the Creative Commons Attribution 4.0 License.
the Creative Commons Attribution 4.0 License.
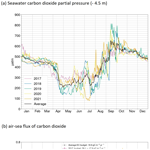
Interannual and seasonal variability of the air–sea CO2 exchange at Utö in the coastal region of the Baltic Sea
Mika Aurela
Juha Hatakka
Lumi Haraguchi
Sami Kielosto
Timo Mäkelä
Jukka Seppälä
Simo-Matti Siiriä
Ken Stenbäck
Juha-Pekka Tuovinen
Pasi Ylöstalo
Lauri Laakso
Oceans alleviate the accumulation of atmospheric CO2 by absorbing approximately a quarter of all anthropogenic emissions. In the deep oceans, carbon uptake is dominated by aquatic phase chemistry, whereas in biologically active coastal seas the marine ecosystem and biogeochemistry play an important role in the carbon uptake. Coastal seas are hotspots of organic and inorganic matter transport between the land and the oceans, and thus they are important for the marine carbon cycling. In this study, we investigate the net air–sea CO2 exchange at the Utö Atmospheric and Marine Research Station, located at the southern edge of the Archipelago Sea within the Baltic Sea, using the data collected during 2017–2021. The air–sea fluxes of CO2 were measured using the eddy covariance technique, supported by the flux parameterization based on the pCO2 and wind speed measurements. During the spring–summer months (April–August), the sea was gaining carbon dioxide from the atmosphere, with the highest monthly sink fluxes typically occurring in May, being −0.26 µmol m−2 s−1 on average. The sea was releasing the CO2 to the atmosphere in September–March, and the highest source fluxes were typically observed in September, being 0.42 µmol m−2 s−1 on average. On an annual basis, the study region was found to be a net source of atmospheric CO2, and on average, the annual net exchange was 27.1 gC m−1 yr−1, which is comparable to the exchange observed in the Gulf of Bothnia, the Baltic Sea. The annual net air–sea CO2 exchanges varied between 18.2 (2018) and 39.1 gC m−1 yr−1 (2017). During the coldest year, 2017, the spring–summer sink fluxes remained low compared to the other years, as a result of relatively high seawater pCO2 in summer, which never fell below 220 µatm during that year. The spring–summer phytoplankton blooms of 2017 were weak, possibly due to the cloudy summer and deeply mixed surface layer, which restrained the photosynthetic fixation of dissolved inorganic carbon in the surface waters. The algal blooms in spring–summer 2018 and the consequent pCO2 drawdown were strong, fueled by high pre-spring nutrient concentrations. The systematic positive annual CO2 balances suggest that our coastal study site is affected by carbon flows originating from elsewhere, possibly as organic carbon, which is remineralized and released to the atmosphere as CO2. This coastal source of CO2 fueled by the organic matter originating probably from land ecosystems stresses the importance of understanding the carbon cycling in the land–sea continuum.
- Article
(4588 KB) - Full-text XML
- BibTeX
- EndNote
Carbon dioxide (CO2) is the key greenhouse gas driving the global climate change and ocean acidification (Feely et al., 2009). The CO2 exchange between the atmosphere and land ecosystems has been extensively studied during the recent decades (Baldocchi et al., 2001; Pastorello et al., 2020). However, to fully understand the global carbon cycling, it is crucial to recognize the role of the oceans, which absorb 26 % of the anthropogenic CO2 emissions (Friedlingstein et al., 2022). Direct measurements of the atmospheric CO2 concentrations and the terrestrial ecosystem fluxes have long traditions across the Earth (Keeling et al., 1976; Baldocchi et al., 2001; Heiskanen et al., 2022), while the logistical and technical challenges have hindered the measurements in the oceans until the last few decades (Bakker et al., 2016; Sloyan et al., 2019).
Approximately half of the net primary production in the Earth takes place in the oceans (Field et al., 1998), which cover 71 % of the Earth's surface. Thus, they are globally the largest ecosystem contributing to the global carbon balance. Solubility and biological pumps effectively transport carbon to the deeper ocean (Volk and Hoffert, 1985). The solubility pump is governed by the large-scale oceanic circulation, which transports water masses to the polar regions, where these water masses cool, absorb atmospheric CO2 due to increased solubility, and sink into the deeper ocean. The biological pump is related to the synthesis of organic carbon in the surface waters and its export to the deeper ocean. On average, the oceans are sinks of CO2, but the air–sea CO2 exchange is highly variable (Takahashi et al., 2002). In coastal areas, a large amount of matter is transported between the land, the oceans, and the atmosphere. Approximately 40 % of the oceanic carbon sequestration through the biological carbon pump occurs in the coastal seas (Muller-Karger et al., 2005).
The CO2 exchange between the atmosphere and the sea (Fas) is driven by the CO2 partial pressure (pCO2) difference between the sea and the atmosphere (sea–atmosphere), and it can be expressed using the temperature–salinity-driven solubility factor of the CO2 (K0) and the gas transfer velocity (k):
Thus, the convention used here is the positive fluxes denoting exchange from the sea to the atmosphere. Several processes affect the efficiency of the gas transfer, such as microscale wave breaking, turbulence, bubbles, sea spray, rain, waves, and surface films (Garbe et al., 2014, p. 56). Typically the gas transfer velocity has been parameterized using wind speed (Wanninkhof, 1992; Wanninkhof et al., 2009; Wanninkhof, 2014).
The Baltic Sea is one of the biggest brackish water basins in the world. This shallow sea, with a mean depth of 54 m, has strong seasonality in temperature (approximately 0–20 °C) and in primary production, being affected by large terrestrial input of organic and inorganic carbon (Räike et al., 2012). Eutrophication is one of the biggest environmental concerns for the Baltic Sea (Andersen et al., 2017), resulting in harmful algae blooms (Kahru and Elmgren, 2014; Kraft et al., 2021) and oxygen depletion in deep waters (Carstensen et al., 2014). In the Baltic Sea, the pCO2 in the seawater is regulated by the interplay between biological, chemical, and physical processes (Omstedt et al., 2009). The net CO2 balance for the whole Baltic Sea has been estimated to be close to neutral (Kuliński et al., 2014; Ylöstalo et al., 2016), but the estimates for its different basins show large variation. Typically, the southern parts of the Baltic Sea act as a sink of CO2 due to their strong primary production (Kuliński and Pempkowiak, 2011; Kuss et al., 2006), whereas the northern part has lower primary production and receives a large amount of organic matter, which remineralizes into inorganic carbon, making this area a source of CO2 (Algesten et al., 2006).
In addition to the spatial variability, the net air–sea exchange of CO2 at a given location in the Baltic Sea also experiences large seasonal variability (Rutgersson et al., 2008) and likely also interannual variability. Our understanding of these variations is still incomplete, and understanding the physical, biological, and chemical constraints on the Baltic Sea carbon cycle requires sustained high-frequency measurements. By using daily FerryBox pCO2 data, Schneider et al. (2014) found the central Baltic Sea to act as a weak sink of the atmospheric CO2, the annual exchange varying within −7 to −11 gC m−2 yr−1. In contrast, Wesslander et al. (2010) used long data series of monthly monitoring data of total alkalinity and pH to calculate the seawater pCO2 and the air–sea CO2 fluxes and found the central Baltic Sea to mainly act as a source of atmospheric CO2, the annual balance varying from close to zero to up to almost 50 gC m−2 yr−1.
The calculation of the air–sea CO2 exchange is typically based on the pCO2 data and wind-speed-dependent parameterization of the gas transfer velocity (Eq. 1). For the coastal seas, direct high-frequency measurements are needed for the reliable estimation of the net CO2 exchange at a given location. For example, monthly cruises can be too infrequent to detect the rapid variations of pCO2 in the Baltic Sea (Kuss et al., 2006; Honkanen et al., 2021). Also, the calculation of the seawater pCO2 from other carbonate system variables introduces some uncertainty in the pCO2 estimate (Steinhoff, 2020). The eddy covariance (EC) technique provides means for directly measuring the instantaneous fluxes of matter and energy between the surface and the atmosphere. However, long-term measurements are technically challenging in a marine environment, and the EC method has been applied only at few locations in the Baltic Sea for measuring the air–sea CO2 fluxes. Currently, continuous long-term air–sea CO2 EC flux measurements only take place at Östergarnsholm (Rutgersson et al., 2008) and Utö (Honkanen et al., 2018).
The aim of this study was to determine the annual CO2 net exchange between the sea and the atmosphere and its seasonal and interannual variability in the Archipelago Sea, a part of the Baltic Sea. Time series of the EC flux data, supported with parameterized gas transfer velocity and measured pCO2 gradient, gathered at Utö within 2017–2021 were used for the determination of the net exchange of CO2. Physical and biological measurements provide background to explain the reasons for seasonal and annual variations in the flux data.
2.1 Study site
The Utö Atmospheric and Marine Research Station (Fig. 1) is located at the outer edge of the Archipelago Sea of the Baltic Sea (59°46′55′ N, 21°21′27′′ E). The Archipelago Sea is bordered by the southwestern coast of Finland, the Åland Sea, the Bothnian Sea, and the Baltic Proper. This area comprises thousands of small islands and provides an important gateway for the water exchange between the Baltic Proper and the Bothnian Sea (Miettunen et al., 2024). Utö is the southernmost permanently inhabited island of Finland, with a few dozen permanent inhabitants throughout the year. The vegetation on this small rocky island (0.8 km2) with few trees is mainly bushes and shrubs (Kilkki et al., 2015), especially on the western side of the island. Southwest of the island, the sea quickly deepens to 80 m (Honkanen et al., 2018), starting the open seas of the northern Baltic Proper. Generally, temperature in the surface waters at Utö varies within 0–18 °C (Laakso et al., 2018). Sea ice is observed at Utö every few years (Jevrejeva et al., 2004; Laakso et al., 2018). The surface salinity at Utö varies typically between 6–7 PSU (Laakso et al., 2018). The flow patterns in the Archipelago Sea contain large variations in time and space (Erkkilä and Kalliola, 2004).
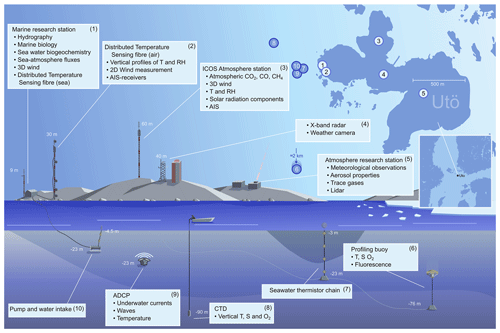
Figure 1Location, map, and scientific measurements of Utö, which include (1) the marine research station and the micrometeorological flux tower next to it, (2) optical temperature fiber tower, (3) the Integrated Carbon Observing System (ICOS) atmosphere station, (4) X-band radar, (5) atmosphere research station, (6) acoustic Doppler current profiler, (7) conductivity–temperature–depth vertical profiling, (8) seawater thermistor chain, (9) Profiling buoy, and (10) submerged pump system.
The island of Utö has a long history of measurements, as the earliest meteorological and hydrographic observations date back to 1881 and 1900, respectively (Laakso et al., 2018). The Utö Atmospheric and Marine Research Station is a comprehensive atmospheric and marine observing system combining continuous long-term observations of the atmosphere, sea physical state, biogeochemistry, and marine ecosystem dynamics. Recently, the site has been developed also towards a marine radar and radio signal research supersite (Rautiainen et al., 2023). The Utö station is run in co-operation between the Finnish Meteorological Institute and the Finnish Environment Institute, comprising a variety of research facilities within and around the island of Utö. It is part of the Joint European Research Infrastructure for Coastal Observatories (JERICO), the Finnish Marine Research Infrastructure (FINMARI), and recently the Aerosol, Clouds and Trace Gases Research Infrastructure (ACTRIS). Also, there is an Integrated Carbon Observing System (ICOS) atmosphere station, measuring atmospheric carbon constituents, on the island. The observing system used in the study is described in the following subsections. In this study, we used the 30 min averaged observations relevant for the air–sea CO2 exchange during 2017–2021.
2.2 Flow-through system
Continuous observations of seawater properties are based on a flow-through system, similar to those on many FerryBox systems (Rantajärvi et al., 2003; Petersen et al., 2011), with a large number of parameters measured (Fig. 2). A submerged borehole pump (Grundfos SP3A-9N) is located approximately 250 m west of the island at the bottom of the sea, at a depth of 23 m ±0.5 m (Honkanen et al., 2021; Kraft et al., 2021), next to an acoustic Doppler current profiler and a thermistor chain. A sample water inlet system, equipped with a thermistor-depth sensor, is floating above the pump at a depth of 4.5 m ±0.5 m. The flow of approximately 55 L min−1 is led to a manifold, located at the marine station, which supplies the water to different instruments for the analysis. The residence time (on average 6 min) depends on the flow rate and is taken into account in the measurement time. Sensors, their flow caps, and tubes connecting them are automatically cleaned once a day using detergent (Triton X-100), supplemented with a weekly to semiweekly manual cleaning of sensors. The submersible pump and the underwater pressure pipe are renewed annually.
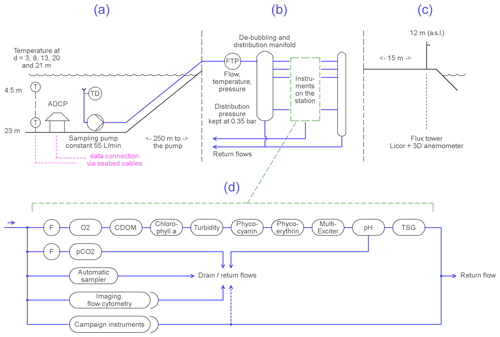
Figure 2Structure of the flow-through system of the marine station. A submersible pump (a) transports sample water to the station (b), where the manifold distributes the water to different sensors, such as the thermosalinograph, TSG (d). The flow rates (f) to the sensors are controlled to be approximately constant. The flux tower (c) is found next to the marine station. TD denotes a standalone temperature–depth sensor, attached to the inlet. CDOM stands for colored dissolved organic matter, and O2 stands for dissolved oxygen.
Temperature and salinity were observed using a thermosalinograph (SBE45 MicroTSG, Seabird Scientific) using a logging interval of 15 s, connected to the flow-through system on the marine station. This measurement was supported by regular CTD (conductivity–temperature–depth) sampling close to the sample water inlet. Also, a standalone temperature–depth sensor (Star-Oddi Tilt and Compass), attached directly to the inlet, measured the in situ temperature, logging data every 10–15 s. These additional observations were used for correcting the temperatures measured with the thermosalinograph, which were affected by the heat exchange between the seawater inside the pipe and the surroundings of the pipe, depending on the temperature structure of the water column.
The partial pressure of carbon dioxide (pCO2) was measured using a SuperCO2 system (Sunburst Sensors) that was connected to the flow-through system (Honkanen et al., 2018). The CO2 of the sample water stream was equilibrated with the sample air in a double shower-head equilibrator chamber. This sample air was directed to a non-dispersive infrared gas analyzer (LI-840A, LI-COR) for the detection of CO2 molar fraction (logging 15 s), which was used for the calculation of pCO2, following the guidelines of Dickson et al. (2007). The sample water may have exchanged the heat with its surroundings during the transport, and thus the pCO2 data were corrected for the temperature effect using the T–pCO2 dependence given by Takahashi et al. (1993).
The drift error of the gas analyzer was compensated using a correction function, which was based on the measurement of four reference gases with differing CO2 molar fractions (0, 234, 397, and 993 ppm CO2) every 4 h. These gases were verified using a cavity ring-down spectroscopy analyzer (Picarro G2401), which was calibrated against gases from the National Oceanic and Atmospheric Administration (NOAA).
A similarly operating SuperCO2 system was tested in the first ICOS Ocean Thematic Centre pCO2 instrument intercomparison workshop. The root mean square error between the SuperCO2 and the reference setup was estimated to be 4.4 µatm (data not published). The effect of the long inlet tube on the pCO2 measurement at Utö was verified to be small (approximately 4 µatm) by using several standalone sensors placed in the inlet location and in a tank connected to the manifold (Honkanen et al., 2021).
The flow-through system was offline during spring 2017 (24 March–31 March), early summer 2018 (1 May–2 July), and summer 2020 (15 July–30 July) due to the pump failure, in addition to the shorter maintenance periods. The infrared source of the gas analyzer of the pCO2 system was malfunctioning during spring 2020 (31 March–26 May).
Chlorophyll a was continuously measured with a WETLabs ECO FLNTU fluorometer (Sea-Bird Scientific), logging data every 15 s, which was connected to the flow-through system. During the study period, we took discrete water samples for laboratory analysis of chlorophyll a, following sampling, extraction, and measurement protocols given in HELCOM (2016). Chlorophyll-a fluorescence was adjusted to represent chlorophyll-a concentrations (µg L−1) using the linear relationship observed between fluorescence intensity and measured concentrations (R2=0.76, n=159).
Inorganic nutrients were analyzed from the bottle samples collected from the flow-through system and frozen at −20 °C until analysis. These samples were typically collected at least monthly and during specific measurement campaigns multiple times per a day. The nitrate, nitrite, phosphate, and silicate concentrations were analyzed using a Lachat Quikchem 8000 flow injection analyzer (until October 2020) and a Skalar SAN++ continuous flow analyzer (from October 2020 onward) following Grasshoff et al. (1999). In this study, we were mainly concerned with oxidized forms of nitrogen (as a sum of nitrate and nitrite, referred to in the text simply as nitrate) and phosphate concentrations.
2.3 Sea–air CO2 flux
The exchange of CO2 between the sea and the atmosphere was measured using the eddy covariance (EC) method, using a 9 m tall micrometeorological flux tower, 12 m a.s.l. (meters above sea level), on the western shore of the island. The setup consists of a closed-path non-dispersive infrared gas analyzer (LI-7000, LI-COR), connected to a 30 cm long sample air dryer (PD-100T-12-MKA, Perma Pure). The EC fluxes were calculated for each 30 min period. The CO2 molar fractions observed with the flux tower were regularly calibrated with the zero (0 ppm CO2) and span (396 ppm CO2) reference gases, in addition to the atmospheric ICOS observations at Utö (Kilkki et al., 2015). The measured EC fluxes were corrected for the flux loss occurring in the system, which mostly originates from the attenuation of CO2 molar fraction fluctuations in the tubing. For this purpose, a cospectrum between the vertical wind speed and CO2 molar fractions was compared with the cospectrum between the vertical wind speed and the (sonic) temperature, in order to produce a frequency-dependent transfer function. More information about this method is given in Honkanen et al. (2018).
The EC method was supported by flux gap filling for those periods, when reliable EC data were not available due to flux footprint originating from the island (outside of the 190–350° wind sector), non-stationary flux conditions, or CO2 molar fraction disturbance by ships passing the flux footprint area. The EC data were considered appropriate 26 % of the time, and the remaining periods were gap-filled as described below. Approximately 60 % of the time, the wind at Utö blows from an appropriate direction suitable for the air–sea exchange measurements (Honkanen et al., 2018). Thus, the reminder of the data removal is caused by the non-stationarity, ship interference, or instrument maintenance or malfunctions. Based on the categorization by Rutgersson et al. (2020), the wind sector 180–260° represents an open-sea footprint area, while the wave field of the sector 260–350° may be disturbed slightly by distant islands (Honkanen et al., 2018). The EC fluxes during non-stationary conditions were discarded if the 30 min flux differed more than 60 % from the mean of the corresponding 5 min fluxes. Since there exists a seaway within the flux footprint area, we applied an empirical variance restriction for the EC data to avoid interference from ship traffic. We did not use the EC data if the variance of the CO2 molar fractions exceeded 0.50 ppm2 during the flux calculation period.
A flux parameterization based on wind speed and pCO2 measurement was used for the gap filling of the EC data during the periods when the EC fluxes were not available due to strict quality control. The gas transfer velocity was parameterized using a combination of a linear and quadratic relationship of 10 m potential wind speed, U10, which was measured at the weather station on the island, instead of using solely quadratic fits, which are commonly used (Wanninkhof, 1992, 2014). The relationship used for the normalized gas transfer velocity, k660 (the k which is corrected to the Schmidt number in 20 °C and 35 PSU), in this paper was determined for Utö based on the data from 2017–2021, by fitting the function to the 2 m s−1 wind speed median bins. This parameterization is site-specific, due to for instance the wind fetch and the bottom geometry, and slightly differs from the one given for the open-ocean conditions, (Wanninkhof, 2014). The effects of our choice on the budgets and other information on the flux measurements are given in Appendix A.
To fill in the measurement gaps in pCO2 and in the air–sea CO2 flux data, we reconstructed the pCO2 time series during the gaps using the flux equation (Eq. 1) inversely, i.e., based on the directly measured eddy covariance fluxes and the wind-speed parameterization of gas transfer velocity. As this method may produce artificial scatter, these reconstructed pCO2 values were averaged with a 7 d running arithmetic mean filter. The pCO2 data were available 85 % of time, and thus the reconstructed and interpolated data were used 15 % of the time.
2.4 Other measurements
Vertical structure of the water column was assessed using the RBR XR-620 CTD (conductivity–temperature–density) castings from a small boat northwest of Utö, where the water depth reaches 90 m. These measurements were done approximately every 10 d during summer and less frequently in winter. The depth of the strongest temperature gradient (thermocline) was determined from each cast used for the interpretation of the mixed-layer depth. If the highest density gradient was less than 0.1 °C m−1, the water column was considered to be fully mixed.
Meteorological observations, including wind speed and direction and air temperature, were measured at Utö's atmosphere research station, located in the eastern part of the island. The wind measurement took place at approximately 25 m a.s.l. on relatively flat rock and bush terrain. In this study, 10 m potential wind speeds, calculated assuming the logarithmic wind profile, were used (Aaltonen, 2021). The solar irradiation was measured using a pyranometer (Delta-T SPN1) placed on the roof of a hotel in the northern part of the island, next to the ICOS atmosphere station.
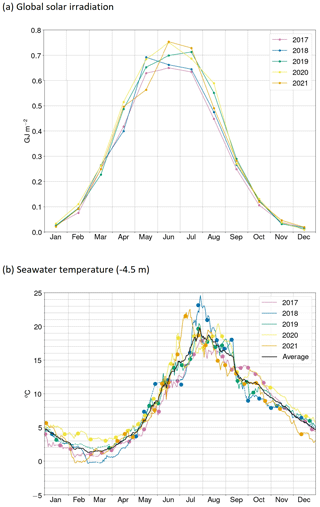
Figure 3(a) Monthly sums of the global solar irradiation at Utö during 2017–2021 and (b) daily averages of the seawater temperature at a depth of approximately 4.5 m at Utö for the years 2017–2021. The solid line shows the inlet temperature measured using the standalone sensor attached to the inlet for May 2019–May 2021; for other times, the solid line is a thermosalinograph temperature that is shift-corrected based on the CTD measurements at 4–5 m depth. The interpolated values are indicated by the dashed lines. The CTD temperatures for the depth 4–5 m are the indicated by large dots.
3.1 Solar irradiation
Solar irradiation is governed by the annual cycle and the cloudiness. Generally, solar irradiance increased from almost negligible in January to a maximum in June, with a solar irradiance monthly sum of ca. 0.7 GJ m−2 (Fig. 3). The annual solar irradiance sums of 2017 and 2018 were low, 3.54 and 3.69 GJ m−2, respectively, compared to the other measured years with 3.86–4.08 GJ m−2. Especially in spring–summer of 2017 and 2018, solar irradiation was low compared to other years, with the exception of May 2018. The spring and summer months had plenty of light in 2019–2021, except for May and August 2021.
3.2 Sea surface temperature
At Utö, the seawater temperature at approximately 4.5 m depth followed the annual cycle of solar irradiance (Fig. 3), with a delay. The temperature minima, on average 1.3 °C, occurred in February–March, whereas the temperature maxima, on average 19.7 °C, were reached in July–August. Typically around September, the water column got fully mixed, with a rapid cooling of surface water.
During the study period, the coolest summer (June–August) surface layer was observed in 2017, when the annual maximum temperature reached only 18.4 °C in late July. The temperature remained up to approximately 2 °C below the 5-year average for most of the year, with the exception of September–October 2017, when the cooling was slightly lower than for other years.
The year 2018 was the warmest of those studied, in terms of the summer peak temperatures. The early spring (March–April) of 2018 was, however, still relatively cold, and there was occasional on–off sea ice in March, with highly variable ice coverage. In July, the sea heated quickly from a relatively low temperature, 13 °C, to the highest temperature observed during the study period, 25 °C. The sea cooled quickly in September 2018, and the surface temperature in October was 2 °C below the average.
The temperature of the surface layer in 2019 followed the 5-year average closely. Throughout the year 2019, the monthly seawater temperatures differed less than 1 °C from the average. The annual minimum of 1.5 °C was observed in early March and the maximum of 21.3 °C in late July.
The year 2020 started particularly warm, the surface seawater remaining above 2.9 °C throughout January–March 2020. The summer of 2020 was characterized by several strong and quick temperature fluctuations. A quick early increase in temperature to 20.3 °C was observed in June 2020, but soon the temperature quickly fell to 13.6 °C. The annual maximum of 20.6 °C was observed relatively late, in late August.
In February 2021, there was varying thin on–off sea ice for 2 weeks. The year 2021 had the second warmest summer, and the temperature increased up to 23.5 °C in mid-July. This maximum took place half a month earlier than the one in 2018. After late summer 2021, the sea surface remained mostly colder than the 5-year average.
3.3 Temperature vertical profile
The thermocline, which was determined from semi-regular CTD casts, is used here to represent the depth of the mixing surface layer, which is separated from the underlying deep water. Typically, a distinct thermocline started to develop in the spring as a result of increased solar irradiance (Fig. 4), which heated the surface waters. Typically, a very shallow (2–5 m) and short-term mixed layer formed in May. The infrequent CTD casts do not reveal the exact duration of such shallow layers. Most likely, mixed-layer depths shallower than 5 m lasted only a short period of time, as these were not observed in two subsequent CTD casts, which were done in summer approximately every 10 d. In May 2018 and May 2021, strong temperature gradients of old winter layers were observed, at 37 and 57 m, respectively. The surface layers gradually deepened due to the wind-induced mixing as the summer progressed, and the mixed layer typically reached depths of 15–20 m. The summer thermocline of 2020 stayed relatively shallow from the late spring to late summer (May–August), and it never reached below 20 m, whereas the summer thermocline depth of 2017 peaked at 35 m in the beginning of June and at 32 m in the beginning of July. In 2017, the mixed surface layer reached 50–80 m already in September–October, in contrast to other years, and thus relatively warm waters, approximately 12 °C, reached such deep layers. Eventually, the overturning due to the water reaching the maximum density temperature caused the water column to be fully mixed in early winter.
3.4 Salinity
Salinity is a good indicator of the change of water masses; for instance in the pelagic conditions of the Baltic Sea, salinity correlates well with total alkalinity (Müller et al., 2016). In the coastal regions, salinity shifts may indicate riverine impact and upwelling. The salinity (measured at a depth of approximately 4.5 m) at Utö typically reached its minimum in late summer, 6.1 on average (Fig. 5). After that, salinity steadily increased to the maximum met at the turn of the year, 6.8 on average. In late winter or early spring of some of the years, the salinity showed large interannual variations, and relatively large drops were observed in February 2021, as salinity fell to 5.2, and in March 2018 to 5.7. Large drops in salinity indicate freshwater intrusion. As they seem to occur during late winter or early spring, they are possibly a result of melting snow or ice.
3.5 Chlorophyll-a fluorescence
The phytoplankton biomass at Utö, inferred from the chlorophyll-a (Chl-a) fluorescence data validated with laboratory measurements, showed seasonal variability with a range from 0.5 to 10 µg L−1 (Fig. 6). Spring bloom formed in April, due to increased irradiance levels and water column stratification and being fueled by inorganic nutrients accumulated during winter. In 2017 spring bloom peaked very early, before mid-April, although we had some gaps in measurements. The spring bloom of 2018 stands out from the 5-year average: the Chl-a fluorescence of April 2018 was twice as high as the 5-year average for April. The spring bloom in 2020 was very weak and delayed until May. Spring blooms typically declined in May, with the exception of 2019, which showed a secondary peak of Chl-a after mid-May. Additional summer blooms were seen in July–August. The algal dynamics are controlled by multiple factors, such as solar irradiation, wind, and temperature; for example, calm and warm weather favors cyanobacterial growth (Kraft et al., 2021).
3.6 Nutrient concentrations
The seasonal dynamics of nutrient concentrations were controlled by the production, remineralization, and mixing. The levels of nitrate and phosphate at a depth of 4.5 m reached their annual maxima in February–March, before the spring bloom (Fig. 7). For both of these inorganic nutrients, the highest pre-spring levels were observed in 2018 and 2021, approximately 8–10 µmol L−1 for nitrate, while the pre-spring nutrient levels for the year 2020 were low, less than 6 µmol L−1 for nitrate, compared to the 5-year average. The pre-spring nutrient data for Utö in the year 2017 were not available. However, based on the early April 2017 data, the nutrient inventories had been depleted earlier than in other years.
The nitrate levels at Utö reached a minimum (0.5 µmol L−1) in May and predominantly stayed this low until September. However, the nitrate levels in 2020 increased to 1.7 µmol L−1 in June–July. In autumn, all years showed a similar increase in the nitrate.
Similarly to nitrate, the phosphate concentrations in July 2020 deviated from other years as the monthly average increased to 0.5 µmol L−1. The phosphate levels reached their minima in August with the monthly average of 0.2 µmol L−1. The lowest concentrations were observed during July–August 2021. In autumn, the phosphate inventories filled up.
3.7 Wind speed
Wind is an important factor enhancing the turbulence in the air–sea interface, and thus it plays an important role in the deepening of the surface layer and in the gas transfer between the sea and the atmosphere. The highest wind speeds occurred during the wintertime, October–February, monthly averages being 8.0 m s−1, and the lowest monthly wind speeds, on average 5.3 m s−1, took place in summer, May–June. The late winter of 2019–2020 was relatively windy, as the monthly averages for January and February were 9.6 m s−1. The lowest monthly mean of wind speed (4.5 m s−1) was observed in May 2021. As the gas transfer velocity has typically been parameterized using a quadratic relation of wind speed (Wanninkhof, 2014), small changes from the 5-year average may prove to be important for enhancing or suppressing the air–sea CO2 flux. For instance, calm weather in late 2019 likely restricted the release of CO2 to the atmosphere.
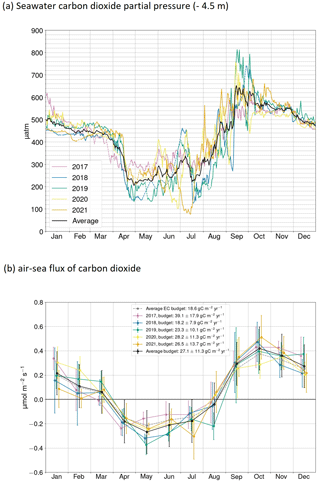
Figure 9(a) Daily means of the CO2 partial pressure in the seawater at a depth of 4.5 m at Utö in 2017–2021. (b) Monthly average CO2 air–sea fluxes: the 5-year average fluxes constructed using both eddy covariance and parameterization (black), the 5-year average eddy covariance fluxes (gray), and the fluxes for different years using both eddy covariance and parameterization (other colors). The error estimates are explained in the Appendix.
3.8 CO2 partial pressure
The partial pressure of CO2 in the seawater (Fig. 9) generally followed the annual cycle primarily governed by the photosynthetic carbon fixation. In spring, when the solar irradiance increased and water column started to be stratified, increased phytoplankton production transformed the inorganic carbon into organic carbon, resulting in a drawdown of the pCO2. On average, the pCO2 reached a minimum of 210 µatm in late April. The spring bloom was typically terminated by the depletion of inorganic nitrogen (Fig. 7). In 2017, pCO2 drawdown started approximately a week earlier than in other years, and also the peak of spring bloom took place earlier. The lowest spring pCO2 was observed in 2018 and 2019, concomitantly with the most intense spring blooms.
The pCO2 generally increased from late April (210 µatm) to early July (340 µatm), after which it experienced another drawdown to 220 µatm. This late-summer drawdown showed some temporal variation, taking place from late June to late July. The lowest half-hourly pCO2 value (58 µatm) recorded during 2017–2021 occurred in mid-July 2021. During the summer months of 2017, the daily averages of pCO2 remained relatively high compared to other years, not falling below 220 µatm.
In late summer, from August onwards, the solar irradiance started to diminish, decreasing the photosynthesis rate, and the respiration of organic matter started to prevail, which was observed as increasing pCO2. This effect was enhanced by the breakdown of the stratification of the water column. In August–September, pCO2 generally increased almost linearly to 630 µatm, where the annual maxima were met. In September 2019, pCO2 reached the highest recorded pCO2 during the study period, 877 µatm (half-hourly value). After this, pCO2 decreased slowly until the spring, approaching the atmospheric CO2 partial pressure.
3.9 Air–sea CO2 flux
The CO2 fluxes between the sea and the atmosphere at Utö (Fig. 9) followed an annual cycle governed by the pCO2. During the spring–summer (approximately April–August) when the seawater pCO2 was below the atmospheric partial pressure of CO2, the sea was a sink of atmospheric carbon dioxide. The sea was a source of CO2 during the time between autumn and spring. The highest average negative (from the atmosphere to the sea) monthly flux (−0.3 µmol m−2 s−1) occurred in May, which coincided with the lowest average monthly pCO2. In May–July 2017, the monthly fluxes remained clearly below the corresponding 5-year averages. The highest positive fluxes (0.4 µmol m−2 s−1) occurred in October, which was also the month with the highest pCO2 values. In winter, the wind speeds were typically higher than in summer, enhancing the gas transfer. As the CO2 partial pressure difference between the sea and the atmosphere decreased in winter, the air–sea CO2 flux decreased simultaneously, slowing the pCO2 decrease. Most (95 %) of the instantaneous half-hourly air–sea CO2 fluxes were within −0.56–0.85 µmol m−2 s−1.
Based on the air–sea CO2 flux data collected within 2017–2021 at Utö, the sea was a source of carbon dioxide (Table 1). On average, 27.1 g of carbon was released for each square meter annually. The highest net source strength (39.2 gC m−2 yr−1) was observed in 2017, whereas the following year, 2018, showed the lowest emission (18.2 gC m−2 yr−1).
Table 1The CO2 balance calculated using the gap filling with the best fit of the gas transfer velocity, the balance using the parameterization by Wanninkhof (2014), sum of the sink fluxes, sum of the source fluxes, and the uncertainty of the annual air–sea CO2 flux at Utö in 2017–2021. The positive values indicate annual CO2 emissions. The unit of the values is gC m−2 yr−1.
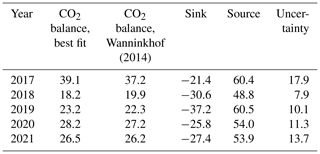
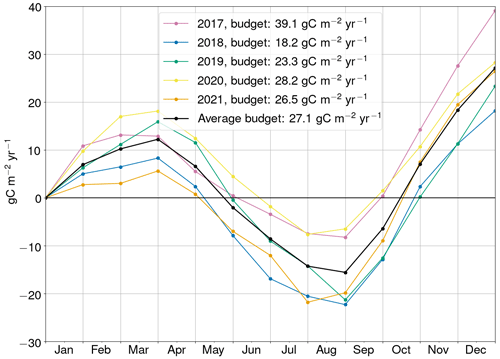
Figure 10Cumulative sum of the monthly means, starting from 1 January, of the air–sea CO2 fluxes at Utö for the years 2017–2021.
The cumulative sums of the air–sea flux CO2 in 2018 were low throughout the year, compared to the 5-year average (Fig. 10). The initial pCO2 in January 2018 was low, generating only small sea-to-air fluxes in the beginning of the year. The spring bloom in 2018 was strong, which made the cumulative sum of the air–sea CO2 flux stay low over the summer. The increase of the monthly flux sums in the latter half of the year was relatively ordinary.
For the year 2017, the high initial pCO2 in January caused the year to start with a high cumulative CO2 emission. The bloom was weak, and the pCO2 stayed relatively high for the summer, causing the cumulative sum to rise above the average sum. Based on the monthly sum slopes, the release of CO2 was ordinary during September–December. The difference to the average had already occurred before autumn–winter, and it remained this way for the rest of the year. Interestingly, the cumulative sums in Septembers 2017 and 2020 were similar, but the CO2 release within October–December 2020 was low.
The annual air–sea CO2 flux budgets calculated for the calendar year (1 January–31 December) are affected by the impact of the previous year or how quickly the sea releases CO2 to the atmosphere in autumn. For this reason, it would be more scientifically relevant to study the sink and source seasons separately. For this purpose, we calculated the sum of the air–sea CO2 sink (negative) fluxes for each year (Table 1). The average annual sum of sink CO2 fluxes was −28.5 gC m−2 yr−1, with a standard deviation of 5.9 gC m−2 yr−1 and a coefficient of variation of 21 % (standard deviation divided by the average). The average sum of source (positive) fluxes was 55.5 gC m−2 yr−1, and the standard deviation of it was 5.0 gC m−2 yr−1 or 9 %. The coefficient of variation suggests that the summer CO2 sink fluxes vary relatively more than the annual source fluxes.
4.1 Net air–sea CO2 exchange
The marine ecosystem at Utö acted as a net source of atmospheric carbon dioxide, on an annual basis in 2017–2021. The average air–sea exchange of CO2 to the atmosphere at Utö was 27.1 gC m−2 yr−1, which is comparable to the CO2 balance measured in pelagic conditions in the Gulf of Bothnia (Algesten et al., 2006). Also, the pCO2 variation at Utö, approximately 200–600 µatm, is similar to the one observed in the Gulf of Bothnia (Algesten et al., 2004). In the Baltic Proper, for comparison, the pCO2 maximum in autumn–winter is lower, approximately 500 µatm (Schneider et al., 2014). Being approximately 80 km from the mainland, the marine ecosystem at Utö is coastal, but it still differs clearly from the European estuaries where pCO2 can peak up to almost 10 000 µatm, and instantaneous fluxes range within 1–9 µmol m−2 s−1 (Frankignoulle et al., 1998).
The net flux of CO2 to the atmosphere suggests that the sea surface system receives carbon (organic or inorganic) from elsewhere (Cole et al., 1994). Organic carbon originates primarily from photosynthesis, either locally (autochthonous) or imported from elsewhere (allochthonous). Microbial degradation and sun-induced photodegradation of organic carbon remineralize carbon and nutrients in the seawater into inorganic forms. Terrestrial–aquatic continuum systems are typically strong CO2 sources due to the net heterotrophy (respiration overweighing the production), stimulated by the high river inputs (Borges et al., 2005). For instance, the Gulf of Bothnia is a net source of CO2 to the atmosphere due to the low productivity and high input of terrestrial organic matter (Algesten et al., 2006; Kuliński and Pempkowiak, 2011).
Phytoplankton blooms dictate the quantity of inorganic carbon fixation (pCO2 drawdown). A part of this biologically fixed carbon is respired and mixed back to the sea surface system eventually, and another part is removed from the short-term carbon cycle due to, for example, sedimentation, refractory compounds, or grazing. Mixing events bring CO2-rich water back to the surface layer. Such an upwelling event probably occurred in July 2020, when simultaneous drops in surface layer temperature, nutrient concentrations, and Chl-a concentration and increases in salinity and the pCO2 were observed. The annual overturning of the water column plays an important factor in bringing the carbon to the surface, to be released to the atmosphere. Similar effects can be generated by the wind-induced upwelling events, which can occasionally occur in the outer archipelago (Lehmann et al., 2012). In winter, the pCO2 is mainly governed by the outgassing of the inorganic carbon into the atmosphere, as it slowly approaches the atmospheric pCO2. The carbon balance of the system is also governed by advection and atmospheric deposition. The advection can depend on, for example, current patterns and river dynamics. The atmospheric deposition of carbon constitutes carbon entering the system by precipitation (wet deposition) and from atmospheric particles (dry deposition). Undoubtedly, the magnitude of many of these carbon flows remains unknown, without a large set of multidisciplinary measurements.
The possible advection of carbon to the sea surface system at Utö might explain some differences between the observed pCO2 variation and the pCO2 variation calculated from the oxygen using the Redfield ratios in the study of Honkanen et al. (2021), especially concerning the seasonal variability. Southward water transport in the Archipelago Sea is typically largest in spring–summer (Miettunen et al., 2024), bringing riverine water to Utö, which could increase the observed pCO2 compared to the pCO2 calculated from the oxygen changes assuming Redfield ratios between the carbon and oxygen.
4.2 Interannual variability of the net air–sea CO2 exchange
The annual net air–sea CO2 exchanges at Utö in 2017–2021 varied within 18.2–39.1 gC m−2 yr−1. The inaccuracies for the annual air–sea CO2 exchanges were within 7.9–17.9 gC m−2 yr−1, indicating an inaccuracy range of approximately 40 % of the total exchange, and thus the positive net exchanges for all years are certain. The inaccuracy arises mostly from the gas transfer velocity parameterization (Appendix A). The magnitude of this interannual variability is higher than the one observed in the pelagic conditions in the Baltic Proper, −7 to −11 gC m−2 yr−1 (Schneider et al., 2014). The 2 years differing the most from the mean of this period (2017 and 2018) are discussed here in detail. In particular, the summers of these 2 years exhibited notable differences in the pCO2 and air–sea CO2 flux dynamics.
The highest annual balance of CO2 (39.1 gC m−2 yr−1) occurred in 2017. The pCO2 in 2017 fell relatively early, already in late March. The nitrate concentration was depleted already in April, and there were no more nutrients to fuel further the spring bloom. The algal biomass quickly decreased from April to May. In comparison with other years, the seawater pCO2 in summer 2017 remained unusually high and values nearly exclusively above the multi-year mean. Therefore, the pCO2 gradient between the sea and atmosphere remained low over the summer, and the sink fluxes were accordingly weaker than other years. The summer of 2017 was also the coolest of the 5 study years. The conditions did not favor phytoplankton production due to a relatively low amount of sunlight, low number of nutrients, and the occasionally deep mixing depth. The thermocline depth was deep in the late summer of 2017, affecting the surface temperatures. This mixing of CO2-rich water to the surface may have diluted the drawdown of pCO2 at the surface, thus lessening the negative fluxes in summer. The source fluxes in early winter 2017 were high, even though the pCO2 gradient was ordinary or even small, compared to other years, but these months were very windy, which increased the gas transfer velocity. For this reason, we believe the sea was able to release more carbon dioxide to the atmosphere before the end of the year.
The year 2018 had the lowest net CO2 source observed at Utö in 2017–2021. In 2018, the spring bloom, observed from Chl-a fluorescence, was high compared to other years, quickly reducing pCO2 in April. In addition to the expressive spring bloom, 2018 also had the warmest summer, with the surface temperature reaching up to 25.0 °C. Temperature directly affects the dissociation and solubility of CO2, but the warm and calm surface water conditions also support cyanobacterial bloom. Actually, in summer 2018, an intense cyanobacteria bloom was observed from mid-July until end of July (Kraft et al., 2021). This was partly seen in Chl-a fluorescence as well, although that method is not well suited for cyanobacteria observations.
Wesslander et al. (2010) found that the interannual variability of the summer pCO2 in the central Baltic Sea and the Kattegat are governed by the maximum phosphate concentrations. The highest annual sum of the sink air–sea CO2 fluxes was observed in 2019, and this year had an average concentration of pre-spring nutrients. Thus, the nutrients alone do not predict the whole interannual variation of air–sea CO2 exchange at Utö. However, the nutrient levels in connection with other environmental conditions play an important role governing the spring–summer blooms. Low saline conditions at Utö in February 2021 and March 2018 may be indicative of intrusion of riverine water masses, inputting the nutrient inventories. The pre-spring nitrate and phosphate levels were high in both 2018 and 2021. The spring bloom of 2021 was ordinary at most, in terms of pCO2, possibly due to the low solar irradiation levels in May 2021. However, the late-summer bloom in July 2021 generated record-low pCO2 and phosphate levels. The late-summer increase of phytoplankton was possibly related to the blooms of cyanobacteria, which had been observed at Utö using imaging flow cytometry (Kraft et al., 2022).
The initial pCO2 in January had some impact on the annual air–sea CO2 exchange as seen in the difference between Januaries of 2017 and 2021. On average, the pCO2 was approximately 500 µatm in the beginning of the year. The year 2017 started with a very high pCO2 (600 µatm), which partly caused the CO2 fluxes to be higher during January than for the other years.
If the inorganic carbon system at Utö is driven by the organic carbon input entering the system, we should see a difference in the annual air–sea CO2 fluxes depending on the dissolved organic carbon concentrations and possibly even on the riverine fluxes of organic matter. Based on the model results by Miettunen et al. (2024), during the first half of the year 2017, the water masses advected southwards in the Archipelago Sea, possibly bringing organic-rich waters to our study area. Additional measurements directing to organic carbon are required to understand these diverse carbon flows.
4.3 Importance of the CO2 emissions in coastal ecosystems
The coastal seas are vulnerable ecosystems, highly impacted by human actions and climate change. These areas provide humans with multiple ecosystem services, ranging from fisheries to carbon sequestration. River discharges into the Baltic Sea are expected to increase with increasing precipitation, which would generate an increased inflow of organic matter (Asmala et al., 2019).
This study demonstrates the importance of multidisciplinary studies combining atmospheric, marine, and ecosystem research with continuous long-term observations. The results present the annual carbon balance between the sea ecosystem and the atmosphere in a coastal sea region, where the biogeochemical processes dominate the annual cycle, in contrast with the open oceans, where the main factor impacting the carbon balance is temperature. Coastal environments, such as marginal seas, upwelling systems, and estuaries, exhibit one of the largest flows of oceanic carbon and nutrients (Borges et al., 2005).
As the carbon uptake by the marine ecosystem forms the base of marine ecosystem food chain and the ecosystem diversity is largest in coastal areas, these kinds of observations are necessary both for climate change and global biodiversity change studies. These results showing the CO2 emissions raise questions on the further need of drainage basin management, as the organic matter fluxes in the land–sea continuum affect the carbon balance of the sea. The carbon balance of the terrestrial ecosystems is currently included in the national legislation, while the role of the marine ecosystems is omitted, even though the management of the marine ecosystems has been recognized as a way to mitigate climate change (Hilmi et al., 2021). Certainly, the carbon flows between the land and oceanic ecosystems require more scientific and public attention.
We analyzed the 5-year (2017–2021) data set of the air–sea CO2 flux measurements made at the Utö Atmospheric and Marine Research Station. The sea area around the island of Utö was found to act as a net source of CO2 with an average annual net air–sea CO2 exchange of 27.1 gC m−2 yr−1. The annual exchange varied between 18.2 in 2018 and 39.2 gC m−2 yr−1 in 2017. Emission of CO2 indicates that the marine system at Utö respires carbon that originated elsewhere, and this possible carbon advection likely involves interannual variability, depending on many meteorological, oceanic, and anthropogenic factors.
The air–sea CO2 exchange at Utö underwent a large biologically driven seasonal cycle each year, with a CO2 sink in spring–summer and a source in autumn–winter. The interannual variability in the pCO2 was greatest in summer, which suggests that the summer pCO2 level had a significant effect on the interannual variability of the net air–sea CO2 exchange. The strength of the algal blooms contributed to the magnitude of the pCO2 drawdown in spring and summer. High pre-spring nutrient concentrations favored the strong bloom during the warm summer of 2018 and thus generated a strong pCO2 drawdown. During the cloudy and cold summer 2017, the pCO2 stayed relatively high compared to the other summers studied.
Typically the calculation of the net air–sea CO2 exchange is based on a calendar year, which may be impacted by the initial pCO2 level at the start of each year. If the autumn of the previous year had a low exchange of carbon with the atmosphere, due to the low wind speeds, the inorganic carbon inventories remained relatively high at the turn of the year. As the exchange is directly proportional to the pCO2 gradient, this increases the fluxes in the beginning of the year.
The net exchange of CO2 between the atmosphere and the sea at Utö is likely governed by the interplay between the advection, biological processes, and sedimentation. The advection replenishes the carbon inventories, while production and sedimentation consume inorganic carbon. Thus, the interannual variability should originate from the yearly variability of these processes. The sustained multidisciplinary research made at Utö can pinpoint the effect of these different carbon flows on the air–sea CO2 exchange in the future.
Both the direct measurements of CO2 flux by EC and the flux calculation based on the measured pCO2 gradient and wind speed introduce uncertainty in the net CO2 exchange estimates.
The EC method measures directly the CO2 exchange between the surface and the lower atmosphere within a given flux footprint area. The sink–source strength of the surface equals the vertical EC flux measured above the surface on a flat and homogeneous terrain under steady-state conditions. The relative nonstationarity (RN), introduced in Sect. 2.3, is an important variable for analyzing the fulfillment of the steady-state assumption (Honkanen et al., 2018). Here, we used a relaxed RN threshold (60 %). We calculated the root mean square error, RMSE, between the measured and parameterized fluxes for different RN thresholds and found out that the RMSE decreases sharply when RN is tightened from 100 % (0.45 µmol m−2 s−1) to 60 % (0.35 µmol m−2 s−1), but it remains stable below this threshold: at RN =10 %, RMSE =0.33 µmol m−2 s−1.
The parameterized air–sea CO2 flux relies on the measurement of the CO2 gradient at the sea–atmosphere interphase, calculation of CO2 solubility, and the parameterization of the gas transfer velocity, which relates to the intensity of the turbulence at this interphase (Eq. 1).
The measurement of the CO2 gradient is a technical challenge. The pumps used for the sample water intake must typically always be wet, and thus the sample water inlet cannot be placed directly at the water surface. Temperature and pCO2 gradients between the inlet depth and the water surface can generate an error on the estimate of the air–sea CO2 gradient, especially during summertime, when a shallow thermocline develops. As these layers shallower than the water inlet are relatively short-term, their effect is not dealt with here. An assessment of the overall effect of pCO2 gradients would require vertical pCO2 measurements within the mixed surface layer.
Sea ice, as a physical barrier separating the sea from the atmosphere, can generate differences between the actual fluxes and parameterized air–sea CO2 fluxes. We observed sea ice at Utö only occasionally in March, when the pCO2 difference between the sea and the atmosphere, and thus the flux between them, is typically small. Also, a solid coverage of sea ice was present only during short periods, and thus the sea ice did not play a significant role in the processes affecting net air–sea CO2 fluxes.
In order to present full time series of pCO2, we reconstructed a small part of the pCO2 data with the method described in Sect. 2.3. The R2 between this reconstructed and measured pCO2 was 0.74, and the RMSE was 69 µatm.
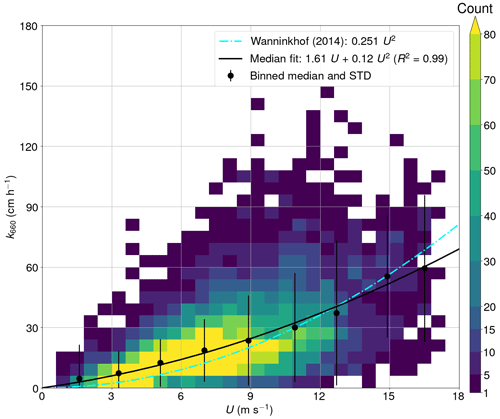
Figure A1Gas transfer velocity, normalized to the Schmidt number of 660, as a function of wind speed.
The most common parameterizations of the gas transfer velocity are based on wind speed (Wanninkhof, 2014), but they are best suited for the open-ocean conditions, where the wind can generate waves freely. As there are numerous islands in the Archipelago Sea, we developed a local parameterization from our measurements of gas transfer velocity and wind speed.
We analyzed the measured gas transfer velocity as a function of wind speed. For the analysis of the gas transfer velocity, a stricter quality control, compared to the general EC flux data, was used in order to robustly analyze the gas transfer velocity as a function of wind speed. We tightened the threshold of relative non-stationarity from 60 % to 30 %. We also used similar data processing as done by Gutierrez-Loza et al. (2022) and included only the periods when the absolute CO2 partial pressure difference between the sea and the atmosphere was at least 50 µatm. Also, the measured negative gas transfer velocities were not used. The RMSE between the parameterized and measured air–sea CO2 flux for these data (n=8758) was 0.30 µmol m−2 s−1 or 33 % error. The comparison between the annual budgets calculated using the parameterization and the EC method is not straightforward as the EC data set contains frequent gaps. However, the average annual air–sea CO2 exchange using only the EC method was 19 gC m−2 yr−1, which is slightly lower than the estimate received using the parameterization and the EC data together (27 gC m−2 yr−1).
The normalized gas transfer velocity, k660, parameterized according to Wanninkhof (2014) generally followed the measurements (Fig. A1) but tended to underestimate for the most common wind speeds (<10 m s−1) and overestimate for the highest wind speeds (>15 m s−1). This could have an effect especially for the negative air–sea CO2 fluxes in summer, when the wind speeds are low. In low wind conditions, it is possible that the coastal characteristics, such as cross-swells, of the study site increase the turbulence in the air–sea interface. With high wind speeds, on the other hand, the fetch may turn out to be a limiting factor for the wave growth, but this applies to a shorter period of time. For comparison, we also calculated the net CO2 exchange at Utö using both the Wanninkhof (2014) and the local parameterization and found only a small difference. The average net CO2 exchange, calculated using the open-ocean parameterization, was 26.6 gC m−2 yr−1, and for different years the annual exchanges are given in Table 1.
The error estimates for the air–sea CO2 fluxes were calculated assuming that the parameterization of the gas transfer velocity is the dominant source of uncertainty. The error estimate for the annual net exchange of air–sea CO2 was obtained by multiplying the annual balance with the median absolute percentage error (between the EC and parameterized flux), weighted by the proportion of parameterized fluxes. The uncertainty for the average net exchange of CO2 was 11.3 gC m−2 yr−1. The error estimates for each year are given in Table 1. The error estimates for the monthly flux averages (shown in Fig. 9) are calculated as a monthly median absolute difference between the EC flux and parameterized flux, weighted by the proportion of parameterized fluxes.
The data set used here can be found in the open archives of Zenodo: https://doi.org/10.5281/zenodo.12636698 (Honkanen et al., 2024).
SK, TM, JS, PY, and LL designed and constructed the flow-through system and the micrometeorological flux tower. KS, SK, JS, LL, LH, PY, and MH were in charge of the operation of the flow-through measurements. TM, JH, LL, and MH operated the flux measurements. JH was in charge of the flux software. MH created the data figures and performed the data analysis. MH led the conceptualization of the research and the writing of the text, which were contributed by LH, MA, JS, JPT, and LL. SMS created Fig. 1, and SK created Fig. 2.
The contact author has declared that none of the authors has any competing interests.
Publisher’s note: Copernicus Publications remains neutral with regard to jurisdictional claims made in the text, published maps, institutional affiliations, or any other geographical representation in this paper. While Copernicus Publications makes every effort to include appropriate place names, the final responsibility lies with the authors.
We thank Ismo and Brita Willström for the CTD casts and maintenance of the Utö station and everybody else involved in Utö's measurements. We acknowledge the Integrated Carbon Observation System (ICOS) for providing the atmospheric CO2 data at Utö. This study utilized data from the Utö Atmospheric and Marine Research Station, which is part of the national Finnish Marine Research Infrastructure (FINMARI) consortium and the Joint European Research Infrastructure for Coastal Observatories (JERICO).
This research has been supported by the Research Council of Finland project SEASINK (Evolving carbon sinks and sources in coastal seas – will ecosystem response temper or aggravate climate change?, project nos. 317297 and 317298). Part of this work was supported by the JERICO-NEXT and JERICO-S3 projects, which have received funding from the European Union Horizon 2020 research and innovation program under grant agreement nos. 654410 and 871153, respectively.
We thank the two anonymous referees and the associate editor, Frédéric Gazeau, for their comments, which improved the publication.
This research has been supported by the Research Council of Finland (grant nos. 317297, 317298, and 342223) and the Horizon 2020 Framework Programme H2020 Excellent Science (grant nos. 654410 and 871153).
This paper was edited by Frédéric Gazeau and reviewed by two anonymous referees.
Aaltonen, A.: Utön tuulivertailu – Edustavatko avoimien merisektoreiden potentiaalituulet tuulioloja merellä 10 metrin korkeudella? (29.11.2021), Internal report, Finnish Meteorological Institute, 2021. a
Algesten, G., Wikner, J., Sobek, S., Tranvik, L., and Jansson, M.: Seasonal variation of CO2 saturation in the Gulf of Bothnia: Indications of marine net heterotrophy, Global Biogeochem. Cy., 18, 4021–4028, https://doi.org/10.1029/2004GB002232, 2004. a
Algesten, G., Brydsten, L., Jonsson, P., Kortelainen, P., Löfgren, S., Rahm, L., Räike, A., Sobek, S., Tranvik, L., Wikner, J., and Jansson, M.: Organic carbon budget for the Gulf of Bothnia, J. Marine Syst., 63, 155–161, https://doi.org/10.1016/j.jmarsys.2006.06.004, 2006. a, b, c
Andersen, J. H., Carstensen, J., Conley, D. J., Dromph, K., Fleming-Lehtinen, V., Gustafsson, B. G., Josefson, A. B., Norkko, A., Villnäs, A., and Murray, C.: Long-term temporal and spatial trends in eutrophication status of the Baltic Sea, Biol. Rev., 92, 135–149, https://doi.org/10.1111/brv.12221, 2017. a
Asmala, E., Carstensen, J., and Räike, A.: Multiple anthropogenic drivers behind upward trends in organic carbon concentrations in boreal rivers, Environ. Res. Lett., 14, 16, https://10.1088/1748-9326/ab4fa9, 2019. a
Bakker, D. C. E., Pfeil, B., Landa, C. S., Metzl, N., O'Brien, K. M., Olsen, A., Smith, K., Cosca, C., Harasawa, S., Jones, S. D., Nakaoka, S., Nojiri, Y., Schuster, U., Steinhoff, T., Sweeney, C., Takahashi, T., Tilbrook, B., Wada, C., Wanninkhof, R., Alin, S. R., Balestrini, C. F., Barbero, L., Bates, N. R., Bianchi, A. A., Bonou, F., Boutin, J., Bozec, Y., Burger, E. F., Cai, W.-J., Castle, R. D., Chen, L., Chierici, M., Currie, K., Evans, W., Featherstone, C., Feely, R. A., Fransson, A., Goyet, C., Greenwood, N., Gregor, L., Hankin, S., Hardman-Mountford, N. J., Harlay, J., Hauck, J., Hoppema, M., Humphreys, M. P., Hunt, C. W., Huss, B., Ibánhez, J. S. P., Johannessen, T., Keeling, R., Kitidis, V., Körtzinger, A., Kozyr, A., Krasakopoulou, E., Kuwata, A., Landschützer, P., Lauvset, S. K., Lefèvre, N., Lo Monaco, C., Manke, A., Mathis, J. T., Merlivat, L., Millero, F. J., Monteiro, P. M. S., Munro, D. R., Murata, A., Newberger, T., Omar, A. M., Ono, T., Paterson, K., Pearce, D., Pierrot, D., Robbins, L. L., Saito, S., Salisbury, J., Schlitzer, R., Schneider, B., Schweitzer, R., Sieger, R., Skjelvan, I., Sullivan, K. F., Sutherland, S. C., Sutton, A. J., Tadokoro, K., Telszewski, M., Tuma, M., van Heuven, S. M. A. C., Vandemark, D., Ward, B., Watson, A. J., and Xu, S.: A multi-decade record of high-quality fCO2 data in version 3 of the Surface Ocean CO2 Atlas (SOCAT), Earth Syst. Sci. Data, 8, 383–413, https://doi.org/10.5194/essd-8-383-2016, 2016. a
Baldocchi, D., Falge, E., Gu, L., Olson, R., Hollinger, D., Running, S., Anthoni, P., Bernhofer, C., Davis, K., Evans, R., Fuentes, J., Goldstein, A., Katul, G., Law, B., Lee, X., Malhi, Y., Meyers, T., Munger, W., Oechel, W., Paw, U. K. T., Pilegaard, K., Schmid, H. P., Valentini, R., Verma, S., Vesala, T., Wilson, K., and Wofsy, S.: FLUXNET: A new tool to study the temporal and spatial variability of ecosystem-scale carbon dioxide, water vapor, and energy flux densities, B. Am. Meteor. Soc., 82, 2415–2434, https://doi.org/10.1175/1520-0477(2001)082<2415:FANTTS>2.3.CO;2, 2001. a, b
Borges, A. V., Delille, B., and Frankignoulle, M.: Budgeting sinks and sources of CO2 in the coastal ocean: Diversity of ecosystems counts, Geophys. Res. Lett., 32, L14601, https://doi.org/10.1029/2005GL023053, 2005. a, b
Carstensen, J., Andersen, J., Gustafsson, B., and Conley, D.: Deoxygenation of the Baltic Sea during the last century, P. Natl. Acad. Sci. USA, 111, 5628–5633, https://doi.org/10.1073/pnas.1323156111, 2014. a
Cole, J. J., Caraco, N. F., Kling, G. W., and Kratz, T.K.: Carbon Dioxide Supersaturation in the Surface Waters of Lakes, Science, 265, 1568–70, https://10.1126/science.265.5178.1568, 1994. a
Erkkilä, A. and Kalliola, R.: Patterns and dynamics of coastal waters in multi-temporal satellite images: support to water quality monitoring in the Archipelago Sea, Finland, Estuar. Coast. Shelf S., 60, 165–177, https://doi.org/10.1016/j.ecss.2003.11.024, 2004. a
Dickson, A. G., Sabine, C. L., and Christian, J. R. (Eds.): Guide to best practices for ocean CO2 measurements, PICES Special Publication 3, 191 pp., https://doi.org/10.25607/OBP-1342, 2007. a
Feely, R., Doney, S., and S. Cooley.: Ocean acidication: present conditions and future changes in a high-CO2 world, Oceanography, 22, 4, 36–47, https://doi.org/10.5670/oceanog.2009.95, 2009. a
Field, C. B., Behrenfeld, M. J., Randerson, J. T., and Falkowski, P.: Primary production of the biosphere: integrating terrestrial and oceanic components, Science, 281, 237–240, https://doi.org/10.1126/science.281.5374.237, 1998. a
Frankignoulle, M., Abril, G., Borges, A., Bourge, I. Canon, C., Delille, B., Libert, E., and Théate, J-M.: Carbon Dioxide Emission from European Estuaries, Science, 282, 434–436, https://doi.org/10.1126/science.282.5388.434, 1998. a
Friedlingstein, P., O'Sullivan, M., Jones, M. W., Andrew, R. M., Gregor, L., Hauck, J., Le Quéré, C., Luijkx, I. T., Olsen, A., Peters, G. P., Peters, W., Pongratz, J., Schwingshackl, C., Sitch, S., Canadell, J. G., Ciais, P., Jackson, R. B., Alin, S. R., Alkama, R., Arneth, A., Arora, V. K., Bates, N. R., Becker, M., Bellouin, N., Bittig, H. C., Bopp, L., Chevallier, F., Chini, L. P., Cronin, M., Evans, W., Falk, S., Feely, R. A., Gasser, T., Gehlen, M., Gkritzalis, T., Gloege, L., Grassi, G., Gruber, N., Gürses, Ö., Harris, I., Hefner, M., Houghton, R. A., Hurtt, G. C., Iida, Y., Ilyina, T., Jain, A. K., Jersild, A., Kadono, K., Kato, E., Kennedy, D., Klein Goldewijk, K., Knauer, J., Korsbakken, J. I., Landschützer, P., Lefèvre, N., Lindsay, K., Liu, J., Liu, Z., Marland, G., Mayot, N., McGrath, M. J., Metzl, N., Monacci, N. M., Munro, D. R., Nakaoka, S.-I., Niwa, Y., O'Brien, K., Ono, T., Palmer, P. I., Pan, N., Pierrot, D., Pocock, K., Poulter, B., Resplandy, L., Robertson, E., Rödenbeck, C., Rodriguez, C., Rosan, T. M., Schwinger, J., Séférian, R., Shutler, J. D., Skjelvan, I., Steinhoff, T., Sun, Q., Sutton, A. J., Sweeney, C., Takao, S., Tanhua, T., Tans, P. P., Tian, X., Tian, H., Tilbrook, B., Tsujino, H., Tubiello, F., van der Werf, G. R., Walker, A. P., Wanninkhof, R., Whitehead, C., Willstrand Wranne, A., Wright, R., Yuan, W., Yue, C., Yue, X., Zaehle, S., Zeng, J., and Zheng, B.: Global Carbon Budget 2022, Earth Syst. Sci. Data, 14, 4811–4900, https://doi.org/10.5194/essd-14-4811-2022, 2022. a
Garbe, C. S, Rutgersson, A., Boutin, J., de Leeuw, G., Delille, B., Fairall, C. W., Gruber, N., Hare, J., Ho, D. T., Johnson, M. T., Nightingale, P. D., Pettersson, H., Piskozub, J., Sahlée, E., Tsai, W., Ward, B., Woolf, D. K., and Zappa, C. J.: Transfer Across the Air-Sea Interface, in: Ocean-Atmosphere Interactions of Gases and Particles, edited by: Liss, P. S. and Johnson, M. T., Springer Earth System Sciences, Springer, Berlin, Heidelberg, https://doi.org/10.1007/978-3-642-25643-1_2, 2014. a
Gutiérrez-Loza, L., Nilsson, E., Wallin, M. B., Sahlée, E., and Rutgersson, A.: On physical mechanisms enhancing air–sea CO2 exchange, Biogeosciences, 19, 5645–5665, https://doi.org/10.5194/bg-19-5645-2022, 2022. a
Grasshoff, K., Kremling, K., and Ehrhardt, M.G.: Methods of seawater analysis, Wiley-VCH Verlag GmbH, https://doi.org/10.1002/9783527613984, 1999. a
Heiskanen, J., Brümmer, C., Buchmann, N., Calfapietra, C., Chen, H., Gielen, B., Gkritzalis, T., Hammer, S., Hartman, S., Herbst, M., Janssens, I. A., Jordan, A., Juurola, E., Karstens, U., Kasurinen, V., Kruijt, B., Lankreijer, H., Levin, I., Linderson, M., Loustau, D., Merbold, L., Myhre, C. L., Papale, D., Pavelka, M., Pilegaard, K., Ramonet, M., Rebmann, C., Rinne, J., Rivier, L., Saltikoff, E., Sanders, R., Steinbacher, M., Steinhoff, T., Watson, A., Vermeulen, A. T., Vesala, T., Vítková, G., and Kutsch, W.: The Integrated Carbon Observation System in Europe, B. Am. Meteor. Soc., 103, E855–E872, https://doi.org/10.1175/BAMS-D-19-0364.1, 2022. a
HELCOM: Guidelines for monitoring of chlorophyll a, Helsinki, Finland, HELCOM, 5 pp., https://doi.org/10.25607/OBP-1064, 2016. a
Hilmi, N., Chami, R., Sutherland, M. D., Hall-Spencer, J. M., Lebleu, L., Benitez, M. B., and Levin, L. A.: The Role of Blue Carbon in Climate Change Mitigation and Carbon Stock Conservation, Front. Clim., 3, 710546, https://doi.org/10.3389/fclim.2021.710546, 2021. a
Honkanen, M., Tuovinen, J.-P., Laurila, T., Mäkelä, T., Hatakka, J., Kielosto, S., and Laakso, L.: Measuring turbulent CO2 fluxes with a closed-path gas analyzer in a marine environment, Atmos. Meas. Tech., 11, 5335–5350, https://doi.org/10.5194/amt-11-5335-2018, 2018. a, b, c, d, e, f, g
Honkanen, M., Müller, J. D., Seppälä, J., Rehder, G., Kielosto, S., Ylöstalo, P., Mäkelä, T., Hatakka, J., and Laakso, L.: The diurnal cycle of pCO2 in the coastal region of the Baltic Sea, Ocean Sci., 17, 1657–1675, https://doi.org/10.5194/os-17-1657-2021, 2021. a, b, c, d
Honkanen, M., Aurela, M., Hatakka, J., Haraguchi, L., Kielosto, S., Mäkelä, T., Seppälä, J., Siiriä, S.-M., Stenbäck, K., Tuovinen, J.-P., Ylöstalo, P., and Laakso, L.: Dataset for Interannual and seasonal variability of the air-sea CO2 exchange at Utö in the coastal region of the Baltic Sea, Zenodo [data set], https://doi.org/10.5281/zenodo.12636698, 2024. a
Jevrejeva, S., Drabkin, V. V., Kostjukov, J., Lebedev, A. A., Leppäranta, M., Mironov, Y., Schmelzer, N., and Sztobryn, M.: Baltic Sea ice seasons in the twentieth century, Clim. Res., 25, 217–227, https://doi.org/10.3354/cr025217, 2004. a
Kahru, M. and Elmgren, R.: Multidecadal time series of satellite-detected accumulations of cyanobacteria in the Baltic Sea, Biogeosciences, 11, 3619–3633, https://doi.org/10.5194/bg-11-3619-2014, 2014. a
Keeling, C. D., Bacastow, R. B., Bainbridge, A. E., Ekdahl Jr., C. A., Guenther, P. R., Waterman, L. S., and Chin, J. F. S.: Atmospheric carbon dioxide variations at Mauna Loa Observatory, Hawaii, Tellus, 28, 538–551, https://doi.org/10.1111/j.2153-3490.1976.tb00701.x, 1976. a
Kilkki, J., Aalto, T., Hatakka, J., Portin, H., and Laurila, T.: Atmospheric CO2 observations at Finnish urban and rural sites, Boreal Env. Res., 20, 227–242, http://hdl.handle.net/10138/228120 (last access: 23 September 2024), 2015. a, b
Kraft, K., Seppälä, J., Hällfors, H., Suikkanen, S., Ylöstalo, P., Anglès, S., Kielosto, S., Kuosa, H., Laakso, L., Honkanen, M., Lehtinen, S., Oja, J., and Tamminen, T.: Application of IFCB High-Frequency Imaging-in-Flow Cytometry to Investigate Bloom-Forming Filamentous Cyanobacteria in the Baltic Sea, Front. Mar. Sci., 8, 594144, https://doi.org/10.3389/fmars.2021.594144, 2021. a, b, c, d
Kraft, K., Velhonoja, O., Eerola, T., Suikkanen, S., Tamminen, T., Haraguchi, L., Ylöstalo, P., Kielosto, S., Johansson, M., Lensu, L., Kälviäinen, H., Haario, H., and Seppälä, J.: Towards operational phytoplankton recognition with automated high-throughput imaging, near-real-time data processing, and convolutional neural networks, Front. Mar. Sci., 9, 867695, https://doi.org/10.3389/fmars.2022.867695, 2022. a
Kuliński, K. and Pempkowiak, J.: The carbon budget of the Baltic Sea, Biogeosciences, 8, 3219–3230, https://doi.org/10.5194/bg-8-3219-2011, 2011. a, b
Kuliński, K., Schneider, B., Hammer, K., Machulik, U., and Schulz-Bull, D. E.: The influence of dissolved organic matter on the acid-base system of the Baltic Sea, J. Marine Syst., 132, 106–115, https://doi.org/10.1016/j.jmarsys.2014.01.011, 2014. a
Kuliński, K., Szymczycha, B., Koziorowska, K., Hammer, K., and Schneider, B.: Anomaly of total boron concentration in the brackish waters of the Baltic Sea and its consequence for the CO2 system calculations, Mar. Chem., 204, 11–19, https://doi.org/10.1016/j.marchem.2018.05.007, 2018.
Kuss, J., Roeder, W., Wlost, K. P., and DeGrandpre, M. D.: Timeseries of surface water CO2 and oxygen measurements on a platform in the central Arkona Sea (Baltic Sea): seasonality of uptake and release, Mar. Chem., 101, 220–232, https://doi.org/10.1016/j.marchem.2006.03.004, 2006. a, b
Laakso, L., Mikkonen, S., Drebs, A., Karjalainen, A., Pirinen, P., and Alenius, P.: 100 years of atmospheric and marine observations at the Finnish Utö Island in the Baltic Sea, Ocean Sci., 14, 617–632, https://doi.org/10.5194/os-14-617-2018, 2018. a, b, c, d
Lehmann, A., Myrberg, K., and Höflich, K.: A statistical approach to coastal upwelling in the Baltic Sea based on the analysis of satellite data for 1990–2009, OCEANOLOGIA, 54, 369–393, https://doi.org /10.5697/oc.54-3.369/, 2012. a
Liblik, T. and Lips, U.: Stratification has strengthened in the Baltic Sea – An analysis of 35 years of observational data, Front. Earth Sci., 7, 174, https://doi.org/10.3389/feart.2019.00174, 2019.
Miettunen, E., Tuomi, L., Westerlund, A., Kanarik, H., and Myrberg, K.: Transport dynamics in a complex coastal archipelago, Ocean Sci., 20, 69–83, https://doi.org/10.5194/os-20-69-2024, 2024. a, b, c
Muller-Karger, F. E., Varela, R., Thunell, R., Luerssen, R., Hu, C., and Walsh, J. J.: The importance of continental margins in the global carbon cycle, Geophys. Res. Lett., 32, L01602, https://doi.org/10.1029/2004GL021346, 2005. a
Müller, J., Schneider, B., and Rehder, G.: Long-term alkalinity trends in the Baltic Sea and their implications for CO2-induced acidification, Limnol. Oceanogr., 61, 1984–2002, https:doi.org/10.1002/lno.10349, 2016. a
Omstedt, A., Gustafsson, E., and Wesslander, K.: Modelling the uptake and release of carbon dioxide in the Baltic Sea surface water, Cont. Shelf Res., 29, 870–885, https://doi.org/10.1016/j.csr.2009.01.006, 2009. a
Pastorello, G., Trotta, C., Canfora, E., Cheah, Y., Christianson, D., Chu, H., Poindexter, C., Chen, J., Elbashandy, A., Humphrey, M., Isaac, P., Polidori, D., Ribeca, A., Van Ingen, C., Zhang, L., Amiro, B., Ammann, C., Arain, M., Ardö, J., Arkebauer, T., Arndt, S., Arriga, N., Aubinet, M., Aurela, M., Baldocchi, D., Barr, A., Beamesderfer, E., Belelli Marchesini, L., Bergeron, O., Beringer, J., Bernhofer, C., Berveiller, D., Billesbach, D., Black, T., Blanken, P., Bohrer, G., Boike, J., Bolstad, P., Bonal, D., Bonnefond, J., Bowling, D., Rosvel, B., Brodeur, J., Brümmer, C., Buchmann, N., Burban, B., Burns, S., Buysse, P., Cale, P., Cavagna, M., Cellier, P., Chen, S., Chini, I., Christensen, T., Cleverly, J., Collalti, A., Consalvo, C., Cook, B., Cook, D., Coursolle, C., Cremonese, E., Curtis, P., D`andrea, E., Da Rocha, H., Dai, X., Davis, K., De Cinti, B., De Grandcourt, A., De Ligne, A., De Oliveira, R., Delpierre, N., Desai, A., Di Bella, C., Di Tommasi, P., Dolman, H., Domingo, F., Dong, G., Dore, S., Duce, P., Dufrêne, E., Dunn, A., Dušek, J., Eamus, D., Eichelmann, U., Elkhidir, H., Eugster, W., Ewenz, C., Ewers, B., Famulari, D., Fares, S., Feigenwinter, I., Feitz, A., Fensholt, R., Filippa, G., Fischer, M., Frank, J., Galvagno, M., Gharun, M., Gianelle, D., Gielen, B., Gioli, B., Gitelson, A., Goded Ballarin, I., Goeckede, M., Goldstein, A., Gough, C., Goulden, M., Graf, A., Griebel, A., Gruening, C., Grünwald, T., Hammerle, A., Han, S., Han, X., Hansen, B., Hanson, C., Hatakka, J., He, Y., Hehn, M., Heinesch, B., Hinko-Najera, N., Hörtnagl, L., Hutley, L., Ibrom, A., Ikawa, H., Jackowicz-Korczynski, M., Janouš, D., Jans, W., Jassal, R., Jiang, S., Kato, T., Khomik, M., Klatt, J., Knohl, A., Knox, S., Kobayashi, H., Koerber, G., Kolle, O., Kosugi, Y., Kotani, A., Kowalski, A., Kruijt, B., Kurbatova, J., Kutsch, W., Kwon, H., Launiainen, S., Laurila, T., Law, B., Leuning, R., Li, Y., Liddell, M., Limousin, J., Lion, M., Liska, A., Lohila, A., López-Ballesteros, A., López-Blanco, E., Loubet, B., Loustau, D., Lucas-Moffat, A., Lüers, J., Ma, S., Macfarlane, C., Magliulo, V., Maier, R., Mammarella, I., Manca, G., Marcolla, B., Margolis, H., Marras, S., Massman, W., Mastepanov, M., Matamala, R., Matthes, J., Mazzenga, F., Mccaughey, H., Mchugh, I., Mcmillan, A., Merbold, L., Meyer, W., Meyers, T., Miller, S., Minerbi, S., Moderow, U., Monson, R., Montagnani, L., Moore, C., Moors, E., Moreaux, V., Moureaux, C., Munger, J., Nakai, T., Neirynck, J., Nesic, Z., Nicolini, G., Noormets, A., Northwood, M., Nosetto, M., Nouvellon, Y., Novick, K., Walter, O., Olesen, J., Ourcival, J., Papuga, S., Parmentier, F., Paul-Limoges, E., Pavelka, M., Peichl, M., Pendall, E., Phillips, R., Pilegaard, K., Pirk, N., Posse, G., Thomas, P., Prasse, H., Prober, S., Rambal, S., Rannik, Ü., Raz-Yaseef, N., Reed, D., Resco De Dios, V., Restrepo-Coupe, N., Reverter, B., Roland, M., Sabbatini, S., Sachs, T., Saleska, S., Sánchez-Cañete, E., Sanchez-Mejia, Z., Schmid, H., Schmidt, M., Schneider, K., Schrader, F., Schroder, I., Scott, R., Sedlák, P., Serrano-Ortíz, P., Shao, C., Shi, P., Shironya, I., Siebicke, L., Šigut, L., Silberstein, R., Sirca, C., Spano, D., Steinbrecher, R., Stevens, R., Sturtevant, C., Suyker, A., Tagesson, T., Takanashi, S., Tang, Y., Tapper, N., Thom, J., Tiedemann, F., Tomassucci, M., Tuovinen, J., Urbanski, S., Valentini, R., Van Der Molen, M., Van Gorsel, E., Van Huissteden, K., Varlagin, A., Verfaillie, J., Vesala, T., Vincke, C., Vitale, D., Vygodskaya, N., Walker, J., Walter-Shea, E., Wang, H., Weber, R., Westermann, S., Wille, C., Wofsy, S., Wohlfahrt, G., Wolf, S., Woodgate, W., Yuelin, L., Zampedri, R., Zhang, J., Zhou, G., Zona, D., Agarwal, D., Biraud, S., Torn, M., and Papale, D.: The FLUXNET2015 dataset and the ONEFlux processing pipeline for eddy covariance data, Sci. Data, 7, 225, https://doi.org/10.1038/s41597-020-0534-3, 2020. a
Petersen, W., Schroeder, F., and Bockelmann, F.-D.: FerryBox – Application of continuous water quality observations along transects in the North Sea, Ocean Dynam., 61, 1541–1554, https://doi.org/10.1007/s10236-011-0445-0, 2011. a
Rantajärvi, E., Stipa, T., Neuvonen, S., Suominen, T., Kaitala, S., Kankaanpää, H., Seppälä, J., Perttilä, M., Raateoja, M., and Haahti, H.: Alg@line in 2003: 10 years of innovative plankton monitoring and research and operational information service in the Baltic Sea, Meri: report series of the Finnish Institute of Marine Research, 48, 22–26, http://hdl.handle.net/10138/167897 (last access: 23 September 2024), 2003. a
Rautiainen, L., Tyynelä, J. Lensu, M., Siiriä, S., Vakkari, V., O'Connor, E., Hämäläinen, K., Lonka, H., Stenbäck, K., Koistinen, J., and Laakso, L.: Utö Observatory for Analysing Atmospheric Ducting Events over Baltic Coastal and Marine Waters, Remote Sens., 15, 2989, https://doi.org/10.3390/rs15122989, 2023. a
Rutgersson, A., Maria, N., Schneider, B., Pettersson, H. and Sahleé, E.: The annual cycle of carbon dioxide and parameters influencing the air–sea carbon exchange in the Baltic Proper, J. Marine Sys., 74, 1–2, 381–394, https://doi.org/10.1016/j.jmarsys.2008.02.005, 2008. a, b
Rutgersson, A., Pettersson, H. Nilsson, E., Bergstrom, H., Wallin, M., Nilsson, E., Sahlée, E., Wu, L., and Mårtensson, E.: Using land-based stations for air–sea interaction studies, Tellus, 72, 1–23, https://doi.org/10.1080/16000870.2019.1697601, 2020. a
Räike, A., Kortelainen, P., Mattsson, T., and Thomas, D.: 36 year trends in dissolved organic carbon export from Finnish rivers to the Baltic Sea, Sci. Total Environ., 435–436, 188–201, https://doi.org/10.1016/j.scitotenv.2012.06.111, 2012. a
Schneider, B., Holzhüter, W., Sadkowiak, B., and Rehder, G.: Detecting sinks and sources of CO2 and CH4 by ferrybox-based measurements in the Baltic Sea: Three case studies, J. Marine Sys., 140, 13–25, https://doi.org/10.1016/j.jmarsys.2014.03.014, 2014. a, b, c
Sloyan, B., Wanninkhof, R., Kramp, M., Johnson, G. Talley, L., Tanhua, T., Mcdonagh, E., Cusack, C., O’Rourke, E., Mcgovern, E., Katsumata, K., Diggs, S., Hummon, J., Ishii, M., Azetsu-Scott, K., Boss, E., Ansorge, I., Perez, F., Mercier, H., and Campos, E.: The Global Ocean Ship-Based Hydrographic Investigations Program (GO-SHIP): A Platform for Integrated Multidisciplinary Ocean Science, Front. Mar. Sci., 6, 445, https://doi.org/10.3389/fmars.2019.00445, 2019. a
Steinhoff, T: Uncertainty analysis for calculations of the marine carbonate system for ICOS-Oceans stations, ICOS OTC Publication, https://doi.org/10.18160/vb7c-z758, 2020. a
Takahashi, T., Olafsson, J., Goddard, J. G., Chipman, D. W., and Sutherland, S. C.: Seasonal variation of CO2 and nutrients in the high-latitude surface oceans: A comparative study, Global Biogeochem. Cy., 7, 843–878, https://doi.org/10.1029/93GB02263, 1993. a
Takahashi, T., Sutherland, S. C., Sweeney, C., Poisson, A., Metzl, N., Tilbrook, B., Bates, N., Wanninkhof, R., Feely, R. A, Sabine, C., Olafsson, J., and Nojiri, Y.: Global sea–air CO2 flux based on climatological surface ocean pCO2, and seasonal biological and temperature effects, Deep-Sea Res. Pt. II, 49, 1601–1622, https://doi.org/10.1016/S0967-0645(02)00003-6, 2002. a
Volk, T. and Hoffert, M. I.: Ocean Carbon Pumps: Analysis of Relative Strengths and Efficiencies in Ocean-Driven Atmospheric CO2 Changes, in: The Carbon Cycle and Atmospheric CO2: Natural Variations Archean to Present, edidet by: Sundquist, E. T. and Broecker, W. S., American Geophysical Union, Geophysical Monograph 32, 99–110, https://doi.org/10.1029/GM032p0099, 1985. a
Wanninkhof, R.: Relationship between wind speed and gas exchange over the ocean, J. Geophys. Res., 97, 7373–7382, https://doi.org/10.1029/92JC00188, 1992. a, b
Wanninkhof, R.: Relationship between wind speed and gas exchange over the ocean revisited, Limnol. Oceanogr.-Meth., 12, 351–362, https://doi.org/10.4319/lom.2014.12.351, 2014. a, b, c, d, e, f, g, h
Wanninkhof, R., Asher, W., Ho, D. Sweeney, C., and McGillis, W.: Advances in Quantifying Air–Sea Gas Exchange and Environmental Forcing, Annu. Rev. Mar. Sci., 1, 213–44, https://doi.org/10.1146/annurev.marine.010908.163742, 2009. a
Wesslander, K., Omstedt, A., and Schneider, B.: Inter-annual and seasonal variations in the air-sea CO2 balance in the central Baltic Sea and the Kattegat, Cont. Shelf Res., 30, 1511–1521, https://doi.org/10.1016/j.csr.2010.05.014, 2010. a, b
Ylöstalo, P., Seppälä, J., Kaitala, S., Maunula, P., and Simis, S.: Loadings of dissolved organic matter and nutrients from the Neva River into the Gulf of Finland – Biogeochemical composition and spatial distribution within the salinity gradient, Mar. Chem., 186, 58–71, https://doi.org/10.1016/j.marchem.2016.07.004, 2016. a