the Creative Commons Attribution 4.0 License.
the Creative Commons Attribution 4.0 License.
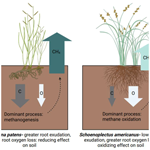
Assessing root–soil interactions in wetland plants: root exudation and radial oxygen loss
Genevieve L. Noyce
Plant rhizosphere processes, such as root exudation and root oxygen loss (ROL), could have significant impacts on the dynamics and magnitude of wetland methane fluxes and other biogeochemical processes but are rarely measured directly. Here, we measure root exudation and ROL from Schoenoplectus americanus and Spartina patens, two plants that have had opposite relationships between biomass and methane flux in field experiments (positive in S. patens, negative in S. americanus). We found contrasting rates of ROL in the two species, with S. americanus releasing orders of magnitude more oxygen (O2) to the soil than S. patens. At the same time, S. patens exuded high amounts of carbon to the soil, and much of the rhizosphere carbon pool was reduced compared to exudates from other wetland species. This work suggests that the relative inputs of O2 and carbon to the rhizosphere vary significantly between wetland plant species, potentially with major consequences on biogeochemical cycling, and highlights the importance of understanding how plant rhizosphere processes mediate soil biogeochemistry at a community level. As global change drivers continue to affect wetlands, future research should consider how feedbacks from plant rhizosphere processes may exacerbate or mitigate coastal wetland methane emissions.
- Article
(4228 KB) - Full-text XML
-
Supplement
(1479 KB) - BibTeX
- EndNote
Rhizosphere processes such as root exudation and root oxygen loss (ROL, a.k.a. radial oxygen loss) can have major impacts on soil biogeochemical cycles and microbial activity (Hinsinger et al., 2006; Haney et al., 2015). Root exudates include soluble organic compounds of varying molecular weights (Oburger and Jones, 2018), which may be readily used by microbes carrying out metabolic pathways including methanogenesis (Megonigal et al., 1999), nitrogen fixation (Li et al., 2021; Hanson, 1977), and sulfate reduction (Hines et al., 1999). Root exudates can have a major influence on carbon cycling in vegetated soil, as a large portion of plant-derived C is transferred to the rhizosphere (Kumar et al., 2006; Lei et al., 2023). Typically, root exudates provide rich substrate for microbial biomass and also have a net-reducing effect on soil redox conditions by fueling O2 sinks directly through increased microbial respiration, as well as driving sulfate reduction, an indirect O2 sink due to the oxidation of the hydrogen sulfide end product (Jensen et al., 2005; Martin et al., 2019). The exact molecular composition of root exudates is rarely studied (Oburger and Jones, 2018), but composition, in addition to total C input, is an important factor for how exudation may impact soil redox conditions. Additionally, the composition of the exudate pool can change depending on environmental contexts such as CO2 concentration (Xiong et al., 2019) and temperature (Wang et al., 2021).
ROL also has a major impact on soil redox conditions, significantly altering biogeochemical cycling by creating a more oxidized soil environment (Hemminga et al., 1988; Li et al., 2024), but the extent of this oxygenation varies at the species level, and thus changes in plant community composition can significantly alter sediment redox conditions (Koop-Jakobsen et al., 2021). ROL is an especially important process among wetland plants, which typically have very high rates of O2 movement to the rhizosphere as an adaptation for growth in anaerobic, saturated soil (Lai et al., 2012). Because of the anaerobic state of wetland soils, O2 derived from ROL is a major driver of aerobic processes in wetland soils (Craft, 2001). ROL is the net result of several plant traits related to gas exchange, such as aerenchyma tissue, and traits linked to ROL vary across and within species (Li et al., 2024). Additionally, diffusion of O2 along and across roots is also affected by plant respiration and other physical root traits including porosity, length, and tissue density (Armstrong and Drew, 2002). ROL is typically greater in daytime, when photosynthesis and stomatal conductance are high (Lai et al., 2012). Many species of monocots develop strong barriers preventing O2 loss under waterlogged conditions (Visser et al., 2000), though this barrier to ROL may come at the cost of nutrient uptake efficiency (Armstrong and Armstrong, 2005; Cheng et al., 2020).
Plant provision of O2 and organic C to wetland soils has far-reaching biogeochemical implications; the soil microbial community drives biogeochemical cycling, and plant functional type has been linked to microbial community dynamics (Robroek et al., 2016). The well-known redox ladder concept holds that (1) heterotrophic microbes compete for organic C electron donors, and (2) their competitive ability is determined by the free energy yield of respiration. That result is that terminal electron acceptors (TEAs) are consumed in predictable order: O2> NO3> Fe(III) > SO4 > CO2/CH4 (methanogenesis). Root C exudates favor TEA depletion and drive the system towards methanogenesis, a microbial respiration pathway that is never TEA-depleted if organic C is available. In contrast, ROL drives the system away from methanogenesis by regenerating oxidized TEAs such as NO3 and Fe(III), and, especially in tidal systems, SO4 oxidation of these other molecules typically proceeds faster than aerobic oxidation of CH4 (Bethke et al., 2011). Despite the cascading effects on ecosystem biogeochemistry, our current understanding of the balance of root oxygenation to root exudation in wetland plants, and thus the resulting redox impact of plant roots on anaerobic soils (Fig. 1), is limited.
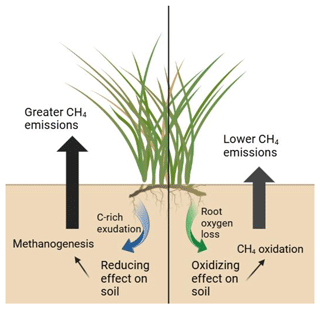
Figure 1A schematic representing the varying rhizosphere processes discussed in this paper and how they directly impact soil methane fluxes. Created in BioRender using graphics from the UMCES IAN media library.
Wetlands can be a major sink or source of carbon (C) depending on environmental conditions, which has wide implications for global climate change (Noyce et al., 2023; Noyce and Megonigal, 2021; Mitsch et al., 2013; Lolu et al., 2020). Due in part to wetland plants' capacity for transporting carbon dioxide (CO2) from the atmosphere to the anaerobic soils in which their roots are located (Kumar et al., 2006), wetlands may store soil organic C at very high levels, with some estimates putting them among the top C-storing terrestrial ecosystems (Valach et al., 2021; Nichols and Peteet, 2019; Mcleod et al., 2011). However, many wetlands are a source of C to the atmosphere through enhanced production of methane (CH4), the rates of which may depend on soil environmental conditions, which are influenced by root exudation (Noyce and Megonigal, 2021). In addition, oxygen inputs can prime aerobic decomposition, causing a loss of previously preserved C (Noyce et al., 2023). As global change impacts the productivity and community composition of wetland plants, rhizosphere processes will likely change as well (Jiang et al., 2020; Zhai et al., 2013), exacerbating species-level differences in root exudation and oxygenation.
As sea levels rise, Schoenoplectus americanus – a salt- and flood-tolerant C3 sedge – has encroached into areas on the Atlantic coast of the United States formerly dominated by Spartina patens, a C4 grass (Mueller et al., 2020). Additionally, Phragmites australis (ssp. australis), an invasive reed, has encroached on native wetland plant habitat in the region (Sciance et al., 2016). We have previously proposed that species-level differences in rhizosphere processes drive observed differences in wetland soil biogeochemistry, where S. patens-dominated areas have higher methane emissions than S. americanus-dominated sites (Noyce and Megonigal, 2021; Mueller et al., 2020), but direct measurements of root exudates and ROL remain elusive in a field environment. Here, we conduct a growth chamber study to identify root-associated metabolites and root exudation rates from these three species (as well as Spartina alterniflora, another common wetland plant for which extensive data are available) and estimate ROL in S. patens and S. americanus. For further experimentation on ROL and the effect of exudates, we focus on S. patens and S. americanus, the two most dominant plants at Kirkpatrick Marsh. We follow with a corresponding incubation experiment to estimate the role that exudate composition plays in methane production in wetland soils colonized by either S. patens or S. americanus. Based on past field data showing greater methanogenesis in S. patens-dominated areas (Mueller et al., 2020), we hypothesized S. patens will have lower rates of root oxygenation and higher rates of root exudation than S. americanus and that the cocktail of exudates coming from the roots of S. patens will drive higher rates of methanogenesis compared to the S. americanus exudate pool.
2.1 Growth conditions
We harvested Schoenoplectus americanus and Spartina patens rhizomes by hand from Kirkpatrick Marsh, a brackish microtidal marsh located on the Rhode River, a subestuary of the Chesapeake Bay, MD, United States, in early July 2023. We collected Phragmites australis (subspecies australis) rhizomes in the same area in August 2023 and acquired Spartina alterniflora plants from Delmarva Native Plants (Hurlock, MD). We placed rhizomes in shallow trays containing Dakota reed and sedge peat hydrated with deionized (DI) water. This cohort of plants was used for all analyses. Once plants from these rhizomes reached >20 cm height, we carefully separated individuals from each other with at least 3 cm of rhizomes and moved them into rhizoboxes ( cm (), inside dimensions) purchased from Vienna Scientific (Alland, Austria) containing the same peat. We monitored growth in the rhizobox for 1 week by measuring plant height every 2–3 d (Fig. S1 in the Supplement) before all analyses, keeping soil consistently moist. Rhizoboxes were half-submerged in distilled water adjusted to the level of near site salinity (∼10 ppt) with InstantOcean between samplings, and the bottom 10 cm of soil was fully saturated. A total of 10 plants from each species at a time were acclimated in the rhizoboxes for 1 week in growth chambers at the Smithsonian Environmental Research Center. Full-spectrum growth lights (∼400 µmol m−2 s−1 PPFD, photosynthetic photon flux density) in the growth chamber were on during daytime for 16 h and off during the nighttime period for 8 h during the root exudate phase of analysis (August–December 2023) and switched to 12 h of light and 12 h of darkness during root O2 analysis (January–April 2024). Temperature was set to 25 °C during the light period (referred to as “day”) and 18 °C during the dark period (referred to as “night”). Humidity was not manipulated but was typically higher in the day period due to temperature (70 %–85 %) and lower (60 %–70 %) during the night period. Conditions were similar to summer field conditions at Kirkpatrick Marsh, where average humidity ranges from 65 %–85 % and the average high temperature for July is 31 °C (Langley et al., 2009). We tracked humidity and temperature using three separate devices: an Onset HOBO logger situated above the plants; a custom-built Arduino microcontroller connected to K30 (Senseair) and BME680 (Adafruit) sensors (measured ambient CO2, temperature, air pressure, humidity, and volatile organic compounds); and a wall-mounted Titan Saturn 5 Digital Environmental Controller.
2.2 Root exudation
Root-associated metabolites were collected using a combination of soil and hydroponic methods (Fig. 2). It is important to note that all methods were non-sterile for all or some of the sampling period, meaning some identified metabolites are related to soil and rhizosphere microbial processes. We will use the phrase “root-associated metabolites” to refer to all products detected using both methods, which likely include root exudates and rhizosphere soil microbial products.
2.2.1 Soil sampling
For the soil method, an individual adventitious root, between 5–15 cm in length, was placed between two pieces of clean unbleached wax paper within the rhizobox, while the rest of the roots were left in the soil. We noted the root's color, depth in the soil, and presence or absence of hairs. The chosen root was thoroughly rinsed with DI to remove soil and gently patted dry with a Kimwipe. We then placed a Whatman GFF (25 mm diameter) filter saturated with DI water over the top of the root (extended from the root tip through the apical zone) between the two pieces of wax paper (Neumann, 2007). The rhizobox was sealed and returned to the growth chamber under the growth lights for ∼1.5 h before the filter was retrieved and immediately frozen at −80 °C prior to analysis. We repeated this process three times during the day (beginning >2 h after lights turned on) and three times during the night period (beginning >5 h prior to lights turning on), for each of the 10 replicate plants per species. On the day of sampling, each plant's height, root depth, sampled root depth (i.e., the depth at which the filter was placed), and color of the sampling root were observed. Following sampling, plants were harvested and dried for aboveground and belowground biomass.
2.2.2 Hydroponic sampling
Following filter analysis, plants were removed from the soil-filled rhizobox, and the roots were thoroughly (but carefully, to prevent tissue damage) washed with DI water. Roots were then allowed to soak in DI water overnight before being rinsed again. We then sampled root-associated metabolites hydroponically in 30 mL DI water in sterile containers for 1.5 h. Hydroponic root-associated metabolites were filtered (0.22 µm) and separated into two portions, with one aliquot for metabolomic analysis and one aliquot to be assessed for total organic carbon (TOC) using a UV–VIS spectrophotometer at 254 nm after Oburger et al. (2022). A subset of data collected via TOC spectrophotometry was also assessed on a Shimadzu TOC analyzer (Fig. S2), and spectrophotometrically derived TOC values were adjusted according to that relationship.
2.2.3 Metabolomic analysis
For metabolomic analysis, polar root-associated metabolites were extracted from filters by soaking in ultra-pure LC-MS Grade distilled water, followed by centrifuging at 7900 rpm for 15 min at 4 °C. The supernatant was then passed through a 0.22 µm filter and again frozen at −80 °C. All metabolomics samples were brought to the Molecular Characterization and Analysis Complex (MCAC) at the University of Maryland Baltimore County (UMBC), where they were processed for untargeted metabolomics on a quadruple time-of-flight (QTOF) mass spectrometer (MS) equipped with a Dionex UltiMate 3000 UHPLC. For each sample, two aliquots were assessed, first in negative and then in positive ionization mode. Compound identification was carried out by MCAC using Bruker MetaboScape software, based on peak, retention time, and score, with the Bruker MetaboBASE Plant Library and the Natural Products Atlas (NPA). Additional details on the untargeted metabolomics workflow are reported in the Supplement. Compounds were selected for further analysis as potential root exudates if they had been reported in the Reference Metabolome Database for Plants (RMDP; https://www.biosino.org/RefMetaDB/, last access: 5 January 2024; Shi et al., 2024) as a known plant metabolite. For all compounds, we calculated nominal oxidation state of carbon (NOSC) after Gunina and Kuzyakov (2022).
2.3 Incubation
Incubations were conducted on soil collected from 2–10 cm depth within a P. australis-dominated area of Kirkpatrick Marsh in August 2023. We removed all large roots and debris from the soil and then homogenized it. We collected a root exudate “stock” solution from two target plant species, S. patens and S. americanus, using 10–20 plants from each species. Roots from the plants were thoroughly cleaned and placed in 100 mL DI water, as in the hydroponic sampling above. TOC in the stock solution was tested on the spectrophotometer as above and diluted with DI to reach a final concentration of 4 mg L−1 TOC of the stock solution for both species. We combined 3 g wet soil with 10 mL DI water in 15 serum vials and flushed the vials with N2 for 15 min to ensure the headspace was anaerobic. We placed vials in a dark incubation chamber set to 25 °C for 2 weeks to acclimate, following Girkin et al. (2018). Samples were kept on a shaker at a low speed (85 rpm) to keep sediment from settling out and maintain homogeneity of the slurries. At the end of 2 weeks, we measured baseline O2 to ensure an anoxic environment within the jars and initial headspace methane. We measured O2 using a PyroScience Firesting sensor and 500 µm needle-tip O2 mini sensor. We measured methane by removing 3 mL headspace from each jar and injecting it into a Shimadzu Nexis GC-2030. We then randomly assigned each serum vial to one of three groups, S. patens, S. americanus, or control, taking care to ensure that headspace baseline average methane and O2 were consistent among the three groups. In week 1, we added 1 mL stock concentration of their given species (or an injection of DI in the control group) daily for 5 d, followed by methane and O2 analysis on the fifth day as above. In week 2, samples received a daily addition of 1.5 mL of stock, followed by analysis, and in week 3, samples received a daily 2 mL addition of stock. We then waited 2 weeks with no stock additions and carried out a final gas sampling. At the end of the experiment we sampled the slurry for dry mass and loss on ignition (LOI) at 450 °C for 4 h.
2.4 Oxygen
2.4.1 Methylene blue
We assessed location of root oxygenation within the root mass of S. patens and S. americanus using the methylene blue method after Armstrong and Armstrong (1988) in three plants of each species. We used the results of this method to determine the extent of barriers to ROL in each species and inform areas of the rooting mass to focus on for planar optode analysis, described below.
2.4.2 Planar optodes
We used 2-D planar optode imaging to characterize and quantify ROL in S. americanus and S. patens. We imaged root oxygenation using a PreSens Precision Sensing (Regensburg, Germany) VisiSens TD camera setup in the same growth chamber environment. We carried out weekly two-point calibrations with anoxic soil (0 %) and water in equilibrium with atmosphere (100 % atm O2). We measured root O2 in rhizoboxes containing individual samples of either S. americanus or S. patens (n=8 for each species) in Dakota reed and sedge soil. For some additional S. patens plants (n=5), we carried out further imaging on roots grown in rhizoboxes on soil that was autoclaved at 121 °C with a triple pre-vacuum cycle in an attempt to reduce microbial sediment O2 demand and better quantify ROL. For a full rationalization of the use of planar optode technology to image wetland plant radial O2 loss, see Koop-Jakobsen et al. (2018) and de la Cruz Jiménez et al. (2021). Each plant was grown in 12 h light and 12 h darkness, and the overhead lights went off for 1 min as images were collected every hour for 3–4 d per plant. The first 24 h period after the O2-sensing optode foil was placed was excluded from the dataset. Per plant, this resulted in at least 24 images captured in light and 24 in darkness (72 h cycle, subtracting the first 24 h). Notably, using this method, O2 can only be imaged if the rate of O2 release exceeds the rate of sediment O2 demand (SOD). We periodically measured soil O2 demand (SOD) in the test soils by injecting known quantities of distilled water in equilibrium with atmosphere (∼21 % O2) at the soil–foil interface and measuring the length of time until complete consumption of the O2. Planar optode imaging of root O2 in a rhizobox environment can overestimate ROL (Frederiksen and Glud, 2006) but is still useful as an estimate, especially for inter-species comparison of data collected using the same method.
2.4.3 Image analysis
Images were exported from the VisiSens software and initially analyzed for change in O2 using the raster analysis package “terra” (Hijmans, 2023). For each plant, we assessed change in average pixel value from the day compared to the night period and standard deviation of pixels across each 24 period. We measured the average percentage of O2 in both day and night periods around the root cap for each plant and recorded the peak and average O2 concentration in root-influenced regions (regions of interest, or ROIs) in the light and dark periods, as well as average O2 concentration in comparable ROIs in the background bulk soil.
Before and after foil placement, we took RGB images of the rooting zone and created root diagrams by tracing root area behind the foil. From these diagrams, we calculated root surface area assuming root diameter based on root width in the images (treating roots as cylinders). We combined SOD measurements with differences in percentage of O2 between background conditions and root conditions to estimate the rate of O2 release from the plant root in the soil.
2.5 Statistical analysis and data availability
Differences in the root exudate profiles produced by the four different plant species were assessed using principal component analysis (PCA) carried out in R, and top contributing root-associated metabolites to differences between groups (species, light vs. darkness, sampling method) were identified using the package “factoextra” (Kassambara and Mundt, 2020). Single-factor species-level differences (TOC, average NOSC) were assessed by Tukey's honest significant difference (HSD) test or Dunn test (Dunn test carried out using “rstatix” package; Kassambara, 2023), depending on the distribution of data, assessed by the Shapiro–Wilk test. Initial analyses (network analysis, heatmap creation) and data exploration were carried out using MetaboAnalyst 6.0 (https://www.metaboanalyst.ca, last access: 5 January 2024), though all final data analyses were carried out in R. Raw mzML files of all detected root-associated metabolites in the four plants are freely available and hosted in the MassIVE database managed by UC San Diego's Center for Computational Mass Spectrometry (MassIVE ID MSV000094652, University of California San Diego, CA, USA). Incubation data (headspace methane) over time were assessed via linear model with treatment (S. americanus, S. patens, or control) as a block factor and with Tukey's t test comparing the three treatments at each time point. Differences in ROL per plant species were assessed by t test, after checking for normality.
3.1 Root exudation
Metabolites identified using both methods included amino acids, organic acids, sugars, fatty acids, and secondary metabolites. We saw greater species-level separation in the hydroponic metabolites across all compounds (Fig. 3) but greater species-level separation in the single-root soil filter metabolites when considering only compounds previously reported as plant metabolites. Compounds that differed most between species in the all-metabolites dataset included secondary metabolites with antifungal and antibacterial properties. Compounds that differed most by methodology included aspartic acid, which was found in the hydroponic samples for all species but not the filter samples. For variation at the species level, sucrose was found more commonly in samples collected on filters and was at the highest levels in P. australis and identified in some individuals in both Spartina species but rarely detected in the S. americanus root exudate pool. Among root-associated metabolites commonly reported as root exudates, those that drove species-level variation in the hydroponic dataset included aspartic acid (8 % contribution to PCA-2) and sucrose (7.3 %) and aristeromycin M (11 % contribution to PCA-1) and histidine (4 %). Aspartic acid was highest in S. americanus, aristeromycin M was greatest in P. australis, and thymine was greatest in S. alterniflora. Other compounds with significant differences by species (identified using MetaboAnalyst) are shown in Figs. S5–S6. Of the 642 compounds identified, we flagged 75 previously reported as plant metabolites in RMDP. The 75 known plant metabolites detected included those commonly seen within root exudate pools such as sucrose, succinic acid, pyruvic acid, valine, alanine, leucine, phenylalanine, arginine, and aspartic acid (Vranova et al., 2013), as well as nicotinic acid, salicylic acid, and benzoic acid (see Table S1 for full list). All compounds discovered in the root-associated metabolite pool fell into the following classes (ordered by relative abundance): organoheterocyclic compounds; lipids; nucleosides, nucleotides, and analogues; organic oxygen compounds; isobenzofurans; benzenoids; organic acids; organic nitrogen compounds; phenylpropanoids and polyketides; coumarins; and phenols. When classed by compound type, there were significant differences at the species level in relative abundance for benzenoids (S. patens > S. alterniflora and P. australis), coumarins (S. patens >P. australis), and organic acids (S. alterniflora > P. australis; S. patens > all).
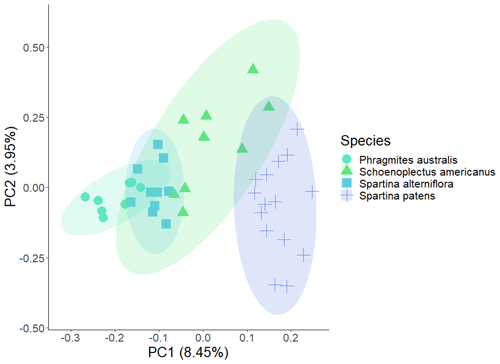
Figure 3PCA (principal component analysis) on all root-associated metabolites (n=647) collected hydroponically from wetland plant roots during daytime. Each point represents one replicate of a species.
Total TOC exudation rate per root biomass was greater in S. patens than any other species (p<0.05, Dunn's test). The P. australis exudation rate was lower on average but showed greater variability, while S. alterniflora and S. americanus had similarly low exudation rates (Fig. 4). The average NOSC of the exudate pool (weighted by amount of each compound exuded) was lowest in S. patens (p<0.05, Tukey's HSD test) and highly constrained across the other three species (Fig. 5).
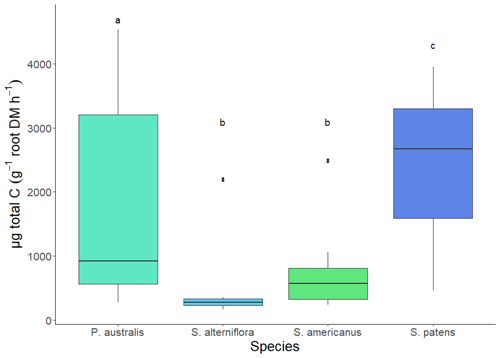
Figure 4Hydroponic TOC (total organic carbon) exudation rate of 4 wetland species (Spartina patens, Phragmites australis, Schoenoplectus americanus, and Spartina alterniflora) in daytime. Letter denotes significance (p<0.05) via Dunn test (non-parametric).
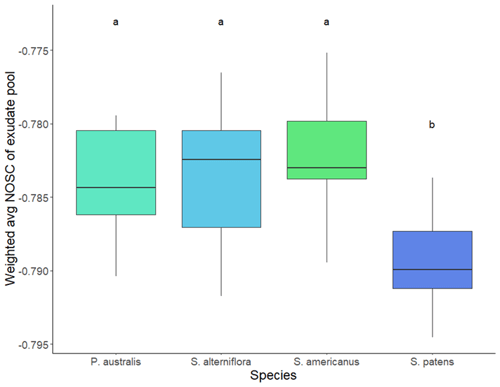
Figure 5Average NOSC (nominal oxidation state of carbon) of hydroponically sampled root exudates in daytime, weighted by relative abundance of compound exuded. Letters denote significance (p<0.05) by Tukey's HSD test.
Diel patterns of root-associated metabolites collected using the soil filter method depended on species. In Spartina alterniflora, relative abundance (calculated from peak intensity) of all detected exudates summed was greater in the day (p=0.03), while in all other species, there was no difference in relative abundance of detected exudates based on time (p=0.8 for S. patens; p=0.9 for S. americanus; p=0.09 for P. australis). In all plants, there was lower diversity of root-exudate compounds during the night (Fig. S3).
3.2 Incubation
Daily addition of root-associated metabolites of any species to the peat slurry resulted in increased headspace methane compared to the control groups. The response varied the greatest in S. americanus, which had total headspace methane values ranging from 0–16 000 ppm across the experiment (Fig. 6). In weeks 1 and 2, there were no significant differences between either treatment and the control. In weeks 3 and 5, headspace methane in S. patens-exudate treated jars was significantly greater than the control (p<0.05), and S. americanus-exudate treated jars were somewhat greater than the control (p<0.1). There was never any difference between the S. americanus and S. patens treatments.
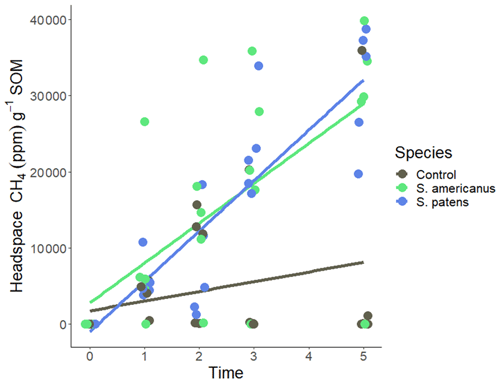
Figure 6Headspace methane (ppm) normalized to soil organic matter (SOM) over time (weeks) in incubation serum vials. Vials contained site soil and DI in slurry. A quantity of 1 mL of root-associated metabolite stock solution collected hydroponically from plants (or DI for controls) was added to each vial per day for 5 d in week 0–1, 1.5 mL d−1 in week 1–2, and 2 mL d−1 in week 2–3. Nothing was added to the vials in weeks 3–5.
3.3 Oxygen
Methylene blue analysis revealed greatest O2 release from the tips of adventitious roots in S. americanus and from the fine roots and root hairs in S. patens (see Fig. S8 for images showing typical patterns of oxygenation). In both species, young light-colored roots consistently showed the greatest oxygenation. Most S. patens roots showed little barrier to ROL compared to S. americanus, which had regions of blue found only at root tips.
When assessed during daytime, soil O2 demand (SOD) in the rhizobox bulk soil (Dakota reed and sedge peat) was high. Accounting for soil porosity of 0.45, SOD occurred at a rate of 812 nmol L−1 s−1. At night, at lower temperatures, the rate was 108 nmol L−1 s−1. SOD was higher deeper in the soil column. Based on this, we focused our planar optode imaging efforts on the top 10 cm of soil. In the autoclaved peat, there was less of a difference between SOD in the night and day periods, and SOD was much lower overall compared to the un-sterilized peat (Table 2). However, once an S. patens plant was added to the rhizobox, SOD increased rapidly over the acclimation period prior to ROL analysis and was on par with the un-sterilized peat within 3–5 d.
Table 2Average (standard deviation) soil oxidation demand (SOD) in the rhizoboxes. The SOD of sterilized peat is comparable to un-sterilized levels within 3 d of planting.

In S. americanus, zones of ROL were most evident on young adventitious roots that were white in color, as in the methylene blue data. Not all roots within the extent of the optode foil had associated ROL. Of the 16 plants imaged over three 24 h light–dark cycles, we collected nine regions of ROL (seven roots across five plants from S. americanus, one plant with two roots in S. patens) that qualified for further analysis (associated with a root, smooth soil surface without macropore present, diel pattern repeating at least once).
In the majority of S. americanus roots analyzed (six of seven), we saw slightly greater buildup of O2 in root-associated regions at night. O2 in regions around roots associated with oxygenated zones built up to highest levels near dawn and dropped to lowest levels around dusk but was always greater than background levels. This probably reflects greater sediment O2 demand in the daytime driven by increased daytime temperatures in the growth chamber. Background (bulk soil) O2 followed a similar diel pattern, though it was less pronounced than around the roots. However, there is likely much greater microbial biomass around roots due to the inputs of carbon and O2 fueling microbial processes, so O2 consumption in the rhizosphere region may be higher than in the bulk soil. In S. patens, we rarely observed regions of O2 build-up behind the optode foils, implying that for most roots, although ROL occurs (as demonstrated by the methylene blue results), it never exceeded the rate of SOD (Tables 2, 3) in the rhizoboxes. However, for one plant of S. patens (of eight assessed on un-sterilized soil), with the optode foil placed on two thicker-than-average roots, O2 buildup was detectable at similar levels of S. americanus in peat. Accounting for root area (estimated from root length and diameter in images with area calculated assuming roots as cylinders), ROL in S. americanus ranged from 65–621 nmol m−2 s−1 and was much greater on average during the high-temperature day period (average =391 nmol m−2 s−1) than the night period (average =62 nmol m−2 s−1). Our observed buildup of O2 in darkness (Fig. 7) was driven by a precipitous decrease in sediment oxygen demand during the night that overshadowed the smaller increase in ROL due to photosynthesis during the day. This drop in SOD is due to the 7 °C difference in temperature between the night and the day period. S. patens ROL was largely not detectable in the high SOD peat background, implying ROL below the rate of SOD, but for the one plant that had detectable ROL in peat on two roots, the rate was much greater during the daytime, at 166 nmol m−2 s−1, and remained undetectable at night. Although autoclaving peat did initially reduce SOD, S. patens ROL was similarly undetectable on this growth medium during both day and night on all but one plant, due to the increase in SOD after introducing a plant (Table 2). Note that, because not all roots showed signs of ROL during methylene blue testing, our numbers when scaled up to the per-root-area level likely represent an overestimation.
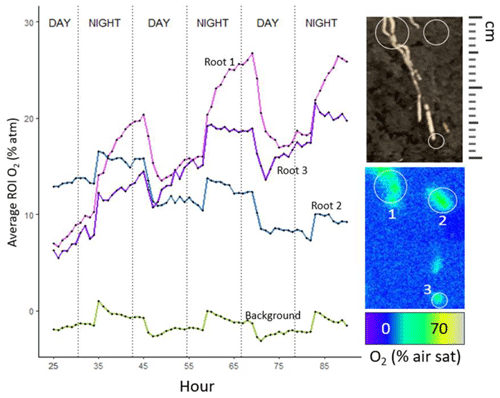
Figure 7Data from a characteristic S. americanus plant with several roots showing ROL over 72 h, with images taken every hour. Panel images at R show region of interest around the root during select time periods, as well as an image of the roots located in the ROI. Note that root images were taken prior to the 72 h collection, and exact locations of roots likely moved as growth occurred over the 4 d period.
Table 3Average (and standard deviation) O2 data from each species for n roots. ROL was determined based on the size of the oxygenated zone within a stable period of at least 3 h. Images from plants without oxygenated zones (n images =3 for S. americanus, n=11 for S. patens) were excluded. Percentage (%) of O2 in ROI refers to root-associated O2 (at least 3 h) as a percentage of atm on peat soil or in an 80 : 20 sand : peat mix (for S. patens only, in order to quantify ROL in lower SOD conditions).
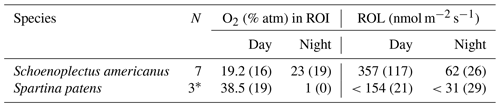
* The S. patens plants likely represent maximum values, as all other plants had undetectable ROL.
4.1 Combined effects of carbon and oxygen
Root exudation and ROL varied across species, with S. patens exhibiting the greatest root exudation rate, as well as the most reduced rhizosphere-associated metabolite pool, while S. americanus consistently showed greatest oxygenation of the rhizosphere. Our data clearly demonstrate that S. patens exudes a greater amount of C per O compared to S. americanus. Interestingly, the composition of the rhizosphere-associated metabolite cocktail alone does not result in different methane yields between the two species (Fig. 6), but differences in the rate of exudation overall, or over longer timescales, and the addition of varying rates of soil oxygenation by roots, likely have differential effects on soil biogeochemistry between the two species. At a marsh scale, these processes are altered by sea level rise, resulting in community change from S. patens to S. americanus in mesohaline regions of Chesapeake Bay (Mueller et al., 2020), with increased ROL and reduced root exudation in S. americanus likely leading to lower methane fluxes from the soil and representing a negative feedback cycle.
We have previously shown that field-scale methane fluxes (measured using a static chamber system) are higher from sites dominated by S. patens compared to sites dominated by S. americanus (Noyce and Megonigal, 2021). Similarly, prior work has also found higher net methane emissions from mesocosms with S. patens compared to mesocosms with S. americanus (Mueller et al., 2020). This has led to a hypothesis that the differential response is due to the cumulative effect of plant rhizosphere processes being measurable through fluxes the soil surface, with S. americanus acting as a “net oxidizer” on the soil, reducing methane emissions through rhizospheric aerobic methane oxidation, while S. patens has the opposite effect as a “net reducer”, increasing methane emissions by supplying carbon substrates to the rhizosphere (Noyce and Megonigal, 2021). Our results support the mechanisms underlying this theory, with S. americanus exuding the lowest TOC per unit biomass of any species studied, and S. patens the most (Fig. 3), while S. patens also released considerably less O2 from roots than S. americanus. Increased ROL in S. americanus resulted in persistent oxic zones in the rhizosphere, which were absent in S. patens; as aerobic CH4 oxidation only depends on the supply of CH4 and O2, these oxic zones may increase rates of CH4 oxidation (Philippot et al., 2009). Some of the lower rates of ROL seen in S. patens could be a result of greater microbial respiration driven by the increased TOC release, though further research would be needed to evaluate how these two processes impact the microbial community at the rhizosphere level. Nevertheless, the lack of persistence of O2 in the S. patens rhizosphere shows that S. patens clearly has a less oxidizing effect on the soil than S. americanus, where O2 released from roots persists through the day and night period. Additionally, the NOSC of the rhizosphere-associated metabolite pool from S. americanus is more oxidized, which may result in a less reduced rhizosphere environment even in the absence of ROL, again lowering methanogenesis and thus methane flux from the soil. S. patens had the lowest NOSC of any of the four species studied, implying that the TOC pool coming from the roots of S. patens is more reduced overall, likely leading to greater consumption of soil oxidizing power. This more reduced pool may also be a greater sink for O2 released by roots, resulting in the inability to image ROL in the majority of the S. patens samples.
4.2 Comparison to other studies
Input rates of total organic carbon (ranging from 200–4300 µg C per g root DM h−1, where DM is dry matter) varied between the four species on a per-root-biomass area but were comparable to other submerged plant species such as Oryza sativa (rice), which typically exudes between 500–4500 µ g C per g root DM h−1 (Aulakh et al., 2001; Xiong et al., 2019). Detected compounds across species were also similar to those found as root exudates in other systems, with organic acids making up the majority of detected compounds that differed on a species level, similar to Typha latifolia and Vetiver zizanioides (Wu et al., 2012). Unlike some other studies of root exudates in other wetland species (Wu et al., 2012; Chen et al., 2016), we did not detect oxalic acid in our rhizosphere-associated metabolite pool for any species, possibly due to methodological differences, as both the other studies were conducted on plants grown entirely under hydroponic conditions. Growth conditions have been shown to play a significant role in structuring the root exudate composition of various plants, and many of the compounds and compound classes we found in our setup are similar to other metabolomic profiles of the root exudate pool collected using a nonsterile setup (McLaughlin et al., 2023). Organic acids commonly found in our rhizosphere pool were nicotinic acid (reported in Arabidopsis thalania root exudate pool by Pantigoso et al., 2020), succinic acid and fumaric acid (both reported in maize exudate pool by Nardi et al., 1997), and pyruvic acid (reported along with fumaric acid in agricultural grasses by Maurer et al., 2021). Like other studies (reviewed in Vranova et al., 2013), commonly found amino acids in our samples included aspartic acid (found at highest levels in S. americanus), leucine, valine, arginine, and histidine, all of which occurred equally across the four species. Addition of amino acids to soil has been shown to reduce methane yield (Liu et al., 2021), and overall, amino acid relative abundance was higher in S. americanus than other species (Table S1). Organic acids, which were highest in the S. patens rhizosphere (Fig. S4), have been shown to increase methane yield (Girkin et al., 2018). These variations in the composition of the root exudate cocktail may affect biogeochemical conditions in the rhizosphere, favoring methane production in S. patens and decreasing methane production in S. americanus on longer timescales than we assessed in our incubation experiment.
ROL rates in S. americanus are comparable to results from other plants using this method, such as Spartina anglica, which Koop-Jakobsen and Wenzhöfer (2015) found to have a ROL rate of 249–300 nmol m−2 s−1, slightly lower than our measured rate in S. americanus (357 nmol m−2 s−1) and higher than our measured rate in S. patens (>154 nmol m−2 s−1). Colmer (2003) found similar rates of ROL to our values for S. patens at root apices of O. sativa (175 nmol m−2 s−1) and similar to S. americanus in P. australis (390 nmol m−2 s−1), Hordeum marinum (273 nmol m−2 s−1), and Phalaris aquatica (300 nmol m−2 s−1); all of these wetland species showed a “tight” or “partial” barrier to ROL, similar to what we saw in S. americanus using methylene blue (Colmer, 2003). Conversely, S. patens showed less of a barrier to ROL on many roots in the methylene blue images and had lower ROL in optode images as well. In most S. patens plants, we saw no buildup of O2 in the rhizosphere detectable on planar optodes, similar to some previous studies (Waldo et al., 2019). Lai et al. (2012) found significant variation in ROL by species in an assessment of 35 wetland plants, with the highest rates in Caldesia reniformis (almost 800 nmol m−2 s−1), greater than but similar to our highest measurements from S. americanus. These high rates out of S. americanus suggest a major role in oxidation of the rhizosphere and surrounding anoxic soil and a significant impact on soil biogeochemistry in regions dominated by this species. However, the lack of ROL seen in mature, darker-colored roots during methylene blue analysis makes scaling up O2 input to the soil difficult using traditional belowground biomass metrics. Further quantification of ROL from these plant species using more detailed and quantitative methods such as root-sleeving electrodes is a key avenue for future research on this topic.
Using previous estimates of photosynthetic rates in S. americanus and S. patens (DeJong et al., 1982), we can roughly estimate that the rate of O2 released to the rhizosphere represents on average 55 %–25 % of the total maximum fixed O2 for these plants. This high fraction is unlikely to be derived from photosynthesis alone and thus implies that most of the rhizosphere O2 is derived from gas transport from the atmosphere, as others have found previously (Colmer, 2003). The increased rate of ROL we see in the daytime likely represents the influence of light and temperature on stomatal conductance, though there may be some contribution of photosynthetically derived O2 as well (Lai et al., 2012). Jiang et al. (2020) found significantly greater ROL among wetland plants in the summer during active plant growth than other seasons, though the magnitude of the difference depended on species; similarly, we see greater ROL in the warmer day period. Extended growing seasons and warmer air temperature throughout the year will likely increase ROL – but also increase root exudation, as Zhai et al. (2013) found.
4.3 Implications for global change
As global change alters plant communities along coasts and elsewhere, there may be unexpected biogeochemical consequences due to species-level plant traits governing ROL and root exudation, including alterations in methanogenesis and methane oxidation, which has further implications for global change. Based on the results of this study and field data from a long-term experiment (Noyce and Megonigal, 2021), it is evidenced that S. americanus has a net oxidizing effect on soil, while S. patens has a net reducing effect. With sea level rise continuing to result in plant community transition at Kirkpatrick Marsh and other locations in the mesohaline Chesapeake, encroachment of S. americanus S. patens areas may reduce methane production due to reduced root exudation and increased sediment oxygenation. The combination of root exudation rate (higher in S. patens) and ROL (higher in S. americanus) at the species level likely has significant consequences for methane emissions, as shown in the field (Noyce and Megonigal, 2021; Mueller et al., 2020), which may be exacerbated by increased temperature, as both ROL (Jiang et al., 2020) and root exudation (Zhai et al., 2013) increase with temperature. At the Global Change Research Wetland (GCReW) on Kirkpatrick Marsh, increased temperature has exacerbated the differences in methane emissions between S. americanus- and S. patens-dominated areas (Noyce and Megonigal, 2021), possibly due to the effect of temperature on rhizosphere processes (Uselman et al., 2000; Zhai et al., 2013). Additionally, elevated CO2 has been shown to increase root exudation in wetland plants (Sánchez-Carrillo et al., 2018) and increase ROL in rice plants (Li et al., 2024), although which process dominates, and has the greatest impact on soil methane production, has not been studied. The effects of temperature and elevated CO2 combined suggest that differences in methane emissions occurring as a result of rhizosphere processes may continue to diverge at a species level as global change progresses, though future research is needed to understand the interplay between these two global change drivers, along with sea level rise.
Methane emissions are also just one component of ecosystem–climate feedbacks; understanding the net effect of global change drivers requires consideration of potential shifts in carbon sequestration rates to fully understand the radiative forcing implications. Though both high rates of rhizodeposits and high rates of ROL can prime decomposition, in anaerobic wetland soils soil organic carbon preservation is likely promoted when the dominant plants have high rates of substrate input (such as S. patens). In this situation, energetically favorable terminal electron acceptors are expected to be quickly depleted and the remaining carbon compounds preserved, essentially protecting the soil organic matter (SOM) from microbial decomposition (Kuzyakov, 2002). In contrast, high ROL (such as occurs from S. americanus) likely favors higher rates of aerobic decomposition, which is expected to reduce soil accretion and net carbon sequestration (Noyce et al., 2023).
Understanding plant responses to global change is critical for predicting future climate feedbacks. Here, we have demonstrated two methods that can be used to assess and quantify root-associated metabolites and ROL and link plant traits to observed biogeochemical patterns. Further research using these methods can now be undertaken to quantify how production of root-associated metabolites and ROL will respond to combinations of elevated CO2, sea level rise, and other consequences of global change.
All R code used in the analysis of this work is available on the Smithsonian Institution data website at https://serc.si.edu/environmental-data and https://serc.si.edu/gcrew/data, last access: 11 August 2024.
Raw mzML files of all detected root-associated metabolites in the four plants are freely available and hosted in the MassIVE database managed by UC San Diego's Center for Computational Mass Spectrometry (MassIVE ID MSV000094652, https://doi.org/10.25345/C55D8NR99, Haviland, 2024). Incubation data (headspace methane) over time were assessed via linear model with treatment (S. americanus, S. patens, or control) as a block factor and with Tukey's t test comparing the three treatments at each time point. Differences in ROL per plant 60 species were assessed by t test, after checking for normality. Additional datasets are available on the Smithsonian Environmental Research Center data-hosting website athttps://serc.si.edu/environmental-data and https://serc.si.edu/gcrew/data, last access: 11 August 2024.
The supplement related to this article is available online at: https://doi.org/10.5194/bg-21-5185-2024-supplement.
GLN and KAH both contributed to the conceptualization, formal analysis, acquisition of funds, investigation, method development, project administration, manuscript revision and proofreading, and validation of the work. KAH contributed to the original creation of the manuscript and project software needs, including analysis and data and code curation.
The contact author has declared that neither of the authors has any competing interests.
Publisher’s note: Copernicus Publications remains neutral with regard to jurisdictional claims made in the text, published maps, institutional affiliations, or any other geographical representation in this paper. While Copernicus Publications makes every effort to include appropriate place names, the final responsibility lies with the authors.
We are very grateful for the contributions of many people at the Smithsonian Environmental Research Center who helped make this work happen, especially Taylor Smith, Alia Al-Haj, Chelsea Nitsch, Pat Megonigal, Roy Rich, Selina Cheng, Jamie Pullen, Drew Peresta, Gary Peresta, Carey Pelc, Stephanie Wilson, and Zoe Read. Additionally, we owe thanks to Cynthia Tope-Niedermaier at UMBC who carried out our metabolomics sample processing and annotation. We are also grateful to Delmarva Native Plants for donating plants to this work for use in methods' development and for donating plugs of S. alterniflora for use in the root exudation sampling and analyses. We also thank two anonymous reviewers for their helpful feedback on this paper.
This research has been supported by the Biological and Environmental Research (grant nos. DE-SC0014413, DE-SC0019110, and DE-SC0021112); the Smithsonian Institution, Office of Fellowships, Smithsonian Institution (Postdoctoral Fellowship Program); and the Smithsonian Institution, Smithsonian Environmental Research Center (Life on a Sustainable Planet Program).
This paper was edited by Sara Vicca and reviewed by two anonymous referees.
Armstrong, J. and Armstrong, W.: Phragmites australis – A preliminary study of soil-oxidizing sites and internal gas transport pathways, New Phytol., 108, 373–382, https://doi.org/10.1111/j.1469-8137.1988.tb04177.x, 1988.
Armstrong, J. and Armstrong, W.: Rice: Sulfide-induced Barriers to Root Radial Oxygen Loss, Fe2+ and Water Uptake, and Lateral Root Emergence, Ann. Bot.-London, 96, 625–638, https://doi.org/10.1093/aob/mci215, 2005.
Armstrong, W. and Drew, M. C.: Root growth and metabolism under oxygen deficiency. in: Plant roots, CRC Press, 1139–1187, https://doi.org/10.1201/9780203909423.ch42, 2002.
Aulakh, M. S., Wassmann, R., Bueno, C., Kreuzwieser, J., and Rennenberg, H.: Characterization of Root Exudates at Different Growth Stages of Ten Rice (Oryza sativa L.) Cultivars, Plant. Biol., 3, 139–148, https://doi.org/10.1055/s-2001-12905, 2001.
Bethke, C. M., Sanford, R. A., Kirk, M. F., Jin, Q., and Flynn, T. M.: The thermodynamic ladder in geomicrobiology, Am. J. Sci., 311, 183 pp., https://doi.org/10.2475/03.2011.01, 2011.
Chen, Z.-J., Tian, Y.-H., Zhang, Y., Song, B.-R., Li, H.-C., and Chen, Z.-H.: Effects of root organic exudates on rhizosphere microbes and nutrient removal in the constructed wetlands, Ecol. Eng., 92, 243–250, https://doi.org/10.1016/j.ecoleng.2016.04.001, 2016.
Cheng, H., Liu, Y., Jiang, Z.-Y., and Wang, Y.-S.: Radial oxygen loss is correlated with nitrogen nutrition in mangroves, Tree Physiol., 40, 1548–1560, https://doi.org/10.1093/treephys/tpaa089, 2020.
Colmer, T. D.: Long-distance transport of gases in plants: a perspective on internal aeration and radial oxygen loss from roots, Plant Cell Environ., 26, 17–36, https://doi.org/10.1046/j.1365-3040.2003.00846.x, 2003.
Craft, C. B.: Biology of wetland soils, Wetland soils: genesis, hydrology, landscapes, and classification, 107–137, 2001.
DeJong, T. M., Drake, B. G., and Pearcy, R. W.: Gas exchange responses of Chesapeake Bay tidal marsh species under field and laboratory conditions, Oecologia, 52, 5–11, 1982.
de la Cruz Jiménez, J., Pellegrini, E., Pedersen, O., and Nakazono, M.: Radial Oxygen Loss from Plant Roots–Methods, Plants, 10, 2322, https://doi.org/10.3390/plants10112322, 2021.
Frederiksen, M. S. and Glud, R. N.: Oxygen dynamics in the rhizosphere of Zostera marina: A two-dimensional planar optode study, Limnol. Oceanogr., 51, 1072–1083, https://doi.org/10.4319/lo.2006.51.2.1072, 2006.
Girkin, N. T., Turner, B. L, Ostle, N., and Sjögersten, S.: Composition and concentration of root exudate analogues regulate greenhouse gas fluxes from tropical peat, Soil Biol.Biochem., 127, 280–285, https://doi.org/10.1016/j.soilbio.2018.09.033, 2018.
Gunina, A. and Kuzyakov, Y.: From energy to (soil organic) matter, Glob. Change Biol., 28, 2169–2182, https://doi.org/10.1111/gcb.16071, 2022.
Haney, C. H., Samuel, B. S., Bush, J., and Ausubel, F. M.: Associations with rhizosphere bacteria can confer an adaptive advantage to plants, Nature Plants, 1, 1–9, https://doi.org/10.1038/nplants.2015.51, 2015.
Hanson, R. B.: Nitrogen Fixation (Acetylene Reduction) in a Salt Marsh Amended with Sewage Sludge and Organic Carbon and Nitrogen Compounds, Appl. Environ. Microb., 33, 846–852, https://doi.org/10.1128/aem.33.4.846-852.1977, 1977.
Haviland, K.: Rhizosphere-associated metabolites of four wetland plant species, CCMS [data set], https://doi.org/10.25345/C55D8NR99, 2024.
Hemminga, M. A., Kok, C. J., and de Munck, W.: Decomposition of Spartina anglica roots and rhizomes in a salt marsh of the Westerschelde Estuary, Mar. Ecol. Prog. Ser., 48, 175–184, 1988.
Hester, M. W., Mendelssohn, I. A., and McKee, K. L.: Species and population variation to salinity stress in Panicum hemitomon, Spartina patens, and Spartina alterniflora: morphological and physiological constraints, Environ. Exp. Bot., 46, 277–297, https://doi.org/10.1016/S0098-8472(01)00100-9, 2001.
Hijmans R.: terra: Spatial Data Analysis, R package version 1.7-65, https://CRAN.R-project.org/package=terra (last access: 5 March 2024), 2023.
Hines, M. E., Evans, R. S., Sharak Genthner, B. R., Willis, S. G., Friedman, S., Rooney-Varga, J. N., and Devereux, R.: Molecular Phylogenetic and Biogeochemical Studies of Sulfate-Reducing Bacteria in the Rhizosphere of Spartina alterniflora, Appl. Environ. Microb., 65, 2209–2216, https://doi.org/10.1128/AEM.65.5.2209-2216.1999, 1999.
Hinsinger, P., Plassard, C., and Jaillard, B.: Rhizosphere: A new frontier for soil biogeochemistry, J. Geochem. Explor., 88, 210–213, https://doi.org/10.1016/j.gexplo.2005.08.041, 2006.
Jensen, S. I., Kühl, M., Glud, R. N., Jørgensen, L. B., and Priemé, A.: Oxic microzones and radial oxygen loss from roots of Zostera marina, Mar. Ecol. Prog. Ser., 293, 49–58, hhtps://https://doi.org/10.3354/meps293049, 2005.
Jiang, X., Tian, Y., Ji, X., Lu, C., and Zhang, J.: Influences of plant species and radial oxygen loss on nitrous oxide fluxes in constructed wetlands, Ecol. Eng., 142, 105644, https://doi.org/10.1016/j.ecoleng.2019.105644, 2020.
Kassambara, A: rstatix: Pipe-Friendly Framework for Basic Statistical Tests, R package version 0.7.2 [code], https://CRAN.R-project.org/package=rstatix (last access: 5 March 2024), 2023.
Kassambara, A. and Mundt, F.: factoextra: Extract and Visualize the Results of Multivariate Data Analyses, R package version 1.0.7 [code], https://CRAN.R-project.org/package=factoextra (last access: 5 March 2024), 2020.
Koop-Jakobsen, K. and Wenzhöfer, F.: The Dynamics of Plant-Mediated Sediment Oxygenation in Spartina anglica Rhizospheres–a Planar Optode Study, Estuar. Coast., 38, 951–963, https://doi.org/10.1007/s12237-014-9861-y, 2015.
Koop-Jakobsen, K., Mueller, P., Meier, R. J., Liebsch, G., and Jensen, K.: Plant-Sediment Interactions in Salt Marshes – An Optode Imaging Study of O2, pH, and CO2 Gradients in the Rhizosphere, Front. Plant Sci., 9, 541, https://doi.org/10.3389/fpls.2018.00541, 2018.
Koop-Jakobsen, K., Meier, R. J., and Mueller, P.: Plant-Mediated Rhizosphere Oxygenation in the Native Invasive Salt Marsh Grass Elymus athericus, Front. Plant Sci., 12, 669751, https://doi.org/10.3389/fpls.2021.669751, 2021.
Kumar, R., Pandey, S., and Pandey, A.: Plant roots and carbon sequestration, Curr. Sci. India, 91, 885–890, http://www.jstor.org/stable/24094284, 2006.
Kuzyakov, Y.: Factors affecting rhizosphere priming effects, J. Plant Nutr. Soil Sc., 165, 382–396, 2002.
Lai, W.-L., Zhang, Y., and Chen, Z.-H.: Radial oxygen loss, photosynthesis, and nutrient removal of 35 wetland plants, Ecol. Eng., 39, 24–30, https://doi.org/10.1016/j.ecoleng.2011.11.010, 2012.
Langley, J. A., McKee, K. L., Cahoon, D. R., Cherry, J. A., and Megonigal, J. P.: Elevated CO2 stimulates marsh elevation gain, counterbalancing sea-level rise, P. Natl. Acad. Sci. USA, 106, 6182–6186, 2009.
Lei, X., Shen, Y., Zhao, J., Huang, J., Wang, H., Yu, Y., and Xiao, C.: Root exudates mediate the processes of soil organic carbon input and efflux, Plants, 12, 630, https://doi.org/10.3390/plants12030630, 2023.
Li, J., Zhang, H., Xie, W., Liu, C., Liu, X., Zhang, X., Li, L., and Pan, G.: Elevated CO2 increases soil redox potential by promoting root radial oxygen loss in paddy field, J. Environ. Sci., 136, 11–20, https://doi.org/10.1016/j.jes.2023.01.003, 2024.
Li, Y., Yuan, L., Xue, S., Liu, B., and Jin, G.: Artificial root exudates excite bacterial nitrogen fixation in the subsurface of mine soils, Appl. Soil Ecol., 157, 103774, https://doi.org/10.1016/j.apsoil.2020.103774, 2021.
Liu, H., Chen, Y., Ye, J., Xu, H., Zhu, Z., and Xu, T.: Effects of different amino acids and their configurations on methane yield and biotransformation of intermediate metabolites during anaerobic digestion, J. Environ. Manage., 296, 113152, https://doi.org/10.1016/j.jenvman.2021.113152, 2021.
Lolu, A. J., Ahluwalia, A. S., Sidhu, M. C., Reshi, Z. A., and Mandotra, S. K.: Carbon Sequestration and Storage by Wetlands: Implications in the Climate Change Scenario, in: Restoration of Wetland Ecosystem: A Trajectory Towards a Sustainable Environment, edited by: Upadhyay, A. K., Singh, R., and Singh, D. P., Springer, Singapore, 45–58, https://doi.org/10.1007/978-981-13-7665-8_4, 2020.
Martin, B. C., Bougoure, J., Ryan, M. H., Bennett, W. W., Colmer, T. D., Joyce, N. K., Olsen, Y. S., and Kendrick, G. A.: Oxygen loss from seagrass roots coincides with colonisation of sulphide-oxidising cable bacteria and reduces sulphide stress, ISME J., 13, 707–719, https://doi.org/10.1038/s41396-018-0308-5, 2019.
Maurer, D., Malique, F., Alfarraj, S., Albasher, G., Horn, M. A., Butterbach-Bahl, K., Dannenmann, M., and Rennenberg, H.: Interactive regulation of root exudation and rhizosphere denitrification by plant metabolite content and soil properties, Plant Soil, 467, 107–127, https://doi.org/10.1007/s11104-021-05069-7, 2021.
McLaughlin, S., Zhalnina, K., Kosina, S., Northen, T. R., and Sasse, J.: The core metabolome and root exudation dynamics of three phylogenetically distinct plant species, Nat. Commun., 14, 1649, 2023.
Mcleod, E., Chmura, G. L., Bouillon, S., Salm, R., Björk, M., Duarte, C. M., Lovelock, C. E., Schlesinger, W. H., and Silliman, B. R.: A blueprint for blue carbon: toward an improved understanding of the role of vegetated coastal habitats in sequestering CO2, Front. Ecol. Environ., 9, 552–560, https://doi.org/10.1890/110004, 2011.
Megonigal, J. P., Whalen, S. C., Tissue, D. T., Bovard, B. D., Allen, A. S., and Albert, D. B.: A Plant-Soil-Atmosphere Microcosm for Tracing Radiocarbon from Photosynthesis through Methanogenesis, Soil Sci. Soc. Am. J., 63, 665–671, https://doi.org/10.2136/sssaj1999.03615995006300030033x, 1999.
Mitsch, W. J., Bernal, B., Nahlik, A. M., Mander, Ü., Zhang, L., Anderson, C. J., Jørgensen, S. E., and Brix, H.: Wetlands, carbon, and climate change, Landscape Ecol., 28, 583–597, https://doi.org/10.1007/s10980-012-9758-8, 2013.
Mueller, P., Mozdzer, T. J., Langley, J. A., Aoki, L. R., Noyce, G. L., and Megonigal, J. P.: Plant species determine tidal wetland methane response to sea level rise, Nat. Commun., 11, 5154, https://doi.org/10.1038/s41467-020-18763-4, 2020.
Nardi, S., Reniero, F., and Concheri, G.: Soil organic matter mobilization by root exudates of three maize hybrids, Chemosphere, 35, 2237–2244, 1997.
Neumann, G.: Root exudates and organic composition of plant roots, Handbook of methods used in rhizosphere research, Swiss Federal Institute for Forest, Snow, and Landscape Research, https://doi.org/10.3389/fpls.2019.00157, 2007.
Nichols, J. E. and Peteet, D. M.: Rapid expansion of northern peatlands and doubled estimate of carbon storage, Nat. Geosci., 12, 917–921, https://doi.org/10.1038/s41561-019-0454-z, 2019.
Noyce, G. L. and Megonigal, J. P.: Biogeochemical and plant trait mechanisms drive enhanced methane emissions in response to whole-ecosystem warming, Biogeosciences, 18, 2449–2463, https://doi.org/10.5194/bg-18-2449-2021, 2021.
Noyce, G. L., Smith, A. J., Kirwan, M. L., Rich, R. L., and Megonigal, J. P.: Oxygen priming induced by elevated CO2 reduces carbon accumulation and methane emissions in coastal wetlands, Nat. Geosci., 16, 63–68, https://doi.org/10.1038/s41561-022-01070-6, 2023.
Oburger, E. and Jones, D. L.: Sampling root exudates – Mission impossible?, Rhizosphere, 6, 116–133, https://doi.org/10.1016/j.rhisph.2018.06.004, 2018.
Oburger, E., Staudinger, C., Spiridon, A., Benyr, V., Aleksza, D., Wenzel, W., and Santangeli, M.: A quick and simple spectrophotometric method to determine total carbon concentrations in root exudate samples of grass species, Plant Soil, 478, 273–281, https://doi.org/10.1007/s11104-022-05519-w, 2022.
Pantigoso, H. A., Yuan, J., He, Y., Guo, Q., Vollmer, C., and Vivanco, J. M.: Role of root exudates on assimilation of phosphorus in young and old Arabidopsis thaliana plants, PLOS ONE, 15, e0234216, https://doi.org/10.1371/journal.pone.0234216, 2020.
Philippot, L., Hallin, S., Börjesson, G., and Baggs, E. M.: Biochemical cycling in the rhizosphere having an impact on global change. Plant Soil, 321, 61–81, https://doi.org/10.1007/s11104-008-9796-9, 2009.
Robroek, B. J. M., Albrecht, R. J. H., Hamard, S., Pulgarin, A., Bragazza, L., Buttler, A., and Jassey, V. E.: Peatland vascular plant functional types affect dissolved organic matter chemistry, Plant Soil, 407, 135–143, https://doi.org/10.1007/s11104-015-2710-3, 2016.
Sánchez-Carrillo, S., Álvarez-Cobelas, M., Angeler, D. G., Serrano-Grijalva, L., Sánchez-Andrés, R., Cirujano, S., and Schmid, T.: Elevated Atmospheric CO2 Increases Root Exudation of Carbon in Wetlands: Results from the First Free-Air CO2 Enrichment Facility (FACE) in a Marshland, Ecosystems, 21, 852–867, https://doi.org/10.1007/s10021-017-0189-x, 2018.
Sciance, M. B., Patrick, C. J., Weller, D. E., Williams, M. N., McCormick, M. K., and Hazelton, E. L. G.: Local and regional disturbances associated with the invasion of Chesapeake Bay marshes by the common reed Phragmites australis, Biol. Invasions, 18, 2661–2677, https://doi.org/10.1007/s10530-016-1136-z, 2016.
Shi, H., Wu, X., Zhu, Y., Jiang, T., Wang, Z., Li, X., Liu, J., Zhang, Y., Chen, F., Gao, J., and Xu, X.: RefMetaPlant: a reference metabolome database for plants across five major phyla, Nucleic Acids Res., 52, D1614–D1628, https://doi.org/10.1093/nar/gkad980, 2024.
Uselman, S. M., Qualls, R. G., and Thomas, R. B.: Effects of increased atmospheric CO2, temperature, and soil N availability on root exudation of dissolved organic carbon by a N-fixing tree (Robinia pseudoacacia L.), Plant soil, 222, 191–202, https://doi.org/10.1023/A:1004705416108, 2000.
Valach, A. C., Kasak, K., Hemes, K. S., Anthony, T. L., Dronova, I., Taddeo, S., Silver, W. L., Szutu, D., Verfaillie, J., and Baldocchi, D. D.: Productive wetlands restored for carbon sequestration quickly become net CO2 sinks with site-level factors driving uptake variability, PLOS ONE, 16, e0248398, https://doi.org/10.1371/journal.pone.0248398, 2021.
Visser, E. J. W., Colmer, T. D., Blom, C. W. P. M., and Voesenek, L. A. C. J.: Changes in growth, porosity, and radial oxygen loss from adventitious roots of selected mono- and dicotyledonous wetland species with contrasting types of aerenchyma, Plant Cell Environ., 23, 1237–1245, https://doi.org/10.1046/j.1365-3040.2000.00628.x, 2000.
Vranova, V., Rejsek, K., Skene, K. R., Janous, D., and Formanek, P.: Methods of collection of plant root exudates in relation to plant metabolism and purpose: A review, J. Plant Nutr. Soil Sci., 176, 175–199, https://doi.org/10.1002/jpln.201000360, 2013.
Waldo, N. B., Hunt, B. K., Fadely, E. C., Moran, J. J., and Neumann, R. B.: Plant root exudates increase methane emissions through direct and indirect pathways, Biogeochemistry, 145, 213–234, https://doi.org/10.1007/s10533-019-00600-6, 2019.
Wang, Q., Chen, L., Xu, H., Ren, K., Xu, Z., Tang, Y., and Xiao, J.: The effects of warming on root exudation and associated soil N transformation depend on soil nutrient availability, Rhizosphere, 17, 100263, https://doi.org/10.1016/j.rhisph.2020.100263, 2021.
Wu, F. Y., Chung, A. K. C., Tam, N. F. Y., and Wong, M. H.: Root Exudates of Wetland Plants Influenced by Nutrient Status and Types of Plant Cultivation, Int. J. Phytoremediat., 14, 543–553, https://doi.org/10.1080/15226514.2011.604691, 2012.
Xiong, L., Liu, X., Vinci, G., Spaccini, R., Drosos, M., Li, L., Piccolo, A., and Pan, G.: Molecular changes of soil organic matter induced by root exudates in a rice paddy under CO2 enrichment and warming of canopy air, Soil Biol. Biochem., 137, 107544, https://doi.org/10.1016/j.soilbio.2019.107544, 2019.
Zhai, X., Piwpuan, N., Arias, C. A., Headley, T., and Brix, H.: Can root exudates from emergent wetland plants fuel denitrification in subsurface flow constructed wetland systems?, Ecol. Eng., 61, 555–563, https://doi.org/10.1016/j.ecoleng.2013.02.014, 2013.