the Creative Commons Attribution 4.0 License.
the Creative Commons Attribution 4.0 License.
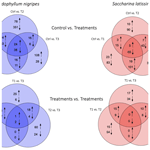
Multifactorial effects of warming, low irradiance, and low salinity on Arctic kelps
Anaïs Lebrun
Marc Meynadier
Steeve Comeau
Pierre Urrutti
Samir Alliouane
Robert Schlegel
Jean-Pierre Gattuso
Frédéric Gazeau
The Arctic is projected to warm by 2 to 5 °C by the end of the century. Warming causes melting of glaciers, shrinking of the areas covered by sea ice, and increased terrestrial runoff from snowfields and permafrost thawing. Warming, decreasing coastal underwater irradiance, and lower salinity are potentially threatening polar marine organisms, including kelps, that are key species of hard-bottom shallow communities. The present study investigates the physiological responses of four kelp species (Alaria esculenta, Laminaria digitata, Saccharina latissima, and Hedophyllum nigripes) to these environmental changes through a perturbation experiment in ex situ mesocosms. Kelps were exposed for 6 weeks to four experimental treatments: an unmanipulated control; a warming condition under the CO2 emission scenario SSP5-8.5; and two multifactorial conditions combining warming, low salinity, and low irradiance reproducing the future coastal Arctic exposed to terrestrial runoff under two CO2 emission scenarios (SSP2-4.5 and SSP5-8.5). The physiological effects on A. esculenta, L. digitata, and S. latissima were investigated, and gene expression patterns of S. latissima and H. nigripes were analyzed. Across all species and experimental treatments, growth rates were similar, underlying the acclimation potential of these species to future Arctic conditions. Specimens of A. esculenta increased their chlorophyll a content when exposed to low irradiance conditions, suggesting that they may be resilient to an increase in glacier and river runoff with the potential to become more dominant at greater depths. S. latissima showed a lower carbon : nitrogen (C : N) ratio under the SSP5-8.5 multifactorial conditions' treatment, suggesting tolerance to coastal erosion and permafrost thawing. In contrast, L. digitata showed no response to the conditions tested on any of the investigated physiological parameters. The down-regulation of genes coding for heat-shock proteins in H. nigripes and S. latissima underscores their ability to acclimate to heat stress, which portrays temperature as a key influencing factor. Based on these results, it is expected that kelp communities will undergo changes in species composition that will vary at local scale as a function of the changes in environmental drivers.
- Article
(6445 KB) - Full-text XML
-
Supplement
(1177 KB) - BibTeX
- EndNote
The Arctic region is warming at more than twice the global average rate (Richter-Menge et al., 2017). Over the next 80 years, sea surface temperature is projected to increase by 2 °C according to the Shared Socioeconomic Pathway (SSP) 1-2.6, which foresees an increasing shift towards sustainable practices, and up to 5 °C according to SSP5-8.5, which assumes an energy-intensive and fossil-fuel-based economy (Kwiatkowski et al., 2020). Warming causes glacier and sea ice to melt at a faster rate, causing an increase in terrestrial runoff from thawing snowfields and permafrost (Shiklomanov and Shiklomanov, 2003; Stroeve et al., 2014). Total freshwater inflow into the Arctic Ocean rose by around 7 % between 1936 and 1999 and 14 % between 1980 and 2009 (Peterson et al., 2002; Ahmed et al., 2020). Combined with vertical mixing by waves and wind action, cryosphere melting results in local turbid and low-salinity waters down to 20 m (Karsten, 2007). Coastal areas are therefore exposed to warming and changing light and salinity conditions (Lebrun et al., 2022).
In the coastal Arctic, kelps are key ecosystem engineers that form underwater forests. Kelp are large brown macroalgae of the class Phaeophyceae and the order Laminariales. Kelp forests provide a food source, habitat, and nursery ground for numerous fish and invertebrates as well as protection of coastlines from erosion (Filbee-Dexter et al., 2019). They support complex food webs and have a substantial role in storing and sequestering carbon (Krause-Jensen and Duarte, 2016). Saccharina latissima, Alaria esculenta, Laminaria digitata, and Hedophyllum nigripes are four abundant kelp species that inhabit the Northern Hemisphere and extend to subarctic and Arctic waters (Bischof et al., 1999; Müller et al., 2009). As a result of warming, which induces more sea-ice-free areas, the surface area suitable for kelps increased by about 45 % from 1940–1950 to 2000–2017 (Krause-Jensen et al., 2020). Temperature requirements and seasonal variability tolerance in irradiance and salinity for reproduction and growth determine the geographical distribution of kelp species (Wiencke et al., 1994; Muth et al., 2021). The temperature tolerance of these kelp species found in the Arctic appears broad (0–20 °C); however, there is significant variability in temperature optima across ecotypes (Bolton and Lüning, 1982; Andersen et al., 2013; Diehl et al., 2021). Similarly, low salinity is well tolerated (Karsten, 2007), yet it is unclear how low-salinity stress may interact with other stressors. Irradiance has a major impact on their depth distribution (e.g., Roleda et al., 2005; Krause-Jensen et al., 2012). Turbid waters alter kelp fitness by limiting photosynthesis. This has already induced a shift in the vertical distribution of kelps such as Laminaria and Saccharina genera to shallower waters in Arctic fjords (Bartsch et al., 2016; Filbee-Dexter et al., 2019). Because optimal temperature, irradiance, and salinity ranges vary between kelp species, their response to environmental changes will likely be species-specific (Eggert, 2012; Karsten, 2012).
We hypothesized that (1) warming will enhance the growth rate of Arctic kelps in Kongsfjorden during summer and that (2) the combined effects of high temperature, low salinity, and low irradiance will negatively impact their physiology, although responses will be species-specific. To test these hypotheses and fill knowledge gaps on the multifactorial effects of climate change across species (Renaud et al., 2019; Scherrer et al., 2019), we carried out a land-based mesocosm experiment exposing four kelp species (S. latissima, A. esculenta, L. digitata, and H. nigripes) found in common biomass between 5 to 10 m depth to four treatments for 6 weeks. The treatments consisted of a control; a warming condition mimicking the future offshore (T1); and two multifactorial conditions combining warming, low salinity, and low irradiance mimicking the future coastal Arctic (T2 and T3). In order to best represent in situ conditions, the different kelp species were incubated together in each mesocosm at densities mimicking natural communities. The physiological effects on A. esculenta, L. digitata, and S. latissima were investigated, and gene expression patterns of S. latissima and H. nigripes were analyzed.
2.1 Specimen collection
In June 2021, 188 sporophytes of A. esculenta, L. digitata, S. latissima, and H. nigripes shorter than 1 m were collected by research divers in Kongsfjorden (Svalbard, Norway). They were collected between 2 and 7 m depth at Hansneset and the Old Pier (Fig. 1). All samples were placed into holding tanks (>1 m3) with flow-through ambient seawater until their placement into final mesocosms on 3 July 2021.
2.2 Mesocosm experiment
The experiment was carried out from 3 July 2021 (t0) to 28 August 2021 (tfinal), in 12 1 m3 mesocosms set up in Ny-Ålesund on the outdoor platform of the Kings Bay Marine Laboratory in order to expose communities to natural light cycles. Each mesocosm received three to six individuals of A. esculenta and S. latissima, two to four individuals of L. digitata and zero to two individuals of H. nigripes for a total kelp biomass (wet weight) per mesocosm of about 1500 g for S. latissima and L. digitata (mingled with H. nigripes) and 1000 g for A. esculenta. Biomasses are representative of those found at Hansneset down to 7 m depth (Hop et al., 2012). Since H. nigripes can be mistaken for L. digitata, each stipe of these two species was cut at tfinal to detect individuals with mucilage, corresponding to H. nigripes (n=16, Dankworth et al., 2020).
The experimental setup is briefly described below. More information can be found in Miller et al. (2024a). Seawater flowing through the mesocosms was pumped from 10 m depth in front of the Kings Bay Marine Laboratory (78.929° N, 11.930° E) using a submersible pump (© Albatros). The regulated flow-through system (7–8 L min−1 in each mesocosm) allowed for the automated control of temperature and salinity. Temperature was adjusted by mixing ambient seawater with warmed seawater (15 °C), and salinity was regulated by addition of freshwater. Irradiance was modified by placing spectra and attenuating light filters on top of each mesocosm to simulate current irradiance and future in situ irradiance (see below for more details). Each mesocosm was equipped with one 12 W wave pump (Sunsun JVP-132) to ensure proper mixing.
Table 1Temperature, salinity, and photosynthetically active radiation (PAR) during the experiment. The T1 and T2 treatments represent future coastline exposed to runoff conditions, whereas the T3 treatment represents future conditions on shores not exposed to runoff. The quartiles and medians were calculated based on data acquired from 10 July 2021 for PAR and 16 July 2021 for temperature and salinity (once the targeted treatments were reached) until the end of the experiment.
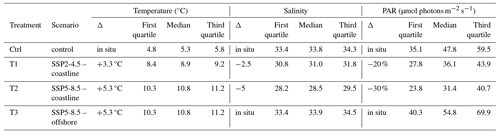
Four experimental treatments in triplicate (4×3 mesocosms) were used to study conditions representative of present and future Arctic coastal communities in proximity or not to glaciers following two different SSP scenarios (Ctrl, T1, T2, T3; Table 1). Treatments 1 and 2 (T1 and T2) mimicked the conditions expected close to glaciers and, therefore, combined warming, low irradiance, and low salinity. T1 followed SSP 2-4.5, which describes a middle-of-the-road projection that does not shift markedly from historical patterns, while T2 followed SSP5-8.5, which assumes an energy-intensive and fossil-fuel-based economy. T3 focused on the projected change outside glacial fjords following SSP 5-8.5, where warming acts as a single driver. Temperature was increased by 3.3 °C in T1 and 5.3 °C in T2 and T3 as an offset increase from the control condition (Ctrl), which mimicked the in situ temperature recorded in real time during the whole experiment. Based on in situ measurements of temperature and salinity in Kongsfjorden taken from week 22 to 35 in 2020, salinity offsets were determined from the in situ relationship between temperature and salinity and extrapolated to apply to future warming. This resulted in a salinity decrease by 2.5 in T1 and 5 in T2 (Miller et al., 2024a). Based on in situ photosynthetically active radiation (PAR) data collected in May 2021 with a LI-COR (model 192), irradiance was reduced from the control by a mean of 25 % for T1, corresponding to the difference between the glacier-proximal inner region and the middle of the fjord, and 40 % for T2, corresponding to the difference between the inner and outer parts of the fjord. To simulate the in situ light spectrum (Kai Bischof, personal communication, 2020) and reach the irradiance matching the targeted treatments, green (RL244) and neutral Lee filters© (RL211; RL298) were placed on top of each mesocosm accordingly (Table 1). During the first week, all the mesocosms were maintained under in situ conditions of temperature, salinity, and irradiance. The light filters were then added to the mesocosms of T1 and T2 treatments on 10 July 2021, and all treatments gradually reached their targeted temperature and salinity conditions in 6 d. The experiment then lasted for 6 weeks.
2.3 Tissue sampling
Tissue samples were collected in the meristem (located directly above the stipe-frond junction on the frond) of 10 individuals of A. esculenta, L. digitata, and S. latissima at the beginning of the experiment (t0, 3 July 2021) and on the healthy organisms, namely complete organisms (frond, stipe, and holdfast) that exhibited a firm brown frond without signs of disease at the end (tfinal), pending determination of chlorophyll a (chl a; see Sect. 2.4) and the carbon : nitrogen (C : N) ratio (see Sect. 2.5). Samples were stored in aluminum foil at −20 °C. Additional tissue samples were collected in the meristem of S. latissima and H. nigripes at tfinal for gene expression analysis (n=8 for each species; see Sect. 2.7). These tissue samples were immediately flash-frozen in liquid nitrogen before being stored at −80 °C.
2.4 Chl a content
Samples were blotted dry, weighed (wet weight), and ground with a glass pestle. Chl a was extracted in 90 % aqueous acetone for 24 h in the dark at 4 °C. After cold centrifugation (0 °C, 15 min, 3000 rpm), the supernatants were transferred one at a time into a glass vial, and the initial fluorescence (F0) of chl a and pheophytin pigment was measured using a fluorometer (Turner Design 10-AU fluorometer; 667 nm). The Fa fluorescence (fluorescence after acidification) was measured 1 min after the addition of 10 µL of 0.3 N HCl to transform chl a into pheophytin pigment and subtract Fa from F0. The chl a content was calculated using the formula of Lorenzen (1967) but modified for determining chl a from mass of tissue rather than volume of seawater as (Eq. 1)
where k is the calibration factor of pigment to fluorescence intensity (µg chl a per mg solvent per instrument fluorescence unit), m is the mass of acetone used for extraction (mg), and mkelp is the fresh weight of kelp (mg). Chl a content is expressed in µg per g of fresh weight (µg gFW−1). No dilution factor was used as this was dry tissue mass.
2.5 C : N mass ratio
Samples were dried at 60 °C for 48 h and weighed (dry weight), and their sizes were adjusted to ensure that they did not weigh more than 10 mg, the detection limits specific to the CHN analyzer (PerkinElmer, Inc 2400). C and N contents are expressed in mg per g of dry weight (mg gDW−1).
2.6 Growth rate
Growth rate was determined using the hole puncture method of Parke (1948). Sporophytes were punctured at t0 in the meristem section of each organism, 2 cm above the base of the frond. The distance from the base of the frond to the hole was measured at tfinal. The growth rate was calculated as follows (Eq. 2):
with dist as the distance (in cm) from the base of the frond to the hole at time t (in d).
Weekly growth rates for selected individuals were determined at different time points during the experiment for S. latissima (weeks 1 and 4) and A. esculenta (weeks 2 and 5). Results can be found in the Supplement (Fig. S2).
2.7 Gene expression analysis
We chose to target two species for gene expression, S. Latissima and H. nigripes. S. latissima was selected because it was the most abundant by biomass in the sampling area and appeared in robust physical health upon visual inspection at tfinal. H. nigripes was selected because it is an endemic Arctic species and, thus, a model comparison specimen.
Total RNA extraction was conducted using the method described by Heinrich et al. (2012), which uses a CTAB extraction followed with a commercial Qiagen kit. The quantity and purity of the extracted RNA were evaluated using a Nanodrop ND-1000 spectrophotometer (ThermoFisher), which measures RNA concentration at 260 nm and assesses purity by detecting the presence of other compounds such as DNA at 230 nm and proteins at 280 nm. The integrity of total RNA was determined by automated capillary electrophoresis using an Agilent 2100 bioanalyzer (Agilent Technologies). The cDNA libraries were constructed by poly(A) enrichment and sequenced on a NovaSeq 6000 instrument by the Genome Quebec platform. The 100 bp paired reads were clipped using default values of the Illumina software. The quality of raw sequences was checked using FastQC v.0.11.7 (https://www.bioinformatics.babraham.ac.uk/projects/fastqc/, last access: 15 October 2023). Sequences of low quality were trimmed using Trimmomatic v.0.39 (Bolger et al., 2014). For each species, a de novo transcriptome was constructed using the Trinity v.2.14.0 tool (Grabherr et al., 2011). The most homologous sequences were clustered using the CD-HIT-EST algorithm, part of the CD-HIT v.4.8.1 tool (Li and Godzik, 2006). To ensure the quality of the de novo transcriptomes, another transcriptome per species was generated using the rnaSPAdes v.3.14.1 (Bushmanova et al., 2019). Transcriptomes generated using rnaSPAdes and Trinity were compared using BUSCO v.5.4.3, and transcriptomes generated with Trinity were retained due to lower duplicated sequences (Simão et al., 2015). Transcript quantification was performed by pseudo-alignment using Kallisto v0.46.0, mapping RNA sequences to an index created from de novo transcriptomes (Bray et al., 2016). Exploration of differentially expressed genes (DEGs) was performed with the “DESeq2 v1.34.0” R package (Love et al., 2014). For each species, DEGs were obtained from the following comparisons: T1 vs. C, T2 vs. C, T3 vs. C, T2 vs. T1, T3 vs. T1, and T3 vs. T2. Transcripts with an adjusted p<0.05 and log2 fold change (FC) >2 or were considered significantly differentially expressed genes. Functional annotation of the genes was performed with eggNOG-mapper v2.1.10 against the eggNOG database v.5.0.2 (Huerta-Cepas et al., 2017, 2019). To ensure they were properly annotated, annotation was also performed with TransDecoder v5.5.0 to predict coding sequences (Haas and Papanicolaou, 2015), which were aligned against a Pfam profile database v35.0 (Mistry et al., 2021) using the HMMER v3.3 alignment tool (Finn et al., 2011). Gene ontology (GO) (Gene Ontology Consortium, 2015) terms were then retrieved from the pfam2go database (https://pypi.org/project/pfam2go/, last access: 15 October 2023), and functional enrichment was performed with Ontologizer v2.1 to obtain statistically significant GOs from the DEGs of each comparison performed previously (Bauer et al., 2008). Functional enrichment results were summarized as tree plots and scatter plots using REVIGO v1.8.1 (Supek et al., 2011). Investigation of the specific functions of DEGs was carried out by manually checking the involvement of Pfam domains and EggNOG annotations on the SMART database v9.0 (Letunic et al., 2021). Some DEGs whose annotation was questionable (i.e., not referring to plant genomes such as gene collagen) were removed, as well as those whose annotation was not precise enough to be classified. DEGs were then classified into different categories: cytoskeleton, genetic transcription/translation, metabolism, signaling, transport, stress (heat stress and oxidation–reduction processes), and energy production (respiration and photorespiration). Some DEGs (73.2 % in S. latissima and 82.3 % in H. nigripes) were trimmed as they lacked functional annotation. Tools and parameters are summarized in Table S1 in the Supplement.
2.8 Statistics
Rosner's generalized extreme Studentized deviate (ESD) test was used to detect the outliers using the function rosnerTest of the R package “EnvStats” (Millard, 2013). Out of a total of 165 individual chl a measurements, when combining all species and conditions, 11 were identified as outliers and removed. After the removal of the outliers, the normal distribution of the data was verified with a Shapiro–Wilk test using the function shapiro.test from the “stats” R package (R Core Team, 2023, p>0.105). No outliers were identified in the C : N and growth rate data, and normality was verified (p>0.089).
Chl a content and C : N were analyzed using a linear mixed model with a hierarchical structure (HLM) to evaluate treatment effects by species. The model was fitted using the function lmer in the R package “lme4” (Bates et al., 2015). The fixed factors for the model were treatment and species, while the mesocosm was a random factor. For growth rate measurements, a generalized linear mixed model (GLMM) with a Gaussian distribution was preferred – based on an Akaike information criterion – to test for the effects of the species, treatment, and mesocosm replica.
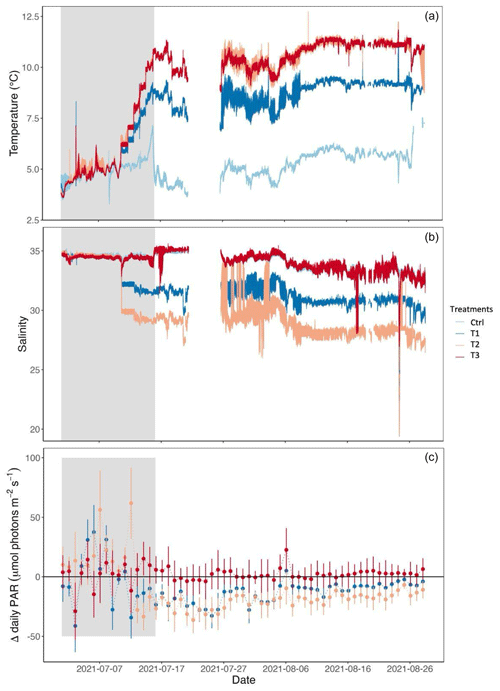
Figure 2(a) Temperature, (b) salinity, and (c) Δ daily photosynthetically active radiation (PAR) between the control and the treatments. Temperature and salinity were measured every minute. PAR values were integrated over 10 min intervals and averaged over the day. The gray-shaded region corresponds to the beginning of the experiment, before the treatment conditions of temperature, salinity, and irradiance were reached. A few days of temperature and salinity data was lost (from 21 to 26 July 2021).
3.1 Experimental conditions
The median temperature value in the control treatment was 5.3 °C during the experimental period (16 July to 28 August 2021), calculated based on the mean value across replicates (Fig. 2, Table 1). The median salinity was 33.8, and the median daily PAR was 47.8 µmol photons m−2 s−1. In treatment T1, the median temperature, salinity, and PAR were 8.9 °C, 31, and 36.1 µmol photons m−2 s−1, respectively. For treatments T2 and T3, the median temperature was elevated to 10.8 °C. In T2, median salinity and PAR were decreased to 28.5 and 31.4 µmol photons m−2 s−1.
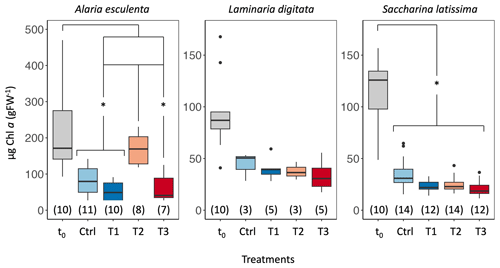
Figure 3Chlorophyll a (chl a) content of Alaria esculenta, Laminaria digitata, and Saccharina latissima exposed to the four treatments, expressed per unit of fresh weight (gFW). t0 values correspond to the chl a content at the start of the experiment, while Ctrl, T1, T2, and T3 correspond to the final chl a content of organisms maintained in the respective treatments for 6 weeks. The horizontal lines in each box plot represent the median. The whiskers extend to the furthest data points within 1.5 times the interquartile range (the top and bottom of the box). Statistically significant differences are shown with an asterisk (p<0.05). The number in parentheses below each box plot corresponds to the sample size.
3.2 Chl a content
The factors' species and treatment were found to be significant predictors, including their interaction when assessing differences in measured chl a content at t0 and tfinal (p<0.001; Table S2). For A. esculenta, the concentration of chl a decreased significantly between t0 and the control at tfinal (p<0.01, Fig. 3, Table S3). Values in the T2 treatment were also significantly higher than the control, T1, and T3 treatments (all p were <0.01). Values in the control, T1, and T3 treatments were not statistically different from each other (p>0.92). Similarly to A. esculenta, chl a content of S. latissima significantly decreased between t0 and tfinal (p=0.02) for the control but was not significantly impacted by the treatments (p>0.99). The chl a content of L. digitata was not significantly impacted by time and treatments (p>0.99).
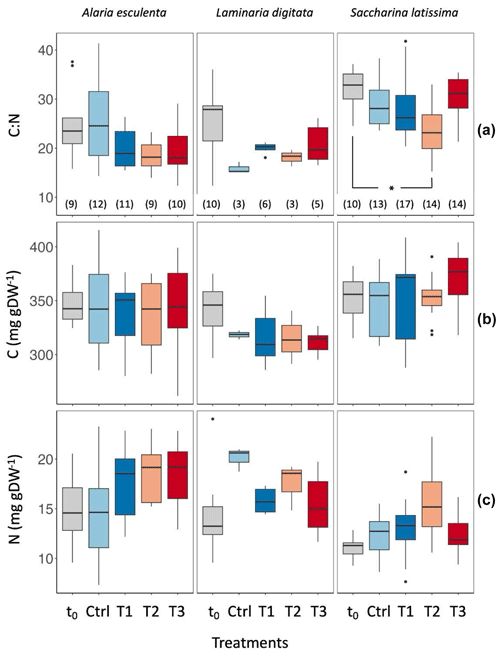
Figure 4(a) Carbon : nitrogen (C : N), (b) carbon contents, and (c) nitrogen contents of Alaria esculenta, Laminaria digitata, and Saccharina latissima exposed to the four treatments, expressed per unit of dry weight (gDW). t0 values correspond to samples taken at the start of the experiment, while Ctrl, T1, T2, and T3 correspond to the final values from organisms maintained in the respective treatments for 6 weeks. The horizontal lines in each box plot represent the median. The whiskers extend to the furthest data points within 1.5 times the interquartile range (the top and bottom of the box). Statistically significant differences are shown with an asterisk (p<0.05). The number in parentheses below each box plot in (a) corresponds to the sample size; this is the same in (b) and (c).
3.3 C : N ratio
The statistical significance of predictor variables species and treatment for C : N, carbon content, and N content were significantly different for species and treatment (p<0.001; Table S4), with the exception of treatment as a non-significant predictor for carbon content. There were no significant interactions between species and treatment. The pairwise comparisons determined that for S. latissima, C : N ratios at t0 ranged from 24.5 up to 37.1 (Fig. 4). No statistical difference was found between t0, the control, T1, and T3 treatment at tfinal (p>0.93, Tables S4, S5). In contrast, C : N ratios of individuals in the T2 treatment were significantly lower than at t0, ranging from 15.2 to 29.5 (Fig. 4a, p=0.045). Although carbon content showed no significant difference across treatments and time (Fig. 4b, p=1), there was a notable increase in nitrogen content in the T2 treatment compared to t0, but it was not statistically significant (Fig. 4c, p=0.06). The C : N ratios and carbon and nitrogen contents of A. esculenta and L. digitata were not significantly impacted by the treatments (p>0.32).
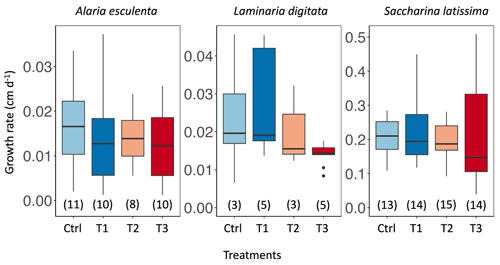
Figure 5Growth rate of Alaria esculenta, Laminaria digitata, and Saccharina latissima exposed to the four treatments during 6 weeks. The horizontal lines in each box plot represent the median. The horizontal lines in each box plot represent the median. The whiskers extend to the furthest data points within 1.5 times the interquartile range (the top and bottom of the box). The number in parentheses below each box plot corresponds to the sample size.
3.4 Growth rate
The growth rates of A. esculenta, L. digitata, and S. latissima were not significantly impacted by the treatments (Fig. 5, p=1, Tables S6, S7). They ranged from 0 to 0.037 cm d−1 for A. esculenta, 0.007 to 0.046 cm d−1 for L. digitata, and 0.040 up to 0.509 cm d−1 for S. latissima. The growth rate of S. latissima was significantly higher than for the two other species for each treatment (p<0.01).
The growth rate of A. esculenta significantly decreased between week 2 and week 6 (p<0.01, Fig. S1a) over time in the control. For S. latissima, significant differences in growth over time were only found in the T3 treatment (p=0.02, Fig. S1b). No intermediate measurements of L. digitata growth rate were taken.
3.5 Gene expression analysis
Principal component analysis of global gene expression revealed a clear contrast between the control and the different treatments for both S. latissima and H. nigripes (Fig. S2). The number of total differentially expressed genes (DEGs, i.e., genes that are either up- or down-regulated when comparing the different treatments to the control) were close between S. latissima (831 including 225 classified; i.e., functionally annotated) and H. nigripes (815 including 144 classified, Fig. 6a) and mostly down-regulated for both species (84 % and 65 %, respectively). For H. nigripes, the majority of overlapping DEGs were found between treatments T1 and T2 (Fig. 6a). Conversely, for S. latissima, the highest number of overlapping DEGs was observed between treatments T1 and T3. In both species, no overlapping genes were identified when comparing the DEGs between treatment pairs T1 vs. T2 and T2 vs. T3 (Fig. 6b).
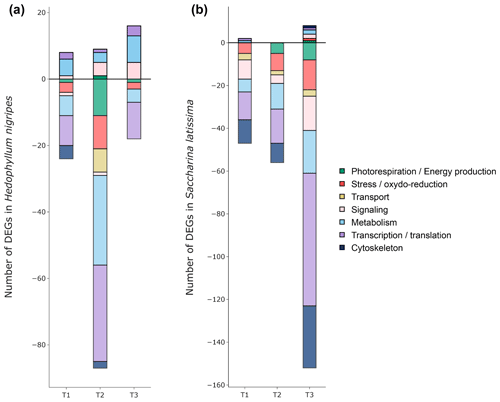
Figure 7Number of classified differentially expressed genes (DEGs) in (a) Hedophyllum nigripes and (b) Saccharina latissima in response to T1, T2, and T3. The upper part of the graph displays up-regulated DEGs and the lower part down-regulated DEGs. Genes were classified with their Pfam and EggNOG annotations (see Sect. 2.7).
The highest number of DEGs were exhibited in the transcription/translation and metabolism classes in H. nigripes (Fig. 7a) and in the transcription/translation and cytoskeleton classes for S. latissima (Fig. 7b). For this last species, the T3 treatments caused the highest number of down-regulated genes (607 including 152 classified) with 60 % belonging to the three classes mentioned above, followed by T1 (314 including 47 classified) and T2 (247 including 56 classified; Figs. 6 and 7). For H. nigripes, 600 genes were observed to be regulated in T2 including 458 genes down-regulated. A substantial portion of the classified down-regulated genes belongs to the transcription/translation and metabolism class (64 %), followed by an approximately equal proportion of genes associated with photorespiration (13 %), stress (11 %), and transport (8 %) and lesser proportions of genes associated with other functions. Genes belonging to the photorespiration/energy production class, involved either in the photosynthesis or respiration process, were found to be down-regulated in H. nigripes in T2 and in S. latissima in T2 and T3. Stress genes were down-regulated in all treatments for both species. The list of DEGs is available in the Supplement.
The analysis of gene expression combined with the investigated physiological parameters shows the ability of Arctic kelps to acclimate to a range of environmental conditions. Indeed, no negative impacts of the treatments were recorded, even according to the highest emission scenarios (SSP5-8.5). This observation confirms that these species, originating from lower latitudes, could thrive in a warmer Arctic. This corroborates the findings of Miller et al. (2024b) that show a tolerant community level response to the same experimental conditions. Further, this also refutes our hypothesis that the combined effects of high temperature, low salinity, and low irradiance will necessarily have a negative impact on their physiology.
4.1 Chl a content
We hypothesized that different species might have different responses to a changing environment. The chl a content of both A. esculenta and S. latissima in the meristem part of the frond showed a significant decrease from t0 to tfinal in the control (−45 % and −70 %, respectively). The same trend was observed in L. digitata, although this is not significant due to the low number of measurements (−57 %, n=3 at tfinal).The high level of chl a measured in early summer matches the anticipation of ice melting and the following increase in turbidity (Aguilera et al., 2002). Decreasing chl a content between June and August has already been reported in situ in Kongsfjorden for S. latissima (Aguilera et al., 2002) with the end of the growth period (Berge et al., 2020).
In contrast to what was observed in the control as well as in the T1 and T3 treatments, for A. esculenta, the chl a content in the warm, less saline, and lower irradiance treatment (T2) remained as high as it was at t0. The decrease in irradiance in this treatment may explain the persistence of elevated chl a levels. PAR is often negatively correlated with chl a content as higher chl a can help maintain elevated photosynthetic rates under reduced PAR (e.g., McWilliam and Naylor, 1967; Zhang et al., 2014). Bartsch et al. (2016) showed that the genus Alaria was more abundant than Laminaria and Saccharina between 10 and 15 m depth. Despite a decrease in irradiance caused by glacial and terrestrial runoff, A. esculenta is the only species that extended its maximum depth (from 15 to 18 m between 1996–1998 and 2012–2014; Bartsch et al., 2016). This shift could be explained by an existing adaptation to low PAR (Niedzwiedz and Bischof, 2023), giving this species a competitive advantage at greater depth. Our findings shed light on the adaptive responses of A. esculenta to low light and, seemingly, tolerance to low salinity and warming, suggesting that this species will most likely be able to withstand future coastal environmental conditions in the Arctic.
The chl a content of L. digitata and S. latissima was also not affected by the treatments. This is in agreement with the study of Diehl and Bischof (2021), where temperature (up to 10 °C), combined with low salinity (down to 25), did not affect the chl a content of S. latissima. However, their growth rate in low-light conditions remained similar to the other treatments. Other physiological processes such as photosynthetic efficiency, or resource allocation, might have been altered to maintain growth rates similar to the control.
4.2 C : N ratio
The C : N ratio of S. latissima was significantly lower in the T2 treatment compared to t0. The decrease in C : N ratio seems driven by an increase in nitrogen uptake. Benthic marine macroalgae and seagrasses from temperate and tropical regions have a mean C : N ratio of 22 (Atkinson and Smith, 1983). In northern Norway, Liesner et al. (2020) reported a C : N ratio of 21 for L. digitata, which is consistent with our measurements for this species as well as for A. esculenta, all treatments and sampling times combined. However, S. latissima exhibited higher ratios with a mean of 29.7±5.5 (t0 and tfinal of the control, T1 and T3 combined), which would suggest nitrogen limitation. While algae in the T2 treatment showed a higher nitrogen content, which is an essential nutrient playing a central role in photosynthesis and protein biosynthesis, the growth rate remained similar to the other treatments. Gordillo et al. (2002) showed higher nitrogen uptake at lower salinity (50 % vs. 100 % seawater) in Fucus serratus that was explained by increased N metabolism. Thus, the higher nitrogen content found here in the low saline T2 treatment (salinity down to 28) could have resulted from increased N metabolism. Indeed, the increase in nitrogen concentration in the macroalgae can induce an increase in the activity of nitrate reductase (Korb and Gerard, 2000). This enzyme catalyzes the first step in the reduction of nitrate to organic forms and protein synthesis. In fact, nitrate concentration in water was higher in the T2 treatment (1.68±0.8 µmol L−1) than in the control (0.87±0.9 µmol L−1, data not shown) during the duration of the experiment. Arctic coastal waters are known to be nitrate-limited (Santos-Garcia et al., 2022). The influx of fresh and potentially more nitrate-rich waters may have induced an increase in the N metabolism of S. latissima which was nitrogen limited. Higher nutrient input from land through coastal erosion and permafrost thawing may benefit this species in various processes such as photosynthesis, biosynthesis, immunity, and/or molecule transport (Campbell, 1988; Meyer et al., 2005).
4.3 Growth rate
We also hypothesized that warming may enhance the growth rate of kelp. None of the growth rates of the three study species were affected by the different treatments over the total duration of the experiment. In contrast, previous studies observed an increase of the growth rate of S. latissima when exposed to warmer conditions (8–10 °C vs. 0–4 °C under replete irradiance; Iñiguez et al., 2016; Olischläger et al., 2017; Li et al., 2020; Diehl and Bischof, 2021). This discrepancy with our results can be explained by the duration of the experiment (7 to 18 d in previous studies vs. 6 weeks here), the study period, and the irradiance. Our study was performed at the end of the peak growth (mid-May to July) and after, while other studies were performed in early July or used sporophytes raised from gametophyte cultures. The growth rate of A. esculenta significantly decreased over time in the control, indicating the gradual end of the growth peak, with many of the kelp starting to senesce (Fig. S1a). For S. latissima, no significant differences were found over time in the control, indicating that the experiment started after the growth peak (Fig. S1b; Berge et al., 2020). In the T3 treatment only, growth was only stimulated during the first 4 weeks of the experiment, suggesting that warming may have prolonged the growth rate of S. latissima after the end of the peak growth period. Further studies may focus on this aspect. The T2 treatment did not induce a growth stimulation, suggesting a negative effect of salinity and/or low irradiance.
4.4 Gene expression
Both H. nigripes and S. latissima exhibited different gene expressions in the control compared to the treatments. The fact that treatments are not clustered separately from each other but are grouped together against the control suggests that the common factor among them, which is the increase in temperature, might be the key influencing factor.
Interestingly, and as we hypothesized, the response to these treatments differed between the two species. The analysis of DEGs shows that the low salinity and irradiance treatment (T2) had a higher impact on the number of genes regulated in H. nigripes, while warming alone (T3) had a higher impact on gene regulation on S. latissima. Since no phenotypic response was observed for S. latissima in T3, this suggests that the observed down-regulation might be an acclimation mechanism, enabling the organism to maintain its main processes. Other parameters could be measured to validate this hypothesis (lipid content, photosynthesis rates, accessory pigment concentrations, etc.). Li et al. (2020) found a regulation of genes involved in reducing the osmotic pressure under low-salinity stress in S. latissima (salinity of 20 vs. 30). We did not observe such results with this species nor with H. nigripes, most likely because the reduction in salinity was much smaller in our experiment (up to −5 here vs. −10 in Li et al., 2020). However, for both species, the T2 treatment induced a down-regulation of photorespiratory genes. This is consistent with previous observations in S. latissima (Machado Monteiro et al., 2019). Under stressful conditions like hyposalinity, kelp may prioritize acclimatization and survival strategies over photosynthesis. Photosynthesis was however not measured during the experiment to validate this hypothesis.
Finally, we noticed a down-regulation, rather than the expected up-regulation, of heat-shock proteins (HSPs), despite their typical induction under abiotic stress (Sørensen et al., 2003). The regulation of HSPs in response to salinity variations occurs to a lesser degree compared to its response to temperature changes (Machado Monteiro et al., 2019). Considering that these species originate from lower latitudes, their current exposure to the low temperatures in the Arctic might induce stress, while future warmer waters may reduce it. For example, the increased growth rate observed for S. latissima could likely be a response to the increased activity of RuBisCO, inducing faster energy production and resulting in the observed N limitation. Further, the down-regulation of stress responses may indeed be a function of reduced energy input to maintain homeostasis in warmer waters. It is important to note, however, that the high temperature exposure treatment approaches the thermal optimum for S. latissima (Andersen et al., 2013) and may likely be close to the peak of enzymatic activity. Thus, increased warming beyond what was tested in this experiment may begin to induce a stress response and the subsequent down-regulation of enzymatic activity.
4.5 Future prospects of Alaria esculenta, Saccharina latissima, Laminaria digitata, and Hedophyllum nigripes in the Arctic
Our findings support the hypothesis that A. esculenta is more likely to be resilient to future changes in irradiance than other kelp species. In particular, our results reveal its competitive advantage at depth, through its increased chl a content. However, no discernible positive impact was observed on its growth rate in low-light conditions. This impact may be more evident earlier in the season, during the peak growth. A. esculenta seems resilient to increasing glacier and river runoff, becoming more dominant in low-light environments such as greater depths (Bartsch et al., 2016). The dominance of a single kelp species in specific regions may carry ecological consequences, as reduced diversity threatens ecosystem resilience (Loreau et al., 2001).
For L. digitata, our results demonstrate neither negative nor positive effects of warming, low salinity, and low irradiance. Franke et al. (2021) also found no effect of a 5 °C warming on the growth rate of this species (control: 5 °C, warming: 10 °C). However, in our study, confusion with H. nigripes at t0 has split the data, making the analysis less robust. Indeed, the individuals could only be identified at the end of the experiment, after cutting the stipe. This led to the removal of 16 individuals from the analysis. The slight decrease in the content of chl a over time, as observed for the other two species in the study, could not be confirmed statistically. Bartsch et al. (2016) found that L. digitata was the only species that experienced a significant increase in biomass between 1996–1998 and 2012–2014 on the entire transect they studied (from 0 to 15 m depth). Current and future conditions in the short term seem optimal for this species. Germination of L. digitata is enhanced at 9 °C compared to 5 and 15 °C (Zacher et al., 2016, 2019), and its growth rate is higher at 15 °C compared to 5 °C and 10 °C (Franke et al., 2021). Although warming alone may be beneficial to this species, its combined effects with other environmental factors as well as biotic interactions (e.g., Zacher et al., 2019) might be detrimental once a certain threshold is reached. Müller et al. (2008) found no difference in the germination rate between 7 and 12 °C but showed that germination under UV of type A and B decreased down to less than 30 % at 12 °C compared to almost 80 % at 7 °C. We acknowledge that the low sample size for L. digitata could obscure the observation of potential negative impacts, and, thus, future studies should aim to increase study sample sizes and the temporal variability of L. digitata resilience to future climate scenarios. Unfortunately, this would have been logistically challenging given our experimental setup.
S. latissima is widely studied throughout the Northern Hemisphere. In the Arctic specifically, several studies indicate that future conditions may favor the expansion of this species. This is supported by findings of enhanced germination with temperatures of up to 12 °C (Müller et al., 2008) and mitigation of the negative effects of UV radiation at high temperatures (12 °C; Heinrich et al., 2015). Our results reveal that S. latissima may benefit from increasing N input from coastal erosion and permafrost thawing that could enhance immunity, photosynthesis, biosynthesis, and/or molecule transport, although this was not measured in this study. S. latissima exhibits a high degree of polymorphism, acclimation, and genetic diversity across populations (Bartsch et al., 2008; Guzinski et al., 2016). For example, its growth shows a high phenotypic plasticity that appears to be constrained within specific seasonal growth patterns in accordance with their environment of origin (Spurkland and Iken, 2011). In the Canadian Arctic, Goldsmit et al. (2021) found that suitable habitat of this species may gain 64 000 km2 by 2050, most of this new area being in the northernmost reaches, where temperature is rising and sea ice is receding. Bartsch et al. (2016) found an increase by 30 times in its biomass between 1996–1998 and 2012–2014 at 2.5 m depth at Hansneset (Kongsfjorden, Svalbard, Norway). S. latissima will most likely benefit from future conditions, although the capacity and time of dispersal, as well as competition with other species, predation, and extreme events, must be considered for population projections.
So far, A. esculenta, L. digitata, and S. latissima have adapted successfully to the shifting Arctic environment, and our results suggest that they might thrive in the conditions expected for 2100. In the short term, these species may well continue to spread in this region. Regarding H. nigripes, Franke et al. (2021) suggested a true Arctic affinity with a sporophyte growth optimum of 10 °C. By 2100, this species might continue to thrive in the Arctic, as evidenced by our gene expression analysis, which suggests efficient acclimation with less stress under future scenarios.
Kelp species will, however, face more competition, grazing, and extreme events, such as high sedimentation rate, ice-scouring, and marine heatwaves (Hu et al., 2020). Around Tromsø (Norway), the massive spread of sea urchins may have caused the ecosystem to collapse into a barren state (Sivertsen et al., 1997). Moreover, with warming, the frequency and intensity of marine heatwaves will increase, which could have important consequences for marine species of Arctic flora and fauna. These potential effects of climate change should be taken into account to better assess the future of Arctic kelp communities. It therefore appears essential to continue to study these communities in order to predict and anticipate future changes and impacts on fisheries and local and indigenous people and on a global scale.
All gene expression data were deposited in the NCBI SRA under the accession number PRJNA1150786: https://www.ncbi.nlm.nih.gov/sra/PRJNA1150786 (LOV, 2024). All data (except for gene expression) are available at https://doi.org/10.1594/PANGAEA.971349 (Lebrun et al., 2024).
The supplement related to this article is available online at: https://doi.org/10.5194/bg-21-4605-2024-supplement.
AL, CAM, SC, PU, SA, RS, JPG, and FG were involved in the fieldwork. AL, CAM, SC, JPG, and FG designed the study. SC, PU, and FG designed the system. The experiment was conducted by AL, CAM, SC, SA, RS, JPG, and FG. AL and ACM performed measurements of the chl a content. AL performed the C : N ratio measurement and the RNA extractions. MM processed transcriptomic data. AL analyzed the data and wrote the first draft of the manuscript, which was then finalized by all co-authors.
At least one of the (co-)authors is a member of the editorial board of Biogeosciences. The peer-review process was guided by an independent editor, and the authors also have no other competing interests to declare.
Publisher's note: Copernicus Publications remains neutral with regard to jurisdictional claims made in the text, published maps, institutional affiliations, or any other geographical representation in this paper. While Copernicus Publications makes every effort to include appropriate place names, the final responsibility lies with the authors.
We are grateful to the staff of the Alfred Wegener Institute (AWI), Institut polaire français Paul Emile Victor (IPEV), and Kings bay for field assistance. We thank Cátia Monteiro for her advice on RNA extraction and Erwan Corre for his expertise and guidance to process transcriptomic data. Thanks are also due to Inka Bartsch, Kai Bischof, and Simon Jungblut for their input, which improved the design and interpretation of this study, and Nathalie Leblond for her help with the CHN analysis. This study was conducted in the frame of the project FACE-IT (The Future of Arctic Coastal Ecosystems – Identifying Transitions in Fjord Systems and Adjacent Coastal Areas).
This research has been supported by Horizon 2020 (grant no. 869154), the Prince Albert II of Monaco Foundation (grant no. ORCA 3051), and the Institut Polaire Français Paul Emile Victor (grant no. ARCTOS 1248).
This paper was edited by Andrew Thurber and reviewed by two anonymous referees.
Aguilera, J., Bischof, K., Karsten, U., Hanelt, D., and Wiencke, C.: Seasonal variation in ecophysiological patterns in macroalgae from an Arctic fjord. II. Pigment accumulation and biochemical defence systems against high light stress, Mar. Biol., 140, 1087–1095, https://doi.org/10.1007/s00227-002-0792-y, 2002.
Ahmed, R., Prowse, T., Dibike, Y., Bonsal, B., and O'Neil, H.: Recent Trends in Freshwater Influx to the Arctic Ocean from Four Major Arctic-Draining Rivers, Water, 12, 1189, https://doi.org/10.3390/w12041189, 2020.
Andersen, G. S., Pedersen, M. F., and Nielsen, S. L.: Temperature acclimation and heat tolerance of photosynthesis in Norwegian Saccharina latissima (Laminariales, Phaeophyceae), J. Phycol., 49, 689–700, https://doi.org/10.1111/jpy.12077, 2013.
Atkinson, M. J. and Smith, S. V.: C:N:P ratios of benthic marine plants, Limnol. Oceanogr., 28, 568–574, https://doi.org/10.4319/lo.1983.28.3.0568, 1983.
Bartsch, I., Wiencke, C., Bischof, K., Buchholz, C. M., Buck, B. H., Eggert, A., Feuerpfeil, P., Hanelt, D., Jacobsen, S., Karez, R., Karsten, U., Molis, M., Roleda, M. Y., Schubert, H., Schumann, R., Valentin, K., Weinberger, F., and Wiese, J.: The genus Laminaria sensu lato: recent insights and developments, Eur. J. Phycol., 43, 1–86, https://doi.org/10.1080/09670260701711376, 2008.
Bartsch, I., Paar, M., Fredriksen, S., Schwanitz, M., Daniel, C., Hop, H., and Wiencke, C.: Changes in kelp forest biomass and depth distribution in Kongsfjorden, Svalbard, between 1996–1998 and 2012–2014 reflect Arctic warming, Polar Biol., 39, 2021–2036, https://doi.org/10.1007/s00300-015-1870-1, 2016.
Bates, D., Mächler, M., Bolker, B., and Walker, S.: Fitting Linear Mixed-Effects Models Using lme4, J. Stat. Softw., 67, 1–48, https://doi.org/10.18637/jss.v067.i01, 2015.
Bauer, S., Grossmann, S., Vingron, M., and Robinson, P. N.: Ontologizer 2.0–a multifunctional tool for GO term enrichment analysis and data exploration, Bioinformatics, 24, 1650–1651, https://doi.org/10.1093/bioinformatics/btn250, 2008.
Berge, J., Johnsen, G., and Cohen, J. H.: Polar night marine ecology, Advances in Polar Ecology, 4, https://doi.org/10.1007/978-3-030-33208-2, 2020.
Bischof, K., Hanelt, D., and Wiencke, C.: Acclimation of Maximal Quantum Yield of Photosynthesis in the Brown Alga Alaria esculenta under High Light and UV Radiation, Plant Biol., 1, 435–444, https://doi.org/10.1111/j.1438-8677.1999.tb00726.x, 1999.
Bolger, A. M., Lohse, M., and Usadel, B.: Trimmomatic: a flexible trimmer for Illumina sequence data, Bioinformatics, 30, 2114–2120, https://doi.org/10.1093/bioinformatics/btu170, 2014.
Bolton, J. J. and Lüning, K.: Optimal growth and maximal survival temperatures of Atlantic Laminaria species (Phaeophyta) in culture, Mar. Biol., 66, 89–94, https://doi.org/10.1007/BF00397259, 1982.
Bray, N. L., Pimentel, H., Melsted, P., and Pachter, L.: Near-optimal probabilistic RNA-seq quantification, Nat. Biotechnol., 34, 525–527, https://doi.org/10.1038/nbt.3519, 2016.
Bushmanova, E., Antipov, D., Lapidus, A., and Prjibelski, A. D.: rnaSPAdes: a de novo transcriptome assembler and its application to RNA-Seq data, GigaScience, 8, giz100, https://doi.org/10.1093/gigascience/giz100, 2019.
Campbell, W. H.: Nitrate reductase and its role in nitrate assimilation in plants, Physiol. Plantarum, 74, 214–219, https://doi.org/10.1111/j.1399-3054.1988.tb04965.x, 1988.
Dankworth, M., Heinrich, S., Fredriksen, S., and Bartsch, I.: DNA barcoding and mucilage ducts in the stipe reveal the presence of Hedophyllum nigripes (Laminariales, Phaeophyceae) in Kongsfjorden (Spitsbergen), J. Phycol., 56, 1245–1254, https://doi.org/10.1111/jpy.13012, 2020.
Diehl, N. and Bischof, K.: Coping with a changing Arctic: mechanisms of acclimation in the brown seaweed Saccharina latissima from Spitsbergen, Mar. Ecol. Prog. Ser., 657, 43–57, https://doi.org/10.3354/meps13532, 2021.
Diehl, N., Roleda, M. Y., Bartsch, I., Karsten, U., and Bischof, K.: Summer Heatwave Impacts on the European Kelp Saccharina latissima Across Its Latitudinal Distribution Gradient, Front. Mar. Sci., 8, https://doi.org/10.3389/fmars.2021.695821, 2021.
Eggert, A.: Seaweed Responses to Temperature, in: Seaweed Biology: Novel Insights into Ecophysiology, Ecology and Utilization, edited by: Wiencke, C. and Bischof, K., Springer, Berlin, Heidelberg, 47–66, https://doi.org/10.1007/978-3-642-28451-9_3, 2012.
Filbee-Dexter, K., Wernberg, T., Fredriksen, S., Norderhaug, K. M., and Pedersen, M. F.: Arctic kelp forests: Diversity, resilience and future, Global Planet. Change, 172, 1–14, https://doi.org/10.1016/j.gloplacha.2018.09.005, 2019.
Finn, R. D., Clements, J., and Eddy, S. R.: HMMER web server: interactive sequence similarity searching, Nucl. Acid. Res., 39, W29, https://doi.org/10.1093/nar/gkr367, 2011.
Franke, K., Liesner, D., Heesch, S., and Bartsch, I.: Looks can be deceiving: contrasting temperature characteristics of two morphologically similar kelp species co-occurring in the Arctic, Botan. Mar., 64, 163–175, https://doi.org/10.1515/bot-2021-0014, 2021.
Gene Ontology Consortium: Gene Ontology Consortium: going forward, Nucl. Acids Res., 43, D1049-1056, https://doi.org/10.1093/nar/gku1179, 2015.
Goldsmit, J., Schlegel, R. W., Filbee-Dexter, K., MacGregor, K. A., Johnson, L. E., Mundy, C. J., Savoie, A. M., McKindsey, C. W., Howland, K. L., and Archambault, P.: Kelp in the Eastern Canadian Arctic: Current and Future Predictions of Habitat Suitability and Cover, Front. Mar. Sci., 8, https://doi.org/10.3389/fmars.2021.742209, 2021.
Gordillo, F. J. L., Dring, M. J., and Savidge, G.: Nitrate and phosphate uptake characteristics of three species of brown algae cultured at low salinity, Mar. Ecol. Prog. Ser., 234, 111–118, https://doi.org/10.3354/meps234111, 2002.
Grabherr, M. G., Haas, B. J., Yassour, M., Levin, J. Z., Thompson, D. A., Amit, I., Adiconis, X., Fan, L., Raychowdhury, R., Zeng, Q., Chen, Z., Mauceli, E., Hacohen, N., Gnirke, A., Rhind, N., di Palma, F., Birren, B. W., Nusbaum, C., Lindblad-Toh, K., Friedman, N., and Regev, A.: Full-length transcriptome assembly from RNA-Seq data without a reference genome, Nat. Biotechnol., 29, 644–652, https://doi.org/10.1038/nbt.1883, 2011.
Guzinski, J., Mauger, S., Cock, J. M., and Valero, M.: Characterization of newly developed expressed sequence tag-derived microsatellite markers revealed low genetic diversity within and low connectivity between European Saccharina latissima populations, J. Appl. Phycol., 28, 3057–3070, https://doi.org/10.1007/s10811-016-0806-7, 2016.
Haas, B. and Papanicolaou, A.: TransDecoder 5.5.0, https://github.com/TransDecoder/TransDecoder/wiki (last access: 15 October 2023), 2015.
Heinrich, S., Frickenhaus, S., Glöckner, G., and Valentin, K.: A comprehensive cDNA library of light- and temperature-stressed Saccharina latissima (Phaeophyceae), Eur. J. Phycol., 47, 83–94, https://doi.org/10.1080/09670262.2012.660639, 2012.
Heinrich, S., Valentin, K., Frickenhaus, S., and Wiencke, C.: Temperature and light interactively modulate gene expression in Saccharina latissima (Phaeophyceae), J. Phycol., 51, 93–108, https://doi.org/10.1111/jpy.12255, 2015.
Hop, H., Wiencke, C., Vögele, B., and Kovaltchouk, N. A.: Species composition, zonation, and biomass of marine benthic macroalgae in Kongsfjorden, Svalbard, Bot. Mar., 55, 399–414, https://doi.org/10.1515/bot-2012-0097, 2012.
Hu, S., Zhang, L., and Qian, S.: Marine Heatwaves in the Arctic Region: Variation in Different Ice Covers, Geophys. Res. Lett., 47, e2020GL089329, https://doi.org/10.1029/2020GL089329, 2020.
Huerta-Cepas, J., Forslund, K., Coelho, L. P., Szklarczyk, D., Jensen, L. J., von Mering, C., and Bork, P.: Fast Genome-Wide Functional Annotation through Orthology Assignment by eggNOG-Mapper, Mol. Biol. Evol., 34, 2115–2122, https://doi.org/10.1093/molbev/msx148, 2017.
Huerta-Cepas, J., Szklarczyk, D., Heller, D., Hernández-Plaza, A., Forslund, S. K., Cook, H., Mende, D. R., Letunic, I., Rattei, T., Jensen, L. J., von Mering, C., and Bork, P.: eggNOG 5.0: a hierarchical, functionally and phylogenetically annotated orthology resource based on 5090 organisms and 2502 viruses, Nucl. Acids Res., 47, D309–D314, https://doi.org/10.1093/nar/gky1085, 2019.
Iñiguez, C., Carmona, R., Lorenzo, M. R., Niell, F. X., Wiencke, C., and Gordillo, F. J. L.: Increased temperature, rather than elevated CO2, modulates the carbon assimilation of the Arctic kelps Saccharina latissima and Laminaria solidungula, Mar. Biol., 163, 248, https://doi.org/10.1007/s00227-016-3024-6, 2016.
Karsten, U.: Research note: Salinity tolerance of Arctic kelps from Spitsbergen, Phycol. Res., 55, 257–262, https://doi.org/10.1111/j.1440-1835.2007.00468.x, 2007.
Karsten, U.: Seaweed Acclimation to Salinity and Desiccation Stress, in: Seaweed Biology: Novel Insights into Ecophysiology, Ecology and Utilization, edited by: Wiencke, C. and Bischof, K., Springer, Berlin, Heidelberg, 87–107, https://doi.org/10.1007/978-3-642-28451-9_5, 2012.
Korb, R. E. and Gerard, V. A.: Nitrogen assimilation characteristics of polar seaweeds from differing nutrient environments, J. Phycol., 36, 38–38, https://doi.org/10.1046/j.1529-8817.1999.00001-114.x, 2002.
Krause-Jensen, D. and Duarte, C. M.: Substantial role of macroalgae in marine carbon sequestration, Nat. Geosci., 9, 737–742, https://doi.org/10.1038/ngeo2790, 2016.
Krause-Jensen, D., Marbà, N., Olesen, B., Sejr, M. K., Christensen, P. B., Rodrigues, J., Renaud, P. E., Balsby, T. J. S., and Rysgaard, S.: Seasonal sea ice cover as principal driver of spatial and temporal variation in depth extension and annual production of kelp in Greenland, Glob. Change Biol., 18, 2981–2994, https://doi.org/10.1111/j.1365-2486.2012.02765.x, 2012.
Krause-Jensen, D., Archambault, P., Assis, J., Bartsch, I., Bischof, K., Filbee-Dexter, K., Dunton, K. H., Maximova, O., Ragnarsdóttir, S. B., Sejr, M. K., Simakova, U., Spiridonov, V., Wegeberg, S., Winding, M. H. S., and Duarte, C. M.: Imprint of Climate Change on Pan-Arctic Marine Vegetation, Front. Mar. Sci., 7, https://doi.org/10.3389/fmars.2020.617324, 2020.
Kwiatkowski, L., Torres, O., Bopp, L., Aumont, O., Chamberlain, M., Christian, J. R., Dunne, J. P., Gehlen, M., Ilyina, T., John, J. G., Lenton, A., Li, H., Lovenduski, N. S., Orr, J. C., Palmieri, J., Santana-Falcón, Y., Schwinger, J., Séférian, R., Stock, C. A., Tagliabue, A., Takano, Y., Tjiputra, J., Toyama, K., Tsujino, H., Watanabe, M., Yamamoto, A., Yool, A., and Ziehn, T.: Twenty-first century ocean warming, acidification, deoxygenation, and upper-ocean nutrient and primary production decline from CMIP6 model projections, Biogeosciences, 17, 3439–3470, https://doi.org/10.5194/bg-17-3439-2020, 2020.
Laboratoire Oceanographie de Villefranche-sur-Mer (LOV): Multifactorial effects of warming, low irradiance, and low salinity on Arctic kelps, https://www.ncbi.nlm.nih.gov/sra/PRJNA1150786, 22 August 2024.
Lebrun, A., Comeau, S., Gazeau, F., and Gattuso, J.-P.: Impact of climate change on Arctic macroalgal communities, Global Planet. Change, 219, 103980, https://doi.org/10.1016/j.gloplacha.2022.103980, 2022.
Lebrun, A., Miller, C. A., Meynadier, M., Comeau, S., Urrutti, P., Alliouane, S., Schlegel, R., Gattuso, J.-P., and Gazeau, F.: Multifactorial effects of warming, low irradiance, and low salinity on Arctic kelps, bundled publication, PANGAEA [data set], https://doi.org/10.1594/PANGAEA.971349, 2024.
Letunic, I., Khedkar, S., and Bork, P.: SMART: recent updates, new developments and status in 2020, Nucl. Acids Res., 49, D458–D460, https://doi.org/10.1093/nar/gkaa937, 2021.
Li, H., Monteiro, C., Heinrich, S., Bartsch, I., Valentin, K., Harms, L., Glöckner, G., Corre, E., and Bischof, K.: Responses of the kelp Saccharina latissima (Phaeophyceae) to the warming Arctic: from physiology to transcriptomics, Physiol. Plant, 168, 5–26, https://doi.org/10.1111/ppl.13009, 2020.
Li, W. and Godzik, A.: Cd-hit: a fast program for clustering and comparing large sets of protein or nucleotide sequences, Bioinformatics, 22, 1658–1659, https://doi.org/10.1093/bioinformatics/btl158, 2006.
Liesner, D., Fouqueau, L., Valero, M., Roleda, M. Y., Pearson, G. A., Bischof, K., Valentin, K., and Bartsch, I.: Heat stress responses and population genetics of the kelp Laminaria digitata (Phaeophyceae) across latitudes reveal differentiation among North Atlantic populations, Ecol. Evol., 10, 9144–9177, https://doi.org/10.1002/ece3.6569, 2020.
Loreau, M., Naeem, S., Inchausti, P., Bengtsson, J., Grime, J. P., Hector, A., Hooper, D. U., Huston, M. A., Raffaelli, D., Schmid, B., Tilman, D., and Wardle, D. A.: Biodiversity and Ecosystem Functioning: Current Knowledge and Future Challenges, Science, 294, 804–808, https://doi.org/10.1126/science.1064088, 2001.
Lorenzen, C. J.: Determination of Chlorophyll and Pheo-Pigments: Spectrophotometric Equations, Limnol. Oceanogr., 12, 343–346, https://doi.org/10.4319/lo.1967.12.2.0343, 1967.
Love, M. I., Huber, W., and Anders, S.: Moderated estimation of fold change and dispersion for RNA-seq data with DESeq2, Genome Biol., 15, 550, https://doi.org/10.1186/s13059-014-0550-8, 2014.
Machado Monteiro, C. M., Li, H., Bischof, K., Bartsch, I., Valentin, K. U., Corre, E., Collén, J., Harms, L., Glöckner, G., and Heinrich, S.: Is geographical variation driving the transcriptomic responses to multiple stressors in the kelp Saccharina latissima?, BMC Plant Biol., 19, 513, https://doi.org/10.1186/s12870-019-2124-0, 2019.
McWilliam, J. R. and Naylor, A. W.: Temperature and Plant Adaptation. I. Interaction of Temperature and Light in the Synthesis of Chlorophyll in Corn, Plant Physiol., 42, 1711, https://doi.org/10.1104/pp.42.12.1711, 1967.
Meyer, C., Lea, U. S., Provan, F., Kaiser, W. M., and Lillo, C.: Is nitrate reductase a major player in the plant NO (nitric oxide) game?, Photosynth. Research.
Millard, S. P.: Hypothesis Tests, in: EnvStats: An R Package for Environmental Statistics, edited by: Millard, S. P., Springer, New York, NY, 149–173, https://doi.org/10.1007/978-1-4614-8456-1_7, 2013.
Miller, C. A., Gazeau, F., Lebrun, A., Gattuso, J.-P., Alliouane, S., Urrutti, P., Schlegel, R. W., and Comeau, S.: Productivity of mixed kelp communities in an Arctic fjord exhibit tolerance to a future climate, Sci. Total Environ., 930, 172571, https://doi.org/10.1016/j.scitotenv.2024.172571, 2024a.
Miller, C. A., Urrutti, P., Gattuso, J.-P., Comeau, S., Lebrun, A., Alliouane, S., Schlegel, R. W., and Gazeau, F.: Technical note: An autonomous flow-through salinity and temperature perturbation mesocosm system for multi-stressor experiments, Biogeosciences, 21, 315–333, https://doi.org/10.5194/bg-21-315-2024, 2024b.
Mistry, J., Chuguransky, S., Williams, L., Qureshi, M., Salazar, G. A., Sonnhammer, E. L. L., Tosatto, S. C. E., Paladin, L., Raj, S., Richardson, L. J., Finn, R. D., and Bateman, A.: Pfam: The protein families database in 2021, Nucl. Acids Res., 49, D412–D419, https://doi.org/10.1093/nar/gkaa913, 2021.
Müller, R., Wiencke, C., and Bischof, K.: Interactive effects of UV radiation and temperature on microstages of Laminariales (Phaeophyceae) from the Arctic and North Sea, Clim. Res., 37, 203–213, https://doi.org/10.3354/cr00762, 2008.
Müller, R., Laepple, T., Bartsch, I., and Wiencke, C.: Impact of oceanic warming on the distribution of seaweeds in polar and cold-temperate waters, 52, 617–638, https://doi.org/10.1515/BOT.2009.080, 2009.
Muth, A. F., Bonsell, C., and Dunton, K. H.: Inherent tolerance of extreme seasonal variability in light and salinity in an Arctic endemic kelp (Laminaria solidungula), J. Phycol., 57, 1554–1562, https://doi.org/10.1111/jpy.13187, 2021.
Niedzwiedz, S. and Bischof, K.: Glacial retreat and rising temperatures are limiting the expansion of temperate kelp species in the future Arctic, Limnol. Oceanogr., 68, 816–830, https://doi.org/10.1002/lno.12312, 2023.
Olischläger, M., Iñiguez, C., Koch, K., Wiencke, C., and Gordillo, F. J. L.: Increased pCO2 and temperature reveal ecotypic differences in growth and photosynthetic performance of temperate and Arctic populations of Saccharina latissima, Planta, 245, 119–136, https://doi.org/10.1007/s00425-016-2594-3, 2017.
Parke, M.: Studies on British Laminariaceae. I. Growth in Laminaria Saccharina (L.) Lamour, J. Mar. Biol. Assoc. UK, 27, 651–709, https://doi.org/10.1017/S0025315400056071, 1948.
Peterson, B. J., Holmes, R. M., McClelland, J. W., Vörösmarty, C. J., Lammers, R. B., Shiklomanov, A. I., Shiklomanov, I. A., and Rahmstorf, S.: Increasing River Discharge to the Arctic Ocean, Science, 298, 2171–2173, https://doi.org/10.1126/science.1077445, 2002.
R Core Team, R: A language and environment for statistical computing. R Foundation for Statistical Computing, Vienna, Austria, https://www.R-project.org/ (last access: 15 October 2023), 2023.
Renaud, P. E., Wallhead, P., Kotta, J., Włodarska-Kowalczuk, M., Bellerby, R. G. J., Rätsep, M., Slagstad, D., and Kukliński, P.: Arctic Sensitivity? Suitable Habitat for Benthic Taxa Is Surprisingly Robust to Climate Change, Front. Mar. Sci., 6, https://doi.org/10.3389/fmars.2019.00538, 2019.
Richter-Menge, J., Overland, J. E., Mathis, J. T., Osborne, E., and Eds: Arctic Report Card 2017: Arctic shows no sign of returning to reliably frozen region of recent past decades, https://doi.org/10.25923/8ntk-7817, 2017.
Roleda, M., Wiencke, C., Lüder, U., and Hanelt, D.: Response of Arctic kelp zoospores to ultraviolet and photosynthetically active radiation in relation to growth depth, in: EPIC38th, 8th Intern. Phycol. Cong., 13–19 August 2005, Durban, South Africa, Phycologia, Phycologia, 44, 13336, 2005.
Santos-Garcia, M., Ganeshram, R. S., Tuerena, R. E., Debyser, M. C. F., Husum, K., Assmy, P., and Hop, H.: Nitrate isotope investigations reveal future impacts of climate change on nitrogen inputs and cycling in Arctic fjords: Kongsfjorden and Rijpfjorden (Svalbard), Biogeosciences, 19, 5973–6002, https://doi.org/10.5194/bg-19-5973-2022, 2022.
Scherrer, K. J. N., Kortsch, S., Varpe, Ø., Weyhenmeyer, G. A., Gulliksen, B., and Primicerio, R.: Mechanistic model identifies increasing light availability due to sea ice reductions as cause for increasing macroalgae cover in the Arctic, Limnol. Oceanogr., 64, 330–341, https://doi.org/10.1002/lno.11043, 2019.
Shiklomanov, I. A. and Shiklomanov, A. I.: Climatic Change and the Dynamics of River Runoff into the Arctic Ocean, Water Resour., 30, 593–601, https://doi.org/10.1023/B:WARE.0000007584.73692.ca, 2003.
Simão, F. A., Waterhouse, R. M., Ioannidis, P., Kriventseva, E. V., and Zdobnov, E. M.: BUSCO: assessing genome assembly and annotation completeness with single-copy orthologs, Bioinformatics, 31, 3210–3212, https://doi.org/10.1093/bioinformatics/btv351, 2015.
Sivertsen, K.: Geographic and environmental factors affecting the distribution of kelp beds and barren grounds and changes in biota associated with kelp reduction at sites along the Norwegian coast, Can. J. Fish. Aquat. Sci., 54, 2872–2887, https://doi.org/10.1139/f97-186, 1997.
Sørensen, J. G., Kristensen, T. N., and Loeschcke, V.: The evolutionary and ecological role of heat shock proteins, Ecol. Lett., 6, 1025–1037, https://doi.org/10.1046/j.1461-0248.2003.00528.x, 2003.
Spurkland, T. and Iken, K.: Salinity and irradiance effects on growth and maximum photosynthetic quantum yield in subarctic Saccharina latissima (Laminariales, Laminariaceae), Botanica Marina, 54, 355–365, https://doi.org/10.1515/bot.2011.042, 2011.
Stroeve, J. C., Markus, T., Boisvert, L., Miller, J., and Barrett, A.: Changes in Arctic melt season and implications for sea ice loss, Geophys. Res. Lett., 41, 1216–1225, https://doi.org/10.1002/2013GL058951, 2014.
Supek, F., Bošnjak, M., Škunca, N., and Šmuc, T.: REVIGO summarizes and visualizes long lists of gene ontology terms, PLoS One, 6, e21800, https://doi.org/10.1371/journal.pone.0021800, 2011.
Vihtakari M: ggOceanMaps: plot data on oceanographic maps using “ggplot2”, R package version 2.0.4, https://mikkovihtakari.github.io/ggOceanMaps/ (last access: 15 October 2023), 2023.
Wiencke, C., Bartsch, I., Bischoff, B., Peters, A., and Breeman, A.: Temperature Requirements and Biogeography of Antarctic, Arctic and Amphiequatorial Seaweeds, J. Botan. Mar., 37, 247–259, https://doi.org/10.1515/botm.1994.37.3.247, 1994.
Zacher, K., Bernard, M., Bartsch, I., and Wiencke, C.: Survival of early life history stages of Arctic kelps (Kongsfjorden, Svalbard) under multifactorial global change scenarios, Polar Biol., 39, 2009–2020, https://doi.org/10.1007/s00300-016-1906-1, 2016.
Zacher, K., Bernard, M., Daniel Moreno, A., and Bartsch, I.: Temperature mediates the outcome of species interactions in early life-history stages of two sympatric kelp species, Mar. Biol., 166, 161, https://doi.org/10.1007/s00227-019-3600-7, 2019.
Zhang, D.-W., Yuan, S., Xu, F., Zhu, F., Yuan, M., Ye, H.-X., Guo, H.-Q., Lv, X., Yin, Y., and Lin, H.-H.: Light intensity affects chlorophyll synthesis during greening process by metabolite signal from mitochondrial alternative oxidase in Arabidopsis, Plant Cell Environ., 39, 12–25, https://doi.org/10.1111/pce.12438, 2014.