the Creative Commons Attribution 4.0 License.
the Creative Commons Attribution 4.0 License.
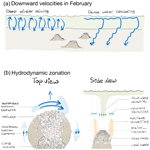
Building your own mountain: the effects, limits, and drawbacks of cold-water coral ecosystem engineering
Anna-Selma van der Kaaden
Sandra R. Maier
Siluo Chen
Laurence H. De Clippele
Evert de Froe
Theo Gerkema
Johan van de Koppel
Furu Mienis
Christian Mohn
Max Rietkerk
Karline Soetaert
Dick van Oevelen
Framework-forming cold-water corals (CWCs) are ecosystem engineers that build mounds in the deep sea that can be up to several hundred metres high. The effect of the presence of cold-water coral mounds on their surroundings is typically difficult to separate from environmental factors that are not affected by the mounds. We investigated the environmental control on and the importance of ecosystem engineering for cold-water coral reefs using annotated video transect data, spatial variables (MEMs), and hydrodynamic model outputs in a redundancy analysis and with variance partitioning. Using available hydrodynamic simulations with cold-water coral mounds and simulations where the mounds were artificially removed, we investigated the effect of coral mound ecosystem engineering on the spatial configuration of reef habitat and discriminated which environmental factors are and which are not affected by the mounds.
We find that downward velocities in winter, related to non-engineered environmental factors, e.g. deep winter mixing and dense-water cascading, cause substantial differences in reef cover at the broadest spatial scale (20–30 km). Such hydrodynamic processes that stimulate the food supply towards the corals in winter seem more important for the reefs than cold-water coral mound engineering or similar hydrodynamic processes in summer. While the ecosystem-engineering effect of cold-water corals is frequently discussed, our results also highlight the importance of non-engineered environmental processes.
We further find that, due to the interaction between the coral mound and the water flow, different hydrodynamic zones are found on coral mounds that likely determine the typical benthic zonations of coral rubble at the mound foot, the dead coral framework on the mound flanks, and the living corals near the summit. Moreover, we suggest that a so-called Massenerhebung effect (well known for terrestrial mountains) exists, meaning that benthic zonation depends on the location of the mound rather than on the height above the seafloor or water depth. Our finding that ecosystem engineering determines the configuration of benthic habitats on cold-water coral mounds implies that cold-water corals cannot grow at deeper depths on the mounds to avoid the adverse effects of climate change.
- Article
(6914 KB) - Full-text XML
- BibTeX
- EndNote
Framework-forming cold-water corals are benthic suspension feeders that rely on particulate organic matter originating from the sunlit ocean surface (van Oevelen et al., 2018; Van Engeland et al., 2019; Carlier et al., 2009). They build habitats of live and dead reef frameworks for many species and have a high biodiversity and productivity as compared to the surrounding deep-sea environment (van Oevelen et al., 2009; Cathalot et al., 2015; Bongiorni et al., 2010). Many of the associated reef fauna are also suspension feeders that require similar food advection by currents as the framework-forming corals themselves (Maier et al., 2021). With their structurally complex framework, cold-water corals engineer their environment, increasing the food supply towards themselves and their associated fauna (van der Kaaden et al., 2020; Hennige et al., 2021; Bartzke et al., 2021; Mienis et al., 2019). These important deep-sea ecosystems are expected to be severely impacted by ocean warming and acidification (Hennige et al., 2020; Morato et al., 2020; Ragnarsson et al., 2016; Sweetman et al., 2017), and understanding what drives cold-water coral reef growth at different spatial scales provides insights into their expected response to contemporary global changes.
When the positive feedbacks of an organism on its own growth are strong enough, the ecosystem engineer can locally create its own optimal environment and exist in suboptimal ambient environmental conditions where it would not survive otherwise (Jones et al., 1994; Crooks, 2002; Hastings et al., 2007). Recently, reef-forming cold-water corals were identified as a self-organized system, meaning that the corals enhance their resource intake by optimizing their spatial configuration on the seafloor (van der Kaaden et al., 2023). By adapting their spatial configuration in the ecosystem, ecosystem engineers can respond gradually to environmental changes, providing resilience in the face of relatively rapid global change (Bastiaansen et al., 2020; Rietkerk et al., 2021).
Framework-forming cold-water corals also engineer their environment at much larger spatial scales because the reefs can develop into mounds, where coral growth and sediment infill on the reefs is higher than in the off-reef area (Wang et al., 2021; van der Land et al., 2014). These mounds can be hundreds of metres high, enabling corals to feed higher in the water column, where particulate organic matter quality and quantity is higher (Snelgrove et al., 2017; Nakatsuka et al., 1997). Moreover, the interaction of mounds with (tidal) currents also increases the food supply towards the coral reefs (van der Kaaden et al., 2021). Internal (tidal) waves created around the mounds (van Haren et al., 2014; Cyr et al., 2016; Davies et al., 2009) increase the horizontal and vertical food flux towards the reefs (Duineveld et al., 2012; Froe et al., 2022; Soetaert et al., 2016).
Soetaert et al. (2016) simulated the flow of particulate organic matter to cold-water coral reefs in the Logachev cold-water coral mound province with a coupled 3D model. They found that the flux of particulate organic matter is concentrated towards the corals living on the mounds due to the physical presence of the mounds. Recent field observations indeed indicate the existence of this food transport pathway (de Froe et al., 2022). van der Kaaden et al. (2021) further investigated the interaction of coral mounds and hydrodynamics for various mound sizes. For this, the bathymetry was modified to mimic different mound sizes and was used in 3D hydrodynamic simulations. They found that, like coral reefs, coral mounds exert several spatial feedbacks through ecosystem engineering of bottom currents and vertical velocities. Thus, the ecosystem engineering effect of framework-forming cold-water corals is well established at both the scale of reefs and of coral mounds. However, how the effect of cold-water coral mounds on the hydrodynamics (i.e. “coral mound engineering”) affects the spatial configuration of cold-water coral reef habitats and their associated fauna remains unclear.
Furthermore, separating those environmental factors that are affected by coral mound engineering and those environmental factors that are not affected by cold-water corals is seemingly impossible since one cannot simply remove all coral mounds and compare measurements of the environment with and without (i.e. smoothed topography) mounds. In this study, we make use of the unique situation at the Logachev cold-water coral mound province, for which hydrodynamic model simulations with and without coral mounds are available (van der Kaaden et al., 2021). Specifically, we combined this hydrodynamic model output with annotated video transects from Maier et al. (2021) and De Clippele et al. (2021) and variables identifying spatial scale (Moran eigenvector maps) to identify the main environmental drivers of reef cover in a redundancy analysis (RDA). Variance partitioning showed how much of the spatial structure of reef cover is related to the different hydrodynamic variables, and by comparing hydrodynamic simulations with and without coral mounds, we investigated which hydrodynamic variables are caused by feedbacks between the mounds and the hydrodynamics. This combined approach allowed us to investigate the environmental control on reef cover at different spatial scales and to discriminate the effects of coral mound engineering and non-engineered environmental factors.
2.1 Site description
The Logachev cold-water coral mound province (Fig. 1) is located west of Ireland on the SE Rockall Bank margin (NE Atlantic) (de Haas et al., 2009; Kenyon et al., 2003; Mienis et al., 2006). Cold-water coral mound clusters occur between 600 and 1100 m depth, usually in the Wyville Thomson Overflow Water (WTOW) current that flows in a SW direction (Schulz et al., 2020; Johnson et al., 2010). The mounds are situated at the location of increased internal-tide generation as compared to the rest of the SE Rockall Bank margin (van der Kaaden et al., 2021). Mound summits are situated around the depth of the permanent pycnocline (White and Dorschel, 2010). A bottom-trapped internal tidal wave at diurnal frequency travels past the Rockall Bank margin, causing internal waves with amplitudes of over 120 m near the mounds (van Haren et al., 2014).
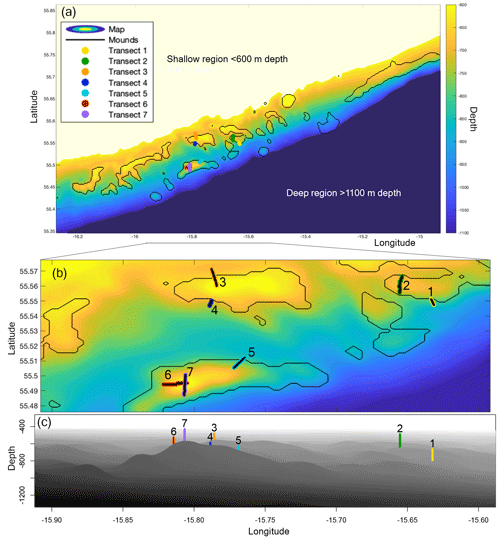
Figure 1(a) Map of the Logachev cold-water coral mound province, as used in the hydrodynamic simulations, depicting the depth (m) in colour. The mounds, as selected by connected-component labelling, are encircled by black lines. The seven transects are plotted in colour. (b) A zoom-in on the Logachev cold-water coral mound province on the seven transects. The transects are numbered and highlighted in black for clarity. (c) A side view of the zoom-in on Logachev coral mound province, with the centre of the transects indicated by the coloured line. Bathymetric data are derived from the Irish National Seabed Survey (INSS) at 250 m resolution.
2.2 Response and explanatory variables
Redundancy analysis (RDA) identifies the main trends in response variables (i.e. benthic cover) along continuous axes and how these trends correlate to explanatory variables (i.e. depth, hydrodynamic, and spatial variables) (Borcard et al., 2011). Below, we first describe how response and explanatory variables were obtained from the available data (Table 1), and then we detail the RDA approach.
2.2.1 Video frame extraction and annotation (response variables)
Video transects 1 to 6 were recorded with the remotely operated vehicle (ROV) Holland-1 between 600 and 850 m depth during the Changing Oceans Expedition 2012 Cruise 073 with the RRS James Cook. The ROV was equipped with a Reson 7125 multibeam system and a downward-facing HD camera for continuous recording and two lasers for size reference (Roberts and shipboard party, 2013). From these videos, 1196 frames were manually annotated by De Clippele et al. (2021) for live reef-forming corals, dead coral frameworks, coral rubble, rocks, and sediment, following the annotation protocol by van der Kaaden and De Clippele (2021).
Video transect 7 was recorded during the RV Pelagia cruise 64PE360 in October 2012 between 600 and 700 m depth with a towed camera frame from the NIOZ (Royal Netherlands Institute for Sea Research) and two lasers for size reference. A total of 187 frames were manually annotated by Maier et al. (2021) for live reef-forming corals, less-degraded dead frameworks, more-degraded dead frameworks, coral rubble, rocks, sediment, sponges, and other macrofauna, following the same annotation protocol (van der Kaaden and De Clippele, 2021). We combined the two datasets for our analysis and used the benthic cover (percentage of the annotated frames) of live coral, dead coral, rubble, rocks, and sediment as response variables in the RDA.
2.2.2 Bathymetry and hydrodynamic variables (explanatory variables)
Bathymetric and hydrodynamic variables were obtained from recently published 3D hydrostatic Regional Ocean Modelling System (ROMS) simulations for the study area (van der Kaaden et al., 2021). The model uses 3D free-surface, hydrostatic equations with grid refinement on a staggered Arakawa C-grid in the horizontal and topography-following coordinates in the vertical (Shchepetkin and McWilliams, 2005). The model covered an area of 85 km × 58 km with a horizontal resolution of 250 m, nested into a model grid of 190 km × 188 km with a resolution of 750 m. In the vertical, the model contained 32 levels with enhanced resolution near the surface and bottom. Tidal forcing (TPXO7) and realistic water (WOA) and atmospheric (COADS) forcings were applied at the boundaries of the lower-resolution model domain. The model was run with climatological forcing, and model output was obtained every 6 h for two runs of 30 d in February and August. A detailed description of the model and selected variables is given in van der Kaaden et al. (2021) and (2022).
We included 30 d averages of the following hydrodynamic variables because they are thought to be related to the coral food supply: horizontal current speed at the bottom layer of the model (“Speed”), upward and downward velocities between 250–300 m depth (“Wup” and “Wdown”), and depth-integrated energy conversion rate (“EC”) to the diurnal (K1) internal tide that is trapped at the Rockall Trough margin (van der Kaaden et al., 2021). The energy conversion rate is a measure of internal tidal wave activity (Gerkema et al., 2004). February and August bottom current speeds and energy conversion rates correlated strongly (Spearman correlation coefficient rS=0.81 and rS=0.95, respectively) which is undesirable in an RDA so we used the average of the w months (i.e. 60 d average). For each annotated frame, values of all described variables were taken from the model grid cell closest to the position of the respective annotated frames (based on longitude and latitude coordinates).
2.2.3 Spatial variables from Moran's eigenvector maps (explanatory variables)
To investigate the spatial configuration of reef cover and environmental variables at multiple spatial scales, we used Moran's eigenvector maps (MEMs). In the MEM approach, the study area is divided into groups (i.e. a map with one group, two groups, three groups, etc.), and each annotated video frame is assigned a value that represents its proximity to the centre of the group in which it is located (Borcard et al., 2011). Moran's eigenvector map 1 thus represents variations on the broadest spatial scale of the entire study area (∼25 km), and the highest MEMs (i.e. when the study area is divided into the highest number of groups) describe variation at the finest spatial scales of the difference between individual annotated video frames (∼4 m). MEMs merely describe a certain spatial scale and thus do not exert any control on cold-water coral (CWC) growth; their specific values are therefore meaningless with respect to CWC growth.
Moran's eigenvector maps were created from a distance-based connectivity matrix with the adespatial package in R (Dray et al., 2022). The connectivity matrix was calculated from the transformed spherical longitude and latitude coordinates using a minimum distance of 0 and the maximum distance at which all the data points were connected. This resulted in 15 significant (Moran's adjusted p<0.05) MEMs with positive eigenvalues. At the broadest spatial scale (signalling differences between transects) data points were classified according to their east-to-west position (MEM 1) or according to their north-to-south position (MEM 2). At finer scales (signalling differences within transects) data points were classified by MEMs according to their position along a mound flank (MEMs 3, 4, and 5) or according to regular intervals along transects (MEM 6 to MEM 15). Thus, MEM 1 and MEM 2 describe the between-transect spatial scale, and higher MEMs describe the within-transect spatial scale. Data points on the same transect will thus have very similar values for MEM 1 and 2 but can have very different values for MEM 15.
2.3 RDA and variance partitioning
We explored our data following the protocol by Zuur et al. (2010) to ensure compliance with the assumptions of the RDA and variance partitioning. We applied a Hellinger transformation to the benthic cover data to minimize differences in standard deviations and applied scaling during RDA and variance partitioning to homogenize the variable variances. With two variance partitioning analyses, we calculated the percentage of variance in benthic cover that was explained by (1) hydrodynamic variables, depth, and spatial variables and (2) environmental variables (hydrodynamic variables and depth), between-transect spatial variables, and within-transect spatial variables. The latter analysis provides information on whether benthic cover varies most between transects or within transects.
For parsimonious analyses and to avoid high collinearity, we selected the explanatory variables to be used in RDA and variance partitioning using forward selection with a significance level of 0.05 and the extra stopping criterion based on the adjusted coefficient of multiple determination ( of the global model (Blanchet et al., 2008). In this way, we selected the variables in the explanatory datasets separately after verifying that the RDA with variables from the respective dataset was significant (Blanchet et al., 2008). We included depth as a separate variable in the final set of explanatory variables. Forward selection was done in R with the adespatial package (Dray et al., 2022), collinearity was tested by calculating variation inflation factors (VIFs) with the vegan package, and RDA and variance partitioning were also performed with the vegan package (Oksanen et al., 2022).
Table 1Response and explanatory variables as used in RDA and variance partitioning, with their units and range (min, mean, max). Vertical velocities are 30 d averages of February or August, and bottom current speed and energy conversion rates are 60 d averages of February + August. The range of values was calculated based on the variables as used in RDA and variance partitioning.
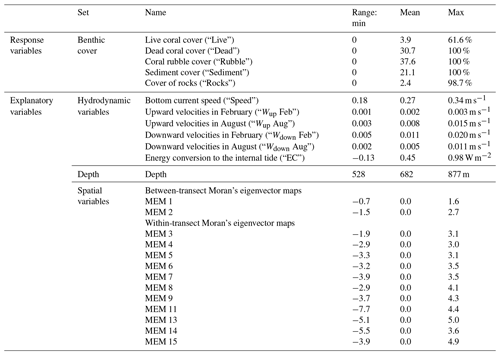
2.4 Hydrodynamic simulations with and without coral mounds (coral mound engineering effect)
Some hydrodynamic (i.e. part of the explanatory) variables included in the analyses might be affected by the presence of cold-water coral mounds (i.e. coral mound engineering effect). We try to discriminate the environmental factors that are affected by coral mound engineering and environmental factors that are not affected by cold-water corals by comparing hydrodynamic model simulations with and without cold-water coral mounds from van der Kaaden et al. (2021). van der Kaaden et al. (2021) mimicked a situation without cold-water coral mounds by smoothing the bathymetry and simulated the hydrodynamics on the smoothed and unmodified bathymetry. We calculated the coral mound engineering effect by subtracting horizontal bottom current speed, upward velocities in February, upward velocities in August, downward velocities in February, downward velocities in August, and energy conversion rates (Table 2) of the simulations with smoothed bathymetry (i.e. without coral mounds) from simulations with unmodified bathymetry (i.e. with coral mounds).
We define a coral mound engineering effect as a positive or negative feedback, meaning that the magnitude of the hydrodynamic variables increases or decreases, respectively, with increasing coral mound height. To investigate which hydrodynamic variables are influenced by the size of a coral mound, we calculated Spearman rho correlation coefficients between the mean absolute effect of mound presence and mound height. A significant correlation thus indicates a significant positive or negative effect of coral mound engineering on the hydrodynamic variable.
Mound height was defined using the model topography from van der Kaaden et al. (2021) as the maximum difference in topography at the location of the mounds, where we determined the location of mounds using connected component labelling in MATLAB (Fig. 1). Mound height is therefore the maximum topographic height corrected for the underlying continental slope.
3.1 General transect observations
There is variability in benthic cover within and between transects (Fig. 2), but clear trends are not obvious. For instance, transect 3, located on the lower southern flank of the northernmost mound, and transect 6, located at the western flank of the highest mound (Fig. 1), have a relatively low live- and dead-coral cover overall. Transect 7, across the highest mound in the area, shows a relatively high dead-coral cover and low sediment and rubble cover overall.
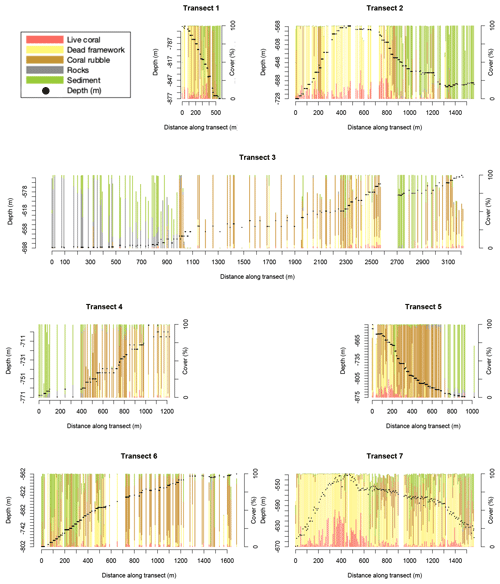
Figure 2Stacked bar plots show the benthic cover (in percent of the annotated frame) of the seven transects. Each bar represents one annotated video frame. Note: for visibility, only frames at >5.5 m distance apart are shown. Benthic cover types shown are the cover of live framework-forming corals (red), dead-coral framework (yellow), coral rubble (brown), rocks (grey), and sediment (green). Other cover types are not shown so bar plots might not reach 100 %. Black dots show the transect depth in metres (left y axis).
3.2 Redundancy analysis (RDA)
We investigated the relation between benthic cover and environmental variables with a redundancy analysis (RDA) in which we studied the spatial benthic configuration by including Moran's eigenvector maps (MEMs) in the analysis. RDA axes 1 to 4 were significant (p<0.001). The first axis of the redundancy analysis explains 27 % of the variation in benthic cover and separates frames with high reef cover (i.e. live corals and dead framework) from frames with low reef cover (i.e. rocks, sediment, and coral rubble cover; Figs. 3 and 4a). The most important variables explaining high versus low reef cover are downward velocities in February, the spatial variable between-transect MEM 2, and depth. Less important variables are bottom current speed, upward velocities in February, between-transect MEM 1, and within-transect MEM 4. The other explanatory variables have only a minor contribution on the first axis. In all, high reef cover is associated with strong downward velocities, shallower water depths, strong bottom currents, and weak upward velocities.
The second RDA axis explains 11.5 % of the variation and separates frames with high coral rubble cover from frames with low coral rubble cover (Figs. 3 and 4b). The most important variable explaining high versus low coral rubble cover is the spatial variable within-transect MEM 3. Less important variables are between-transect MEM 1, upward velocities in August, between-transect MEM 2, depth, and bottom current speed. High coral rubble cover is associated with strong upward velocities in August, greater depths, and strong bottom current speeds.
The third axis explained 6.3 % of the variation in benthic cover (Fig. 4c). The third axis separates frames with high sediment cover from frames with low sediment cover. The most important variables explaining high versus low sediment cover are between-transect MEM 1 and between-transect MEM 2. Less important variables are upward velocities in February, downward velocities in August, and within-transect MEM 5. High sediment cover is associated with weak upward velocities in February and strong downward velocities in August. We do not discuss the fourth axis as it explained <1 % of the variation in benthic cover.
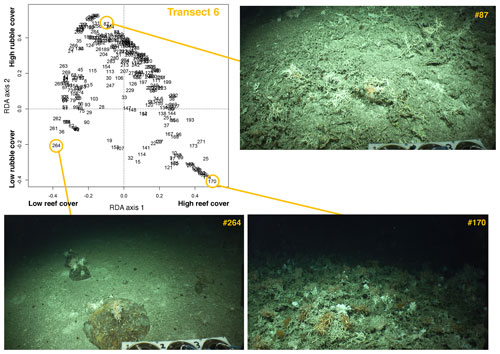
Figure 3Annotated video frames from transect 6 plotted on the first (x axis) and second (y axis) redundancy analysis (RDA) axes, with three representative images from the seafloor. The first RDA axis separates images with low reef cover (such as image no. 264) from images with high reef cover (such as image no. 170). The second RDA axis separates images with low rubble cover (such as images no. 264 and no. 170) from images with high coral rubble cover (such as image no. 87). Note: images are directly extracted from the videos without modifications or annotations. The videos were obtained during the Changing Oceans 2012 expedition with RRS James Cook; © 2012 Laurence De Clippele.
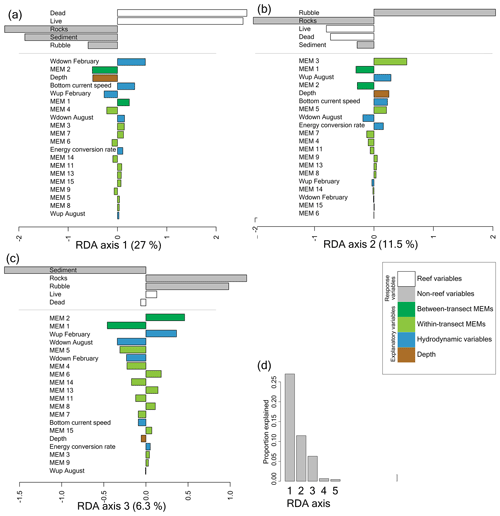
Figure 4Bar plot of the components of the first (a), second (b), and third (c) axes of the redundancy analysis (RDA), explaining 27 %, 11.5 %, and 6.3 % of the variation in benthic cover, respectively. The x axis shows the value on the RDA axis. RDA axes 1 to 4 were significant (p<0.05), but axis 4 is not shown as it explained <1 % of the variation in benthic cover (d). The benthic cover (response) variables are shown at the top (reef variables in white and non-reef variables in grey), and below that, the explanatory variables are shown in colour. Variables are ordered from most important to least important and coloured according to the variable type as shown in the legend. Response and explanatory variables on the same side of the axis are correlated.
3.3 Variance partitioning
Variance partitioning showed that 39.6 % of the variance in the benthic cover was explained by either depth (11.3 %), hydrodynamic variables (14.2 %), or spatial variables (14.1 %), and 5.5 % was explained by a combination of those variables (Fig. 5). These fractions represent the variation in benthic cover that is explained purely by a (set of) variable(s), meaning that 14.1 % of the variation in benthic cover has a spatial configuration that is not explained by depth or hydrodynamic variables. Of the spatial variables, 2.4 % of the variation in benthic cover is explained purely by between-transect MEMs 1 and 2, i.e. related to variations at the scale of the study area, and 8.8 % by all within-transect MEMs 3 to 15, i.e. related to variations on coral mounds. Since 2.4 % and 8.8 % do not add up to 14.1 %, a fraction of the variation in benthic cover (2.9 %) is explained by a combination of between- and within-transect MEMs. A total of 54.9 % of the variance in benthic cover remained unexplained by the explanatory variables used in this study.
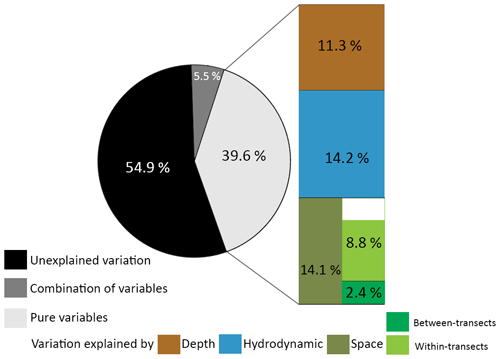
Figure 5Pie chart of the proportion of unexplained variation in the data (black), the proportion explained by a combination of sets of variables (dark grey), and the proportion explained by the explanatory variables (light grey). The explained variance is separated in the variation explained purely by depth (brown), hydrodynamic variables (blue), or spatial variables (green). Spatial variables are further separated in MEMs describing the between-transect spatial scale (dark green) and the within-transect spatial scale (light green). The sum of the variance explained purely by the between- and within-transect spatial scale did not add up to 14.1 %, possibly because the combined spatial scales also explain a fraction of the variance. All pure fractions were significant (p<0.001).
3.4 Coral mound engineering effect
The comparison of hydrodynamic model output with and without coral mounds from van der Kaaden et al. (2021) showed that the presence of cold-water coral mounds causes an acceleration or deceleration of bottom currents near coral mound foots and a deceleration on some mound summits (Fig. 6a). Energy conversion rate to the internal tide is increased at those mound flanks that face the tidal current and is decreased at the right side of mounds that face the incoming residual current (Fig. 6b). Vertical velocities in August and February are increased in the presence of cold-water coral mounds, downward velocities are increased on mound summits, and upward velocities are increased on mound flanks (Fig. 6c–f). Vertical velocities are increased more in February than in August. In August, upward velocities are stronger at the northern mound sides, whereas downward velocities are stronger at the southern side (Fig. 6c–d). There was a significant correlation between mound height and the absolute effect on all hydrodynamic variables, except for downward velocities in February (Fig. 7).
Table 2Hydrodynamic variables used to visualize the effect of cold-water coral mounds on the hydrodynamics and to calculate Spearman rho correlation coefficients. The values display the minimum, mean, and maximum values of the hydrodynamic variables at the locations of cold-water coral mounds (Fig. 1) for the unmodified bathymetry (with mounds, left) and for the smoothed bathymetry (without mounds, right). Vertical velocities are 30 d averages of February or August, and bottom current speed and energy conversion rates are 60 d averages of February + August.
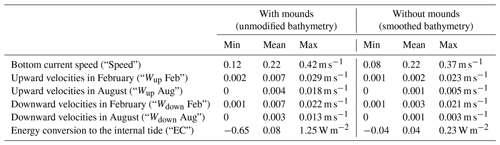
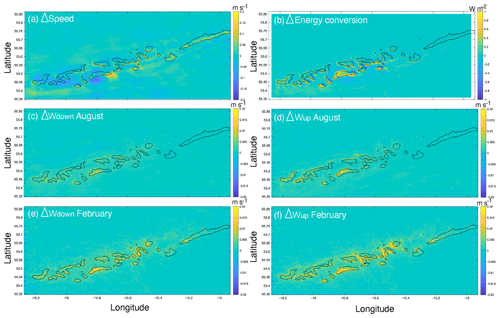
Figure 6The effect of the presence of cold-water coral mounds on bottom current speed (a), energy conversion to the internal tide (b), downward velocities in August (c), upward velocities in August (d), downward velocities in February (e), and upward velocities in February (f). The mound effect was calculated by subtracting the hydrodynamic variables (Table 2) obtained from hydrodynamic simulations without coral mounds from the variables obtained from hydrodynamic simulations with coral mounds. Positive Δ values in the panels thus indicate an enhancing effect of coral mounds on the hydrodynamic variables.
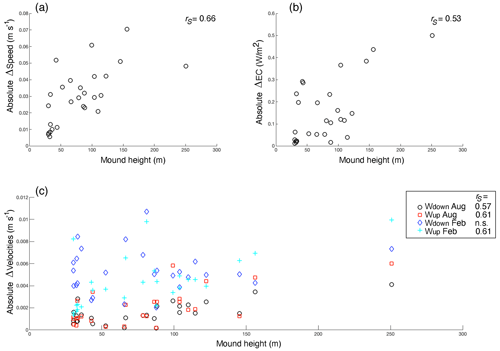
Figure 7The relationships between mound height and the mean absolute effect of mound presence on (a) the bottom current speed, (b) the energy conversion rate to the internal tide, and (c) vertical velocities (i.e. downward and upward velocities in August and February, indicated with different colours and symbols, as in the legend). Significant (p<0.05) Spearman rho correlation coefficients (rS) for the relationships are indicated in the top right of the figures (n=27 mounds), with n.s. denoting correlations that were not significant. Note that the y axes indicate the effect of mound presence on the hydrodynamic variables, not the actual values.
Through mound formation (ecosystem engineering), cold-water corals can feed higher in the water column. This coral mound engineering also affects the hydrodynamics around coral mounds, possibly increasing the food supply towards the reefs (van der Kaaden et al., 2021; Soetaert et al., 2016). Cold-water corals and most of the fauna associated with the deep-reef habitats rely on organic matter originating from close to the surface ocean (de Froe et al., 2022; van Oevelen et al., 2018; Carlier et al., 2009). Our results underline that, at the Logachev cold-water coral mound province, conditions for cold-water coral reefs are better higher in the water column since a high reef cover was positively associated to shallower areas (Figs. 2 and 4), and depth explained a large part of the variation in benthic cover (Fig. 5). To determine how coral mound engineering affects the configuration of cold-water corals reefs, we investigated (1) the environmental control on cold-water coral reefs at multiple spatial scales and (2) which of the environmental factors are or are not engineered (i.e. affected) by the corals (coral mound engineering).
Ventilation and hydrodynamics that stimulate the vertical and lateral food supply have been identified as the most important drivers of cold-water coral reef growth over the past 20 000 years (da Costa Portilho-Ramos et al., 2022). In our analysis we included simulated hydrodynamic variables that indicate increased vertical or lateral food supply or ventilation. By including spatial variables (Moran Eigenvector Maps, MEMs) in our analysis, we were able to investigate whether these hydrodynamic variables had their largest influence on reef cover at the between- or the within-transect scale. We found that reef cover varied most at the scale of the study area (∼25 km), and this variation seems to be associated most with non-engineered (i.e. not under coral control) downward velocities in winter (Sect. 4.1).
By explaining 45.1 % of the variation in benthic cover, our set of variables performed well in comparison to similar deep-sea studies using RDA: e.g. Vad et al. (2020) explained 15.1 % of the variation in benthic cover, and Kazanidis et al. (2021) explained 26.7 % of macrofauna variation. Henry et al. (2013) explained 65 % of the variation in reef fauna using a combination of MEMs, hydrography, and bathymetric variables. So the inclusion of both spatial factors (MEMs) and hydrodynamic variables appears to benefit the analysis. In particular, within-transect MEMs described a relatively large part of the variation in our benthic cover data that was not explained by the environmental variables. Henry et al. (2013) similarly found that community composition was structured mainly by broad-scale environmental forcings, but that fine-scale MEMs described a significant fraction of the variation in community composition. This within-transect variation in benthic cover can be related to spatial patterns of fauna (Henry et al., 2013), such as self-organized regular spatial patterns in coral reefs (van der Kaaden et al., 2023) and the typical reef zonation on cold-water coral mounds (Sect. 4.2).
The variation that remained unexplained in our study might be related to hydrodynamics at a finer resolution or other food supply mechanisms that were not described fully by our set of hydrodynamic variables, such as enhanced surface productivity (Eisele et al., 2011; Wienberg et al., 2022, 2020); downward migration of zooplankton (Guihen et al., 2018; Yahel et al., 2005); other interspecific interactions causing, for example, recycling of material within cold-water coral reefs (Maier et al., 2020a; Henry et al., 2013; Rix et al., 2018); resuspension (de Froe et al., 2022; Mienis et al., 2009); and particle trapping by the reef framework (Mienis et al., 2019; Maier et al., 2021; Bartzke et al., 2021), or to sediment supply, which is important for reef stabilization and coral mound formation (Bartzke et al., 2021; Pirlet et al., 2011; Wang et al., 2021). Furthermore, all transects included in this study were located on cold-water coral mounds and not in between mounds. Since there are no reefs in between cold-water coral mounds (Rengstorf et al., 2014), extending transects to include the off-mound region would likely increase the variation explained by the within-transect spatial scale, and this could emphasize the coral mound engineering effect.
4.1 Broad-scale environmental control on reef cover that is not engineered by cold-water corals
Reef cover (live coral and dead framework) varied most from north to south in the study area. Strong downward velocities in February favour a high reef cover (Fig. 4), likely through an accelerated downward transport of organic matter from near the ocean surface (Soetaert et al., 2016; Frederiksen et al., 1992; Davies et al., 2009; Findlay et al., 2013). Downward velocities in February are stronger with coral mounds present than without coral mounds (Fig. 6c). Modelling the hydrodynamics around one mound of different heights, van der Kaaden et al. (2021) also showed that vertical velocities increased as mound height increased, suggesting a positive feedback mechanism. However, when including all mounds at the Logachev cold-water coral mound province, the magnitude of the effect on downward velocities in February is not correlated to the height of a coral mound (Fig. 7). We thus argue that high reef cover is associated with enhanced downward velocities, mostly due to broad-scale, non-engineered, environmental processes.
Since downward velocities in February did not significantly correlate to downward velocities in August (rS=0.26, p=0.18) and since downward velocities in August were barely associated to reef cover (Fig. 4), reef cover seems mostly steered by processes prevailing in winter and not in summer. At the Rockall trough, deep winter mixing extends as far down into the water column as 600 m (Holliday et al., 2000), and during winter months, dense water is created over the Rockall Bank that cascades down over the Rockall trough margin (White et al., 2005). Both processes cause an accelerated downward flow of organic matter that peaks near the winter–spring transition, at the onset of the phytoplankton bloom that is particularly early (March and/or April) at the Rockall Bank (Mohn and White, 2007; Duineveld et al., 2007). These broad-scale, non-engineered, environmental processes offer a food supply mechanism for the cold-water corals during and shortly after the food-limited winter months (Maier et al., 2020b) and have a larger control on coral survival than food supply mechanisms in summer (Fig. 8a).
This finding suggests that broad-scale environmental processes exert a stronger control on cold-water coral cover than engineered environmental variables. However, the rather coarse resolution of the hydrodynamic simulations might cause us to overestimate the importance of broad-scale environmental variables. Variance partitioning showed that 8.8 % of the variation in benthic cover was explained solely by within-transect MEMs compared to only 2.4 % by between-transect MEMs. This signals that the variation at the within-transect scale might be related to environmental variables at a finer resolution. Such fine-scale variation in hydrodynamic variables is more likely to be caused by the interaction between water flow and fine-scale topography, i.e. ecosystem engineering. Since MEMs 3 to 5, which describe the mound foot-to-summit spatial scale, play a role in explaining the variation in benthic cover, cold-water coral mound ecosystem engineering might cause self-organized variation in benthic cover.
Environmental factors other than the hydrodynamic variables investigated here might also affect coral reef growth and subsequent cold-water coral mound formation. For example, certain water masses (Schulz et al., 2020), the permanent pycnocline (White and Dorschel, 2010), internal waves (Wang et al., 2019; Wienberg et al., 2020), seawater density (Flögel et al., 2014), and (terrestrial) sediment supply (Pirlet et al., 2011; Vandorpe et al., 2017; Lo Iacono et al., 2014) have been suggested to restrain mound formation. Cold-water coral mounds can become buried following changes to the sedimentary regime (Lo Iacono et al., 2014), and for the Logachev cold-water coral mound province, it has been hypothesized that the mounds stopped growing when reaching the permanent pycnocline (White and Dorschel, 2010) or the WTOW upper boundary (Schulz et al., 2020). van der Kaaden et al. (2021) found no levelling off of the engineering effect of the coral mound on the local hydrodynamics, even when the mound was higher than at present. Such a levelling off is also not apparent in our results (Fig. 6). This underlines that, even though coral mounds engineer their local hydrodynamic environment, coral mound formation could be restricted by non-engineered environmental processes. Still, for cold-water coral mounds that are not buried, it can be interesting to investigate the general hydrodynamic regime that arises around cold-water coral mounds and how this regime might explain reef zonation on mounds.
4.2 Coral mound ecosystem engineering causes self-organized reef zonation
Here, we demonstrated the effects of coral mound ecosystem engineering: bottom current speeds are accelerated at some parts of the mound and decelerated in other parts. At the mound flank, upward velocities are enhanced, whereas at the mound summits, downward velocities are enhanced (Fig. 6). Previous studies (e.g. van der Kaaden et al., 2021; Davies et al., 2009; Findlay et al., 2013; Cyr et al., 2016; Mienis et al., 2007) showed that cold-water coral mounds affect downward water motions. Our results also indicate that coral mounds influence downward velocities in February (Fig. 6). However, the magnitude of this effect does not correlate to mound height, likely because of the prevailing influence of non-engineered, broad-scale environmental processes in winter (discussed in Sect. 4.1).
These findings are in line with hydrodynamic theory of water flow passed an object (Juva et al., 2020; Dewey et al., 2005; Baines, 1995; Genin et al., 1986) and with other studies reporting the hydrodynamics around coral mounds (e.g. Genin et al., 1986; de Froe et al., 2022; Findlay et al., 2013; Davies et al., 2009; Juva et al., 2020). Such a regime can be generalized as follows: (1) flow acceleration at the mound side facing the incoming current and at the lee and (2) flow deceleration at the other two mound sides, (3) flow deceleration at the summit if the summit is flat, (4) upwelling along the lower mound flanks, and (5) downwelling around the mound upper flanks and summit. As these typical hydrodynamic zones likely determine the typical benthic cover zones, we will argue in the following that reef zonation on cold-water coral mounds is self-organized.
Cold-water coral mounds show a typical, often-reported benthic zonation (Fig. 2), with mostly sediment cover at the mound foot, coral rubble at the lower flank, dead-coral frameworks on the higher flank, and living corals at the summit (e.g. Cathalot et al., 2015; Davies et al., 2009; Genin et al., 1986; De Clippele et al., 2021; Maier et al., 2021; Vertino et al., 2014; Freiwald and Roberts, 2005; Dorschel et al., 2005). van der Kaaden et al. (2023) found self-organized regular patterns in reef cover on the Logachev cold-water coral mounds, with overall reef cover varying along the transects. This suggests that the overall reef cover along a coral mound is steered by environmental conditions that change along the mound, and that, within these benthic zones, cold-water corals self-organize into regular patterns.
Indeed, we found that high cold-water coral reef and rubble cover correlated to the spatial scale from mound foot-to-summit (MEM 3 and 4) and to strong bottom current speeds (Fig. 4), suggesting that reef growth is more prominent on those mound flanks where (engineered) bottom currents are accelerated (i.e. front and rear) than on the flanks where (engineered) bottom currents are decelerated (Fig. 8b; Mohn et al., 2014). High coral rubble cover correlated to strong upward velocities in August (Fig. 4b), whereas high reef and sediment cover correlated to weak upward velocities in February. This suggests that strong upwelling occurs mostly around the mound flanks and not around the mound summit and foot (Fig. 8b).
Flow induced up- and downwelling over coral mounds is often mentioned as an important food supply mechanism to cold-water coral reefs (Soetaert et al., 2016; Davies et al., 2009; Wagner et al., 2011; Findlay et al., 2014). During a tidal cycle, isopycnals are depressed at the mound flank, and at the turning of the tide, an internal bore is formed that propagates over the mound flank towards the summit (Legg and Klymak, 2008; Mohn et al., 2014). The vertical length scale of these excursions follows the length scale of the mound (van Haren et al., 2014; Cyr et al., 2016). Larger coral mounds generally have a larger effect on hydrodynamics than smaller mounds (Fig. 7; Lim et al., 2018; van der Kaaden et al., 2021). This underlines the mound ecosystem engineering aspect of hydrodynamic zonation and benthic zonation: as the coral mound grows taller, the formerly higher flank regions on the mound that were suitable for coral growth become unsuitable lower flank regions, thereby maintaining the typical reef zonation and causing a so-called Massenerhebung effect (Fig. 8c).
The Massenerhebung effect was first described for the zonation of flora and fauna on terrestrial mountains (Frahm and Gradstein, 1991; Grubb and Whitmore, 1966). Terrestrial mountains exhibit zones with a typical flora and fauna that are determined by various factors such as temperature, humidity, and solar radiation. These zones vary with relative altitude on the mountain but not with the absolute height above the ground because of feedbacks between the size of the mountain and the environment (Grubb, 1971; Grubb and Whitmore, 1966). A perhaps surprising result of this effect is that, for example, the treeline lies higher on higher mountains than on lower mountains. Genin et al. (1986) report that cold-water coral presence is also related to the position on the mound rather than the actual height above the seafloor or depth in the water column. Here, we hypothesize that this Massenerhebung effect, whereby the distribution of organisms is determined by feedbacks between the mountain and the environment, applies to cold-water coral mounds too. Since depth is also an important variable explaining benthic cover, it is likely that the position of cold-water coral reefs on coral mounds is determined by the feedbacks between the mound and the hydrodynamics but that shallower mounds have a denser reef cover than deeper mounds.
4.3 Ecosystem-engineering effects on cold-water corals and climate change
Cold-water coral mounds seem to exhibit a zone with predominantly upward water motions on the mound flanks and a zone with predominantly downward water motions around the mound summit. The summit and upper flank seem to be most suitable for cold-water coral growth, likely because downward water motions accelerate the downward transport of organic matter. In contrast, on the coral mound flanks, coral rubble and dead-coral frameworks are more abundant than live corals. Nutrient-rich deep water is pushed up towards the lower mound flanks (Cyr et al., 2016), and the community associated with the dead coral framework releases substantial amounts of nutrients (Maier et al., 2021). These nutrients might be transported by the predominantly upward water motions towards the ocean surface (Soetaert et al., 2016; Findlay et al., 2014; de Froe et al., 2022). This might benefit the coral reefs as a global review (Maier et al., 2023) and studies on millennial timescales (Eisele et al., 2011; Wienberg et al., 2022) show the benefits of increased primary productivity for coral reef growth. The upward water motions around the mound flanks likely also resuspend deposited particles. These particles are transported higher up the mound by lateral advection where they supply the corals with additional food (Findlay et al., 2013; Davies et al., 2009; Mienis et al., 2009).
Factors other than food supply also influence cold-water coral growth, such as biogeochemical seawater properties (Flögel et al., 2014; da Costa Portilho-Ramos et al., 2022; Fink et al., 2012). Deep water that is upwelled around cold-water coral mounds typically has lower temperatures, oxygen content, and aragonite saturation than water from higher in the water column that is downwelled (Findlay et al., 2013, 2014; Mienis et al., 2007; Flögel et al., 2014). The mound zone influenced by downward water motions therefore likely experiences higher temperatures, oxygen content, and aragonite saturation state than the region of upward water motions. While higher oxygen content and aragonite saturation state would benefit cold-water corals (Fink et al., 2012; Dodds et al., 2007; Tittensor et al., 2009), higher temperatures might have an adverse effect (Morato et al., 2020; Chapron et al., 2021). Currently, most cold-water corals occur within their thermal tolerance (Mienis et al., 2007; Chapron et al., 2021). A higher temperature may be beneficial but only up to the upper limit of the thermal tolerance range (Büscher et al., 2022; Dodds et al., 2007).
Increased temperatures from global change increase the energy demand of cold-water corals (Dodds et al., 2007; Dorey et al., 2020; Chapron et al., 2021). When not compensated for by sufficient food supply, the framework-forming cold-water coral habitat is expected to move to deeper waters (Morato et al., 2020). Our results show that this poses a problem to cold-water corals and their associated fauna living on mounds as the corals prefer the downwelling zone where they profit from a higher vertical particle flux, oxygen content, and aragonite saturation state. The temperatures in this zone, however, might become unfavourable in the future. Further down the mound flank is the upwelling zone that seems less suitable for cold-water coral growth. Studies looking into the geological history of cold-water coral reefs have shown the sensitivity of cold-water coral reefs to a decreased food supply (e.g. Frank et al., 2011; Wienberg et al., 2010; da Costa Portilho-Ramos et al., 2022; Fink et al., 2013; Eisele et al., 2011). This suggests that cold-water corals on cold-water coral mounds might not be able to change their position towards the lower mound flank to escape the adverse effects of climate change.
A sufficient food supply may compensate for adverse environmental effects up to a certain limit (Büscher et al., 2017; Dorey et al., 2020; da Costa Portilho-Ramos et al., 2022; Hebbeln et al., 2020). But we show that the hydrodynamically stimulated food supply in winter is one of the major factors controlling coral reef cover, at least at the Logachev cold-water coral mound province. Such vertical mixing is thought to dampen with climate change since stratification might increase (Gerkema et al., 2004; Müller et al., 2014; Pereira et al., 2002), possibly decreasing benthic–pelagic coupling (Capotondi et al., 2012; Li et al., 2018; Reid et al., 2009). So, in the worst possible case, climate change might make cold-water coral mounds entirely less suitable for cold-water coral growth.
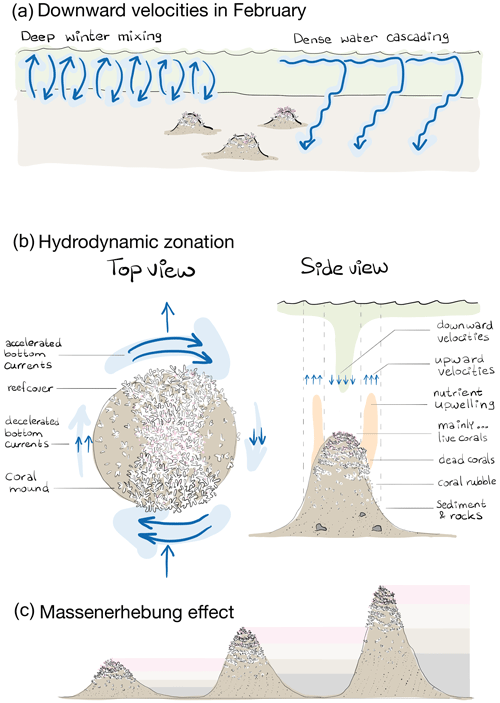
Figure 8(a) Schematic representation of the broad-scale environmental control on reef cover, i.e. downward velocities in February likely resulting from deep winter mixing and dense-water cascading. Note that the two processes happen at the same time and place but are separated here in space for clarity. (b) Schematic representation of the hydrodynamic zones in a top and side view and the associated benthic zones of “mainly … live corals, dead corals, coral rubble, sediment, and rocks”. The top view shows that bottom currents are accelerated at the front and rear of the mound and decelerated at the right and left side, looking downstream of the incoming flow. Reef cover is higher on those sides of the mound with accelerated currents and at the mound summit. The side view shows the benthic zones of mainly live corals at the mound summit, dead coral cover at the higher flank, coral rubble at the lower flank, and sediment with rocks at the mound foot. The zone of upward velocities and upwelling of nutrients released by the community associated with dead coral frameworks occurs around the mound flanks, whereas downward velocities with transport of organic matter from near the surface happens around the mound summit. The up- and downward water motions do not necessarily happen simultaneously but can result from (spring neap) tidal motions. (c) Schematic representation of the Massenerhebung effect on three mounds of different sizes with the benthic zones of mainly live corals (pink), dead corals (light brown), coral rubble (dark brown), and sediment (grey). The Massenerhebung effect means that reef cover is determined by the position on the mound rather than the actual height above the seafloor or depth below the sea surface.
We showed that downward velocities in winter, likely driven by non-engineered processes as deep winter mixing and dense-water cascading, control reef growth to such an extent that reef cover differs substantially at the scale of the Logachev cold-water coral mound province. These broad-scale hydrodynamically enhanced food delivery processes in winter seem to influence reef cover more than processes related to coral mound ecosystem engineering and are more important for cold-water coral survival than hydrodynamically enhanced food delivery in summer. Nonetheless, by engineering long-lasting mounds, cold-water corals can stimulate the vertical transport of particles from near the ocean surface towards the mound summits. As coral mounds grow, they increasingly affect the hydrodynamics around them and thereby affect cold-water coral reef growth. The initiated hydrodynamic zones shift as the mound grows, leading to a typical reef zonation whereby mound upper flanks and summits have the densest reef cover. Reef zonation on cold-water coral mounds is therefore controlled by coral mound engineering, and reef cover is determined by the position on the mound rather than the actual height above the seafloor or depth in the water column (i.e. the Massenerhebung effect).
Much research has shown the benefits of cold-water coral mound engineering for the corals (e.g. Soetaert et al., 2016; van der Kaaden et al., 2021, 2020, 2023; Findlay et al., 2013; Davies et al., 2009), but here, we show that the hydrodynamic zones around cold-water coral mounds might restrict cold-water corals to grow deeper on a mound to escape the adverse effects of climate warming. When studying cold-water coral ecosystems and their response to global change, the effects of broad-scale, non-engineered environmental processes should not be underestimated, and it is especially important to gain a better understanding of how benthic–pelagic coupling will change in winter and to quantify how much alternative food supply mechanisms and coral mound engineering could compensate for a lower food availability.
Data used in this research from Maier et al. (2021) (raw data for “Reef communities associated with “dead” cold-water coral framework drive high resource retention and fast recycling in the deep sea”) are already published and properly cited in this paper and are publicly available at https://doi.org/10.5281/zenodo.4076146 (Maier et al., 2021). Data used in this research from De Clippele et al. (2021) (environmental data and image area measurements of different substrate types extracted from video transects recorded in the Logachev cold-water coral mound province) are already published and properly cited in this paper and are publicly available at https://doi.org/10.1594/PANGAEA.959612 (De Clippele et al., 2023).
ASvdK, SC, EdF, and DvO were involved in the conceptualization and design of the study. LHDC, FM, and SRM provided the videos and annotated images. ASvdK, SC, LHDC, and SRM were involved in data analysis. DvO supervised the study. DvO, JvdK, MR, and KS were involved in securing funding for this research. ASvdK wrote the paper. FM collected the video data. All co-authors provided input to the study design, data analysis, data visualization, paper writing, and paper revisions.
The contact author has declared that none of the authors has any competing interests.
Publisher’s note: Copernicus Publications remains neutral with regard to jurisdictional claims made in the text, published maps, institutional affiliations, or any other geographical representation in this paper. While Copernicus Publications makes every effort to include appropriate place names, the final responsibility lies with the authors.
We thank two anonymous reviewers and Andres Rüggeberg for their valuable comments that improved our manuscript.
This research has been made possible due to collaboration funding between the Royal Dutch Institute for Sea Research and Utrecht University. Sandra R. Maier was funded by the Greenland Research Council. Laurence H. De Clippele and Christian Mohn were funded by the European Union's Horizon 2020 research and innovation programme under grant agreement no. 818123 (iAtlantic). The output of this study reflects only the authors' views, and the European Union cannot be held responsible for any use that may be made of the information contained therein. Funding for video collection was provided by the Netherlands Organisation for Scientific Research (NWO-VENI grant no. 863.11.012).
This paper was edited by Peter Landschützer and reviewed by Andres Rüggeberg and two anonymous referees.
Baines, P. G.: Topographic effects in stratified flows, Cambridge University Press, ISBN 9781108722902, 1995.
Bartzke, G., Siemann, L., Büssing, R., Nardone, P., Koll, K., Hebbeln, D., and Huhn, K.: Investigating the Prevailing Hydrodynamics Around a Cold-Water Coral Colony Using a Physical and a Numerical Approach, Front. Mar. Sci., 8, 1–17, https://doi.org/10.3389/fmars.2021.663304, 2021.
Bastiaansen, R., Doelman, A., Eppinga, M. B., and Rietkerk, M.: The effect of climate change on the resilience of ecosystems with adaptive spatial pattern formation, Ecol. Lett., 23, 414–429, https://doi.org/10.1111/ele.13449, 2020.
Blanchet, F. G., Legendre, P., and Borcard, D.: Forward selection of explanatory variables, Ecology, 89, 2623–2632, https://doi.org/10.1890/07-0986.1, 2008.
Bongiorni, L., Mea, M., Gambi, C., Pusceddu, A., Taviani, M., and Danovaro, R.: Deep-water scleractinian corals promote higher biodiversity in deep-sea meiofaunal assemblages along continental margins, Biol. Conserv., 143, 1687–1700, https://doi.org/10.1016/j.biocon.2010.04.009, 2010.
Borcard, D., Gillet, F., and Legendre, P.: Numerical Ecology with R, edited by: Gentleman, R., Hornik, K., and Parmigiani, G., Springer Science & Business Media, 21–54, https://doi.org/10.1007/978-1-4419-7976-6, 2011.
Büscher, J. V., Form, A. U., and Riebesell, U.: Interactive effects of ocean acidification and warming on growth, fitness and survival of the cold-water coral Lophelia pertusa under different food availabilities, Front. Mar. Sci., 4, https://doi.org/10.3389/fmars.2017.00101, 2017.
Büscher, J. V., Form, A. U., Wisshak, M., Kiko, R., and Riebesell, U.: Cold-water coral ecosystems under future ocean change: Live coral performance vs. framework dissolution and bioerosion, Limnol. Oceanogr., 67, 2497–2515, https://doi.org/10.1002/lno.12217, 2022.
Capotondi, A., Alexander, M. A., Bond, N. A., Curchitser, E. N., and Scott, J. D.: Enhanced upper ocean stratification with climate change in the CMIP3 models, J. Geophys. Res., 117, 1–23, https://doi.org/10.1029/2011JC007409, 2012.
Carlier, A., Le Guilloux, E., Olu, K., Sarrazin, J., Mastrototaro, F., Taviani, M., and Clavier, J.: Trophic relationships in a deep Mediterranean cold-water coral bank (Santa Maria di Leuca, Ionian Sea), Mar. Ecol. Prog. Ser., 397, 125–137, https://doi.org/10.3354/meps08361, 2009.
Cathalot, C., Van Oevelen, D., Cox, T. J. S., Kutti, T., Lavaleye, M., Duineveld, G., and Meysman, F. J. R.: Cold-water coral reefs and adjacent sponge grounds: hotspots of benthic respiration and organic carbon cycling in the deep sea, Front. Mar. Sci., 2, 1–12, https://doi.org/10.3389/fmars.2015.00037, 2015.
Chapron, L., Galand, P. E., Pruski, A. M., Peru, E., Vétion, G., Robin, S., and Lartaud, F.: Resilience of cold-water coral holobionts to thermal stress, P. Roy. Soc. B-Bio., 288, 1965, https://doi.org/10.1098/rspb.2021.2117, 2021.
De Clippele, L. H., van der Kaaden, A. S., Maier, S. R., de Froe, E., and Roberts, J. M.: Biomass Mapping for an Improved Understanding of the Contribution of Cold-Water Coral Carbonate Mounds to C and N Cycling, Front. Mar. Sci., 8, https://doi.org/10.3389/fmars.2021.721062, 2021.
da Costa Portilho-Ramos, R., Titschack, J., Wienberg, C., Rojas, M. G. S., Yokoyama, Y., and Hebbeln, D.: Major environmental drivers determining life and death of cold-water corals through time, PLoS Biol, 20, https://doi.org/10.1371/journal.pbio.3001628, 2022.
Crooks, J. A.: Characterizing ecosystem-level consequences of biological invasions: the role of ecosystem engineers, OIKOS, 97, 153–166, 2002.
Cyr, F., Van Haren, H., Mienis, F., Duineveld, G., and Bourgault, D.: On the influence of cold-water coral mound size on flow hydrodynamics, and vice versa, Geophys. Res. Lett., 43, 1–9, https://doi.org/10.1002/2015GL067038, 2016.
Davies, A. J., Duineveld, G. C. A., Lavaleye, M. S. S., Bergman, M. J. N., van Haren, H., and Roberts, J. M.: Downwelling and deep-water bottom currents as food supply mechanisms to the cold-water coral Lophelia pertusa (Scleractinia) at the Mingulay Reef complex, Limnol. Oceanogr., 54, 620–629, https://doi.org/10.4319/lo.2009.54.2.0620, 2009.
De Clippele, L., van der Kaaden, A.-S., Maier S. M., de Froe, E., and Roberts, M.: Environmental data and image area measurements of different substrate types extracted from video transects recorded in the Logachev cold-water coral mound province, Pangaea [data set], https://doi.org/10.1594/PANGAEA.959612, 2023.
de Froe, E., Maier, S. R., Horn, H. G., Wolff, G. A., Blackbird, S., Mohn, C., Schultz, M., van der Kaaden, A., Cheng, C. H., Wubben, E., van Haastregt, B., Friis Moller, E., Lavaleye, M., Soetaert, K., Reichart, G., and van Oevelen, D.: Hydrography and food distribution during a tidal cycle above a cold-water coral mound, Deep-Sea Res. Pt. I, 189, 103854, https://doi.org/10.1016/j.dsr.2022.103854, 2022.
de Haas, H., Mienis, F., Frank, N., Richter, T. O., Steinacher, R., de Stigter, H., van der Land, C., and van Weering, T. C. E.: Morphology and sedimentology of (clustered) cold-water coral mounds at the south Rockall Trough margins, NE Atlantic Ocean, Facies, 55, 1–26, https://doi.org/10.1007/s10347-008-0157-1, 2009.
Dewey, R., Richmond, D., and Garrett, C.: Stratified tidal flow over a bump, J. Phys. Oceanogr., 35, 1911–1927, https://doi.org/10.1175/JPO2799.1, 2005.
Dodds, L. A., Roberts, J. M., Taylor, A. C., and Marubini, F.: Metabolic tolerance of the cold-water coral Lophelia pertusa (Scleractinia) to temperature and dissolved oxygen change, J. Exp. Mar. Biol. Ecol., 349, 205–214, https://doi.org/10.1016/j.jembe.2007.05.013, 2007.
Dorey, N., Gjelsvik, Ø., Kutti, T., and Büscher, J. V.: Broad Thermal Tolerance in the Cold-Water Coral Lophelia pertusa From Arctic and Boreal Reefs, Front. Physiol., 10, https://doi.org/10.3389/fphys.2019.01636, 2020.
Dorschel, B., Hebbeln, D., Ruggeberg, A., and Dullo, C.: Carbonate budget of a cold-water coral carbonate mound: Propeller Mound, Porcupine Seabight, Int. J. Earth Sci., 96, 73–83, https://doi.org/10.1007/s00531-005-0493-0, 2005.
Dray, S., Bauman, D., Blanchet, G., Borcard, D., Clappe, S., Guénard, G., Jombart, T., Larocque, G., Legendre, P., Madi, N., and Wagner, H. H.: _adespatial: Multivariate Multiscale Spatial Analysis_. R package version 0.3-20, https://CRAN.R-project.org (last access: 13 February 2024), 2022.
Duineveld, G. C. A., Lavaleye, M. S. S., Bergman, M. J. N., de Stigter, H., and Mienis, F.: Trophic structure of a cold-water coral mound community (Rockall Bank, NE Atlantic) in relation to the near-bottom particle supply and current regime, Bull. Mar. Sci., 81, 449–467, https://doi.org/10.1080/17451000.2017.1398404, 2007.
Duineveld, G. C. A., Jeffreys, R. M., Lavaleye, M. S. S., Davies, A. J., Bergman, M. J. N., Watmough, T., and Witbaard, R.: Spatial and tidal variation in food supply to shallow cold-water coral reefs of the Mingulay Reef complex (Outer Hebrides, Scotland), Mar. Ecol. Prog. Ser., 444, 97–115, https://doi.org/10.3354/meps09430, 2012.
Eisele, M., Frank, N., Wienberg, C., Hebbeln, D., López Correa, M., Douville, E., and Freiwald, A.: Productivity controlled cold-water coral growth periods during the last glacial off Mauritania, Mar. Geol., 280, 143–149, https://doi.org/10.1016/j.margeo.2010.12.007, 2011.
Findlay, H. S., Artioli, Y., Moreno Navas, J., Hennige, S. J., Wicks, L. C., Huvenne, V. A. I., Woodward, E. M. S., and Roberts, J. M.: Tidal downwelling and implications for the carbon biogeochemistry of cold-water corals in relation to future ocean acidification and warming, Global Change Biol., 19, 2708–2719, https://doi.org/10.1111/gcb.12256, 2013.
Findlay, H. S., Hennige, S. J., Wicks, L. C., Navas, J. M., Woodward, E. M. S., and Roberts, J. M.: Fine-scale nutrient and carbonate system dynamics around cold-water coral reefs in the northeast Atlantic, Sci. Rep., 4, 1–10, https://doi.org/10.1038/srep03671, 2014.
Fink, H. G., Wienberg, C., Hebbeln, D., Mcgregor, H. V, Schmiedl, G., Taviani, M., and Freiwald, A.: Oxygen control on Holocene cold-water coral development in the eastern Mediterranean Sea, Deep-Sea Res. Pt. I, 62, 89–96, https://doi.org/10.1016/j.dsr.2011.12.013, 2012.
Fink, H. G., Wienberg, C., De Pol-Holz, R., Wintersteller, P., and Hebbeln, D.: Cold-water coral growth in the Alboran Sea related to high productivity during the Late Pleistocene and Holocene, Mar. Geol., 339, 71–82, https://doi.org/10.1016/j.margeo.2013.04.009, 2013.
Flögel, S., Dullo, W. Chr., Pfannkuche, O., Kiriakoulakis, K., and Rüggeberg, A.: Geochemical and physical constraints for the occurrence of living cold-water corals, Deep-Sea Res. Pt. II, 99, 19–26, https://doi.org/10.1016/j.dsr2.2013.06.006, 2014.
Frahm, J.-P. and Gradstein, R. S.: An Altitudinal Zonation of Tropical Rain Forests Using Bryophytes, J. Biogeogr., 18, 669–678, 1991.
Frank, N., Freiwald, A., López Correa, M., Wienberg, C., Eisele, M., Hebbeln, D., Van Rooij, D., Henriet, J. P., Colin, C., van Weering, T., de Haas, H., Buhl-Mortensen, P., Roberts, J. M., De Mol, B., Douville, E., Blamart, D., and Hatté, C.: Northeastern Atlantic cold-water coral reefs and climate, Geology, 39, 743–746, https://doi.org/10.1130/G31825.1, 2011.
Frederiksen, R., Jensen, A., and Westerberg, H.: The distribution of the scleractinian coral Lophelia pertusa around the Faroe islands and the relation to internal tidal mixing, Sarsia, 77, 157–171, https://doi.org/10.1080/00364827.1992.10413502, 1992.
Freiwald, A. and Roberts, J. M.: Cold-Water Corals and Ecosystems, 1st ed., Springer-Verlag, 1243 pp., 2005.
Genin, A., Dayton, P. K., Lonsdale, P. F., and Spiess, F. N.: Corals on seamount peaks provide evidence of current acceleration over deep-sea topography, Nature, 322, 59–61, 1986.
Gerkema, T., Lam, F. P. A., and Maas, L. R. M.: Internal tides in the Bay of Biscay: Conversion rates and seasonal effects, Deep-Sea Res. Pt. II, 51, 2995–3008, https://doi.org/10.1016/j.dsr2.2004.09.012, 2004.
Grubb, P. J.: Interpretation of the “Massenerhebung” Effect on Tropical Mountains, Nature, 229, 44–45, https://doi.org/10.1038/229044a0, 1971.
Grubb, P. J. and Whitmore, T. C.: A Comparison of Montane and Lowland Rain Forest in Ecuador: II. The Climate and its Effects on the Distribution and Physiognomy of the Forests, J. Ecol., 54, 303–333, 1966.
Guihen, D., White, M., and Lundälv, T.: Zooplankton drive diurnal changes in oxygen concentration at Tisler cold-water coral reef, Coral Reefs, 37, 1013–1025, https://doi.org/10.1007/s00338-018-1711-0, 2018.
Hastings, A., Byers, J. E., Crooks, J. A., Cuddington, K., Jones, C. G., Lambrinos, J. G., Talley, T. S., and Wilson, W. G.: Ecosystem engineering in space and time, Ecol. Lett., 10, 153–164, https://doi.org/10.1111/j.1461-0248.2006.00997.x, 2007.
Hebbeln, D., Wienberg, C., Dullo, W. C., Freiwald, A., Mienis, F., Orejas, C., and Titschack, J.: Cold-water coral reefs thriving under hypoxia, Coral Reefs, 39, 853–859, https://doi.org/10.1007/s00338-020-01934-6, 2020.
Hennige, S. J., Larsson, A. I., Orejas, C., Gori, A., De Clippele, L. H., Lee, Y. C., Jimeno, G., Georgoulas, K., Kamenos, N. A., and Roberts, J. M.: Using the Goldilocks Principle to model coral ecosystem engineering, P. Roy. Soc. B-Bio., 288, 1956, https://doi.org/10.1098/rspb.2021.1260, 2021.
Henry, L.-A., Moreno Navas, J., and Roberts, J. M.: Multi-scale interactions between local hydrography, seabed topography, and community assembly on cold-water coral reefs, Biogeosciences, 10, 2737–2746, https://doi.org/10.5194/bg-10-2737-2013, 2013.
Holliday, N. P., Pollard, R. T., Read, J. F., and Leach, H.: Water mass properties and fluxes in the Rockall Trough, 1975–1998, Deep-Sea Res. Pt. I, 47, 1303–1332, https://doi.org/10.1016/S0967-0637(99)00109-0, 2000.
Lo Iacono, C., Gràcia, E., Ranero, C. R., Emelianov, M., Huvenne, V. A. I., Bartolomé, R., Booth-Rea, G., Prades, J., Ambrosog, S., Dominguezg, C., Grinyóg, J., Rubioh, E., and Torrenth, J.: The West Melilla cold water coral mounds, Eastern Alboran Sea: Morphological characterization and environmental context, Deep-Sea Res. Pt. II, 99, 316–326, https://doi.org/10.1016/j.dsr2.2013.07.006, 2014.
Johnson, C., Sherwin, T., Smythe-Wright, D., Shimmield, T., and Turrel, W.: Wyville Thomson Ridge Overflow Water: Spatial and temporal distribution in the Rockall Trough, Deep-Sea Res. Pt. I,, 57, 1153–1162, https://doi.org/10.1016/j.dsr.2010.07.006, 2010.
Jones, C. G., Lawton, J. H., Shachak, M., Jones, C. G., Lawton, J. H., and Shachak, M.: Organisms as Ecosystem Engineers, Oikos, 69, 373–386, 1994.
Juva, K., Flögel, S., Karstensen, J., Linke, P., and Dullo, W.-C.: Tidal dynamics control on cold-water coral growth: A high-resolution multivariable study on eastern Atlantic cold-water coral sites, Front. Mar. Sci., 7, 132, https://doi.org/10.3389/FMARS.2020.00132, 2020.
Kazanidis, G., Henry, L. A., Vad, J., Johnson, C., De Clippele, L. H., and Roberts, J. M.: Sensitivity of a cold-water coral reef to interannual variability in regional oceanography, Divers. Distrib., 27, 1719–1731, https://doi.org/10.1111/ddi.13363, 2021.
Kenyon, N. H., Akhmetzhanov, A. M., Wheeler, A. J., van Weering, T. C. E., de Haas, H., and Ivanov, M. K.: Giant carbonate mud mounds in the southern Rockall Trough, Mar. Geol., 195, 5–30, https://doi.org/10.1016/S0025-3227(02)00680-1, 2003.
Legg, S. and Klymak, J.: Internal Hydraulic Jumps and Overturning Generated by Tidal Flow over a Tall Steep Ridge, J. Phys. Oceanogr., 38, 1949–1964, https://doi.org/10.1175/2008jpo3777.1, 2008.
Li, G., Cheng, L., Zhu, J., Trenberth, K. E., Mann, M. E., and Abraham, J. P.: Increasing ocean stratification over the past half-century, Nat. Clim. Change, 10, 1116–1123, https://doi.org/10.1038/s41558-020-00918-2, 2018.
Lim, A., Huvenne, V. A. I., Vertino, A., Spezzaferri, S., and Wheeler, A. J.: New insights on coral mound development from groundtruthed high-resolution ROV-mounted multibeam imaging, Mar. Geol., 403, 225–237, https://doi.org/10.1016/j.margeo.2018.06.006, 2018.
Maier, S. R., Kutti, T., Bannister, R. J., Fang, J. K. H., van Breugel, P., van Rijswijk, P., and van Oevelen, D.: Recycling pathways in cold-water coral reefs: Use of dissolved organic matter and bacteria by key suspension feeding taxa, Sci. Rep., 10, 1–13, https://doi.org/10.1038/s41598-020-66463-2, 2020a.
Maier, S. R., Bannister, R. J., van Oevelen, D., and Kutti, T.: Seasonal controls on the diet, metabolic activity, tissue reserves and growth of the cold-water coral Lophelia pertusa, Coral Reefs, 39, 173–187, https://doi.org/10.1007/s00338-019-01886-6, 2020b.
Maier, S. R., Mienis, F., de Froe, E., Soetaert, K., Lavaleye, M., Duineveld, G., Beauchard, O., van der Kaaden, A.-S., Koch, B. P., and van Oevelen, D.: Reef communities associated with “dead” cold-water coral framework drive resource retention and recycling in the deep sea, Deep-Sea Res. Pt. I, 175, 103574, https://doi.org/10.1016/j.dsr.2021.103574, 2021a.
Maier, S. R., Mienis, F., de Froe, E., Soetaert, K., Lavalaye, M., Duineveld, G., Beauchard, O., van der Kaaden, A.-S., Koch, B., abd van Oevelen, D.: Raw data for “Reef communities associated with “dead” cold-water coral framework drive high resource retention and fast recycling in the deep sea”, Zenodo [data set], https://doi.org/10.5281/zenodo.4076146, 2021b.
Maier, S. R., Brooke, S., De Clippele, L. H., de Froe, E., van der Kaaden, A., Kutti, T., Mienis, F., and van Oevelen, D.: On the paradox of thriving cold-water coral reefs in the food-limited deep sea, Biol. Rev., 98, 1768–1795, https://doi.org/10.1111/brv.12976, 2023.
Mienis, F., van Weering, T., de Haas, H., de Stigter, H., Huvenne, V., and Wheeler, A.: Carbonate mound development at the SW Rockall Trough margin based on high resolution TOBI and seismic recording, Mar. Geol., 233, 1–19, https://doi.org/10.1016/j.margeo.2006.08.003, 2006.
Mienis, F., de Stigter, H. C., White, M., Duineveld, G., de Haas, H., and van Weering, T. C. E.: Hydrodynamic controls on cold-water coral growth and carbonate-mound development at the SW and SE Rockall Trough Margin, NE Atlantic Ocean, Deep-Sea Res. Pt. I, 54, 1655–1674, https://doi.org/10.1016/j.dsr.2007.05.013, 2007.
Mienis, F., de Stigter, H. C., de Haas, H., and van Weering, T. C. E.: Near-bed particle deposition and resuspension in a cold-water coral mound area at the Southwest Rockall Trough margin, NE Atlantic, Deep-Sea Res. Pt. I, 56, 1026–1038, https://doi.org/10.1016/j.dsr.2009.01.006, 2009.
Mienis, F., Bouma, T. J., Witbaard, R., van Oevelen, D., and Duineveld, G. C. A.: Experimental assessment of the effects of cold-water coral patches on water flow, Mar. Ecol. Prog. Ser., 609, 101–117, https://doi.org/10.3354/meps12815, 2019.
Mohn, C. and White, M.: Remote sensing and modelling of bio-physical distribution patterns at Porcupine and Rockall Bank, Northeast Atlantic, Cont. Shelf Res., 27, 1875–1892, https://doi.org/10.1016/j.csr.2007.03.006, 2007.
Mohn, C., Rengstorf, A., White, M., Duineveld, G., Mienis, F., Soetaert, K., and Grehan, A.: Linking benthic hydrodynamics and cold-water coral occurrences: A high-resolution model study at three cold-water coral provinces in the NE Atlantic, Prog. Oceanogr., 122, 92–104, https://doi.org/10.1016/j.pocean.2013.12.003, 2014.
Morato, T., González-Irusta, J. M., Dominguez-Carrió, C., Wei, C. L., Davies, A., Sweetman, A. K., Taranto, G. H., Beazley, L., García-Alegre, A., Grehan, A., Laffargue, P., Murillo, F. J., Sacau, M., Vaz, S., Kenchington, E., Arnaud-Haond, S., Callery, O., Chimienti, G., Cordes, E., Egilsdottir, H., Freiwald, A., Gasbarro, R., Gutiérrez-Zárate, C., Gianni, M., Gilkinson, K., Wareham Hayes, V. E., Hebbeln, D., Hedges, K., Henry, L. A., Johnson, D., Koen-Alonso, M., Lirette, C., Mastrototaro, F., Menot, L., Molodtsova, T., Durán Muñoz, P., Orejas, C., Pennino, M. G., Puerta, P., Ragnarsson, S., Ramiro-Sánchez, B., Rice, J., Rivera, J., Roberts, J. M., Ross, S. W., Rueda, J. L., Sampaio, Í., Snelgrove, P., Stirling, D., Treble, M. A., Urra, J., Vad, J., van Oevelen, D., Watling, L., Walkusz, W., Wienberg, C., Woillez, M., Levin, L. A., and Carreiro-Silva, M.: Climate-induced changes in the suitable habitat of cold-water corals and commercially important deep-sea fishes in the North Atlantic, Global Change Biol., 26, 2181–2202, https://doi.org/10.1111/gcb.14996, 2020.
Müller, M., Cherniawsky, J. Y., Foreman, M. G. G., and Von Storch, J. S.: Seasonal variation of the M 2 tide, Ocean Dynam., 64, 159–177, https://doi.org/10.1007/s10236-013-0679-0, 2014.
Nakatsuka, T., Handa, N., Harada, N., Sugimoto, T., and Imaizumi, S.: Origin and decomposition of sinking particulate organic matter in the deep water column inferred from the vertical distributions of its δ15N, δ13 and δ14, Deep-Sea Res. Pt. I, 44, 1957–1979, https://doi.org/10.1016/s0967-0637(97)00051-4, 1997.
Oksanen, J., Simpson, G., Blanchet, F., Kindt, R., Legendre, P., Minchin, P., O'Hara, R., Solymos, P., Stevens, M., Szoecs, E., Wagner, H., Barbour, M., Bedward, M., Bolker, B., Borcard, D., Carvalho, G., Chirico, M., de Caceres, M., Durand, S., Evangelista, H., FitzJohn, R., Friendly, M., Furneaux, B., Hannigan, G., Hill, M., Lathi, L., McGlinn, D., Ouellette, M., Ribeiro Cunha, E., Smith, T., Stier, A., ter Braak, C., and Weedon, J.: _vegan: Community Ecology Package_. R package version 2.6-4, https://cran.r-project.org/web/packages/vegan/index.html (last access: 13 February 2024), 2022.
Pereira, A. F., Beckmann, A., and Hellmer, H. H.: Tidal Mixing in the Southern Weddell Sea: Results from a Three-Dimensional Model, J. Phys. Ocean., 32, 2151–2170, https://doi.org/10.1175/1520-0485(2002)032<2151:TMITSW>2.0.CO;2, 2002.
Pirlet, H., Colin, C., Thierens, M., Latruwe, K., Van Rooij, D., Foubert, A., Frank, N., Blamart, D., Huvenne, V. A. I., Swennen, R., Vanhaecke, F., and Henriet, J. P.: The importance of the terrigenous fraction within a cold-water coral mound: A case study, Mar. Geol., 282, 13–25, https://doi.org/10.1016/j.margeo.2010.05.008, 2011.
Ragnarsson, S. Á., Burgos, J. M., Kutti, T., van den Beld, I., Egilsdóttir, H., Arnaud-Haond, S., and Grehan, A.: The Impact of Anthropogenic Activity on Cold-Water Corals, in: Marine Animal Forests, Springer International Publishing, 1–35, https://doi.org/10.1007/978-3-319-17001-5_27-1, 2016.
Reid, P. C., Fischer, A. C., Lewis-Brown, E., Meredith, M. P., Sparrow, M., Andersson, A. J., Antia, A., Bates, N. R., Bathmann, U., Beaugrand, G., Brix, H., Dye, S., Edwards, M., Furevik, T., Gangstø, R., Hátún, H., Hopcroft, R. R., Kendall, M., Kasten, S., Keeling, R., Le Quéré, C., Mackenzie, F. T., Malin, G., Mauritzen, C., Ólafsson, J., Paull, C., Rignot, E., Shimada, K., Vogt, M., Wallace, C., Wang, Z., and Washington, R.: Impacts of the Oceans on Climate Change, Adv. Mar. Biol., 56, 1–150, 2009.
Rengstorf, A. M., Mohn, C., Brown, C., Wisz, M. S., and Grehan, A. J.: Predicting the distribution of deep-sea vulnerable marine ecosystems using high-resolution data: Considerations and novel approaches,Deep-Sea Res. Pt. I, 93, 72–82, https://doi.org/10.1016/j.dsr.2014.07.007, 2014.
Rietkerk, M., Bastiaansen, R., Banerjee, S., Koppel, J. Van De, Baudena, M., and Doelman, A.: Evasion of tipping in complex systems through spatial pattern formation, Science, 169, https://doi.org/10.1126/science.abj0359, 2021.
Rix, L., De Goeij, J. M., Van Oevelen, D., Struck, U., Al-Horani, F. A., Wild, C., and Naumann, M. S.: Reef sponges facilitate the transfer of coral-derived organic matter to their associated fauna via the sponge loop, Mar. Ecol. Prog. Ser., 589, 85–96, https://doi.org/10.3354/meps12443, 2018.
Roberts, J. M. and shipboard party: Changing Oceans Expedition 2012, RRS James Cook 073 Cruise Report, 224 pp., 2013.
Schulz, K., Soetaert, K., Mohn, C., Korte, L., Mienis, F., Duineveld, G., and van Oevelen, D.: Linking large-scale circulation patterns to the distribution of cold water corals along the eastern Rockall Bank (northeast Atlantic), J. Mar. Syst., 212, 103456, https://doi.org/10.1016/j.jmarsys.2020.103456, 2020.
Shchepetkin, A. F. and McWilliams, J. C.: The regional oceanic modeling system (ROMS): A split-explicit, free-surface, topography-following-coordinate oceanic model, Ocean Model (Oxf), 9, 347–404, https://doi.org/10.1016/j.ocemod.2004.08.002, 2005.
Snelgrove, P. V. R., Soetaert, K., Solan, M., Thrush, S., Wei, C. L., Danovaro, R., Fulweiler, R. W., Kitazato, H., Ingole, B., Norkko, A., Parkes, R. J., and Volkenborn, N.: Global Carbon Cycling on a Heterogeneous Seafloor, Trends Ecol Evol, 33, https://doi.org/10.1016/j.tree.2017.11.004, 2017.
Soetaert, K., Mohn, C., Rengstorf, A., Grehan, A., and Van Oevelen, D.: Ecosystem engineering creates a direct nutritional link between 600-m deep cold-water coral mounds and surface productivity, Sci Rep, 6, 1–9, https://doi.org/10.1038/srep35057, 2016.
Sweetman, A. K., Thurber, A. R., Smith, C. R., Levin, L. A., Mora, C., Wei, C.-L., Gooday, A. J., Jones, D. O. B., Rex, M., Yasuhara, M., Ingels, J., Ruhl, H. A., Frieder, C. A., Danovaro, R., Würzberg, L., Baco, A., Grupe, B. M., Pasulka, A., Meyer, K. S., Dunlop, K. M., Henry, L.-A., and Roberts, J. M.: Major impacts of climate change on deep-sea benthic ecosystems, Elem Sci Anth, 5, 4, https://doi.org/10.1525/elementa.203, 2017.
Tittensor, D. P., Baco, A. R., Brewin, P. E., Clark, M. R., Consalvey, M., Hall-Spencer, J., Rowden, A. A., Schlacher, T., Stocks, K. I., and Rogers, A. D.: Predicting global habitat suitability for stony corals on seamounts, J Biogeogr, 36, 1111–1128, https://doi.org/10.1111/j.1365-2699.2008.02062.x, 2009.
Vad, J., Kazanidis, G., Henry, L. A., Jones, D. O. B., Gates, A. R., and Roberts, J. M.: Environmental controls and anthropogenic impacts on deep-sea sponge grounds in the Faroe-Shetland Channel, NE Atlantic: The importance of considering spatial scale to distinguish drivers of change, ICES Journal of Marine Science, 77, 451–461, https://doi.org/10.1093/icesjms/fsz185, 2020.
Vandorpe, T., Wienberg, C., Hebbeln, D., Van den Berghe, M., Gaide, S., Wintersteller, P., and Van Rooij, D.: Multiple generations of buried cold-water coral mounds since the Early-Middle Pleistocene Transition in the Atlantic Moroccan Coral Province, southern Gulf of Cádiz, Palaeogeogr Palaeoclimatol Palaeoecol, 485, 293–304, https://doi.org/10.1016/j.palaeo.2017.06.021, 2017.
van der Kaaden, A., Maier, S. R., Siteur, K., De Clippele, L. H., van de Koppel, J., Purkis, S. J., Rietkerk, M., Soetaert, K., and van Oevelen, D.: Tiger reefs: Self-organized regular patterns in deep-sea cold-water coral reefs, Ecosphere, 14, 10, https://doi.org/10.1002/ecs2.4654, 2023.
van der Kaaden, A.-S. and De Clippele, L. H.: Image/Video Annotation and Analysis. Zenodo protocol and functions. https://doi.org/10.5281/zenodo.5547565, https://doi.org/10.5281/zenodo.5547565, 2021.
van der Kaaden, A.-S., van Oevelen, D., Rietkerk, M., Soetaert, K., and van de Koppel, J.: Spatial Self-Organization as a New Perspective on Cold-Water Coral Mound Development, Front. Mar. Sci., 7, 1–8, https://doi.org/10.3389/fmars.2020.00631, 2020.
van der Kaaden, A.-S., Mohn, C., Gerkema, T., Maier, S. R., de Froe, E., van de Koppel, J., Rietkerk, M., Soetaert, K., and van Oevelen, D.: Feedbacks between hydrodynamics and cold-water coral mound development, Deep-Sea Res. Pt. I, 178, 103641, https://doi.org/10.1016/j.dsr.2021.103641, 2021.
van der Kaaden, A.-S., Mohn, C., Gerkema, T., Maier, S. R., de Froe, E., van de Koppel, J., Rietkerk, M., Soetaert, K., and van Oevelen, D.: Corrigendum to “Feedbacks between hydrodynamics and cold-water coral mound development”, Deep-Sea Res. Pt. I,, 184, 103743, https://doi.org/10.1016/j.dsr.2022.103743, 2022.
van der Land, C., Eisele, M., Mienis, F., de Haas, H., Hebbeln, D., Reijmer, J. J. G., and van Weering, T. C. E.: Carbonate mound development in contrasting settings on the Irish margin, Deep-Sea Res. Pt. II, 99, 297–306, https://doi.org/10.1016/j.dsr2.2013.10.004, 2014.
Van Engeland, T., Rune Godø, O., Johnsen, E., Duineveld, G. C. A., and van Oevelen, D.: Cabled ocean observatory data reveal food supply mechanisms to a cold-water coral reef, Prog. Oceanogr., 172, 51–64, https://doi.org/10.1016/j.pocean.2019.01.007, 2019.
van Haren, H., Mienis, F., Duineveld, G. C. A., and Lavaleye, M. S. S.: High-resolution temperature observations of a trapped nonlinear diurnal tide influencing cold-water corals on the Logachev mounds, Prog. Oceanogr., 125, 16–25, https://doi.org/10.1016/j.pocean.2014.04.021, 2014.
van Oevelen, D., Duineveld, G., Lavaleye, M., Mienis, F., Soetaert, K., and Heip, C. H. R.: The cold-water coral community as a hot spot for carbon cycling on continental margins: A food-web analysis from Rockall Bank (Northeast Atlantic), Limnol. Oceanogr., 54, 1829–1844, https://doi.org/10.4319/lo.2009.54.6.1829, 2009.
van Oevelen, D., Duineveld, G. C. A., Lavaleye, M. S. S., Kutti, T., and Soetaert, K.: Trophic structure of cold-water coral communities revealed from the analysis of tissue isotopes and fatty acid composition, Mar. Biol. Res., 14, 287–306, https://doi.org/10.1080/17451000.2017.1398404, 2018.
Vertino, A., Spezzaferri, S., Rüggeberg, A. R., Stalder, C., and Wheeler, A. J.: An overview on cold-water coral ecosystems and facies, in: Cushman Foundation Species Publication No. 44, 12–19, 2014.
Wagner, H., Purser, A., Thomsen, L., Jesus, C. C., and Lundälv, T.: Particulate organic matter fluxes and hydrodynamics at the Tisler cold-water coral reef, J. Mar. Syst., 85, 19–29, https://doi.org/10.1016/j.jmarsys.2010.11.003, 2011.
Wang, H., Lo Iacono, C., Wienberg, C., Titschack, J., and Hebbeln, D.: Cold-water coral mounds in the southern Alboran Sea (western Mediterranean Sea): Internal waves as an important driver for mound formation since the last deglaciation, Mar. Geol., 412, 1–18, https://doi.org/10.1016/j.margeo.2019.02.007, 2019.
Wang, H., Titschack, J., Wienberg, C., Korpanty, C., and Hebbeln, D.: The Importance of Ecological Accommodation Space and Sediment Supply for Cold-Water Coral Mound Formation, a Case Study from the Western Mediterranean Sea, Front. Mar. Sci., 8, 1–14, https://doi.org/10.3389/fmars.2021.760909, 2021.
White, M. and Dorschel, B.: The importance of the permanent thermocline to the cold water coral carbonate mound distribution in the NE Atlantic, Earth Planet. Sc. Lett., 296, 395–402, https://doi.org/10.1016/j.epsl.2010.05.025, 2010.
White, M., Mohn, C., de Stigter, H., and Mottram, G.: Deep-water coral development as a function of hydrodynamics and surface productivity around the submarine banks of the Rockall Trough, NE Atlantic, in: Cold-water Corals and Ecosystems, edited by: Freiwald, A. and Roberts, J. M., 503–514, 2005.
White, M., Roberts, J. M., and van Weering, T.: Do bottom-intensified diurnal tidal currents shape the alignment of carbonate mounds in the NE Atlantic?, Geo-Mar. Lett., 27, 391–397, https://doi.org/10.1007/s00367-007-0060-8, 2007.
Wienberg, C., Frank, N., Mertens, K. N., Stuut, J. B., Marchant, M., Fietzke, J., Mienis, F., and Hebbeln, D.: Glacial cold-water coral growth in the Gulf of Cádiz: Implications of increased palaeo-productivity, Earth Planet. Sc. Lett., 298, 405–416, https://doi.org/10.1016/j.epsl.2010.08.017, 2010.
Wienberg, C., Titschack, J., Frank, N., De Pol-Holz, R., Fietzke, J., Eisele, M., Kremer, A., and Hebbeln, D.: Deglacial upslope shift of NE Atlantic intermediate waters controlled slope erosion and cold-water coral mound formation (Porcupine Seabight, Irish margin), Quat. Sci. Rev., 237, 106310, https://doi.org/10.1016/j.quascirev.2020.106310, 2020.
Wienberg, C., Krengel, T., Frank, N., Wang, H., Van Rooij, D., and Hebbeln, D.: Cold-water coral mounds in the western Mediterranean Sea New insights into their initiation and development since the Mid-Pleistocene in response to changes of African hydroclimate, Quat. Sci. Rev., 293, 107723, https://doi.org/10.1016/j.quascirev.2022.107723, 2022.
Yahel, R., Yahel, G., and Genin, A.: Near- bottom depletion of zooplankton over coral reefs: I: Diurnal dynamics and size distribution, Coral Reefs, 24, 75–85, https://doi.org/10.1007/s00338-004-0449-z, 2005.
Zuur, A. F., Ieno, E. N., and Elphick, C. S.: A protocol for data exploration to avoid common statistical problems, Methods Ecol. Evol., 1, 3–14, https://doi.org/10.1111/j.2041-210x.2009.00001.x, 2010.