL glass flask with 50 mL of autoclaved 20 % Z8 culture medium with addition of vitamins (Kotai, 1972). The medium culture was inoculated with 5–10 mL of the T. suecica culture from NIVA's algal culture collection. The culture was incubated for ∼ 1 week with fluorescent light tubes giving 20–60 µmol photons m−2 s−1, provided by cool-white fluorescence lamps (TLD 36W/950, Philips, London, UK), on an Infors Multicrom 2 incubator shaker (Infors AG, Bottmingen, Switzerland) at 20±2 °C, with orbital shaking at 90 rpm. After incubation, the culture was used for the inoculation of the 1 L culture, except for ∼ 10 mL, which was held back to start a new 50 mL culture by adding 40 mL of freshly prepared Z8 medium in the same culture conditions as described above. The 1 L culture was prepared by static cultivation with 1 L autoclaved 20 % Z8 medium with the addition of 1 mL L−1 vitamins in 2 L glass culture bottles. Approximately 40 mL of the 50 mL stock culture was added to 1 L of medium. The culture was exposed to fluorescent light tubes of 20–60 µM m−2 s−1 and placed in a 20 °C temperature-controlled room for approximately 1 week.
The culture medium was prepared at least 24 h before usage to allow the equilibrium of media components. The 20 % Z8 culture medium was made by mixing 0.2 L of Z8 medium into 0.8 L seawater and briefly aired with CO2 (<1 min) to avoid precipitation of salts during autoclaving. The seawater was pasteurized seawater collected from 60 m depth in the Oslofjord. The medium was autoclaved for 15 min at 121 °C. Then, 1 mL L−1 of vitamin stock solution was added to the 20 % Z8 medium (Kotai, 1972).
The studied alkaline minerals were magnesium hydroxide (CAS no. 1309-42-8), calcium hydroxide (CAS no. 1305-62-0), and sodium hydroxide (CAS no. 1310-73-2) – all with ≥97.0 % purity. Magnesium hydroxide (batch no. 18417-01A) was provided by Negative Emission Materials, Inc. via a factory in Canada producing the mineral by a hydrometallurgy process and purification from natural magnesium silicate. The two other alkaline minerals were purchased from Sigma-Aldrich (United Kingdom).
The density of living Tetraselmis suecica was determined using the double-staining method with fluorescein diacetate (FDA) and 5-chloromethylfluorescein diacetate (CMFDA) (NSF, 2010). This double-staining method, FDA/CMFDA, is based on the validation work of the US Navy Research Laboratory to distinguish between living and dead cells after disinfection by a ballast water treatment (Steinberg et al., 2011). This viability method is the only one recognized by both the International Maritime Organization (IMO) and the United States Coast Guard (USCG) for approval of ballast water discharge from 70 000 commercial ships at a global scale (USGC, 2012; IMO, 2018).
The following staining protocol was used: a 2.5 mM CMFDA stock solution was prepared by dissolving 1 mg of CMFDA in 0.86 mL DMSO (dimethyl sulfoxide). It was then divided into 50 µL batches and stored at −20 °C. The 5 mM FDA stock solution was prepared by dissolving 10 mg FDA in 4.8 mL DMSO. The FDA stock solution was divided into 100 µL batches and stored at −20 °C. For each analysis, a 4 mL subsample was collected, and 4 µL of 10 % HCl was added, bringing the pH back to approximately 8 prior to staining. A total of 4 µL of each stock solution was added to each subsample, resulting in final concentrations of 2.5 µM CMFDA and 5 µM FDA. The subsamples were then incubated in darkness for 10 min, after which they were loaded into 1 mL Sedgewick Rafter counting chambers etched with two 1 mm grids. Chambers were examined at 100× magnification using compound epifluorescent microscopes with standard blue-light excitation (480 nm) and green-bandpass emission (530 nm) filter cubes. Samples were counted within a 45 min period after incubation. The stained Tetraselmis suecica cells were counted in triplicate (3×1 mL) The untreated algal samples without alkaline mineral were used as positive controls. Both T. suecica and local diatoms are nearly 100 % stainable with these stains according to our 15 years of experience with this method in our local seawater. Samples treated with sodium hydroxide (NaOH) to increase the pH to approximately 14 were used as negative controls. No fluorescence could be observed in the negative controls, indicating an instant kill effect of the algal cells.
Temperature, salinity, and pH in the bioassays were measured in situ by using a calibrated handheld WTW multimeter (WTW Multi 3620 IDS/3420 IDS displayer) with a conductivity probe (TetraCon 925 Xylem) and a pH electrode (SenTix 945P). The three-point calibration method with Hamilton pH buffer solutions (4, 7, and 10) was used for the calibration of the pH electrode, according to WTW instructions. The temperature in the test waters varied within a range of 18–23 °C for all experiments during the 6 d of regrowth phase as all experiments were conducted at room temperature. The same temperature was registered in the alkaline test waters compared to the corresponding control waters. The salinity of the test waters, with or without alkaline mineral, was around 32–33 PSU at the start of the 6 d regrowth phase for all experiments. The salinity stayed relatively stable for most of the regrowth phase, except for the last day with an increase up to 35–36 PSU on average. This increase was due to the evaporation of the test water at room temperature during the weekend period included at the end of the 6 d of experimentation.
2.2 Whole effluent toxicity (WET) test
The WET test consisted of a marine algal growth inhibition test of 72 h performed by NIVA's ecotoxicity laboratory according to NIVA's standard procedure, which is based on the International Standard ISO 10253: Water Quality – Marine algal growth inhibition test with Skeletonema costatum and Phaeodactylum tricornutum. In this study, the diatom S. costatum (NIVA-strain BAC 1) was used as a test organism.
A 100 mg L−1 Mg(OH)2 sample was diluted by using a modified ISO 10253 media, except that no Fe-EDTA stock solution was added, as the tested compound Mg(OH)2 was shown to be affected by the presence of EDTA, causing precipitation of Mg(OH)2. A preliminary study was made to verify the microalgal growth in this modified media. Although less growth was observed when compared to normal ISO 10253 media, the specific daily growth rate was still greater than 0.9 d−1, which was considered valid. A total of six concentrations of Mg(OH)2 were tested (1, 10, 25, 50, 75, and 100 mg L−1). The test was performed with 15 mL samples in 30 mL glass vials. Each concentration was tested in triplicate with six replicates for each control (one control set with normal ISO 10253 and another control set with modified ISO 10253): the same number of replicates for analysis of blank samples but without microalgae added.
All samples were inoculated with 5×106 cells L−1 of S. costatum from an exponentially growing laboratory culture and incubated on a shaking table at 20±1 °C under continuous illumination of 63 µM m−2 s−1 of photosynthetic active radiation (PAR).
The cell density was determined by FDA and CMFDA double staining and fluorescence at 645 nM in SpectraMax iD3 microplates after approximately 24, 48, and 72 h (±2 h). The fluorescence measurements were directly correlated to the algal density, as a correlation factor (r2) of 1 between the measured fluorescence and the cell density was calculated. The fluorescence values of the exposed samples without algae (blanks) were measured to investigate potential biases caused by the effect of the tested substance on the fluorescence readings. As no such effects were detected, no further transformation of data was necessary.
The temperature, pH, and salinity were measured in situ at the beginning and at the end of each WET test. The temperature varied from 19.9 to 20.3 °C for both WET tests. The pH at the start of the experiment varied from 8.09 to 9.38 in all vials for both tests, with increasing pH for increasing Mg(OH)2 concentrations as expected. The pH at the end of the experiment varied from 8.27 to 8.54 in all vials for both tests. The salinity was stable with 32–35 PSU in all vials during the entire experiment for both tests.
2.3 Natural assemblage of ambient marine algal test
For the preparation of the ambient algal culture, either a 25 L grab sample from the surface water of the Oslofjord was directly used for the test or a 2 L subsample was mixed to 2 L of 60 m deep seawater from the Oslofjord for further algal growth. For growth, the culture was incubated in a 5 L glass beaker in a climate room at 20 °C and with constant light from fluorescent light tubes of 20–60 µM m−2 s−1 for 4 d. The total density of algal cells in the culture after incubation was approximately 1000 cell mL−1. A total of 500 mL of the culture was then mixed in a 2 L glass beaker with a magnetic stirrer at approximately 90 rpm and added to 1500 mL of a prepared Mg(OH)2 suspension, resulting in Mg(OH)2 concentrations of 1, 10, 25, 50, 75, and 100 mg L−1 and an initial algal density of approximately 125–250 cell mL−1. The Mg(OH)2 suspensions were prepared by mixing 2.7, 27, 66, 133, 200, or 270 mg of Mg(OH)2 in 1.5 L of unfiltered 60 m seawater from the Oslofjord, with a magnetic stirrer (300 rpm) over the night prior test start. The final solutions were slowly mixed continuously with a magnetic stirrer at approximately 90 rpm, in a climate room at 20 °C and with constant light from fluorescent light tubes of 20–60 µmol photons m−2 s−1 for 72 h. The water quality and algal density were monitored daily in each beaker, the same methods described in 2.1. Moreover, cell count and viability were quantified using the same protocol as in 2.1, with fluorescence measured at 645 nm. For the control treatments, 500 mL of the ambient algal culture was mixed with 1.5 L of unfiltered 60 m deep seawater from the Oslofjord, without Mg(OH)2, and incubated as described above. Those tests were carried out on different weeks. Therefore, different control treatments applied for 1–10 mg L−1 Mg(OH)2 treatments, 50–75 mg L−1 Mg(OH)2 treatments, and 100 mg L−1 Mg(OH)2 treatments (see Appendix C). Aliquots from the 100 mg L−1 treatment were collected from the initial time point and final time point (t=3 d) for microscopy-based assessment of community composition by taxa.
2.4 Data analysis
Effects on T. suecica cell survival with Ca(OH)2 and Mg(OH)2 in simulated dispersions from a moving ship were analysed with Student's t test with the type of alkaline mineral as an independent grouping variable and percent survival compared to control treatments after the regrowth phase as the dependent variable. Data were log-transformed to obtain similar variation between groups.
In the WET test, the growth rate of S. costatum in each Mg(OH)2 sample was calculated from the logarithmic increase of cell density from start to 72 h and expressed as the percentage of the growth rate of control samples. The concentrations causing 50 % growth inhibition (EC50) were calculated using a non-linear regression analysis of the growth rate versus log cell concentration of control water (Hill, 1910; Vindimian et al., 1983). The non-observed effect concentration (NOEC) and the lowest observed effect concentration (LOEC) were calculated using Dunnett's test/t test for non-homogenous variance and the Williams multiple sequential t test for homogenous variance.
The effects of Mg(OH)2 on the natural marine algal assemblage were investigated by dividing the different exposure concentrations (1, 10, 25, 50, 75, and 100 mg L−1) within two groups based on the LOEC (25 mg L−1) from the WET test. This resulted in one low concentration group (1, 10, and 25 mg L−1) and one high concentration group (50, 75, and 100 mg L−1). The difference in percent survival compared to control treatment between the high and low concentration groups was investigated via Student's t test. This approach, with three replicates in each group, allowed us to investigate the effects of increased MgOH2 concentrations.
3.1 Exposure of Tetraselmis suecica to simulated dispersion of alkaline minerals from a moving ship
There were significant differences in living cells of Tetraselmis suecica (percent survival compared to control treatments; Table 1) between the alkaline minerals in the end of the regrowth phase (Student's t test; t=9.4, P<0.0001), which were reflected in both the dispersion and the regrowth phases. At the start of the regrowth phase, the surviving cell densities in the Mg(OH)2 treatments were similar to the ones observed in control treatment, while only one living cell was observed in one of the Ca(OH)2 treatments (Day 0; Table 1). In the Mg(OH)2 and Ca(OH)2 treatments, algal cell densities increased during the regrowth phase (Day 1–6; Table 1). At the end of the regrowth phase, the algal cell densities in the Mg(OH)2 treatments were similar to those in control treatments, while the algal cell densities in Ca(OH)2 treatments showed lower values than in control treatments (Fig. 2).
Table 1Densities of living Tetraselmis suecica (cell mL−1) and their relation to control treatment (% Contr.) during the regrowth phase of a bioassay mimicking dispersion of the alkaline minerals Mg(OH)2 or Ca(OH)2 from a ship. Before the regrowth phase, algae were exposed to either 100 g L−1 Mg(OH)2 or 127 g L−1 Ca(OH)2 (achieving similar amounts of hydroxide in the different alkaline mineral suspensions) for 1 h. After this, subsamples from each treatment were diluted 10 000 times, and algae growth was studied during a 6 d regrowth phase. Each alkaline mineral was assayed in triplicate. Values at Day 0 correspond to 1 h after dilution, and effects of each alkaline mineral were investigated in triplicate.

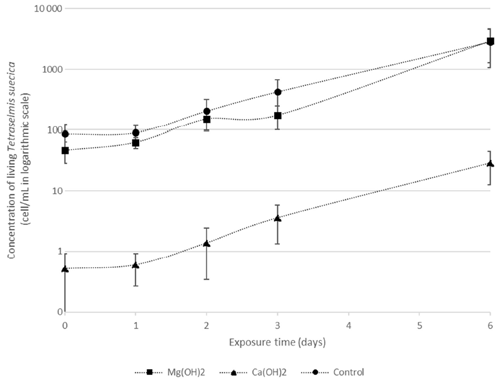
Figure 2Densities of living Tetraselmis suecica (cell mL−1) during the regrowth phase of a bioassay mimicking dispersion of the alkaline minerals Mg(OH)2 or Ca(OH)2 from a ship. Before the regrowth phase, algae were exposed to either 100 g L−1, Mg(OH)2 or 127 g L−1 Ca(OH)2 (achieving similar concentrations of hydroxide ions in the different solutions) for 1 h. After this, subsamples from each treatment were diluted 10 000 times, and algae growth was studied during the 6 d regrowth phase.
The pH in the control treatments was around 8.0–8.2 during the regrowth phase (Fig. 3), while alkaline mineral treatments resulted in elevated pH (∼ 8.5) at Day 1 after the dilution step. Thereafter, pH decreased and reached similar values to the control treatments on Day 3 for all alkaline mineral treatments (Fig. 3).
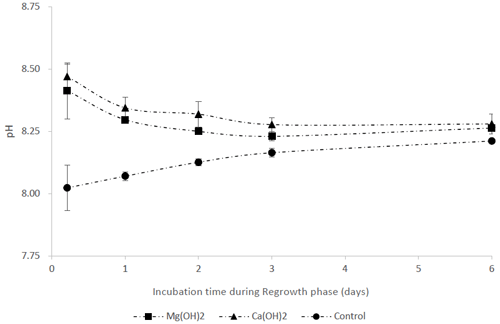
Figure 3pH during the regrowth phase in a bioassay mimicking the dispersion of the alkaline minerals Mg(OH)2 or Ca(OH)2 from a ship. Before the regrowth phase, algae were exposed to either 100 g L−1 Mg(OH)2 or 127 g L−1 Ca(OH)2 (achieving similar concentrations of hydroxide ions in the different alkaline mineral solutions) for 1 h. After this, subsamples from each treatment were diluted 10 000 times to achieve the following concentrations during the regrowth phase: 10 mg L−1 Mg(OH)2 or 12.7 mg L−1 Ca(OH)2.
3.2 WET tests
The results of the lowest observed effect concentration (LOEC) and the non-observed effect concentration (NOEC) of Mg(OH)2 were similar in both WET tests, with 50 and 25 mg L−1 Mg(OH)2, respectively. The Mg(OH)2 concentration causing 50 % algal growth inhibition was close to 100 mg L−1 in both tests, within a range of 82–111 mg L−1 (Table 2).
Table 2Results of the duplicate whole effluent toxicity (WET) tests (WET tests 1 and 2) for three endpoints (EC50, LOEC, and NOEC) after 72 h exposure of the marine microalgae Skeletonema costatum with a total of six different concentrations of magnesium hydroxide (1, 10, 25, 50, 75, and 100 mg L−1). Those concentrations were prepared by diluting an initial Mg(OH)2 solution in the algal culture medium, prior to algal inoculation. The initial solution was a freshly prepared 1 L suspension of 100 g L−1 Mg(OH)2 in ambient 60 m deep seawater from the Oslofjord. EC50: concentration causing 50 % algal growth inhibition. LOEC: lowest observed effect concentration. NOEC: non-observed effect concentration (NOEC).
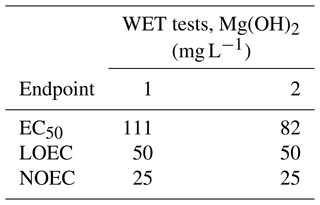
3.3 Natural assemblage of ambient marine algal species
There was a significant difference in algal survival between the low concentration group (1, 10, and 25 mg L−1 Mg(OH)2) and the high concentration group (50, 75, and 100 mg L−1 Mg(OH)2) after 3 d of exposure (, P<0.01; Table 3). The analysis of the algal biodiversity composition in the 100 mg L−1 Mg(OH)2 suspension showed that the dominant surviving species was diatoms, including Skeletonema spp., with 80 % and 94 % of the total on Day 0 and Day 3, respectively. The biodiversity composition of the natural algal assemblage in the beginning and at the end of the experiment for the 100 mg L−1 Mg(OH)2 treatment is given in Table 4.
Table 3Densities of living ambient algal cells (cell mL−1) and their survival in percentage compared to corresponding control water without Mg(OH)2 (% Contr.), during 3 d of exposure to six different concentrations of Mg(OH)2 (1, 10, 25, 50, 75, and 100 mg L−1) when incubated in a 20 °C temperature-controlled room with constant light. Low and high concentration groups refer to the groups used in Student's t test; see Sect. 2.4 statistics for more information.

4.1 Dispersal model and experimental design
The current ship dispersal model suggests a dilution rate of over a 2 min period in the near-field of the wake, given a dispersal rate of 500 kg s−1. This is consistent with a recent study where the dispersal of Ca(OH)2 from a ship was modelled. The study showed that dilution rates could vary between 710–7100, depending on the diffusion potential of the Ca(OH)2, at a dispersal rate of 100 kg s−1, 810 m in the wake behind the ship (Caserini et al., 2022). This distance corresponds to 2 min at the modelled ship speed of 25 km h−1. Another study from the Cefas Burnham Laboratory, in which maximum (but safe levels of) discharge of industrial waste from ships was sought after, calculated that ship discharge dilution rates of within 5 min was possible (Chris Vivian, personal communication, 2023). Thus, the model in the current paper predicts dilution rates that are within what another model suggests. Still, regarding models of safe levels of discharge of industry waste, it is important to note that maximum dispersal (discharge) is not the sole criterion for ocean alkalinity enhancement but rather an intermediate between a high dispersal rate for maximum input and a low dispersal rate to promote maximum dissolution for the alkaline material of choice. For example, in the dispersal model scenario used for designing the experiments in the current study, a dilution after 1 h resulted in a final concentration of Mg(OH)2 and Ca(OH)2 of 10 and 12.7 mg L−1, respectively. At these concentrations, both alkaline materials are expected to fully dissolve for optimal CO2 uptake while also not resulting in elevated calcium carbonate saturation states, leading to “runaway” secondary precipitation of calcium carbonate (e.g. secondary precipitation was observed at ΩAr>7 for Ca(OH)2 on the timescale of 4–5 h; Moras et al., 2022). Still, it cannot be excluded that some uncontrolled CaCO3 precipitation could have occurred at 100 mg L−1 of Mg(OH)2 and 127 mg L−1 of Ca(OH)2 during the initial 1 h of exposure in the present study.
4.2 Regrowth of Tetraselmis suecica
Similar algal densities were observed in both control and Mg(OH)2 treatments at the beginning of the regrowth phase (Day 0, Table 1). This could be related to the short exposure time or to the low solubility of Mg(OH)2: 0.012 g L−1 in pure water and around 0.008 g L−1 in seawater (Yang et al., 2023). For comparison, the solubility of Ca(OH)2 is 1.73 g L−1 at 20–25 °C. Accordingly, pH increased during the dispersion phase from approximately 8.0 to 9.5 in the Mg(OH)2 treatment, which was lower compared to the expected pH of 12 in Ca(OH)2 (Hartmann et al., 2023). However, pH was similar at the beginning of the regrowth period for both alkaline mineral treatments at ∼ 8.3–8.6 (Fig. 3), giving similar potential regrowth conditions. The similar growth rates observed in controls, Mg(OH)2-added treatments, and Ca(OH)2-added treatments (Fig. 2) suggest that the algae previously exposed to 100 g L−1 Ca(OH)2 were able to recover during this phase, at least when the algae were incubated in optimal culture conditions, which might not be the case in natural oceanic conditions. Taken together, our data indicate high algal mortality in Ca(OH)2 at high concentrations of 127 g L−1 during the first hour after the alkaline mineral discharge from a moving ship, while no such toxic effect was observed when algae were exposed to Mg(OH)2. This emphasizes that the local and temporary biological impact of alkaline mineral discharge in the initial phase of the dispersion, in addition to alkalinity increase capability, needs to be considered when evaluating mCDR strategies. Following this, it is important to keep in mind that in this study the toxicity comparison was based on the criterion that each alkaline mineral has the same hydroxide content, not taking in account the difference in alkalinity enhancement between the alkaline minerals.
4.3 Growth inhibition test with Skeletonema costatum
The results from the WET tests indicate that no growth inhibition of S. costatum was observed for Mg(OH)2 concentrations equal to or below 25 mg L−1 (NOEC). This is somewhat in accordance with the simulated dispersion test, showing no growth inhibition of T. suecica during the 6 d of regrowth phase in 10 mg L−1 of magnesium hydroxide. The results from dispersion phase indicate no or a low effect of 1 h of exposure with 100 g L−1 of magnesium hydroxide on T. suecica. The WET tests indicate a 50 % growth inhibition effect of Mg(OH)2 concentrations (EC50) between 82 and 111 mg L−1 after 72 h of exposure. This toxicity effect might be explained by the temporary local CO2 limitation impact, limiting the algal growth, due to increasing pH at these high alkaline mineral concentrations. These EC50 values were much higher than Mg(OH)2 solubility of ∼12.2 mg L−1 in pure water (Yang et al., 2023). This raises questions regarding the cause of growth inhibition in the current study. It has been suggested that trace metals, such as Cr, Mo, Ni, and Pb, in industrial and natural mineral products used as alkaline minerals may impair organism growth (Bach et al., 2019; Hartmann et al., 2023). However, this might not be the case here as the Mg(OH)2 powder used in this study was 97 %–98 % ultrapure with <0.01 % Ni or Cr. Further studies are needed to verify and investigate the underlying mechanism for the growth inhibition of S. costatum observed in the current WET tests.
4.4 Regrowth test with assemblage of ambient algal species
The same toxicity effect of Mg(OH)2 was observed in the tests performed with local marine algal species, i.e. no significant toxicity effect of Mg(OH)2 concentrations below 25 mg L−1 but significant toxicity effect for concentrations above 50 mg L−1. Skeletonema spp. were represented in the natural assemblage, as one of the dominant species, while Skeletonema costatum was used in the WET tests. This suggests that the results from the WET tests using laboratory monoculture are still representative and applicable to similar species growing in natural marine environment. Thus, the results from the natural seawater test demonstrated that toxicity effects observed with Mg(OH)2 on laboratory cultures might be applicable to a wider range of marine algal species.
Thus, the simulated dispersion scenario, the WET tests, and ambient algal tests results suggest that Mg(OH)2 is a suitable alkaline enhancement mineral with respect to minimizing biological impacts on marine microalgae during temporary and local extreme alkaline mineral discharge upon initial phase of the dispersion. While our studies focused on marine microalgae, most other studies focused on the impact of OAE on organisms with parts containing calcium carbonate and therefore are sensitive to seawater acidification (Cripps et al., 2013; Fakhraee et al., 2023; Gomes et al., 2016; Renforth and Henderson, 2017). Microalgae play an important role as primary producers and impacts may be reflected in the entire marine ecosystem by affecting higher trophic-level organisms, such as zooplankton and fish (Pauly and Christensen, 1995; Chassot et al., 2010). Accordingly, microalgae are considered a useful and crucial indicator to evaluate the deterioration of environmental quality (Lee et al., 2023). Thus, the current study applying microalgae assays to investigate the effects of Mg(OH)2 suggests a low negative biological impact of Mg(OH)2. However, it is important to keep in mind that these laboratory assays, in addition to proximate the biological impact, are employed because they are relatively fast and cost-effective. Thus, further studies on other functional groups and species are required to ensure a low impact of the OAE.
The bioassays based on initial local and temporary discharge simulation from the scenario of alkaline mineral dispersion from a ship demonstrated that Mg(OH)2 resulted in lower biological impacts on marine microalgae when compared to Ca(OH)2. Further laboratory studies must be completed to include a wider range of biological biodiversity from different trophic levels and on a larger scale, such as in mesocosm studies, prior to field deployment. The observed low negative biological impact of Mg(OH)2 was confirmed by the standardized toxicity test using a more sensitive marine algae species as well as by the tests with a wider range of local ambient marine algal species. Additionally, there are potentially positive biological impacts of OAE, including remediation of ocean acidification conditions by reducing pH and increasing saturation state of calcium carbonate, which were not addressed in this study. Overall, these results indicate that Mg(OH)2 is a suitable mineral for OAE application. Still, it is important to consider that Mg(OH)2 needs to be maintained in suspension right below the ocean's surface to be an effective OAE. Thus, in addition to further toxicity assessment of Mg(OH)2 on an aquatic environment, techniques for optimization of its dissolution, including injection and distribution methods, in seawater need to performed.
Table 1 of the article was generated from the raw data presented in Table A1.
Table A1Daily averages (n=3) of density of living Tetraselmis suecica (cell mL−1) during the regrowth phase (Day 0–Day 6) of the triplicate tests mimicking dispersion of the alkaline minerals Mg(OH)2 or Ca(OH)2 from a ship. Before the regrowth phase, algae were exposed to either 100 g L−1 Mg(OH)2 or 127 g L−1 Ca(OH)2 (resulting in similar molar concentration of hydroxide in the two alkaline mineral suspensions) for 1 h. After this, subsamples from each treatment were diluted 10 000 times, and algae growth was studied during a 6 d regrowth phase. Each alkaline mineral treatment and corresponding control treatment was assayed in triplicate. Values at Day 0 correspond to 1 h after dilution, and effects of each alkaline mineral were investigated in triplicate.
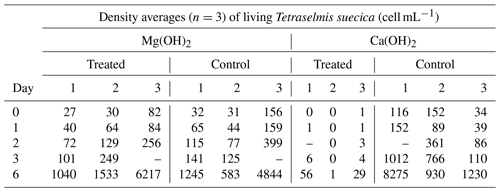
Table 3 of the article was generated from the raw data presented in Tables B1 and B2. The complete laboratory analysis reports can be provided upon request.
Table B1Calibration data for WET Test 1 and for WET Test 2 to correlate the fluorescence measurements to the cell density of Skeletonema costatum. The cell density was determined by fluorescence with a SpectraMax iD3 microplate after approximately 72 h (±2 h). The fluorescence measurements were directly correlated to the algal density, as a correlation factor (r2) of 1 between the measured fluorescence and the cell density was calculated.
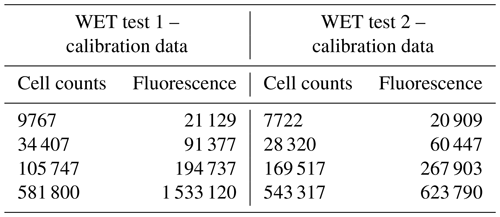
Table B2Fluorescence measurements of the control and Mg(OH)2 treatments for WET Test 1 and WET Test 2 after 72 h exposure according to ISO 10253 (2016). A total of six concentrations of Mg(OH)2 were tested (1, 10, 25, 50, 75, and 100 mg L−1). Each concentration was tested in triplicate, with six replicates for each control (one control set with normal ISO 10253 and another control set with modified ISO 10253).
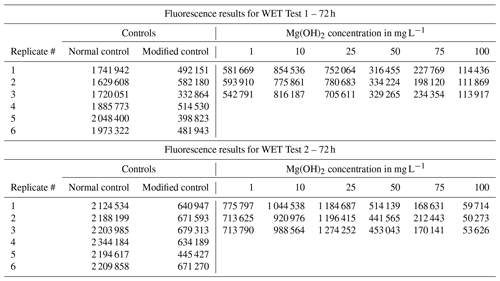
Table 3 of the article was generated from the raw data presented in Table C1 below.
Table C1Daily triplicate enumeration of density of living ambient algal cells (cell mL−1) with FDA/CMFDA method in Mg(OH)2 treated and control treatments during 3 d of exposure to six different concentrations of Mg(OH)2 (1, 10, 25, 50, 75, and 100 mg L−1) when incubated in a 20 °C temperature-controlled room with constant light. Some of those tests were conducted separately with different control waters. Those tests were carried out in different weeks. Therefore, different control treatments were applied with one control for 1–10 mg L−1 Mg(OH)2 treatments, one control for 50–75 mg L−1 Mg(OH)2 treatments, and one control for 100 mg L−1 Mg(OH)2 treatments.
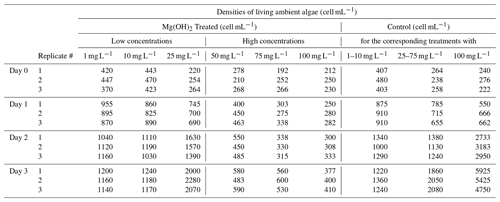
The raw data are presented in Appendix A for the Tetraselmis test, in Appendix B for the WET tests, and in Appendix C for the natural algal assemblage test.
SD established the study plan, collected all data for data analyses, and drafted the first version of this article. EH was involved in statistical analyses and writing of the manuscript in collaboration with all authors. TJN performed the laboratory experiments (both dispersion and regrowth phases) and recorded the biological and chemical analysis results. ALK was involved in the quality assurance of the final manuscript. AEDT was involved in implementations of the results and writing of the manuscript.
NIVA received funding from Negative Emissions Materials, Inc. (Claymont, DE, USA) to perform the study and from the Carbon to Sea Initiative (Washington, DC, USA) for the writing of this publication after a result disclosure agreement with Negative Emissions Materials, Inc. The Carbon to Sea Initiative is a non-profit organization with a mission to evaluate ocean-based CO2 removal pathways as desirable and feasible climate solutions. The authors have no other competing interests to declare.
Publisher’s note: Copernicus Publications remains neutral with regard to jurisdictional claims made in the text, published maps, institutional affiliations, or any other geographical representation in this paper. While Copernicus Publications makes every effort to include appropriate place names, the final responsibility lies with the authors.
This article is part of the special issue “Environmental impacts of ocean alkalinity enhancement”. It is not associated with a conference.
We would like to thank Evgeniy Yakushev (NIVA) for the development of the BROM model for magnesium hydroxide-specific application and Ana Catarina Almeida (NIVA) for their expertise during the test plan and experiment execution of bioassays and WET tests. In memory of Jeremy Ley (CEO of Negative Emissions Materials Inc.), whose contributions and enthusiasm have greatly impacted this work.
This research has been supported by EU Horizon 2020 (grant no. 869192 – NEGEM), the Windward Fund (Washington, DC, USA; master service agreement no. Windward-NOR16-MSA-2023), and Negative Emissions Materials, Inc. (see competing interests for more info).
This paper was edited by Kai G. Schulz and reviewed by three anonymous referees.
Bach, L. T., Gill, S. J., Rickaby, R. E., Gore, S., and Renforth, P.: CO2 removal with enhanced weathering and ocean alkalinity enhancement: potential risks and co-benefits for marine pelagic ecosystems, Front. Clim., 1, 7, https://doi.org/10.3389/fclim.2019.00007, 2019.
Burns, W. and Corbett, C. R.: Antacids for the sea? Artificial ocean alkalinization and climate change, One Earth, 3, 154–156, https://doi.org/10.1016/j.oneear.2020.07.016, 2020.
Butenschön, M., Lovato, T., Masina, S., Caserini, S., and Grosso, M.: Alkalinization scenarios in the Mediterranean Sea for efficient removal of atmospheric CO2 and the mitigation of ocean acidification, Front. Clim., 3, 614537, https://doi.org/10.3389/fclim.2021.614537, 2021.
Caserini, S., Storni, N., and Grosso, M.: The availability of limestone and other raw materials for ocean alkalinity enhancement, Global Biogeochem. Cy., 36, e2021GB007246, https://doi.org/10.1029/2021GB007246, 2022.
Chassot, E., Bonhommeau, S., Dulvy, N. K., Mélin, F., Watson, R., Gascuel, D., and Le Pape, O.: Global marine primary production constrains fisheries catches, Ecol. Lett., 13, 495–505, https://doi.org/10.1111/j.1461-0248.2010.01443.x, 2010.
Cripps, G., Widdicombe, S., Spicer, J., and Findlay, H. S.: Biological impacts of enhanced alkalinity in Carcinus maenas, Mar. Pollut. Bull., 71, 190–198, https://doi.org/10.1016/j.marpolbul.2013.03.015, 2013.
DOSI: Ocean Alkalinity Enhancement, Deep Ocean Stewardship Initiative Policy Brief, https://www.dosi-project.org/wp-content/uploads/Alkalinity-Enhancement-Policy-Brief.pdf (last access: 31 July 2024), 2022.
Ebenezer, V. and Ki, J. S.: Toxic Effects of Aroclor 1016 and Bisphenol A on Marine Green Algae Tetraselmis Suecica, Diatom Ditylum Brightwellii and Dinoflagellate Prorocentrum Minimum, The Korean Journal of Microbiology, 52, 306–312, https://doi.org/10.7845/KJM.2016.6050, 2016.
Fakhraee, M., Li, Z., Planavsky, N. J., and Reinhard, C. T.: A biogeochemical model of mineral-based ocean alkalinity enhancement: impacts on the biological pump and ocean carbon uptake, Environ. Res. Lett., 18, 044047, https://doi.org/10.1088/1748-9326/acc9d4, 2023.
GESAMP: High level review of a wide range of proposed marine geoengineering techniques, edited by: Boyd, P. W. and Vivian, C. M. G., IMO/FAO/UNESCO-IOC/UNIDO/WMO/IAEA/UN/UN Environment/UNDP/ISA Joint Group of Experts on the Scientific Aspects of Marine Environmental Protection, Rep. Stud. GESAMP No. 98, 144 pp., 2019.
Gomes, H. I., Mayes, W. M., Rogerson, M., and Stewart, D. I.: Alkaline residues and the environment: a review of impacts, management practices and opportunities, J. Clean. Prod., 112, 3571–3582, https://doi.org/10.1016/j.jclepro.2015.09.111, 2016.
Hartmann, J., Suitner, N., Lim, C., Schneider, J., Marín-Samper, L., Arístegui, J., Renforth, P., Taucher, J., and Riebesell, U.: Stability of alkalinity in ocean alkalinity enhancement (OAE) approaches – consequences for durability of CO2 storage, Biogeosciences, 20, 781–802, https://doi.org/10.5194/bg-20-781-2023, 2023.
Hill, A. V.: The possible effects of aggregation of the molecules of haemoglobin on its dissociation curves, J. Physiol., 40, IV–VII, 1910.
Ilyina, T., Wolf-Gladrow, D., Munhoven, G., and Heinze, C.: Assessing the potential of calcium-based artificial ocean alkalinization to mitigate rising atmospheric CO2 and ocean acidification, Geophys. Res. Lett., 40, 5909–5914, https://doi.org/10.1002/2013GL057981, 2013.
IMO (International Maritime Organization): resolution MEPC.300 (72) Code for approval of ballast water management systems (BWMS CODE), IMO, https://downloads.regulations.gov/EPA-HQ-OW-2019-0482-0624/attachment_10.pdf (last access: 31 July 2024), 2018.
ISO 10253: 2016. Water Quality – Marine algal growth inhibition test with Skeletonema costatum and Phaeodactylum tricornutum, International Standard Organization, 3rd Edn., 2016.
Jiang, L.-Q., Feely, R. A., Carter, B. R., Greeley, D. J., Gledhill, D. K., and Arzayus, K. M.: Climatological distribution of aragonite saturation state in the global oceans, Global Biogeochem. Cy., 29, 1656–1673, https://doi.org/10.1002/2015GB005198, 2015.
Kotai, J.: Oslo: Instructions for preparation of modified nutrient solution Z8 for algae, Norwegian Institute for Water Research, Oslo, Publication B-11/69, 1972.
Lee, J., Hong, S., An, S. A., and Khim, J. S.: Methodological advances and future directions of microalgal bioassays for evaluation of potential toxicity in environmental samples: A review, Environ. Int., 173, 107869, https://doi.org/10.1016/j.envint.2023.107869, 2023.
Li, J., Schiavo, S., Rametta, G., Miglietta, M. L., La Ferrara, V., Wu, C., and Manzo, S.: Comparative toxicity of nano ZnO and bulk ZnO towards marine algae Tetraselmis suecica and Phaeodactylum tricornutum, Environ. Sci. Pollut. Res., 24, 6543–6553, https://doi.org/10.1007/s11356-016-8343-0, 2017.
Moras, C. A., Bach, L. T., Cyronak, T., Joannes-Boyau, R., and Schulz, K. G.: Ocean alkalinity enhancement – avoiding runaway CaCO3 precipitation during quick and hydrated lime dissolution, Biogeosciences, 19, 3537–3557, https://doi.org/10.5194/bg-19-3537-2022, 2022.
NASEM (National Academies of Sciences, Engineering, and Medicine): A Research Strategy for Ocean-based Carbon Dioxide Removal and Sequestration, Washington, DC, The National Academies Press, https://doi.org/10.17226/26278, 2021.
NSF: International Generic Protocol for the Verification of Ballast Water Treatment Technology, U.S. Environmental Protection Agency, Washington, DC, EPA/600/R-10/146, https://cfpub.epa.gov/si/si_public_record_report.cfm?Lab=NRMRL&dirEntryId=230926 (last access: 31 July 2024) 2010.
Pathak, M., Slade, R., Shukla, P. R., Skea, J., Pichs-Madruga, R., Ürge-Vorsatz, D.: Technical Summary, in: Climate Change 2022: Mitigation of Climate Change. Contribution of Working Group III to the Sixth Assessment Report of the Intergovernmental Panel on Climate Change, edited by: Shukla, P. R., Skea, J., Slade, R., Al Khourdajie, A., van Diemen, R., McCollum, D., Pathak, M., Some, S., Vyas, P., Fradera, R., Belkacemi, M., Hasija, A., Lisboa, G., Luz, S., and Malley, J., Cambridge University Press, Cambridge, UK and New York, NY, USA, https://doi.org/10.1017/9781009157926.002, 2022.
Pauly, D. and Christensen, V.: Primary production required to sustain global fisheries, Nature, 374, 255–257, https://doi.org/10.1038/374255a0, 1995.
Petersen, K., Heiaas, H. H., and Tollefsen, K. E.: Combined effects of pharmaceuticals, personal care products, biocides and organic contaminants on the growth of Skeletonema pseudocostatum, Aquat. Toxicol., 150, 45–54, 2014.
Renforth, P. and Henderson, G.: Assessing ocean alkalinity for carbon sequestration, Rev. Geophys., 55, 636–674, https://doi.org/10.1002/2016RG000533, 2017.
Seoane, M., Rioboo, C., Herrero, C., and Cid, A.: Toxicity induced by three antibiotics commonly used in aquaculture on the marine microalga Tetraselmis suecica (Kylin) Butch, Mar. Environ. Res., 10, 1–7, https://doi.org/10.1016/j.marenvres.2014.07.011, 2014.
Siegel, D. A., DeVries, T., Doney, S., and Bell, T.: Assessing the sequestration time scales of some ocean-based carbon dioxide reduction strategies, Environ. Res. Lett., 16, 104003, https://doi.org/10.1088/1748-9326/ac0be0, 2021.
Steinberg, M. K., Lemieux, E. J., and Drake, L. A.: Determining the viability of marine protists using a combination of vital, fluorescent stains, Mar. Biol., 158, 1431–1437, 2011.
Tollefson, J.: Start-ups are adding antacids to the ocean to slow global warming. Will it work?, Nature, 618, 902–904, https://doi.org/10.1038/d41586-023-02032-7, 2023.
USGC (United States Coast Guard): Ballast Water Management System Specifications and Approvals. Title 46 – Shipping, Subpart 162.060, 2012.
Vagi, M. C., Kostopoulou, M. M., Petsas, A. S., Lalousi, M. E., Rasouli C. H., and Lekkas, T. D.: Toxicity of organophosphorous pesticides to the marine alga Tetraselmis suecica, Global NEST J., 7, 222–227, 2005.
Vindimian, E., Robaut, R., and Fillion, G.: A method for co-operative and non-cooperative binding studies using non-linear regression analysis on a microcomputer, J. Appl. Biochem., 5, 261–268, 1983.
Wee, J. L., Millie, D. F., Nguyen, N. K., Patterson, J., Cattolico, R. A., John, D. E., and Paul, J. H.: Growth and biochemical responses of Skeletonema costatum to petroleum contamination, J. Appl. Phycol., 28, 3317–3329, 2016.
Yakushev, E. V., Protsenko, E. A., Bruggeman, J., Wallhead, P., Pakhomova, S. V., Yakubov, S. Kh., Bellerby, R. G. J., and Couture, R.-M.: Bottom RedOx Model (BROM v.1.1): a coupled benthic–pelagic model for simulation of water and sediment biogeochemistry, Geosci. Model Dev., 10, 453–482, https://doi.org/10.5194/gmd-10-453-2017, 2017.
Yang, B., Leonard, J., and Langdon, C.: Seawater alkalinity enhancement with magnesium hydroxide and its implication for carbon dioxide removal, Mar. Chem., 253, 104251, https://doi.org/10.1016/j.marchem.2023.104251, 2023.
- Abstract
- Introduction
- Methods
- Results
- Discussion
- Conclusion
- Appendix A: Raw data for the Tetraselmis bioassay studies
- Appendix B: Raw data for the WET tests
- Appendix C: Raw data for the natural algal assemblage tests
- Data availability
- Author contributions
- Competing interests
- Disclaimer
- Special issue statement
- Acknowledgements
- Financial support
- Review statement
- References
- Abstract
- Introduction
- Methods
- Results
- Discussion
- Conclusion
- Appendix A: Raw data for the Tetraselmis bioassay studies
- Appendix B: Raw data for the WET tests
- Appendix C: Raw data for the natural algal assemblage tests
- Data availability
- Author contributions
- Competing interests
- Disclaimer
- Special issue statement
- Acknowledgements
- Financial support
- Review statement
- References