the Creative Commons Attribution 4.0 License.
the Creative Commons Attribution 4.0 License.
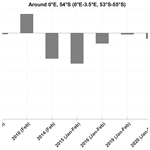
Anomalous summertime CO2 sink in the subpolar Southern Ocean promoted by early 2021 sea ice retreat
Kirtana Naëck
Jacqueline Boutin
Sebastiaan Swart
Marcel du Plessis
Liliane Merlivat
Laurence Beaumont
Antonio Lourenco
Francesco d'Ovidio
Louise Rousselet
Brian Ward
Jean-Baptiste Sallée
The physical and biogeochemical processes governing the air–sea CO2 flux in the Southern Ocean are still widely debated. The Southern Ocean Carbon and Heat Impact on Climate cruise in summer 2022 aimed at studying these processes in the Weddell Sea and in its vicinity. A CARbon Interface OCean Atmosphere (CARIOCA) drifting buoy was deployed in January 2022 in the subpolar Southern Ocean, providing hourly surface ocean observations of fCO2 (fugacity of CO2), dissolved oxygen, salinity, temperature, and chlorophyll a fluorescence for 17 months. An underwater glider was piloted with the buoy for the first 6 weeks of the deployment to provide vertical ocean profiles of hydrography and biogeochemistry. These datasets reveal an anomalously strong ocean carbon sink for over 2 months, occurring in the region of Bouvet Island and associated with large plumes of chlorophyll a (Chl a). Based on Lagrangian backward trajectories reconstructed using various surface currents fields, we identified that the water mass reaching the Bouvet Island region originated from the southwest, from the vicinity of the sea ice edge in spring 2021. A strong phytoplankton bloom developed there in November 2021. We propose that it was promoted by early sea ice retreat in 2021 in the Weddell Sea. These waters, depleted in carbon, then traveled to the position of the CARIOCA buoy. The very low values of ocean fCO2, measured by the buoy (down to 310 µatm), are consistent with net community production previously observed during blooms occurring near the sea ice edge, partly compensated by air–sea CO2 flux along the water mass trajectory. Early sea ice retreat might therefore have caused a large CO2 sink farther north than usual in summer 2022, in the Atlantic sector of the subpolar Southern Ocean. Such events might become more frequent in the future as a result of climate change.
- Article
(5922 KB) - Full-text XML
-
Supplement
(772 KB) - BibTeX
- EndNote
According to the latest Global Carbon Budget, atmospheric CO2 concentrations keep increasing and are projected to be 51 % higher than pre-industrial levels in 2023. The ocean helps mitigate the atmospheric CO2 increase by acting as a carbon sink. Indeed, during the past decade (2013–2022), the global ocean absorbed 2.9 ± 0.4 Gt C yr−1, representing 26 % of worldwide CO2 emissions annually (Friedlingstein et al., 2023). The Southern Ocean plays an important role in the ocean's buffering capacity. Defined as the ocean surrounding Antarctica, south of 30–35° S, it covers only about 20 %–30 % of the global ocean surface yet serves as a main pathway for anthropogenic carbon uptake (Frölicher et al., 2015; Gruber et al., 2019). It is, in fact, responsible for about 40 % of the global oceanic uptake of anthropogenic CO2 (DeVries, 2014; Frölicher et al., 2015; Gruber et al., 2019; Mayot et al., 2023).
The direction of the air–sea CO2 flux is determined by the difference between the fugacity of CO2 at the ocean surface, fCO2, and in the atmosphere, fCO2atm. When the fCO2 is undersaturated with respect to the atmosphere, the ocean is a carbon sink (Wanninkhof, 2014). Any process that affects fCO2 at local or regional scale, like the biological activity or the ocean circulation, can therefore affect the ocean carbon pump (Henley et al., 2020). Ultimately, it is the balance between the biological pump, the solubility pump, and ocean circulation which will determine whether the surface ocean will behave as a sink or a source of carbon to the atmosphere.
The lack of fCO2 observations have posed a daunting challenge to the ocean community to understand the full suite of mechanisms controlling the air–sea CO2 flux and to assess its net magnitude. This is particularly true in the Southern Ocean, which is renowned to be pivotal in controlling global air–sea CO2 flux but is also one of the regions suffering the most from fCO2 observation scarcity. As a result, there are large discrepancies in the Southern Ocean carbon sink estimates from the literature (sink south of 30° S derived from fCO2 products: 1.6 [1.3, 1.7] Gt C yr−1; inversion estimates: 1.5 [1.3, 1.9] Gt C yr−1; global ocean biogeochemical models (GOBMs): 1.4 ± 0.3 Gt C yr−1), which result in large uncertainties in our global estimates of air–sea CO2 flux (Friedlingstein et al., 2023).
The difficulty of assessing CO2 uptake by the Southern Ocean is exacerbated by the fact that the Southern Ocean carbon sink is much more variable than previously thought. This basin has a large interdecadal variability, and the observation-based products, such as those derived from SOCAT (Bakker et al., 2016) and GOBMs, have shown different interannual trends for the past years. Present-day fCO2 products may overestimate the decadal variability of the Southern Ocean carbon sink by 50 %–130 % due to data sparsity (Hauck et al., 2023). When derived from the fCO2 product, mean decadal amplitude is 6.31 Tmol yr−1 and mean interannual amplitude is 1.68 Tmol yr−1. On the other hand, when derived from the GOBMs, both mean decadal amplitude and mean interannual amplitude are 2.1 Tmol yr−1 (Mayot et al., 2023). In this regard, a better understanding of the different processes governing the air–sea flux of CO2 is essential to anticipate the effects of climate change on the Southern Ocean's capacity to continue sequestering carbon (Hauck et al., 2023; Mayot et al., 2023; Meijers et al., 2023). Indeed, although the carbon sink significantly strengthened since the early 2000s, a shift in climate trends could cause the Southern Ocean to lose its newly regained capacity for carbon uptake (Landschützer et al., 2015). To better monitor carbon-related properties, there has been an increased deployment of BGC-ARGO floats via the SOCCOM project (Sarmiento et al., 2023) and recent studies using uncrewed surface vehicles (Nicholson et al., 2022; Sutton et al., 2021).
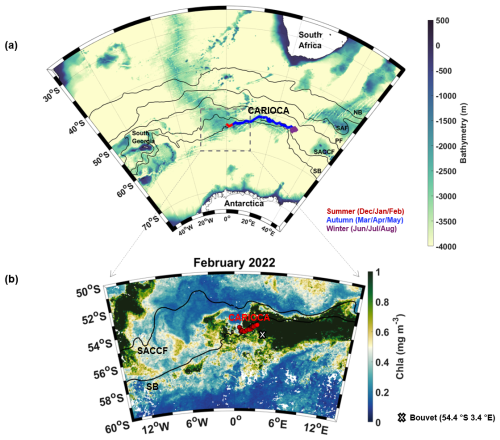
Figure 1(a) CARIOCA buoy trajectory from 26 January 2022 to 27 June 2022 superimposed on a bathymetry map of the study zone. Fronts from Park et al. (2019) are indicated, from north to south: Northern Boundary (NB) of the ACC, Subantarctic Front (SAF), Polar Front (PF), Southern Antarctic Circumpolar Current Front (SACCF), and Southern Boundary (SB) of the ACC. (b) CCI Chl a map with CARIOCA buoy trajectory in February.
The SO-CHIC (Southern Ocean Carbon and Heat Impact on Climate) European program was launched to tackle this knowledge gap (The SO-CHIC consortium et al., 2023). In this context, during the S. A. Agulhas II summer research cruise in January 2022 (Ward et al., 2022), a CARIOCA (CARbon Interface OCean Atmosphere) buoy was deployed near the Southern Boundary, northeast of the Weddell Sea, and west of Bouvet Island. At the same time, an underwater ocean glider (SG675) alongside deep CTD sections was deployed. The glider followed the buoy for 6 weeks to provide observations of the underlying ocean conditions. The CARIOCA buoy acquired fCO2 measurements in the subpolar region (Fig. 1) where surface fCO2 observations have been sparse in the past 20 years (Gruber et al., 2019).
In this paper, we use these unique field observations to investigate the cause of fCO2 variations along the trajectory of the buoy, with a particular focus on the role of biological activity in shaping these variations. The Southern Ocean is a high-nutrient, low-chlorophyll (HNLC) region, with iron as the key limiting nutrient for phytoplankton growth. Sources of iron to the ocean mixed layer therefore condition the efficiency of the biological carbon pump (Ardyna et al., 2019; Blain et al., 2008; Boyd et al., 2007). Iron can come from island and/or plateau sources, ocean ridge sources, Fe-rich entrained waters (Tagliabue et al., 2014), or from a sea ice source (Ardyna et al., 2019). In summer 2022, west and northeast of Bouvet Island (54.4° S, 3.4° E; Fig. 1), an unusually large CO2 sink was observed along the path of the buoy, as well as high biological activity. This study aims to investigate the processes that contributed to this large summer 2022 CO2 sink.
2.1 CARIOCA measurements
The CARIOCA buoy was deployed on 23 January 2022 at 54° S, 0° W. It has a floating anchor, following the currents at 15 m depth, in a quasi-Lagrangian way. A three-wavelength spectrophotometer (434, 596, and 830 nm) was used to measure fCO2. The fCO2 sensor included an exchanger where a dye solution (thymol blue) was brought into equilibrium with seawater via a semi-permeable CO2 membrane. The absorption coefficient of the dye was measured by the spectrophotometer and was then related to the carbonate properties of seawater. The three-wavelength measurements enable correction of any modification of the optical path or of the opacity of the optical cell (Copin-Montégut et al., 2004). The accuracy of the fCO2 is estimated ± 3 µatm, and its precision is ± 1 µatm (Boutin et al., 2008). A thermosalinograph measured the sea surface temperature at 2 m depth, SST, and the conductivity, from which sea surface salinity, SSS, was derived. An optode measured dissolved oxygen (O2). Calibrated values were given by the manufacturer for pure water, and they were corrected from the effects of temperature and salinity as described in Merlivat et al. (2015). A fluorometer measured the fluorescence which was calibrated in Chl a units using the calibrated Seaglider (SG675) Chl a (see Sect. 2.3). An anemometer measured wind speed, and a barometer measured atmospheric pressure (Patm) at 2 m above the sea surface. The wind at 10 m above the ocean was derived assuming a neutral atmosphere. As from 4 June 2022, the CARIOCA atmospheric sensor stopped working, and the wind and atmospheric pressure data were replaced with data from ERA5. During their common period, CARIOCA data and colocated ERA5 data were very similar (mean difference = 0.26 m s−1 and R2 = 0.86, not shown). The CARIOCA measurements, sensors, and accuracy are summarized in Table 1. The buoy acquired 17 months of data, which were transmitted in real time via ARGOS and stopped functioning on 24 June 2023. In this study, we only use observations from 26 January 2022 to 27 June 2022, the period during which a strong undersaturation of ocean fCO2 with respect to the atmosphere was observed in January–March and gradually declined until June.
2.2 CARIOCA derived parameters
The air–sea flux of CO2 represents the exchanges of CO2 at the ocean–atmosphere interface. It was calculated using a bulk formula as
where fCO2ssw and fCO2atm are the fugacity of CO2 at the ocean surface and in the atmosphere and K is the CO2 exchange coefficient (Wanninkhof, 1992, 2014). The fCO2atm was determined using atmospheric CO2 concentration data (xCO2atm) from SPO (South Pole Antarctica) and CARIOCA Patm according to Eq. (2) in Boutin et al. (2008).
To eliminate fCO2 changes linked to temperature and salinity, the concentration of dissolved inorganic carbon (DIC) was estimated. At given SST and SSS, the DIC and alkalinity were estimated from fCO2. The alkalinity was calculated according to Lee et al. (2006). The MATLAB routine CO2SYS, with dissociation constants K1 and K2 of Lueker et al. (2000), was used to derive DIC. Oxygen saturation (O2sat), the degree of oxygen saturation (pO2sat), and the oxygen flux at the air–sea interface (FO2) were calculated as per Merlivat et al. (2015). The oxygen anomaly was calculated by subtracting the dissolved oxygen concentration calculated at saturation from the dissolved oxygen concentration (O2 − O2sat). During photosynthesis, a positive anomaly is expected, whereas during respiration and/or remineralization, a negative anomaly is expected. The oxygen measurements of the CARIOCA drifter could not be recalibrated at sea, because unfortunately the O2 measured by the CTD at the deployment was not calibrated. An empirical correction of +8 µmol kg−1 was applied to bring the values of O2 − O2sat above zero during periods of high biological activity. These high biological periods are detected from CARIOCA fluorescence and opposite variations in O2 and DIC. The carbon net community production, NCPc, and the oxygen net community production, NCPo2, were estimated during periods of high biological activity, during which diurnal cycles with DIC and O2 − O2sat in opposition were observed by the CARIOCA buoy. FO2 was used when estimating the NCPo2, following the same methodology as in Merlivat et al. (2015), but using the mixing layer depth as in Merlivat et al. (2022) and Pellichero et al. (2020) (see Sect. 2.3).
2.3 Seaglider measurements and derived parameters
A Kongsberg Seaglider (SG675) was deployed alongside the CARIOCA buoy. A Seaglider is an autonomous underwater vehicle designed to fly through the water column from the sea surface to 1000 m depth following a sawtooth pattern, moving vertically and horizontally at nominal speeds of 0.1 and 0.3 m s−1, respectively. Upon surfacing roughly every 6 h, Seagliders communicate with the base station via Iridium, thereby transferring data in near-real time. A key characteristic of Seagliders is their ability to be piloted from land, allowing researchers to control the direction of sampling. For this experiment, SG675 followed the CARIOCA buoy for a month and a half, from 31 January 2022 to 10 March 2022 (39 d) (Fig. 1), providing vertical profiles of temperature, salinity, oxygen, and fluorescence of the upper 1000 m of the water column. There was a time lapse of about 1 d between the CARIOCA buoy and the glider, with the glider being about 1 d behind the buoy each time. For this study, only profiles between 31 January and 10 March 2022 were considered, which was when the glider and the CARIOCA buoy were nearest to each other (less than 20 km apart). The glider data were processed using the GliderTools package (Gregor et al., 2019). For the salinity data, outliers and spikes were removed and a smoothing was applied with a Savitzky–Golay filter. The glider salinity data were also validated using the CTD salinity profile (on 23 January 2022 at 14:46 GMT). The CTD salinity data had already been calibrated, and the CARIOCA SSS data were in turn validated using the already validated glider surface salinity data. The glider salinity at 2 m and the CARIOCA SSS (at 2 m) were in good agreement, with a small difference of about 0.05 pss (practical salinity scale). Using GliderTools (Gregor et al., 2019), the glider fluorescence profiles were also quality-controlled. Outliers, spikes, and bad profiles were removed, and the data were corrected for in situ dark counts. A quenching correction using the same method as Thomalla et al. (2018) was applied. The glider fluorescence was calibrated and converted to chlorophyll a using the chlorophyll a CTD water samples less than 24 h after deployment.
The mixed layer depth, MLD, was estimated from the glider data using the density threshold of 0.03 kg m−3 (de Boyer Montégut et al., 2004). It has been observed in past studies that DIC can be more sensitive to the mixing layer, XLD, which can be determined using turbulence measurements (Nicholson et al., 2022; Pellichero et al., 2020; Sutherland et al., 2014; Giunta and Ward 2022). The empiric relationship approximated by Nicholson et al. (2022) was used to estimate XLD. (The MLD and the XLD are surface layers directly influenced by the atmosphere. The MLD has homogenized, formed as a result of mixing, while the XLD is still actively influenced by turbulence and still mixing.)
2.4 S. A. Agulhas II TSG
The S. A. Agulhas II was equipped with a thermosalinograph (TSG), and ship measurements of salinity and temperature were collected, from South Africa to Antarctica and back again, from 3 December 2021 to 28 January 2022. The S. A. Agulhas II TSG SSS data were calibrated using underway water samples collected during the SO-CHIC cruise (Ward et al., 2022).
2.5 Satellite, analysis, and reanalysis datasets
Chlorophyll a satellite images are from the Climate Change Initiative product version 6.0 (Sathyendranath et al., 2023), from 1997 to 2022, for spring (November: 1997–2021) and summer (January, February: 1998–2022). Monthly and weekly means were used for the Chl a. For the surface salinity data, Glorys reanalysis (Global Ocean Physics Reanalysis, 2024), Mercator analysis (CMEMS and MDS, 2024a) at 5 m depth, and SMOS CATDS CECv9.0 (18 d) satellite data (Boutin et al., 2024) were used. Daily and monthly data from Mercator analysis were used as of July 2021, and Glorys reanalysis was used for the years before July 2021. A colocalization between Mercator salinity data and CARIOCA SSS indicates that both datasets were in good agreement, showing similar patterns, but with Mercator salinity data being lower than the CARIOCA salinity by about 0.1 pss from February 2022 to mid-April 2022 (Fig. A1 in Appendix A).
The Mercator and/or Glorys products were also used to analyze vertical salinity (not shown) and surface current velocities. Sea ice data were obtained from OSI SAF (EUMETSAT: Product Navigator – Global Sea Ice Concentration Climate Data Record v3.0 – OSI SAF, 2022a; EUMETSAT: Product Navigator – Global Sea Ice Concentration Interim Climate Data Record Release 3 – OSI SAF, 2022b).
2.6 Lagrangian analysis
Backward trajectories of water masses were computed with a Lagrangian analysis (Sergi et al., 2020) using the LAMTA software (Rousselet et al., 2024). After initializing the particles at specific dates, longitudes, and latitudes, a backward advection was performed to estimate the trajectories of these particles several months before. To determine which water masses reached the CARIOCA buoy, particles were initialized at the CARIOCA buoy coordinates, with one coordinate point per hour (i.e., 24 points advected backwards in time each day). Three current products were tested, namely, GlobCurrent (CMEMS and MDS, 2024b), Mercator Analysis (CMEMS and MDS, 2024a) and/or Glorys reanalysis (Global Ocean Physics Reanalysis, 2024), and OSCAR (ESR, 2009) (Fig. S1 in the Supplement). Using Mercator analysis and/or Glorys reanalysis, different depths were also tested, namely 5, 15, 34, and 55 m. Using OSCAR, the backward trajectories in November, January, and February were also computed, from 1997 to 2022. In the next sections, we are showing the results obtained using the total currents, i.e., the sum of the geostrophic and Ekman currents, at 15 m depth. The reliability of numerical trajectories for reconstructing phytoplanktonic blooms from nutrient sources and up to mesoscale precision has been validated in the Southern Ocean from multi satellite data, surface drifters, and lithogenic isotopes (Sergi et al., 2020; d'Ovidio et al., 2015; Sanial et al., 2014).
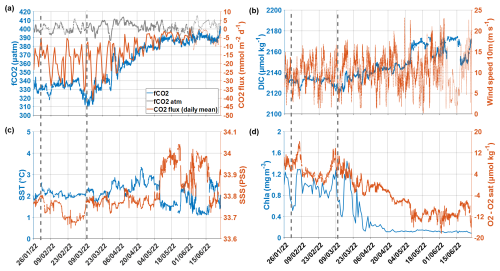
Figure 2CARIOCA measurements time series from 26 January 2022 to 27 June 2022: (a) Atmospheric and surface ocean fCO2 (fCO2atm and fCO2) and daily mean of CO2 flux, (b) DIC and wind speed, (c) SST and SSS, and (d) Chl a and O2 − O2sat, with the period during which the glider followed the buoy (31 January to 10 March 2022) indicated by dotted gray lines. The thinner dotted lines show the data derived from ERA5 (fCO2atm and CO2 flux derived from ERA5 Patm, as well as ERA5 wind, as from 4 June 2022). The whole time series from 26 January 2022 to 24 June 2023 is shown on Fig. S2 in the Supplement.
3.1 CARIOCA buoy and Seaglider observations
Low surface salinities were measured in February 2022, both by the CARIOCA buoy (Fig. 2c) and by the glider (Fig. 3a), with minimum values of salinity in the second half of February. The CARIOCA buoy measured surface salinities around 33.7 pss between 17 February and 8 March 2022. Both the glider and the CARIOCA drifter observed maxima of surface Chl a around 7 February and 7 March 2022 (Figs. 2d and 3b). According to the glider profiles, the low values of salinity and high concentrations of Chl a, observed from early February to early March, were well mixed within the mixed layer, confined to the top 60 m or so (Fig. 3). According to Fig. 2a, a very low fCO2 was observed by the CARIOCA buoy in summer 2022, while the buoy was in this fresh water mass, enriched in Chl a and oxygen. The dfCO2 is about −60 µatm from the end of January to the end of March 2022. Minima of fCO2 (∼ 320 µatm) and of DIC (∼ 2120 µmol kg−1) occurred on 7 February and 7 March 2022, which also coincided with maximum concentrations of chlorophyll a (∼ 1.3 mg m−3) and of oxygen. Around these dates, diurnal cycles of DIC and O2 − O2sat, with the DIC and O2 − O2sat in opposite phases, were clearly observed and allowed NCP estimates (see next section). According to CARIOCA measurements (Fig. 2a), after March, as the CARIOCA buoy drifted away from the phytoplankton bloom, fCO2 started increasing, until it reached values close to equilibrium with the atmosphere on 27 June 2022. The CARIOCA derived air–sea CO2 flux averaged to −18.8 from January to March 2022, and after March it started increasing until it reached values close to zero in June 2022.
3.2 NCP and impact of wind on XLD
During the two periods dominated by biological activity, on 5–7 February 2022 and on 4–7 March 2022, Chl a increased by 0.5 mg m−3 and DIC decreased by 10 µmol kg−1 (Figs. 2 and B1 in Appendix B). Just before the peaks of Chl a (corresponding to minimum concentrations of DIC and maximum concentrations of oxygen), there were very low wind speeds, associated with a shoaling of the mixed and mixing layers (Figs. 2 and B1), which itself might have enhanced Chl a bloom at the surface (Fig. 3b). During the February event, NCPc was estimated to reach −91 , while NCPo2 was 132 . During the March event, NCPc of −104 and NCPo2 of 138 were estimated (Fig. B1 in Appendix B). It is interesting to note that the two values of the photosynthetic quotient, PQNCPo2/NCPc, respectively equal to 1.45 and 1.33, are in close good agreement with the value of 1.4 expected in a new production regime (Laws, 1991).
After 7 February and 15 March 2022, there was a strengthening of wind speeds and a deepening of the XLD, probably entraining waters rich in DIC from the subsurface layer. Indeed, the CARIOCA derived DIC was higher at the same time as higher wind speeds and lower concentrations of O2 − O2sat and of Chl a (Figs. 2 and B1). The DIC and O2 changes derived from the CARIOCA measurements are also consistent with the orders of magnitude that could be estimated, using GLODAP climatological DIC and O2 profiles (not shown), considering the change in XLD. This might suggest that mixing events driven by high winds sometimes compensated for the CO2 undersaturation driven by biological activity.
However, the mixing events shown here are only on synoptic timescales. For instance, after 10 February 2022, the wind decreased again and DIC concentrations stopped increasing (Fig. B1a). In fact, outside the brief periods of local biological production and of mixing events, the DIC and the fCO2 remained relatively stable at a low value from January to March. For instance, between 11 February 2022 and 3 March 2022, the DIC remained at a mean value of 2133 µmol kg−1 (Fig. 2).
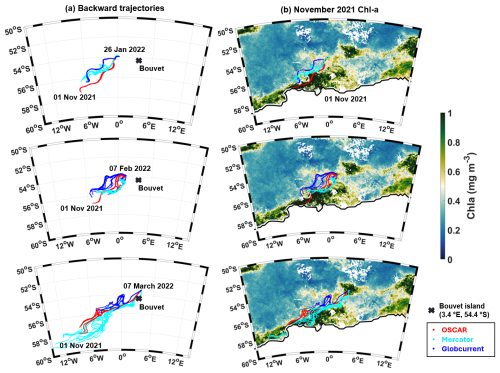
Figure 4(a) Backward trajectories from the CARIOCA buoy's location in January (top), February (middle), and March (bottom) 2022 to November 2021, using different current products (Mercator analysis, OSCAR, GlobCurrent). (b) CCI Chl a in November 2021, with the sea ice concentration at 10 %, on 1 November 2021, and backward trajectories superimposed.
3.3 Origin of fresh water mass and phytoplankton bloom
In summer 2022, the CARIOCA buoy and glider were found in productive waters of low salinity (Figs. 2 and 3). The presence of this low-salinity upper ocean was also confirmed by the S. A. Agulhas II thermosalinograph SSS observations. Moreover, SSS images from the SMOS satellite mission, as well as salinity estimates at 5 m from an ocean analysis (Mercator), are in agreement with the in situ measurements and show low salinities in that region in summer 2022 (Fig. C1 in Appendix C). This surface fresh layer suggests a stratification of water masses, which might have favored the sustained growth of phytoplankton in a shallow mixed layer, where more light was available.
To investigate the origin of these waters, we computed backward trajectories of virtual particles that were released at the CARIOCA buoy location. These particles were advected backward in a suite of different velocity field estimates (see Methods). According to salinity maps from Mercator (not shown) and to backward trajectories spanning several months before the 2022 deployment of the instruments, the fresh water mass in which the CARIOCA buoy and glider drifted was advected from a region southwest of their position, close to the sea ice edge, in the Weddell Sea (Fig. 4).
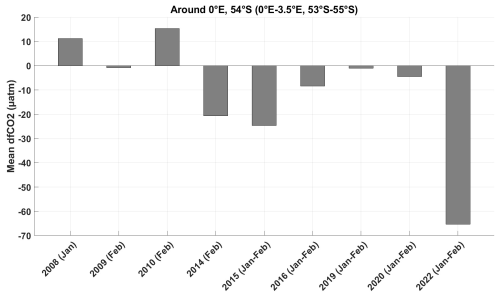
Figure 5Histogram of dfCO2 in January and February, estimated using all SOCAT data available between 53–55° S and 0–3.5° E, from 2000 to 2022. The summer 2019 data are from a Wave Glider (Nicholson et al., 2022).
On Fig. 4, backward trajectories, computed using currents from OSCAR, Mercator, and GlobCurrent, show that waters found at the CARIOCA buoy location on 26 January 2022 (54° S, 0.2° E) and on 7 February 2022 (54° S, 0.8° E) came from a region around 55.5–57° S and 4–6° W, near the sea ice edge, on 1 November 2021, where surface salinity decreased and a large phytoplankton bloom (high Chl a) occurred. The waters reaching the CARIOCA drifter on 7 March 2022 (53.4° S, 3.7° E) originated from a region even closer to the sea ice edge, at around 56–59° S and 5–12° W, on 1 November 2021, and then passed near Bouvet Island before reaching the buoy. These correspond to an export of waters from the Weddell Sea, south of 55° S (Vernet et al., 2019), which are possibly enriched in dissolved iron originating from sea ice melt (Lannuzel et al., 2016; Person et al., 2021).
3.4 Comparison between summer 2022 and previous campaigns in summer
We compare dfCO2 observed during previous campaigns using SOCAT and another available dataset (Nicholson et al., 2022) from 2000 to 2022 for the same region and season as in our case study (Fig. 5).
There was a mean outgassing in summers 2008 and 2010. In these years there was no local bloom observed on CCI Chl a maps, and we checked with backward trajectories that the waters reaching 54° S, 0° E did not come from a spring bloom near the ice edge either. On the other hand, in 2014, 2015, 2016, and 2020, an undersaturation smaller than the 2022 one was observed in summer (January–February) even though there was no local bloom. The waters reaching the region in these years, as identified from backward trajectories, came mostly from a bloom near the sea ice edge in November (except in February 2014, when the waters came from a bloom occurring in December; in 2016 we could not check due to missing CCI Chl a data). In 2009 and 2019 the ocean fCO2 was close to equilibrium with the atmosphere and there was no local bloom. In November 2008, a bloom occurred near the ice edge. However, backward trajectory analysis indicates that the waters reaching the region in February 2009 did not originate directly from the bloom but instead came from areas with lower Chl a concentrations. The situation in 2019 was comparable. Although there was no bloom in November (2018), a bloom occurred in December (Fig. D1) near the ice edge. However, in the following months, these waters were not advected far enough north and did not reach 54° S, 0° E. Summer 2022 was the summer with the largest negative dfCO2 around 54° S, 0° E.
The comparison was deepened in summer 2019 (see Appendix D), as this was the year in which the most data from a Wave Glider (Nicholson et al., 2022) and from a ship campaign (Norwegian RV Kronprins Haakon cruise) (Ogundare et al., 2021) were available at the same position and same dates as our case study. It showed that in summer 2022, the fresh water mass and phytoplankton bloom were farther north than in summer 2019 (Figs. D1 and D2).
In summer 2022, the CARIOCA buoy measured an unusually large fCO2 undersaturation near the Southern Boundary and Bouvet Island, east of 54° S, 0° E. The CARIOCA CO2 flux integrated over the studied period (26 January 2022–27 June 2022) is −1.88 mol m−2, which, assuming negligible flux the rest of the year, is considerably greater than the climatological values in that region. Actually, according to Gruber et al. (2019; their Fig. 2b–d), climatologies of yearly air–sea CO2 fluxes are close to 0 mol C m−2 in the region sampled by the CARIOCA drifter.
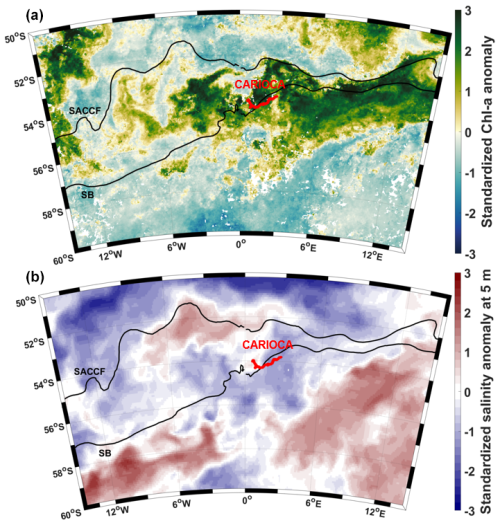
Figure 6Anomalies in February 2022, relative to a 2011–2022 climatology, normalized with the standard deviation of monthly values. (a) Chl a and (b) salinity at 5 m using Mercator analysis. CARIOCA trajectory in February (red).
A comparison to measurements conducted on other summers indicates that summer 2022 was the most undersaturated since 2008 around 54° S, 0° E (see Sect. 3.4). At that time, the CARIOCA buoy crossed a large phytoplankton bloom (Chl a > 1 mg m−3) (Fig. 1b). Sergi et al. (2020) showed that moderate bloom can develop around Bouvet Island by a combination of fertilization from the island itself and from the proximity of seamounts acting as hydrothermal sources of iron. However, the bloom observed in summer 2022 was the largest bloom observed over the past 12 years (Fig. 6) (and over the last 25 years, not shown) – as large as those typically observed on the Kerguelen Plateau (Blain et al., 2007). Moreover, fCO2 and DIC remain rather stable between January and March, and local biological carbon production appeared to be compensated by entrainment of high DIC into the mixed layer during synoptic mixing events (Nicholson et al., 2022). We thus hypothesize that another process was at play. Indeed, the Lagrangian backward trajectories from the January–February 2022 CARIOCA buoy location are not consistent with an origin from Bouvet Island or from seamounts, in contrast with the case in Sergi et al. (2020).
Salinity in summer 2022 near the CARIOCA buoy was anomalously fresh (Fig. 6), although there was no significant rainfall during that period at the position of the fresh water mass (not shown). Both salinity maps from Mercator (not shown) and backward trajectories enable us to track the travel of this fresh water mass from its formation near the ice edge in spring 2021 to the position of the CARIOCA buoy and glider in summer 2022 (Fig. 4). In 2021, the ice retreated abnormally early from September to mid-November 2021, anomalous northeastward motion of sea ice was observed in the northeast Weddell Sea (Wang et al., 2022, their Figs. 3 and 4), and the austral summer 2022 marked a record for the lowest sea ice extent in Antarctica (Wang et al., 2022).
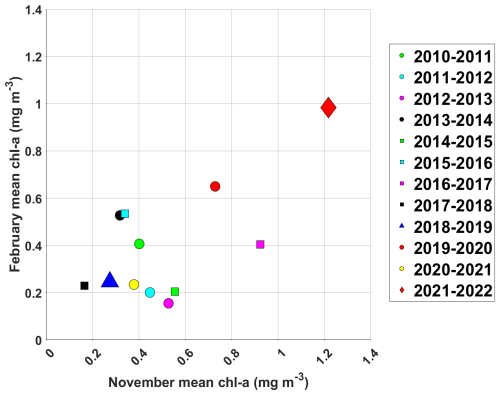
Figure 7Mean Chl a along the CARIOCA buoy position in February plotted against the mean Chl a along the backward trajectories (computed using OSCAR currents) in November for each year from 2010 to 2022.
4.1 Impact of anomalous sea ice retreat on phytoplankton blooms
Previous studies have shown that the melting of sea ice can fertilize the region near the sea ice edge and stratify the water masses (Briggs et al., 2018; McClish and Bushinsky, 2023). Other factors such as light availability, grazing pressure, and phytoplankton community composition should also be considered (Smith and Comiso, 2008; Briggs et al., 2018; Mc Clish and Bushinsky, 2023), and it has been shown that the date of sea ice breakup conditions the onset and magnitude of ice edge blooms (McClish and Bushinsky., 2023). We can suppose that the early sea ice retreat promoted the development of a phytoplankton bloom at the sea ice edge in spring 2021 (Fig. 4) (Wang et al., 2022). Model results of Death et al. (2014) also suggested that subglacial meltwaters may constitute a significant additional source of bioavailable iron to the Southern Ocean, supplementing iceberg sources, and that the impact of the glacial iron flux may be large, extending across much of the Southern Ocean due to the redistribution of the iron by ocean circulation.
We hypothesize that phytoplankton bloom near CARIOCA trajectory in January–February 2022 was favored by stratified, fresher waters (Figs. 3a, 6, and D2) and micronutrients supplied by ice melt and redistributed by ocean circulation. This is supported by fresh and enriched Chl a water that detached from the sea ice edge region around 4° W in November 2021 and migrated northwestward (Fig. 4). This leads to the presence, in December 2021, of a patch of moderate Chl a around 57° S, 6° W (Fig. D1) along the Lagrangian backward trajectory (Fig. 4). Then, this patch migrates northeastward and reaches the CARIOCA buoy in January 2022, with Chl a slightly higher than in December, possibly because of additional fertilization by nearby seamounts or because of the presence of different phytoplankton community composition. In March 2022, the waters reaching the CARIOCA buoy originated from farther south, closer to the ice (Fig. 4), before reaching the CARIOCA buoy (Fig. 4). Hence these waters might have been even more enriched in micronutrients related to ice melt.
4.2 Interannual variation
The backward trajectories show that waters near 54° S, 0° E in February generally came from the southwest in the previous years as well (not shown). We therefore investigate a possible link between the intensity of the bloom at the extremities of the Lagrangian backward trajectory between November and February, from 2010 to 2022, to study a possible link between spring blooms near the sea ice edge and summer blooms near Bouvet Island.
In spring 2021–summer 2022, the blooms at both extremities of the backward trajectories were much more pronounced than usual, with Chl a concentrations above 1 mg m−3 both in November 2021 (along the backward trajectory) and in February 2022 (along the CARIOCA trajectory) (Fig. 7).
This suggests that a strong spring bloom near the sea ice edge could promote the development of a strong summer bloom farther north. Following the same logic, when low concentrations of Chl a were observed in November, similarly low concentrations of Chl a were usually observed in February (see for instance 2018–2019, Fig. 7).
4.3 Interpretation of the low CARIOCA fCO2 measurements and derived DIC
During sea ice retreat, large net community production occurs in the seasonal ice zone (SIZ), likely due to iron delivery or low grazing rates (McClish and Bushinsky, 2023). Moreover, these authors found, based on BGC Argo floats observations, that the bloom NCP increases in the case of early sea ice retreat. Given that the water mass reaching the CARIOCA buoy's location in the subpolar region remained in the bloom near the sea ice edge from September to November 2021, i.e., over the whole bloom period, it seems reasonable to consider that the DIC of this water mass was affected by a bloom NCP typical of early ice retreat of 3 mol C m−2 (Fig. 4 of McClish and Bushinsky, 2023), which would correspond to a fCO2 as low as 240 µatm (Appendix E). Assuming that from the end of November 2021 to January 2022 the fCO2 change along the water mass trajectory was only affected by air–sea exchange and temperature change, we estimate a fCO2 of 342 µatm at the end of January 2022 (see details in Appendix E), a value very close to the one observed by the CARIOCA sensor (Fig. 2a). In this crude estimation, we neglect biological production along the water mass trajectory and mixing with subsurface water. The latter seems at first order reasonable given that the salinity anomaly persisted along the water mass trajectory until January 2022. For what concerns the biological activity, the Chl a was much lower along the water mass trajectory in December 2021–January 2022 than in the vicinity of the sea ice edge in spring 2021, suggesting low NCP. Moreover, the DIC derived from CARIOCA observations in January–February 2022 remained relatively stable (Fig. 2) at a low value, suggesting a balance between biological production and other processes, such as subsurface mixing or consumption by grazers. Our hypothesis is that the very low DIC and fCO2 concentrations and highly negative CO2 fluxes deduced by the CARIOCA measurements are not due to the local summer bloom in itself but rather to a fresh water mass, already depleted in carbon, advected from the ice edge in November 2021 to the position of the buoy in summer 2022.
4.4 Study limits and perspectives
Another possible source of iron fertilization could come from the melting of icebergs (Person et al., 2019). An analysis of satellite images enables us to rule out the hypothesis of iceberg melting in the vicinity of the CARIOCA drifter or of water fertilized by the melting of the supergiant iceberg A68A (Smith and Bigg, 2023) that happened near southern Georgia in fall 2021 being the cause of the bloom. Although there were some iceberg fragments present near the CARIOCA buoy after its deployment, in January 2022 (Fig. S3 in the Supplement), the large size of the fresh water mass coming from the southwest points towards an export of fresh waters from the Weddell Sea.
Our study was focused only on the region surrounding Bouvet Island, from 50 to 60° S and 15° W to 15° E. We could envision further studies on the impact of sea ice retreat at latitudes farther north in the whole Southern Ocean and not just in the Atlantic sector. The year 2022 was a record for lowest sea ice extent in austral summer (Wang et al., 2022). We could hypothesize that these fresh waters, already depleted in carbon, traveled farther north than usual due to this unprecedented low-sea-ice-extent record. It has been shown that due to global warming, there is a tendency for more sea ice retreat in the Southern Ocean in the future (Wang et al., 2022) and for an increased northward fresh water transport (Haumann et al., 2016). This could lead to stronger undersaturation of fCO2 and more negative CO2 fluxes in regions usually in equilibrium with the atmosphere in austral summer. Anomalously large phytoplankton blooms at higher “northern” latitudes could also lead to a shift in the hunting zones of predators and might therefore have an impact on the ecosystem in the long term. Studies have shown that due to higher emissions and a warmer climate, there might be a change in the future location, mechanism, and seasonality of the carbon sink in the Southern Ocean, with an increase of CO2 uptake in certain regions (Hauck et al., 2015; Mongwe et al., 2024). In this context, events such as the one observed in summer 2022 – that is, an increased sea ice retreat and a northeastward advection of a fresh water mass accompanied with a shift of the CO2 sink to higher “northern” latitudes – could become more frequent and should be monitored carefully.
Vertical profiles provided by the glider were very useful to interpret CARIOCA surface measurements. Unfortunately, it was not possible to get additional vertical biogeochemical information from other platforms. Most of the BGC ARGO floats were too far away or did not bring any additional information to our study. Our results highlight the sparsity of data in the subpolar region and their importance to constrain the Southern Ocean CO2 flux.
Lagrangian backward trajectories suggest that the summer 2022 phytoplankton bloom in the subpolar ocean is mainly due to early sea ice retreat during the previous spring and not due to the proximity of Bouvet Island or seamounts, as was the case in Sergi et al. (2020). There was probably some fertilization in March 2022 due to the proximity of Bouvet Island. However, at the end of January 2022, the waters did not come from Bouvet Island, and the CARIOCA buoy was already in Chl-a-rich waters. This coincided with the presence of a fresh water mass and a strong summer 2022 fCO2 undersaturation. We show that the waters sampled by the buoy and the glider originate from a region southwest of the instruments' deployment, in the vicinity of the sea ice edge (Fig. 4). Early sea ice retreat in spring 2021 promoted the development of a large phytoplankton bloom at the ice edge. Waters depleted in carbon from the sea ice edge traveled farther north than usual, up to 54° S, causing a strong undersaturation of fCO2 and highly negative CO2 fluxes in a region usually known to be in equilibrium with the atmosphere (Gruber et al., 2019; Takahashi et al., 2012). Austral summer 2022 was a record for an unusually low sea ice extent (Wang et al., 2022). Our observations highlight the northward migration of the CO2 sink associated with early sea ice retreat, a phenomenon projected by Earth system models under climate change (Mongwe et al., 2024), with significant reduction in biologically derived fCO2 associated with melting ice and the consequent increase in the oceanic CO2 sink in certain sectors of the Southern Ocean.
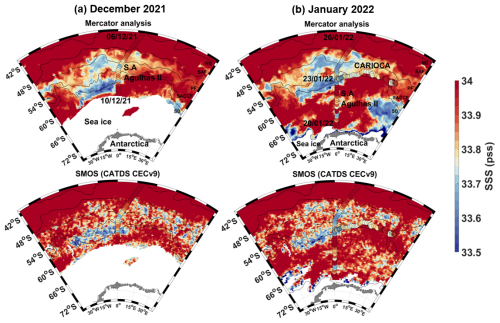
Figure C1Mercator salinity at 5 m and SMOS CATDS CECv9.0 SSS salinity at 5 m, with sea ice mask (0 % threshold of sea ice concentration) and fronts superimposed. (a) December 2021 mean SSS map, with S. A. Agulhas II SSS from 6–10 December 2021; (b) January 2022 mean SSS map, with CARIOCA SSS from 26 January 2022 to 27 June 2022 and S. A. Agulhas II SSS from 20–26 January 2022. SMOS SSS was adjusted by −0.1 pss to fit with CARIOCA and S. A. Agulhas II SSS.
On 19 December 2018, a Wave Glider (a surface autonomous vehicle) was deployed at 54° S, 0° E (Nicholson et al., 2022). It stayed fixed at this position for about 2 months and performed surface measurements until 11 February 2019. A comparison was made between this Wave Glider and the CARIOCA surface data. The CARIOCA buoy stayed near 54° S, 0° E from 26 January to 1 February 2022.
In 2019, the fCO2 measured by the Wave Glider was near equilibrium with the atmosphere (2019 Wave Glider fCO2 ∼ 410 µatm), whereas the CARIOCA buoy measured a large CO2 undersaturation (2022 CARIOCA fCO2 ∼ 330 µatm, Fig. 2a). In summer 2022 (from 26 January to 11 February 2022) the MLD was around 60 m, whereas in summer 2019 (from 19 December 2018 to 11 February 2019) the MLD was deeper, around 100 m (not shown). According to Nicholson's study, in 2019 the DIC concentration was around 2180 µmol kg−1, while in January to March 2022 it was much lower (∼ 2130 µmol kg−1). After March, as the buoy went out of the Chl-a-rich waters, the CARIOCA derived DIC increased gradually until it reached concentrations around 2170 µmol kg−1 in June 2022 (Fig. 2b), close to the ones measured by the Wave Glider in 2019.
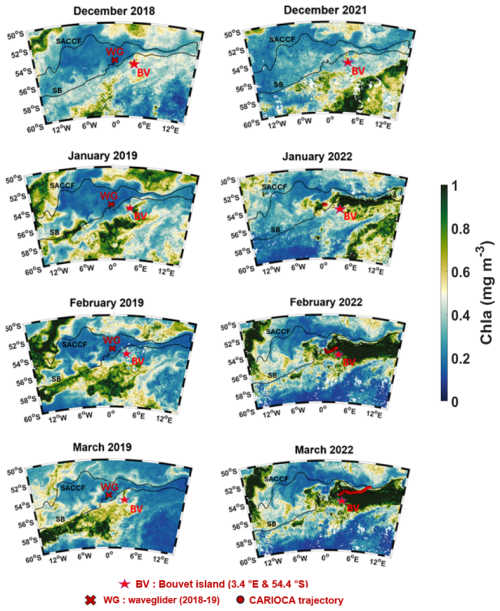
Figure D1CCI Chl a maps from December to March. Left panels: 2018–2019, with Wave Glider position for the given month in red. Right panels: same as left panels but for 2021–2022, with the CARIOCA buoy position for the given month in red. SB: Southern Boundary; SACCF: Southern Antarctic Circumpolar Current Front.
For the Chl a comparison, CCI data were used to determine the Chl a concentrations in the region in summer 2019 and 2022 (Fig. D1). From January to March 2022, a large phytoplankton bloom was observed around the Southern Boundary at the CARIOCA buoy position. This phytoplankton bloom was not present at the Wave Glider and CARIOCA buoy locations in summer 2019. Indeed, in January–March 2019 the bloom was farther south – south of the Southern Boundary (SB) (Fig. D1). The Chl a concentrations were also lower in summer 2019 compared to 2022 and reached up to 1.5 mg m−3 in 2022, whereas in 2019 the maximum Chl a concentrations did not exceed 1 mg m−3.
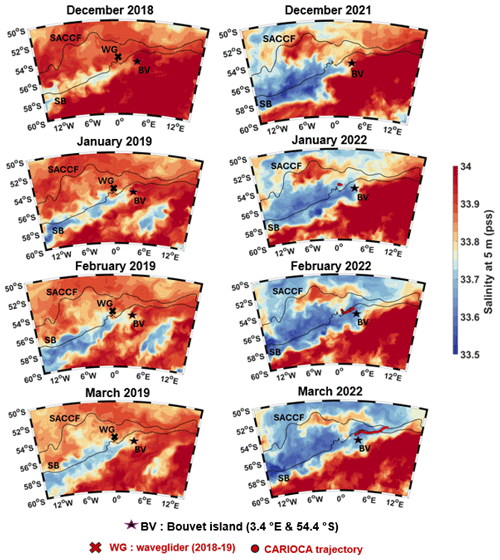
Figure D2Sea surface salinity (SSS) at 5 m from December to March. Left panels: Glorys SSS 2018–2019, with Wave Glider position for the given month in red. Right panels: Mercator SSS 2021–2022, with the CARIOCA buoy position for the given month in red. SB: Southern Boundary; SACCF: Subantarctic Circumpolar Current Front.
The phytoplankton bloom in summer 2022 in fact coincides with the presence of a fresh water mass. Mercator salinity profiles for spring 2021 to summer 2022 (not shown) show that this lower salinity was only present in the first 60 m, which also corresponds to the glider salinity profile (Fig. 3). According to Glorys, in 2019 the lower salinities were much farther south (Fig. D2).
A comparison was also performed with surface transects of the Norwegian RV Kronprins Haakon cruise from 28 February 2019 to 10 April 2019 (Ogundare et al., 2021). A ship transect crossed the CARIOCA path at around the same dates (end of March/beginning of April for both) for different years (2019 and 2022). North of the Southern Antarctic Circumpolar Current Front (SACCF), in April the DIC SST and SSS values for both years were consistent with each other. South of the SACCF, in 2019 the DIC concentration was higher (∼ 2150 µmol kg−1) compared to the CARIOCA derived DIC concentration in 2022 (as low as 2120 µmol kg−1, south of the SACCF). The ship's path in 2019, south of the SACCF, was between 53–57° S and 5–8° E. At these positions, in 2019 higher salinities were measured (ship SSS ∼ 34 pss, CARIOCA SSS ∼ 33.7 pss, before April) (Fig. D3), and the Chl a concentrations were lower compared to 2022 (Fig. D1).
Neglecting mixing and neglecting dfCO2 due to salinity change, the monthly changes of fCO2 are estimated as in Merlivat et al. (2015),
with
where R is the Revelle factor.
where ΔDIC(bio) is the contribution from biological production, and ΔDIC(air–sea) is the contribution from the air–sea flux,
where ρ is the seawater density and F is the air–sea flux (Eq. 1).
We assume an exchange coefficient, K, of 0.1/12 (Boutin et al., 2009) and a Revelle factor, R, of 16 (Sabine et al., 2004).
According to (McClish and Bushinsky, 2023) the highest rates of daily net community production occur during active sea ice retreat, and the bloom net community production is higher in the case of early sea ice retreat. Given that the water mass reaching the CARIOCA buoy's location in the subpolar region remained in the bloom near the sea ice edge from September to November 2021, i.e., over the whole bloom period, it seems reasonable to consider that the DIC of this water mass was affected by a bloom NCP typical of early ice retreat, of 3 mol C m−2 per bloom (Fig. 4 of McClish and Bushinsky, 2023). The MLD measured by the glider in summer 2022 is around 60 m. Assuming the same MLD depth in spring 2021, we estimate that the ΔDIC(bio) due to the bloom near the sea ice edge in spring 2021 was −50 µmol kg−1. Assuming a DIC concentration before melting of around 2200 µmol kg−1 (Briggs et al., 2018) and fCO2 initially in equilibrium with the atmosphere (fCO2 ∼ 400 µatm), the decrease in fCO2 in November due to bloom NCP is estimated to be −160 µatm (November fCO2 ∼ 240 µatm).
We then assume that, at first order, the fCO2 change along the water mass trajectory is only affected by air–sea exchange and temperature change (i.e., we neglect biological production along the water mass trajectory and mixing with subsurface water, which seems at first order reasonable given the relatively low Chl a along the water mass trajectory compared to the vicinity of the sea ice edge and given that the salinity anomaly persisted along the water mass trajectory until January 2022, respectively).
From November 2021 to December 2021, ΔDIC(air–sea) = 22 µmol kg−1, and the ∂fCO2(air–sea) is +42 µatm. After the air–sea flux correction, we obtain a DIC concentration of about 2172 µmol kg−1 and an fCO2 of 282 µatm. The waters from sea ice melt are at −1.8 °C (seawater's freezing point). According to Remote Sensing Systems (REMSS; see datasets) and to backward trajectories, the SST along the water mass trajectory was −0.5 °C at the end of November 2021. Considering a heating of 1.3 °C (∂T = +1.3 °C) during the month of November, the temperature effect leads to an increase in fCO2 of +15 µatm. At the end of December 2021, the fCO2 is therefore 297 µatm.
In January 2022, ΔDIC(air–sea) is +14 µmol kg−1, and the ∂fCO2(air–sea) is +31 µatm. After the air–sea flux correction the fCO2 is 328 µatm, and the DIC is 2186 µmol kg−1. According to REMSS and to backward trajectories, ∂T is +1.0 °C, and the ∂fCO2(SST) is 14 µatm. At the end of January 2021, the fCO2 estimate is therefore 342 µatm.
All data used in this study are freely available and downloadable from the following websites. CARIOCA dataset is available on SEANOE (https://doi.org/10.17882/100800, Naëck et al., 2024). The Seaglider (SG675) dataset is available online at https://doi.org/10.5281/zenodo.11059426 (Swart et al., 2024). The S. A. Agulhas II SO-CHIC 2022 cruise TSG dataset is available on SEANOE (https://doi.org/10.17882/100905, Ward et al., 2024). The S. A. Agulhas II SO-CHIC 2022 cruise CTD data are available on SEANOE (https://doi.org/10.17882/95314, Steiger et al., 2022). ERA5 atmospheric pressure and wind speed hourly data on single levels from 1940 to present are available on the Copernicus Climate Change Service Climate Data Store at https://doi.org/10.24381/cds.adbb2d47 (Hersbach et al., 2023). The Global Ocean Physics Reanalysis and the Global Ocean Physics Analysis and Forecast datasets are available on the Copernicus Marine Service at https://doi.org/10.48670/moi-00021 (CMEMS and MDS, 2024a) and https://doi.org/10.48670/moi-00016 (CMEMS and MDS, 2024b). The SMOS CATDS CECv9 salinity dataset is available online at https://doi.org/10.17882/52804#102161 (Boutin et al., 2023). The ISAS multiyear salinity dataset is available on the data store of the Copernicus Marine Service at https://doi.org/10.17882/46219 (Szekely et al., 2024). The OSCAR dataset is available at https://doi.org/10.5067/OSCAR-03D01 (ESR, 2009). The GlobCurrent dataset is available at https://doi.org/10.48670/mds-00327 (CMEMS and MDS, 2024c). The OCI-CCI chlorophyll a dataset is available online at https://www.oceancolour.org/ (last access: July 2024; https://catalogue.ceda.ac.uk/uuid/690fdf8f229c4d04a2aa68de67beb733/, Sathyendranath et al., 2023). The MW OI SST dataset produced by Remote Sensing Systems is available online at https://www.remss.com (last access: July 2024). The datasets “Global Sea Ice Concentration Climate Data Record v3.0 – Multimission, EUMETSAT SAF on Ocean and Sea Ice” and “Global Sea Ice Concentration Interim Climate Data Record Release 3 – DMSP, EUMETSAT SAF on Ocean and Sea Ice” are available online at https://doi.org/10.15770/EUM_SAF_OSI_0013 (OSI SAF, 2022a) and https://doi.org/10.15770/EUM_SAF_OSI_0014 (OSI SAF, 2022b). The SPASSO package containing the LAMTA software used for the Lagrangian analysis can be downloaded at https://github.com/OceanCruises/SPASSO/ (OceanCruises, 2025).
The supplement related to this article is available online at https://doi.org/10.5194/bg-22-1947-2025-supplement.
KN conducted the data processing, analyzed the results, performed the estimations, and wrote the paper. JB supervised the study and helped in the data processing, interpretation of the results, estimations, and paper's conception. SS and MdP provided the Seaglider (SG675) dataset. They also provided useful input concerning the Seaglider data processing and interpretation of the results. LM provided useful inputs and insights during the course of this study, suggesting various hypotheses and helping us investigate them. LB oversaw the verification of the calibration of CARIOCA sensors. AL helped in the deployment of the CARIOCA buoy during the SO-CHIC 2022 cruise. Their involvement during the COVID period enabled us to collect data vital to this study. Fd'O and LR provided the Python code used for the Lagrangian analysis, which is the basis of our study and without which we could not have proved the origin of the phytoplankton bloom. They helped with discussions and modifications related to this code for our specific study case. BW provided the TSG data of the S. A. Agulhas II ship (SO-CHIC 2022). JBS coordinated the SO-CHIC project and provided part of the financial support. All co-authors discussed, reviewed, and edited the paper.
The contact author has declared that none of the authors has any competing interests.
Publisher's note: Copernicus Publications remains neutral with regard to jurisdictional claims made in the text, published maps, institutional affiliations, or any other geographical representation in this paper. While Copernicus Publications makes every effort to include appropriate place names, the final responsibility lies with the authors.
The authors would like to thank Dimitry Khvorostyanov and Alain Laupin-Vinatier for the automation of CARIOCA data processing chain in real time. We thank Fabrice Bonjean, Sarah Nicholson, and Martin Vancoppenolle for helpful discussions. We also thank all those involved with the deployments of the CARIOCA buoy and glider during the SO-CHIC cruise on the RV S. A. Agulhas II.
This project is supported by funding from the European Union's Horizon 2020 research and innovation program (grant no. 821001 (SO-CHIC)). Louise Rousselet is supported by the CNES TOSCA BioSWOT-AdAC project. Sebastiaan Swart is supported by a Wallenberg Academy Fellowship (grant no. WAF 2015.0186) and the Swedish Research Council (grant no. VR 2019-04400).
This paper was edited by Hermann Bange and reviewed by two anonymous referees.
Ardyna, M., Lacour, L., Sergi, S., d'Ovidio, F., Sallée, J.-B., Rembauville, M., Blain, S., Tagliabue, A., Schlitzer, R., Jeandel, C., Arrigo, K. R., and Claustre, H.: Hydrothermal vents trigger massive phytoplankton blooms in the Southern Ocean, Nat. Commun., 10, 2451, https://doi.org/10.1038/s41467-019-09973-6, 2019.
Bakker, D. C. E., Pfeil, B., Landa, C. S., Metzl, N., O'Brien, K. M., Olsen, A., Smith, K., Cosca, C., Harasawa, S., Jones, S. D., Nakaoka, S., Nojiri, Y., Schuster, U., Steinhoff, T., Sweeney, C., Takahashi, T., Tilbrook, B., Wada, C., Wanninkhof, R., Alin, S. R., Balestrini, C. F., Barbero, L., Bates, N. R., Bianchi, A. A., Bonou, F., Boutin, J., Bozec, Y., Burger, E. F., Cai, W.-J., Castle, R. D., Chen, L., Chierici, M., Currie, K., Evans, W., Featherstone, C., Feely, R. A., Fransson, A., Goyet, C., Greenwood, N., Gregor, L., Hankin, S., Hardman-Mountford, N. J., Harlay, J., Hauck, J., Hoppema, M., Humphreys, M. P., Hunt, C. W., Huss, B., Ibánhez, J. S. P., Johannessen, T., Keeling, R., Kitidis, V., Körtzinger, A., Kozyr, A., Krasakopoulou, E., Kuwata, A., Landschützer, P., Lauvset, S. K., Lefèvre, N., Lo Monaco, C., Manke, A., Mathis, J. T., Merlivat, L., Millero, F. J., Monteiro, P. M. S., Munro, D. R., Murata, A., Newberger, T., Omar, A. M., Ono, T., Paterson, K., Pearce, D., Pierrot, D., Robbins, L. L., Saito, S., Salisbury, J., Schlitzer, R., Schneider, B., Schweitzer, R., Sieger, R., Skjelvan, I., Sullivan, K. F., Sutherland, S. C., Sutton, A. J., Tadokoro, K., Telszewski, M., Tuma, M., van Heuven, S. M. A. C., Vandemark, D., Ward, B., Watson, A. J., and Xu, S.: A multi-decade record of high-quality fCO2 data in version 3 of the Surface Ocean CO2 Atlas (SOCAT), Earth Syst. Sci. Data, 8, 383–413, https://doi.org/10.5194/essd-8-383-2016, 2016.
Blain, S., Quéguiner, B., Armand, L., Belviso, S., Bombled, B., Bopp, L., Bowie, A., Brunet, C., Brussaard, C., Carlotti, F., Christaki, U., Corbière, A., Durand, I., Ebersbach, F., Fuda, J.-L., Garcia, N., Gerringa, L., Griffiths, B., Guigue, C., Guillerm, C., Jacquet, S., Jeandel, C., Laan, P., Lefèvre, D., Lo Monaco, C., Malits, A., Mosseri, J., Obernosterer, I., Park, Y.-H., Picheral, M., Pondaven, P., Remenyi, T., Sandroni, V., Sarthou, G., Savoye, N., Scouarnec, L., Souhaut, M., Thuiller, D., Timmermans, K., Trull, T., Uitz, J., van Beek, P., Veldhuis, M., Vincent, D., Viollier, E., Vong, L., and Wagener, T.: Effect of natural iron fertilization on carbon sequestration in the Southern Ocean, Nature, 446, 1070–1074, https://doi.org/10.1038/nature05700, 2007.
Blain, S., Sarthou, G., and Laan, P.: Distribution of dissolved iron during the natural iron-fertilization experiment KEOPS (Kerguelen Plateau, Southern Ocean), Deep-Sea Res. Pt. II, 55, 594–605, https://doi.org/10.1016/j.dsr2.2007.12.028, 2008.
Boutin, J., Merlivat, L., HÉnocq, C., Martin, N., and SallÉe, J. B.: Air–sea CO2 flux variability in frontal regions of the Southern Ocean from CARbon Interface OCean Atmosphere drifters, Limnol. Oceanogr., 53, 2062–2079, https://doi.org/10.4319/lo.2008.53.5_part_2.2062, 2008.
Boutin, J., Quilfen, Y., Merlivat, L., and Piolle, J. F.: Global average of air–sea CO2 transfer velocity from QuikSCAT scatterometer wind speeds, J. Geophys. Res.-Oceans, 114, C04007, https://doi.org/10.1029/2007JC004168, 2009.
Boutin, J., Vergely, J.-L., and Khvorostyanov, D.: SMOS SSS L3 maps generated by CATDS CEC LOCEAN. debias V8.0, SEANOE [data set], https://doi.org/10.17882/52804#102161, 2023.
Boutin, J., Vergely, J.-L., and Khvorostyanov, D.: De-biased SMOS SSS L3 V9 maps generated by LOCEAN/ACRI-ST Expertise Center, SEANOE [data set], https://doi.org/10.17882/52804, 2024.
Boyd, P. W., Jickells, T., Law, C. S., Blain, S., Boyle, E. A., Buesseler, K. O., Coale, K. H., Cullen, J. J., de Baar, H. J. W., Follows, M., Harvey, M., Lancelot, C., Levasseur, M., Owens, N. P. J., Pollard, R., Rivkin, R. B., Sarmiento, J., Schoemann, V., Smetacek, V., Takeda, S., Tsuda, A., Turner, S., and Watson, A. J.: Mesoscale Iron Enrichment Experiments 1993-2005: Synthesis and Future Directions, Science, 315, 612–617, https://doi.org/10.1126/science.1131669, 2007.
Briggs, E. M., Martz, T. R., Talley, L. D., Mazloff, M. R., and Johnson, K. S.: Physical and Biological Drivers of Biogeochemical Tracers Within the Seasonal Sea Ice Zone of the Southern Ocean From Profiling Floats, J. Geophys. Res.-Oceans, 123, 746–758, https://doi.org/10.1002/2017JC012846, 2018.
Copin-Montégut, C., Bégovic, M., and Merlivat, L.: Variability of the partial pressure of CO2 on diel to annual time scales in the Northwestern Mediterranean Sea, Mar. Chem., 85, 169–189, https://doi.org/10.1016/j.marchem.2003.10.005, 2004.
Death, R., Wadham, J. L., Monteiro, F., Le Brocq, A. M., Tranter, M., Ridgwell, A., Dutkiewicz, S., and Raiswell, R.: Antarctic ice sheet fertilises the Southern Ocean, Biogeosciences, 11, 2635–2643, https://doi.org/10.5194/bg-11-2635-2014, 2014.
de Boyer Montégut, C., Madec, G., Fischer, A. S., Lazar, A., and Iudicone, D.: Mixed layer depth over the global ocean: An examination of profile data and a profile-based climatology, J. Geophys. Res.-Oceans, 109, C12003, https://doi.org/10.1029/2004JC002378, 2004.
DeVries, T.: The oceanic anthropogenic CO2 sink: Storage, air–sea fluxes, and transports over the industrial era, Global Biogeochem. Cy., 28, 631–647, https://doi.org/10.1002/2013GB004739, 2014.
d'Ovidio, F., Della Penna, A., Trull, T. W., Nencioli, F., Pujol, M.-I., Rio, M.-H., Park, Y.-H., Cotté, C., Zhou, M., and Blain, S.: The biogeochemical structuring role of horizontal stirring: Lagrangian perspectives on iron delivery downstream of the Kerguelen Plateau, Biogeosciences, 12, 5567–5581, https://doi.org/10.5194/bg-12-5567-2015, 2015.
ESR: OSCAR third deg. Ver. 1, PO.DAAC, CA, USA [data set], https://doi.org/10.5067/OSCAR-03D01, 2009.
E.U. Copernicus Marine Service Information (CMEMS) and Marine Data Store (MDS): Global Ocean Physics Reanalysis, Copernicus Marine Service [data set], https://doi.org/10.48670/moi-00021, 2024a.
E.U. Copernicus Marine Service Information (CMEMS) and Marine Data Store (MDS): Global Ocean Physics Analysis and Forecast, Copernicus Marine Service [data set], https://doi.org/10.48670/moi-00016, 2024b.
E.U. Copernicus Marine Service Information (CMEMS) and Marine Data Store (MDS): Global Total (COPERNICUS-GLOBCURRENT), Ekman and Geostrophic currents at the Surface and 15 m, https://doi.org/10.48670/mds-00327, 2024c.
Friedlingstein, P., O'Sullivan, M., Jones, M. W., Andrew, R. M., Bakker, D. C. E., Hauck, J., Landschützer, P., Le Quéré, C., Luijkx, I. T., Peters, G. P., Peters, W., Pongratz, J., Schwingshackl, C., Sitch, S., Canadell, J. G., Ciais, P., Jackson, R. B., Alin, S. R., Anthoni, P., Barbero, L., Bates, N. R., Becker, M., Bellouin, N., Decharme, B., Bopp, L., Brasika, I. B. M., Cadule, P., Chamberlain, M. A., Chandra, N., Chau, T.-T.-T., Chevallier, F., Chini, L. P., Cronin, M., Dou, X., Enyo, K., Evans, W., Falk, S., Feely, R. A., Feng, L., Ford, D. J., Gasser, T., Ghattas, J., Gkritzalis, T., Grassi, G., Gregor, L., Gruber, N., Gürses, Ö., Harris, I., Hefner, M., Heinke, J., Houghton, R. A., Hurtt, G. C., Iida, Y., Ilyina, T., Jacobson, A. R., Jain, A., Jarníková, T., Jersild, A., Jiang, F., Jin, Z., Joos, F., Kato, E., Keeling, R. F., Kennedy, D., Klein Goldewijk, K., Knauer, J., Korsbakken, J. I., Körtzinger, A., Lan, X., Lefèvre, N., Li, H., Liu, J., Liu, Z., Ma, L., Marland, G., Mayot, N., McGuire, P. C., McKinley, G. A., Meyer, G., Morgan, E. J., Munro, D. R., Nakaoka, S.-I., Niwa, Y., O'Brien, K. M., Olsen, A., Omar, A. M., Ono, T., Paulsen, M., Pierrot, D., Pocock, K., Poulter, B., Powis, C. M., Rehder, G., Resplandy, L., Robertson, E., Rödenbeck, C., Rosan, T. M., Schwinger, J., Séférian, R., Smallman, T. L., Smith, S. M., Sospedra-Alfonso, R., Sun, Q., Sutton, A. J., Sweeney, C., Takao, S., Tans, P. P., Tian, H., Tilbrook, B., Tsujino, H., Tubiello, F., van der Werf, G. R., van Ooijen, E., Wanninkhof, R., Watanabe, M., Wimart-Rousseau, C., Yang, D., Yang, X., Yuan, W., Yue, X., Zaehle, S., Zeng, J., and Zheng, B.: Global Carbon Budget 2023, Earth Syst. Sci. Data, 15, 5301–5369, https://doi.org/10.5194/essd-15-5301-2023, 2023.
Frölicher, T. L., Sarmiento, J. L., Paynter, D. J., Dunne, J. P., Krasting, J. P., and Winton, M.: Dominance of the Southern Ocean in Anthropogenic Carbon and Heat Uptake in CMIP5 Models, J. Climate, 28, 862–886, https://doi.org/10.1175/JCLI-D-14-00117.1, 2015.
Giunta, V. and Ward, B.: Ocean mixed layer depth from dissipation, J. Geophys. Res.-Oceans, 127, e2021JC017904, https://doi.org/10.1029/2021JC017904, 2022.
Gregor, L., Ryan-Keogh, T. J., Nicholson, S.-A., du Plessis, M., Giddy, I., and Swart, S.: GliderTools: A Python Toolbox for Processing Underwater Glider Data, Front. Mar. Sci., 6, 738, https://doi.org/10.3389/fmars.2019.00738, 2019.
Gruber, N., Landschützer, P., and Lovenduski, N. S.: The Variable Southern Ocean Carbon Sink, Annu. Rev. Mar. Sci., 11, 159–186, https://doi.org/10.1146/annurev-marine-121916-063407, 2019.
Hauck, J., Völker, C., Wolf-Gladrow, D. A., Laufkötter, C., Vogt, M., Aumont, O., Bopp, L., Buitenhuis, E. T., Doney, S. C., Dunne, J., Gruber, N., Hashioka, T., John, J., Quéré, C. L., Lima, I. D., Nakano, H., Séférian, R., and Totterdell, I.: On the Southern Ocean CO2 uptake and the role of the biological carbon pump in the 21st century, Global Biogeochem. Cy., 29, 1451–1470, https://doi.org/10.1002/2015GB005140, 2015.
Hauck, J., Nissen, C., Landschützer, P., Rödenbeck, C., Bushinsky, S., and Olsen, A.: Sparse observations induce large biases in estimates of the global ocean CO2 sink: an ocean model subsampling experiment, Philos. T. R. Soc. A, 381, 20220063, https://doi.org/10.1098/rsta.2022.0063, 2023.
Haumann, F. A., Gruber, N., Münnich, M., Frenger, I., and Kern, S.: Sea-ice transport driving Southern Ocean salinity and its recent trends, Nature, 537, 89–92, https://doi.org/10.1038/nature19101, 2016.
Henley, S. F., Cavan, E. L., Fawcett, S. E., Kerr, R., Monteiro, T., Sherrell, R. M., Bowie, A. R., Boyd, P. W., Barnes, D. K. A., Schloss, I. R., Marshall, T., Flynn, R., and Smith, S.: Changing Biogeochemistry of the Southern Ocean and Its Ecosystem Implications, Front. Mar. Sci., 7, 581, https://doi.org/10.3389/fmars.2020.00581, 2020.
Hersbach, H., Bell, B., Berrisford, P., Biavati, G., Horányi, A., Muñoz Sabater, J., Nicolas, J., Peubey, C., Radu, R., Rozum, I., Schepers, D., Simmons, A., Soci, C., Dee, D., and Thépaut, J.-N.: ERA5 hourly data on single levels from 1940 to present, Copernicus Climate Change Service (C3S) Climate Data Store (CDS) [data set], https://doi.org/10.24381/cds.adbb2d47, 2023.
Landschützer, P., Gruber, N., Haumann, F. A., Rödenbeck, C., Bakker, D. C. E., Van Heuven, S., Hoppema, M., Metzl, N., Sweeney, C., Takahashi, T., Tilbrook, B., and Wanninkhof, R.: The reinvigoration of the Southern Ocean carbon sink, Science, 349, 1221–1224, https://doi.org/10.1126/science.aab2620, 2015.
Lannuzel, D., Vancoppenolle, M., van der Merwe, P., de Jong, J., Meiners, K. M., Grotti, M., Nishioka, J., and Schoemann, V.: Iron in sea ice: Review and new insights, Elem. Sci. Anthr., 4, 000130, https://doi.org/10.12952/journal.elementa.000130, 2016.
Laws, E. A.: Photosynthetic quotients, new production and net community production in the open ocean, Deep-Sea Res., 38, 143–167, https://doi.org/10.1016/0198-0149(91)90059-O, 1991.
Lee, K., Tong, L. T., Millero, F. J., Sabine, C. L., Dickson, A. G., Goyet, C., Park, G.-H., Wanninkhof, R., Feely, R. A., and Key, R. M.: Global relationships of total alkalinity with salinity and temperature in surface waters of the world's oceans, Geophys. Res. Lett., 33, L19605, https://doi.org/10.1029/2006GL027207, 2006.
Lueker, T. J., Dickson, A. G., and Keeling, C. D.: Ocean pCO2 calculated from dissolved inorganic carbon, alkalinity, and equations for K1 and K2: validation based on laboratory measurements of CO2 in gas and seawater at equilibrium, Mar. Chem., 70, 105–119, https://doi.org/10.1016/S0304-4203(00)00022-0, 2000.
Mayot, N., Le Quéré, C., Rödenbeck, C., Bernardello, R., Bopp, L., Djeutchouang, L. M., Gehlen, M., Gregor, L., Gruber, N., Hauck, J., Iida, Y., Ilyina, T., Keeling, R. F., Landschützer, P., Manning, A. C., Patara, L., Resplandy, L., Schwinger, J., Séférian, R., Watson, A. J., Wright, R. M., and Zeng, J.: Climate-driven variability of the Southern Ocean CO2 sink, Philos. T. R. Soc. A, 381, 20220055, https://doi.org/10.1098/rsta.2022.0055, 2023.
McClish, S. and Bushinsky, S. M.: Majority of Southern Ocean Seasonal Sea Ice Zone Bloom Net Community Production Precedes Total Ice Retreat, Geophys. Res. Lett., 50, e2023GL103459, https://doi.org/10.1029/2023GL103459, 2023.
Meijers, A. J. S., Le Quéré, C., Monteiro, P. M. S., and Sallée, J.-B.: Heat and carbon uptake in the Southern Ocean: the state of the art and future priorities, Philos. T. R. Soc. A, 381, 20220071, https://doi.org/10.1098/rsta.2022.0071, 2023.
Merlivat, L., Boutin, J., and d'Ovidio, F.: Carbon, oxygen and biological productivity in the Southern Ocean in and out the Kerguelen plume: CARIOCA drifter results, Biogeosciences, 12, 3513–3524, https://doi.org/10.5194/bg-12-3513-2015, 2015.
Merlivat, L., Hemming, M., Boutin, J., Antoine, D., Vellucci, V., Golbol, M., Lee, G. A., and Beaumont, L.: Physical mechanisms for biological carbon uptake during the onset of the spring phytoplankton bloom in the northwestern Mediterranean Sea (BOUSSOLE site), Biogeosciences, 19, 3911–3920, https://doi.org/10.5194/bg-19-3911-2022, 2022.
Mongwe, P., Gregor, L., Tjiputra, J., Hauck, J., Ito, T., Danek, C., Vichi, M., Thomalla, S., and Monteiro, P. M. S.: Projected poleward migration of the Southern Ocean CO2 sink region under high emissions, Commun. Earth Environ., 5, 1–13, https://doi.org/10.1038/s43247-024-01382-y, 2024.
Naëck, K., Boutin, J., Beaumont, L., Lourenco, A., and Sallée, J.-B.: CARIOCA observations – SO-CHIC Cruise 2022, SEANOE [data set], https://doi.org/10.17882/100800, 2024.
Nicholson, S.-A., Whitt, D. B., Fer, I., Du Plessis, M. D., Lebéhot, A. D., Swart, S., Sutton, A. J., and Monteiro, P. M. S.: Storms drive outgassing of CO2 in the subpolar Southern Ocean, Nat. Commun., 13, 158, https://doi.org/10.1038/s41467-021-27780-w, 2022.
OceanCruises: SPASSO, GitHub [code], https://github.com/OceanCruises/SPASSO/, last access: May 2025.
Ogundare, M. O., Fransson, A., Chierici, M., Joubert, W. R., and Roychoudhury, A. N.: Variability of Sea-Air Carbon Dioxide Flux in Autumn Across the Weddell Gyre and Offshore Dronning Maud Land in the Southern Ocean, Front. Mar. Sci., 7, 614263, https://doi.org/10.3389/fmars.2020.614263, 2021.
OSI SAF: Global Sea Ice Concentration Climate Data Record v3.0 – Multimission, EUMETSAT SAF on Ocean and Sea Ice [data set], https://doi.org/10.15770/EUM_SAF_OSI_0013, 2022a.
OSI SAF: Global Sea Ice Concentration Interim Climate Data Record Release 3 - DMSP, EUMETSAT SAF on Ocean and Sea Ice [data set], https://doi.org/10.15770/EUM_SAF_OSI_0014, 2022b.
Park, Y.-H., Park, T., Kim, T.-W., Lee, S.-H., Hong, C.-S., Lee, J.-H., Rio, M.-H., Pujol, M.-I., Ballarotta, M., Durand, I., and Provost, C.: Observations of the Antarctic Circumpolar Current Over the Udintsev Fracture Zone, the Narrowest Choke Point in the Southern Ocean, J. Geophys. Res.-Oceans, 124, 4511–4528, https://doi.org/10.1029/2019JC015024, 2019.
Pellichero, V., Boutin, J., Claustre, H., Merlivat, L., Sallée, J., and Blain, S.: Relaxation of Wind Stress Drives the Abrupt Onset of Biological Carbon Uptake in the Kerguelen Bloom: A Multisensor Approach, Geophys. Res. Lett., 47, e2019GL085992, https://doi.org/10.1029/2019GL085992, 2020.
Person, R., Aumont, O., Madec, G., Vancoppenolle, M., Bopp, L., and Merino, N.: Sensitivity of ocean biogeochemistry to the iron supply from the Antarctic Ice Sheet explored with a biogeochemical model, Biogeosciences, 16, 3583–3603, https://doi.org/10.5194/bg-16-3583-2019, 2019.
Person, R., Vancoppenolle, M., Aumont, O., and Malsang, M.: Continental and Sea Ice Iron Sources Fertilize the Southern Ocean in Synergy, Geophys. Res. Lett., 48, e2021GL094761, https://doi.org/10.1029/2021GL094761, 2021.
Ricciardulli, L.: ASCAT on MetOp-A Data Product Update Notes, report number 040416, Remote Sensing Systems, Santa Rosa, CA, 5 pp., https://doi.org/10.56236/RSS-bb, 2016.
Rousselet, L., d'Ovidio, F., Izard, L., Della Penna, A., Petrenko, A., Barrillon, S., Nencioli, F., and Doglioli, A.: A Software Package for an Adaptive Satellite-based Sampling for Oceanographic cruises (SPASSOv2.0): tracking fine scale features for physical and biogeochemical studies, hal-04705438, https://hal.science/hal-04705438v1, last access: July 2024.
Sabine, C. L., Feely, R. A., Gruber, N., Key, R. M., Lee, K., Bullister, J. L., Wanninkhof, R., Wong, C. S., Wallace, D. W. R., Tilbrook, B., Millero, F. J., Peng, T.-H., Kozyr, A., Ono, T., and Rios, A. F.: The Oceanic Sink for Anthropogenic CO2, Science, 305, 367–371, https://doi.org/10.1126/science.1097403, 2004.
Sanial, V., van Beek, P., Lansard, B., d'Ovidio, F., Kestenare, E., Souhaut, M., Zhou, M., and Blain, S.: Study of the phytoplankton plume dynamics off the Crozet Islands (Southern Ocean): A geochemical-physical coupled approach, J. Geophys. Res.-Oceans 119, 2227–2237, 2014.
Sarmiento, J. L., Johnson, K. S., Arteaga, L. A., Bushinsky, S. M., Cullen, H. M., Gray, A. R., Hotinski, R. M., Maurer, T. L., Mazloff, M. R., Riser, S. C., Russell, J. L., Schofield, O. M., and Talley, L. D.: The Southern Ocean carbon and climate observations and modeling (SOCCOM) project: A review, Prog. Oceanogr., 219, 103130, https://doi.org/10.1016/j.pocean.2023.103130, 2023.
Sathyendranath, S., Jackson, T., Brockmann, C., Brotas, V., Calton, B., Chuprin, A., Clements, O., Cipollini, P., Danne, O., Dingle, J., Donlon, C., Grant, M., Groom, S., Krasemann, H., Lavender, S., Mazeran, C., Mélin, F., Müller, D., Steinmetz, F., Valente, A., Zühlke, M., Feldman, G., Franz, B., Frouin, R., Werdell, J., and Platt, T.: ESA Ocean Colour Climate Change Initiative (Ocean_Colour_cci): Monthly climatology of global ocean colour data products at 4km resolution, Version 6.0, NERC EDS Centre for Environmental Data Analysis [data set], https://catalogue.ceda.ac.uk/uuid/690fdf8f229c4d04a2aa68de67beb733/ (last access: July 2024), 2023.
Sergi, S., Baudena, A., Cotté, C., Ardyna, M., Blain, S., and d'Ovidio, F.: Interaction of the Antarctic Circumpolar Current With Seamounts Fuels Moderate Blooms but Vast Foraging Grounds for Multiple Marine Predators, Front. Mar. Sci., 7, 416, https://doi.org/10.3389/fmars.2020.00416, 2020.
Smith, R. M. and Bigg, G. R.: Impact of Giant Iceberg A68A on the Physical Conditions of the Surface South Atlantic, Derived Using Remote Sensing, Geophys. Res. Lett., 50, e2023GL104028, https://doi.org/10.1029/2023GL104028, 2023.
Smith, W. O. and Comiso, J. C.: Influence of sea ice on primary production in the Southern Ocean: A satellite perspective, J. Geophys. Res., 113, 2007JC004251, https://doi.org/10.1029/2007JC004251, 2008.
Steiger, N., Sallée, J., Ward, B., Azevedo, A., Binase, Z., Ten Doeschat, A., Els, H., Hamnca, S., Jacobs, L., Katsoulis, M., Lavanchy, S., Lavis, C., Lourenco, A., Du Plessis, M., Rosenthal, H., Soares, B., and Spira, T.: CTD observation – SO-CHIC Cruise 2022, SEANOE [data set], https://doi.org/10.17882/95314, 2022.
Sutherland, G., Reverdin, G., Marié, L., and Ward, B.: Mixed and mixing layer depths in the ocean surface boundary layer under conditions of diurnal stratification, Geophys. Res. Lett., 41, 8469–8476, https://doi.org/10.1002/2014GL061939, 2014.
Sutton, A. J., Williams, N. L., and Tilbrook, B.: Constraining Southern Ocean CO2 Flux Uncertainty Using Uncrewed Surface Vehicle Observations, Geophys. Res. Lett., 48, e2020GL091748, https://doi.org/10.1029/2020GL091748, 2021.
Swart, S., du Plessis, M., Giddy, I., Edholm, J., Spira, T., Biddle, L., and Rosenthal, H. S.: Dataset from autonomous assets collected during the SO-CHIC field campaign in the Southern Ocean (1), Zenodo [data set], https://doi.org/10.5281/zenodo.11059426, 2024.
Szekely, T., Gourrion, J., Pouliquen, S., and Reverdin, G.: CORA, Coriolis Ocean Dataset for Reanalysis, SEANOE [data set], https://doi.org/10.17882/46219, 2024.
Tagliabue, A., Sallée, J.-B., Bowie, A. R., Lévy, M., Swart, S., and Boyd, P. W.: Surface-water iron supplies in the Southern Ocean sustained by deep winter mixing, Nat. Geosci., 7, 314–320, https://doi.org/10.1038/ngeo2101, 2014.
Takahashi, T., Sweeney, C., Hales, B., Chipman, D. W., Newberger, T., Goddard, J. G., Iannuzzi, R. A., and Sutherland, S. C.: The Changing Carbon Cycle in the Southern Ocean, Oceanography, 25, 26–37, 2012.
The SO-CHIC consortium, Sallée, J. B., Abrahamsen, E. P., Allaigre, C., Auger, M., Ayres, H., Badhe, R., Boutin, J., Brearley, J. A., De Lavergne, C., Ten Doeschate, A. M. M., Droste, E. S., Du Plessis, M. D., Ferreira, D., Giddy, I. S., Gülk, B., Gruber, N., Hague, M., Hoppema, M., Josey, S. A., Kanzow, T., Kimmritz, M., Lindeman, M. R., Llanillo, P. J., Lucas, N. S., Madec, G., Marshall, D. P., Meijers, A. J. S., Meredith, M. P., Mohrmann, M., Monteiro, P. M. S., Mosneron Dupin, C., Naeck, K., Narayanan, A., Naveira Garabato, A. C., Nicholson, S.-A., Novellino, A., Ödalen, M., Østerhus, S., Park, W., Patmore, R. D., Piedagnel, E., Roquet, F., Rosenthal, H. S., Roy, T., Saurabh, R., Silvy, Y., Spira, T., Steiger, N., Styles, A. F., Swart, S., Vogt, L., Ward, B., and Zhou, S.: Southern ocean carbon and heat impact on climate, Philos. T. R. Soc. A, 381, 20220056, https://doi.org/10.1098/rsta.2022.0056, 2023.
Thomalla, S. J., Moutier, W., Ryan-Keogh, T. J., Gregor, L., and Schütt, J.: An optimized method for correcting fluorescence quenching using optical backscattering on autonomous platforms: Method for correcting fluorescence quenching, Limnol. Oceanogr.-Meth., 16, 132–144, https://doi.org/10.1002/lom3.10234, 2018.
Vernet, M., Geibert, W., Hoppema, M., Brown, P. J., Haas, C., Hellmer, H. H., Jokat, W., Jullion, L., Mazloff, M., Bakker, D. C. E., Brearley, J. A., Croot, P., Hattermann, T., Hauck, J., Hillenbrand, C. -D., Hoppe, C. J. M., Huhn, O., Koch, B. P., Lechtenfeld, O. J., Meredith, M. P., Naveira Garabato, A. C., Nöthig, E. -M., Peeken, I., Rutgers Van Der Loeff, M. M., Schmidtko, S., Schröder, M., Strass, V. H., Torres-Valdés, S., and Verdy, A.: The Weddell Gyre, Southern Ocean: Present Knowledge and Future Challenges, Rev. Geophys., 57, 623–708, https://doi.org/10.1029/2018RG000604, 2019.
Wang, J., Luo, H., Yang, Q., Liu, J., Yu, L., Shi, Q., and Han, B.: An Unprecedented Record Low Antarctic Sea-ice Extent during Austral Summer 2022, Adv. Atmos. Sci., 39, 1591–1597, https://doi.org/10.1007/s00376-022-2087-1, 2022.
Wanninkhof, R.: Relationship between wind speed and gas exchange over the ocean, J. Geophys. Res., 97, 7373, https://doi.org/10.1029/92JC00188, 1992.
Wanninkhof, R.: Relationship between wind speed and gas exchange over the ocean revisited: Gas exchange and wind speed over the ocean, Limnol. Oceanogr.-Meth., 12, 351–362, https://doi.org/10.4319/lom.2014.12.351, 2014.
Ward, B., Azevedo, A., Binase, Z., ten Doeschate, A., Els, H., Hamnca, S., Jacobs, L., Katsoulis, M., Lavanchy, S., Lavis, C., Lourenco, A., du Plessis, M., Rosenthal, H., Sallee, J.-B., Soares, B., Spira, T., and Steiger, N.: SOCHIC cruise report, Zenodo, https://doi.org/10.5281/ZENODO.6948850, 2022.
Ward, B., Naëck, K., Boutin, J., Sallée, J.-B., Jacobs, L., and Steiger, N.: TSG observations – SO-CHIC Cruise 2022, SEANOE [data set], https://doi.org/10.17882/100905, 2024.
- Abstract
- Introduction
- Materials and methods
- Results
- Discussion
- Conclusion
- Appendix A: Comparison between CARIOCA SSS and Mercator SSS
- Appendix B: Net community production (NCP) estimations
- Appendix C: Fresh water mass as detected by different salinity products
- Appendix D: Comparison between summers 2022 and 2019
- Appendix E: Estimation of DIC and fCO2 variations along the water mass trajectory
- Code and data availability
- Author contributions
- Competing interests
- Disclaimer
- Acknowledgements
- Financial support
- Review statement
- References
- Supplement
- Abstract
- Introduction
- Materials and methods
- Results
- Discussion
- Conclusion
- Appendix A: Comparison between CARIOCA SSS and Mercator SSS
- Appendix B: Net community production (NCP) estimations
- Appendix C: Fresh water mass as detected by different salinity products
- Appendix D: Comparison between summers 2022 and 2019
- Appendix E: Estimation of DIC and fCO2 variations along the water mass trajectory
- Code and data availability
- Author contributions
- Competing interests
- Disclaimer
- Acknowledgements
- Financial support
- Review statement
- References
- Supplement