the Creative Commons Attribution 4.0 License.
the Creative Commons Attribution 4.0 License.
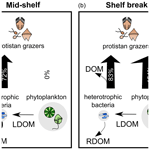
The bacteria–protist link as a main route of dissolved organic matter across contrasting productivity areas on the Patagonian Shelf
M. Celeste López-Abbate
John E. Garzón-Cardona
Ricardo Silva
Juan-Carlos Molinero
Laura A. Ruiz-Etcheverry
Ana M. Martínez
Azul S. Gilabert
Rubén J. Lara
While the sources of dissolved organic matter (DOM) in the open ocean are relatively well identified, its fate due to microbial activity is still evolving. Here, we explored how microbial community structure, growth, and grazing of phytoplankton and heterotrophic bacteria influenced the DOM pool and the transformation of its fluorescent fraction. Dilution experiments were performed during the productive season on the Patagonian Shelf (SW Atlantic Ocean), a region of intense biological activity, with peak productivity observed at the shelf break front. Although phytoplankton biomass was higher than that of bacteria, protists selectively preyed on the faster-growing bacterial population, denoting trophic specificity of grazers. High trophic coupling was suggested by the biomass distribution of protistan consumers and their prey, which predominantly exhibited an inverted trophic pyramid structure. An exception to this pattern was observed at the highly productive shelf break front, where a traditional bottom-heavy pyramid emerged, indicating that most phytoplankton evaded protist predation despite evidence of herbivory. Bacterial consumption of DOM appeared uncoupled from its total amount but was influenced by DOM complexity, while the bacterial production of humic-like substances from protistan plankton precursors observed in most experiments highlighted a potential pathway for carbon sequestration. Protistan grazers also significantly influenced DOM dynamics by scaling their DOM contribution in response to the intensity of grazing on heterotrophic bacteria, regardless of productivity levels. This effect likely arises from reducing the number of active DOM-consuming bacteria and by providing egestion DOM compounds. At the onset of the productive season, high bacterial growth rates stimulate protistan grazing, which serves as a link between bacterial biomass and higher trophic levels. However, as grazing pressure increases, protists can also contribute to the accumulation of a fraction of DOM.
- Article
(3552 KB) - Full-text XML
- BibTeX
- EndNote
Dissolved organic matter (DOM), the second-largest carbon reservoir in the ocean after dissolved inorganic carbon, is subject to dynamic regulation by physical and biological processes (Spencer et al., 2007). Unveiling the nature and dynamics of DOM is essential for gaining deeper understanding of the biochemical pathways through which carbon circulates in marine ecosystems. The optical properties of DOM serve as a marker for its sources and biological reactivity, with chromophoric DOM reflecting phytoplankton production and fluorescence DOM (FDOM) revealing potential sources and biological and photochemical interactions (Stedmon et al., 2003; Romera-Castillo et al., 2010).
Phytoplankton are primary contributors to DOM, yet processes such as viral lysis and protistan grazing also play substantial roles in shaping its composition and quantity. Protistan grazers not only recycle nutrients and enhance bloom sustainability but also impact carbon cycling through DOM production via biomass reworking and excretion (Kujawinski et al., 2004; Baña et al., 2014; Moran et al., 2022). In addition, grazers influence DOM pools by exhibiting selective feeding behaviours regarding bacterial and phytoplanktonic prey. For example, while size-specific grazing prompts compositional shifts in phytoplankton (e.g. Kanayama et al., 2020), the more generalist grazing on bacteria implies that community structure remains relatively unchanged under grazing pressure (Baltar et al., 2016). On the other hand, grazing on bacteria seems to remain close to bacterial production, specially under oligotrophic conditions (Sanders et al., 1992), while phytoplankton may temporally scape protistan grazing under favourable growth conditions, thus allowing bloom formation (Irigoien et al., 2005). This results in varying trophic transfer efficiency between phytoplankton- and bacteria-based food webs, with the former typically involving fewer carbon steps before reaching microcrustaceans (Berglund et al., 2007).
The Patagonian Shelf, one of the world's largest continental shelf regions, serves as a hotspot for carbon cycling due to its high productivity and dynamic mixing processes that connect the euphotic zone with bottom sediments (Laruelle et al., 2018). One of its defining features is a 2500 km long upwelling front at the shelf break, known for recurrent spring blooms and significant biogeochemical transformations that contribute to global carbon sequestration (Romero et al., 2006; Kahl et al., 2017). The emergence of an acidification trend in the water masses adjacent to the shelf is likely linked to ongoing carbon dioxide (CO2) capture, especially in the northern area of the shelf (Orselli et al., 2018).
Our study aimed to evaluate the role of protists in transferring bacterial and phytoplankton biomass under different productivity conditions and to assess potential changes in the chromophoric fraction of DOM (primarily representing aromatic organic compounds) and FDOM in these scenarios. We found that protistan grazing selectively targeted bacteria under both productivity regimes and that intense grazing pressure on bacteria may contribute to the short-term accumulation of DOM substances, irrespective of productivity levels. These findings enhance our understanding of the ecological mechanisms influencing carbon flow in marine ecosystems.
2.1 Sample collection and analyses
Two groups of stations were selected in the mid-shelf (stations 23, 22, and 21 from the coast to the open ocean) and in the shelf break front (stations 14, 13, and 12 from the coast to the open ocean). Mid-shelf stations were separated by ca. 30 km, intercepting the 50 m isobath, while shelf break front stations were separated by ca. 18 km and intercepted the 100 and 200 m isobaths. According to the bioregionalization of the Patagonian Shelf waters proposed by Delgado et al. (2023), mid-shelf stations were located in low- to moderate-productivity regions (mean chlorophyll a concentration during the spring peak between 1.14 and 2.48 µg L−1), while the shelf break front stations were nested in the highly productive upwelling region (mean chlorophyll a concentration during the spring peak of 5.8 µg L−1). Hydrographic data (temperature, salinity, pressure, and fluorescence) were taken with an SBE 9plus CTD profiler during cruise H0917 from 9 to 12 October 2017.
Water samples for dissolved nutrients and for running the experiments were taken from the chlorophyll a maximum with 6 L Niskin bottles attached to the CTD rosette, while dissolved organic carbon (DOC) samples were taken in the surface layer (5 m). The measurement of inorganic nutrients (NO, NO, NH, PO, and SiO) was carried out by analysing 50 mL aliquots of seawater preserved with HgCl2 solution (Kattner and Becker, 1991). The concentration of dissolved inorganic nitrogen (DIN) was calculated as the sum of NO, NO, and NH. Filtered (Whatman GF/F glass fibre filters with 0.7 µm pore size) samples for DOC were collected in pre-combusted 20 mL glass vials and acidified to pH < 2 with H3PO4. Filtrates were analysed using high-temperature (680 °C) catalytic oxidation with Al2O3 particles containing 0.5 % platinum (Pt) in a TOC analyser (Dohrmann DC-190, CA, USA). The resulting CO2 was then quantified using non-dispersive linearized infrared gas analysis (Skoog et al., 1997).
2.2 Satellite chlorophyll a
The spring phytoplankton bloom is a recurrent feature of the Patagonian Shelf and shelf break (e.g. Delgado et al., 2023). A time series of satellite-derived chlorophyll a was constructed to capture the bloom timing at each station during the sampling period. We define blooming conditions as periods where the net rate of biomass change (specific division rate minus specific loss rate) remains positive long enough to result in net biomass accumulation (Behrenfeld and Boss, 2018). Factors like wind stress that may influence plankton aggregation are not considered contributors to these conditions. Moderate Resolution Imaging Spectroradiometer (MODIS) Aqua images of chlorophyll a concentration were downloaded from National Aeronautics and Space Administration (NASA) Ocean Color (https://oceancolor.gsfc.nasa.gov/, last access: December 2023). Daily Level 3 images with a spatial resolution of 4 km were obtained for the period spanning August 2017 to December 2017, capturing the closest pixel to each sampling point. To minimize the percentage of missing values, we computed the 5 d mean and applied a low-pass filter to remove the variability lower than 28 d.
2.3 Experimental setup
Feeding experiments, based on the dilution technique (Landry and Hassett, 1982), were prepared by gently mixing different percentages of unfiltered water and filtered water through <0.2 µm Whatman polycarbonate filters. Four dilution treatments (D) were prepared using the filtered and unfiltered water: 10 %, 40 %, 70 %, and 100 % (whole water). Water was pre-filtered by a 200 µm mesh net to eliminate larger metazoan grazers. Acid-cleaned 1 L glass experimental bottles (three replicates) were deployed daily (24 h) at a deck incubator (200 L) equipped with continuous in situ water flow and covered with a double-knitted mesh fabric (∼ 215 g of fabric per m2) to attenuate the UV radiation. Dissolved inorganic nutrients were added to the incubation bottles following the recommendations by Calbet and Saiz (2018) to ensure phytoplankton growth under non-limiting conditions. Assuming moderate phytoplankton growth rates and maximum chlorophyll a concentrations of 2 µg L−1 in the mid-shelf region and 6 µg L−1 in the shelf break front (Delgado et al., 2023), we supplemented the water with 8.3 µM of nitrogen and silicon and 0.5 µM of phosphorus in the mid-shelf area and with 24.9 µM of nitrogen and silicon and 1.5 µM of phosphorus in the shelf break front. A series of triplicate dilution bottles without added nutrients was set as control treatment.
Subsamples from the initial and final treatments were collected for chlorophyll a and bacteria abundance analyses. To determine chlorophyll a, samples (300 mL) were filtered through Whatman GF/F glass fibre filters with 0.7 µm pore size and stored at −20 °C. Pigments were extracted with 90 % acetone for 24 h in the dark at −20 °C and then determined spectrophotometrically according to Jeffrey and Humphrey (1975). Heterotrophic bacteria were quantified by staining 1 mL seawater sample with 4,6-diamidino-2-phenylindole (DAPI) to a final concentration of 3 µg mL−1 and collected on black polycarbonate filters (25 mm diameter, 0.2 µm pore size). The enumeration was done with a Nikon Eclipse 80i microscope equipped with a fluorescence lamp at 100X magnification and using a UV excitation filter (330–385 nm). A total of 25 images were taken at random points from each polycarbonate filter using a Nikon DXM1200F digital camera, and, subsequently, every cell in the image was enumerated and sized using the software ImageJ. Bacterial cell volumes were calculated by assigning simple geometric shapes to species (coccus, bacillus) and converting into carbon content (µg C L−1) by the allometric model according to Simon and Azam (1989).
Plankton samples were collected from the initial treatments to characterize the community structure at the starting conditions. For the analysis of plankton in the 2–5 µm size range, triplicate samples (3 mL) were fixed with 0.53 mL of glutaraldehyde (f.c. 2 %) and subsequently processed following the methods described by Porter and Feig (1980). The same procedure was applied for plankton in the 5–20 µm size range, using duplicate 100 mL samples for counting. The identification of organisms was done by a combination of light and epifluorescent microscopy. Preserved samples were stained with DAPI (f.c. 5 µg mL−1) and proflavine (f.c. 5 µg mL−1) and collected on black polycarbonate filters (25 mm diameter, 0.2 µm pore size). Most taxa were identified using a blue excitation filter (450–490 nm), while cryptophytes were identified using a green excitation filter (480–550 nm). Cell enumeration was done by settling the preserved sample (1–2 mL) in Utermöhl chambers for 24 h. The entire chamber was analysed under a Wild M20 inverted light microscope. Similarly, the enumeration of plankton in the size fraction 20–200 µm was done by settling a variable volume (50–100 mL, depending on sediment and plankton concentration) of preserved water sample (Lugol's iodine) in Utermöhl chambers for 24 h. It is worth mentioning that, since samples were pre-filtered through a 200 µm mesh to exclude larger consumers from our experiments, colony-forming protists may have been removed. Biomass estimation involved assigning simple geometric shapes to species to quantify cell volume, which was subsequently converted into carbon content (µg C L−1) according to Hillebrand et al. (1999). Protistan taxa abundance was visualized by a heatmap (employing the R package heatmaply). Heterotrophic and autotrophic taxa were segmented into groups based on size, distinguishing between nanoplankton (2–20 µm) and microplankton (20–200 µm) (Sieburth et al., 1978). Nanoplankton included heterotrophic nanoplankton (HNP), coccolithophores, and photosynthetic nanoplankton (PNP), while the microplankton comprised ciliates, heterotrophic dinoflagellates (HD), photosynthetic dinoflagellates (PD), and diatoms. Additionally, HNP comprised both flagellates and ciliates, while PNP encompassed dinoflagellates, diatoms, and flagellates. A side dendrogram was included to group similar sampling stations by ordering rows (stations) so that the sum of the distances between each one would be minimized. Data for ranking rows were normalized to range from 0 to 1. To identify the dominant taxa contributing to station ordination, a biplot based on non-metric multi-dimensional scaling (MDS) was created using the R package vegan.
An additional treatment of pre-filtered (Whatman GF/F glass fibre filters with 0.7 µm pore size) and undiluted water was set to separate protists and measure the absorbance at 254 nm and FDOM. This was aimed to compare the DOM pool at the initial and final incubation conditions in the presence (i.e. in unfiltered, undiluted treatment) and in the absence (i.e. in pre-filtered by 0.7 µm, undiluted treatment) of protists. Biotic effects are known to produce DOM transformations on short timescales. For instance, significant shifts in both the chromophoric and fluorescent fractions of DOM driven by biological processes were identifiable after 24 h (Urban-Rich et al., 2004; Lønborg et al., 2010, 2015), while DOM transformation driven by bacteria occurred within the first 24 h upon their release by phytoplankton (Gruber et al., 2006; Hach et al., 2020). The optical properties of FDOM were evaluated from emission–excitation matrices (EEMs) obtained with a Shimadzu RF-5301 scanning spectrofluorometer with a 150 W xenon lamp and a 1 cm quartz cell. Milli-Q water was used as reference, and the intensity of the Raman peak was regularly checked. The emission wavelength ranged between 250 and 600 nm, while the excitation wavelength ranged between 220 and 370 nm. Dissolved humic-like and protein-like substances were estimated using the wavelengths proposed by Coble (1996). Humic-like material was represented by three fluorophores: FDOMC (Ex/Em: 350/440) and FDOMA (Ex/Em: 250/425) of terrestrial origin and FDOMM (Ex/Em: 310/380) of marine origin. FDOMC indicates highly unsaturated components, FDOMA reflects a moderate degree of unsaturation, and FDOMM suggests a low degree of unsaturation. Protein-like material was characterized by two fluorophores, FDOMB (Ex/Em: 260/300) and FDOMT (Ex/Em: 270/330), both derived from autochthonous biogenic sources. FDOMB is associated with compounds similar to tryptophan and tyrosine, linked to recent organic matter production through primary productivity, while FDOMT indicates tryptophan-like compounds formed from microbial protein breakdown (Jørgensen et al., 2011; Drozdova et al., 2022). Fluorescence intensity of fluorophores was expressed in arbitrary units (AU). Fluorophores were identified using the PARAFAC multivariate algorithm (Stedmon and Bro, 2008), and different biogeochemical indicators, such as the humification index (HIX), fluorescence index (FI), and freshness index (BIX), were calculated (Coble, 1996). The HIX serves as a tool for assessing the degree of humification in DOM, which reflects its aromaticity and the extent of diagenetic alteration (Bai et al., 2015), while the FI distinguishes between DOM of different origins, i.e. terrestrial vs. microbial (McKnight et al., 2001). The BIX aims to estimate the relative contribution of DOM produced in situ by microbes (Huguet et al., 2009).
The absorbance spectra between 240 and 800 nm were measured with a PerkinElmer LAMBDA 35 spectrophotometer. The absorbance at 254 nm (a254) from the chromophoric fraction of DOM was used as a proxy of the DOM amount (Brandstetter et al., 1996). Absorbance at 254 nm represents 50 %–60 % of the carbon content in marine DOM (Görs et al., 2007). It primarily includes unsaturated compounds with aromatic rings, conjugated systems, or carbonyl groups, mostly of biological relevance, such as aromatic amino acids, nucleic acids, photosynthetic pigments, and steroids (Martínez-Pérez et al., 2017). As a result, a254 – although not representing all DOC present – can be used as a reliable proxy for the evolution of DOM concentration during incubation experiments. Net changes in the a254 fraction of DOM across the incubation time were calculated as , where (a254)0 and (a254)t are the a254 at the initial (0) and final (t) conditions, respectively. Lee et al. (2018) identified parameters with more than a 50 % absolute percent difference between the control and treated samples as reliable indicators to distinguish between DOM transformation caused by biodegradation, UV irradiance, and adsorption. Here we used the tendency during incubation of BIX, HIX, and FI and the ratio between FDOM components M and A (FDOMFDOMA) as reliable parameters for the discrimination of biodegradation versus UV photodegradation or adsorption. The pairwise relationship between net changes in a254 in the presence and absence of protists with variables of interest was evaluated by simple regression models. The same procedure was used with other variables to test for pairwise relationships of ecological significance.
2.4 Phytoplankton and bacterial growth and grazing by phagotrophic protists
Rate estimates of phytoplankton growth (μ) and mortality due to protist grazing (m) were obtained using the equations of Landry and Hassett (1982). While initially intended for measuring phytoplankton growth and mortality, we adapted this method to assess bacterial growth rate and bacterivory. This approach has been demonstrated to be effective and reliable for use with natural bacterial communities in non-oligotrophic regions (Tremaine and Mills, 1987). The method is based on measuring the initial and final concentration of chlorophyll a (as a proxy of phytoplankton biomass) and bacterial abundance in triplicate dilution series after an incubation period of 24 h. It assumes that protistan grazing rate is a linear function of prey concentration (Holling type I functional response) and can be calculated as follows:
where μ0 is the apparent growth rate; P0 and Pt are the phytoplankton concentration at the initial (0) and final (t) conditions, respectively; and D is the dilution series. The m:μ ratio × 100 was used to calculate the percentage of the daily bacterial/primary productivity consumed by protistan grazers. We tested model fit by linear regression analysis in every experiment.
3.1 Phytoplankton phenological stages at the sampling area
Mean surface chlorophyll a, derived from satellite observations during the sampling period (9–12 October 2017; Fig. 1a), revealed a band of high phytoplankton concentration at the shelf break front, centred at the 114 m isobath in the latitudinal band at 40° S. While the spring bloom typically begins during September in the latitudinal range of our sampling area (Delgado et al., 2023), phytoplankton at the time of sampling, as estimated from satellite chlorophyll a, were at different phenological stages at each station (Fig. 1b). At the mid-shelf, station 21 showed the highest concentration of satellite chlorophyll a and the phytoplankton community was at the pulse initiation. Stations 22 and 23 showed lower chlorophyll a and were sampled at the bloom stationary phase. At the shelf break front, satellite-derived chlorophyll a levels were elevated at stations 13 and 14, indicating proximity to the bloom peak, whereas station 12 exhibited low chlorophyll a concentration, corresponding to the bloom termination phase.
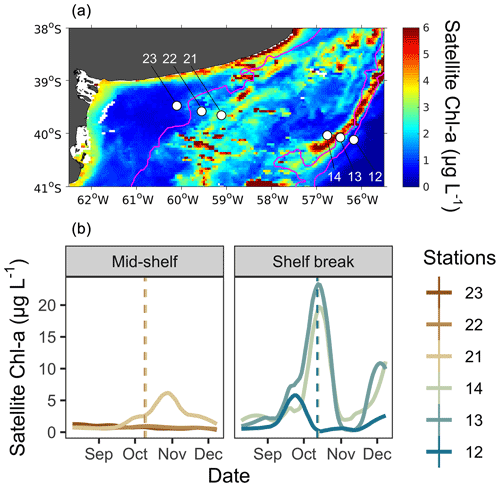
Figure 1(a) Map of the study site showing the locations of sampling stations (white dots) and the mean surface distribution of satellite chlorophyll a (Chl a) during the sampling period (9–12 October). Stations 23, 22, and 21 were located in the mid-shelf area, and stations 14, 13, and 12 were located in the shelf break front. (b) Temporal evolution of surface satellite Chl a concentration at grid points closest to sampling stations in the mid-shelf and in the shelf break front. Solid lines denote satellite Chl a concentration at each station, while dashed lines represent the date of in situ sampling. Lines are colour-coded according to the colours representing each station.
3.2 Hydrography, nutrients, and DOM properties
The thermohaline signature was in the range of the sub-Antarctic shelf waters (33.5<S<34) from station 21 to station 14 in agreement with Berden et al. (2020) and Ferronato et al. (2023). At the mid-shelf area, station 23 and 22 showed relatively higher salinity values (S>33.7), linked to the coastal maximum salinity waters originating at San Matias Gulf (Lucas et al., 2005). These stations also showed weak stratification, while the rest of the stations showed a sharper thermocline. The mixed-layer depth (MLD) was shallower (10 m) at stations 12 and 23, whereas, at all other stations, it averaged 30 m. All samples taken at the chlorophyll a maximum were positioned within the mixed layer.
The concentration of dissolved nutrients (DIN, PO, and SiO) was highest at station 12, while the lowest total nutrient concentration was recorded at station 23 (Fig. 2). The primary nitrogen source was NO, except for station 22, where NH predominated. The only notable distinction between station groups was the concentration of NO, which averaged 1.2 µM at the mid-shelf stations and 7.3 µM at the shelf break front stations. According to Redfield ratios (Redfield et al., 1963), a strong nitrogen depletion in relation to PO and SiO occurred at station 23. While the N:P ratio was closer to 16:1 at the rest of the stations, a general excess of PO in relation to DIN was registered. On the contrary, all stations, except for station 23, showed an SiO depletion in relation to DIN. The concentration of DOC in surface waters was homogeneous at the mid-shelf stations (mean of 79 µM), while, in the shelf break, it varied from 96 µM, at station 14 to 52 µM at station 13 (Fig. 2). The highest fluorescence intensity of protein-like compounds (FDOMT and FDOMB) was found at station 23, while the highest intensity of humic-like fluorophores was observed at station 21 (Fig. 3). The a254 was higher at the mid-shelf stations (mean of 2.3 m−1) compared to the shelf break front stations (mean of 1.3 m−1).
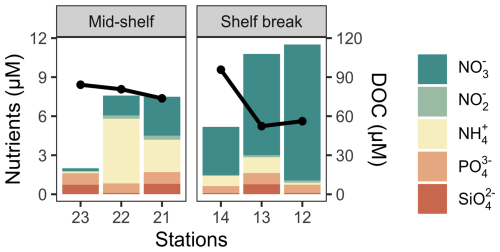
Figure 2Cumulative nutrient concentrations at the deep chlorophyll a maximum (bars) and the concentration of DOC in surface waters (solid black line) across stations in the mid-shelf and in the shelf break front.
3.3 Plankton community structure
The biomass of protistan grazers exceeded that of phytoplankton and heterotrophic bacteria at most sampling stations, except at stations 13 and 14, where phytoplankton biomass dominated (Fig. 4a). Heterotrophic dinoflagellates dominated the biomass across stations, except at station 14, where HNP prevailed. In contrast, no clear distribution pattern was observed among phytoplankton groups. The biomass of heterotrophic bacteria ranged between 2.6 (station 22) and 15 µg C L−1 (station 14) (Fig. 4b). The abundance of this group was positively associated with chlorophyll a concentration (R2=0.7, p=0.04) and with the abundance and biomass of most phytoplankton groups (p<0.05), except coccolithophores. The highest bacterial abundance and biomass were registered under chlorophyll a pulse initiation (stations 21, 13, and 14). Among protistan grazers, the most significant group in terms of biomass was dinoflagellates, ranging from 0 (station 14) to 134 µg C L−1 (station 22; mostly due to the presence of Noctiluca scintillans). Ciliates ranged from 0 (station 14) to 20 µg C L−1 (station 21), while HNP showed the highest biomass at stations 13 and 14 (5 and 6 µg C L−1, respectively) and the lowest value was registered at station 12 (0.6 µg C L−1). Plankton < 5 µm (choanoflagellates and other unidentified flagellates) were the dominant fraction among HNP except at station 13, were micro-sized ciliates and nano-sized flagellates (Telonema sp. and unidentified dinoflagellates) dominated biomass.
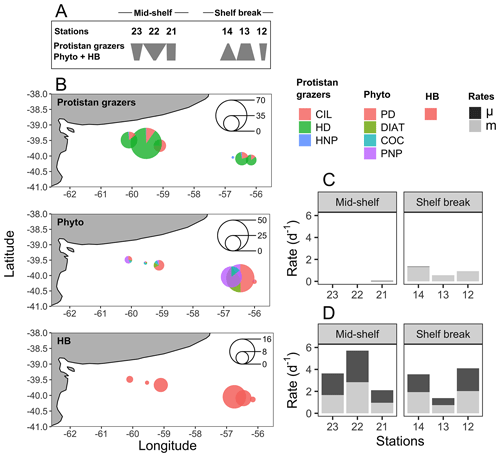
Figure 4(a) Food web structure represented by polygons, illustrating the ranking of carbon biomass for protistan grazers and the total biomass of phytoplankton (phyto) and heterotrophic bacteria (HB) at each sampling station (indicated above the polygons). Inverted or top-heavy pyramids (stations 23, 22, 21, and 12) occur when the biomass of protistan grazers surpasses that of their prey (phytoplankton plus HB). In contrast, the more conventional bottom-heavy structure (stations 14 and 13) is characterized by a greater prey biomass relative to that of consumers. Adapted from Kang et al. (2023). (b) Spatial distribution of the cumulative biomass (µg C L−1) of protistan grazers (upper plot), phytoplankton (mid-plot), and HB (lower plot). Scale circles are shown within each plot. (c) Growth (μ) and grazing (m) rates of phytoplankton. (d) Growth (μ) and grazing (m) rates of HB.
The biomass of photosynthetic taxa was generally dominated by PNP. The highest concentration and biomass of all groups, except for coccolithophores, were registered at station 13 (PNF: 32 µg C L−1; PD: 8 µg C L−1; diatoms: 6 µg C L−1). High biomass of PNP and dinoflagellates was also registered at station 14 (34 µg C L−1) and station 21 (8.5 µg C L−1), respectively. The highest biomass of coccolithophores was registered at station 22 (2 µg C L−1).
Stations 12 and 13 showed differences in microplankton community structure compared to the rest of the stations (Fig. 5). According to the ordination fit between vectors (i.e. taxa) and stations, the protistan grazers that mainly contributed to separating these two stations from the others were the dinoflagellates Gymnodinium spp. and Protoperidinium pellucidum, while Pyramimonas sp. and Dinophysis acuminata were the distinctive photosynthetic species in these stations (MDS; p<0.05). Stations 22 and 23 were also closely associated regarding the protistan grazer community, and the species that contributed most to this association was Strombidinopsis sp.
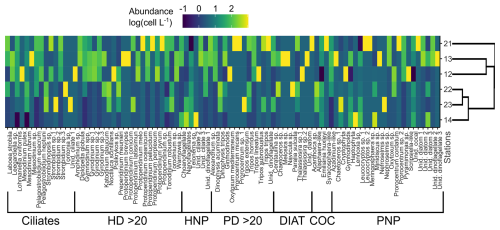
Figure 5Colour-coded, log-transformed cell abundance (cell L−1 × 103) of plankton taxa (columns) at the sampling stations (rows). Group delimitation is indicated at the bottom. The side dendrogram shows the optimal ordering of rows (stations) so that the sum of distances between each one is minimized. HD > 20: heterotrophic dinoflagellates > 20 µm; HNP: heterotrophic nanoplankton; PD > 20: photosynthetic dinoflagellates > 20 µm; Diat: diatoms; Coc: coccolithophores; PNP: photosynthetic nanoplankton.
3.4 Growth and grazing rates
The water temperature of the incubator container was monitored hourly and ranged between 12.2 and 13.8 °C at the mid-shelf stations and between 8 and 11.1 °C at the shelf break stations. Bacteria showed active growth during all experiments, while phytoplankton only revealed significant growth rates under conditions of pulse initiation (stations 21 and 14). Some degree of nutrient limitation was detected in the mid-shelf stations as the growth rate of phytoplankton at the control treatments was lower than in the nutrient-amended treatment; however, differences were not statistically significant. No apparent differences were found at stations 12, 13, and 14. A significant grazing effect on bacteria was found in all experiments (Fig. 4c), while grazing on phytoplankton was only significant at the shelf break stations (Fig. 4d). At the mid-shelf stations, daily bacterial productivity consumed by HNP averaged 72 %, while, at the shelf break stations, it reached 83 %. Mean daily primary productivity consumed by protistan grazers was zero at the mid-shelf stations and 155 % at the shelf break stations. Linear responses were found in all experiments, indicating that no cascading effects, saturating feeding, or starvation occurred within incubation bottles. The abundance of heterotrophic bacteria was negatively correlated with the grazing of HNP (R2=0.8, p=0.016) and growth (R2=0.9, p=0.005).
3.5 Short-term DOM transformations
An accumulation of the a254 DOM fraction was observed at stations 22, 14, 13, and 12 during the incubation period in the experimental setting with protists, while stations 23 and 21 exhibited DOM consumption (Fig. 6a). Conversely, in the experimental setting without protists, the a254 DOM fraction accumulated at stations 22, 21, 14, and 12, while stations 23 and 13 showed DOM consumption (Fig. 7a). Regardless of net DOM production, biodegradation of organic matter occurred in most experiments as denoted by the decrease in the BIX and the increase in the HIX (Figs. 6b, 7b). The prevalence of biodegradation over UV photodegradation or adsorption was further supported by most source discrimination indices (FI and FDOMM/FDOMA; data not shown). The decrease in BIX was coherent with the net-zero to low phytoplankton growth during our experiments. An exception to this general pattern occurred at station 13, in which HIX increased and BIX decreased during the incubation. Station 13 was characterized by the highest abundance of micro-sized phytoplankton (diatoms and photosynthetic dinoflagellates) and registered the lowest concentration of DOC (52 µM) at the moment of sampling. While HIX and BIX indices suggested that DOM modifications are not driven by biodegradation, FDOMM/FDOMA decreased and FI increased during the experiments, thus giving inconsistent results.
Among treatments, a shift from accumulation to consumption of the a254 DOM fraction was registered at stations 21 and 13. In the presence of protists, the net a254 fraction of DOM change was negatively associated with the fluorescence intensity of peaks related to humic-like compounds (FDOMC: R2=0.6, p=0.07; FDOMA: R2=0.8, p=0.01; FDOMM: R2=0.7, p=0.04) and was positively associated with the grazing on HB (R2=0.7, p=0.00; Fig. 8a). In the absence of protists, the net a254 fraction of DOM change was negatively associated with the ratio between bacteria and phytoplankton biomass (R2=0.8, p=0.01; Fig. 8b).
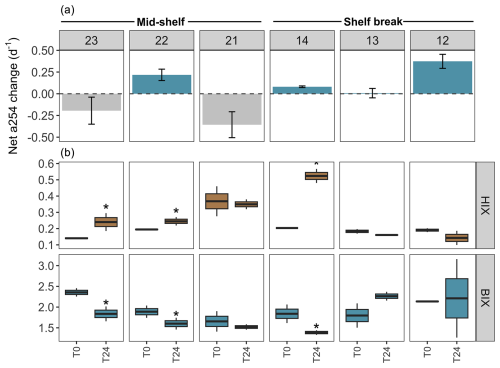
Figure 6DOM transformations in the experimental setting with protists (pre-filtered by 200 µm) across stations in the mid-shelf and the shelf break front. (a) Net changes in the absorbance at 254 nm (a254) from the chromophoric fraction of DOM, a proxy of total DOM concentration. The dashed line indicates the limit between negative (i.e. consumption) and positive (i.e. accumulation) net a254 changes during the incubation period. (b) Shifts in the humification index (HIX) and biological activity index (BIX) during the 24 h incubation. Asterisks indicate significant differences identified by linear regression analysis between initial and final treatments (p<0.05).
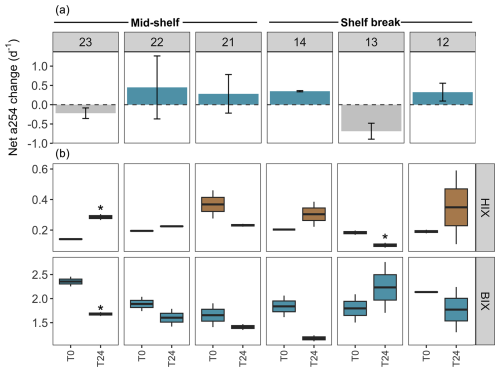
Figure 7DOM transformations in the experimental setting without protists (pre-filtered by 0.7 µm) across stations in the mid-shelf and the shelf break front. (a) Net changes in the absorbance at 254 nm (a254) from the chromophoric fraction of DOM, a proxy of total DOM concentration. The dashed line indicates the limit between negative (i.e. consumption) and positive (i.e. accumulation) net a254 changes during the incubation period. (b) Shift in the humification index (HIX) and biological activity index (BIX) during the 24 h incubation. Asterisks indicate significant differences identified by linear regression analysis between initial and final treatments (p<0.05).
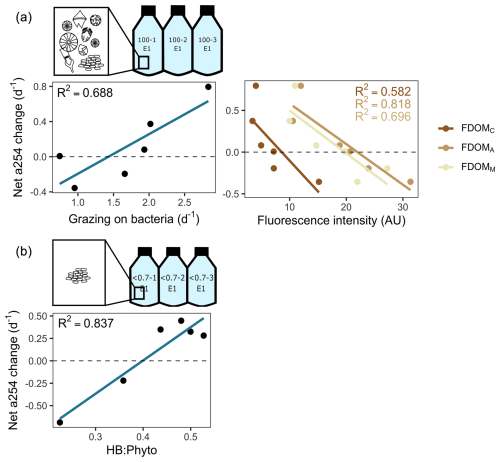
Figure 8Main predictors of the net changes in the absorbance at 254 nm (a254) from the chromophoric fraction of DOM during incubations. (a) Experimental setting with protists. Linear regression plots depict the relationship between net a254 changes and grazing on bacteria and humic-like substances (as depicted by fluorophores FDOMC, FDOMA, and FDOMM). (b) Experimental setting without protists. The linear regression plot depicts the relationship between net a245 changes and the ratio between heterotrophic bacteria (HB) and phytoplankton (Phyto) biomass. Dashed lines indicate the limit between negative (i.e. consumption) and positive (i.e. accumulation) net a254 changes.
4.1 Microbial food web structure
Plankton composition and distribution varied notably across blooming and non-blooming stations, with station 14 standing out for its atypical plankton structure within a bloom region. Dominated by a nanoplanktonic diatom and a small grazer community, this station also showed high bacterial biomass, likely due to low PO and SiO concentrations, but high DOC levels. Nanoplankton, particularly diatoms, cryptophytes, and haptophytes, were predominant across sampling stations, with cyanobacteria assumed to play a significant role in carbon fixation. Similar findings were reported in previous studies by Silva et al. (2009) and Negri et al. (2013, 2016), indicating the widespread dominance of plankton < 5 µm in the region. The contrast in chlorophyll a concentration between the upwelling and the mid-shelf areas may be related to nitrate content, which is the main limiting nutrient on the Patagonian Shelf (Paparazzo et al., 2010). The shelf break front receives a continuous supply from the nutrient-rich upwelling of the Malvinas Current, while nitrate on the mid-shelf is mainly provided by the northward flow of sub-Antarctic waters. However, as it moves north, phytoplankton uptake depletes nitrate, leading to lower concentrations on the northern Patagonian Shelf (Song et al., 2016). This creates a regional contrast in nutrient levels between the shelf and the shelf break.
In the shelf break front, dinoflagellates dominated both photosynthetic and heterotrophic biomass, while ciliates were more abundant in the mid-shelf. Beside these spatial differences, the lack of a clear distribution pattern of plankton highlights the impact of mesoscale structures on spatial plankton heterogeneity (Lehahn et al., 2018), a feature proper of the Patagonian Shelf (Saraceno et al., 2024). Heterotrophic bacterial abundance was comparable to coastal summer values reported by Hozbor et al. (2013), Negri et al. (2016), and Garzón-Cardona et al. (2021). It exhibited a positive correlation with chlorophyll a but was negatively affected by HNP grazing. This dynamic suggests that bacterial populations are primarily regulated by grazing pressure and the availability of phytoplankton-derived DOM rather than by nutrient limitation during periods of high productivity.
4.2 Microbial trophic pathways
In oligotrophic areas, microbial food webs involve multi-step carbon transfer dominated by small organisms that efficiently recycle nutrients. By contrast, productive regions have shorter, more efficient food webs where carbon moves directly to higher trophic levels (Armengol et al., 2019). Our observations showed strong microbial trophic coupling across the mid-shelf and the shelf break front; however, under more productive conditions, a fraction of primary producers escaped grazing. Mid-shelf stations, including post-bloom station 12, exhibited an “inverted pyramid” biomass structure, where consumer biomass exceeded that of its prey, suggesting high trophic efficiency, as shown in former studies (McCauley et al., 2018). In the shelf break front, despite evidence of protistan herbivory, much of the phytoplankton biomass remained ungrazed, likely due to strong top-down pressure from microcrustaceans on both phytoplankton and protistan grazers. This created a more typical bottom-heavy biomass structure, indicative of high primary productivity, where some primary production bypasses protist predation and is consumed by microcrustaceans or exported. Such a food web configuration achieves the highest carbon biomass, regardless of community composition (Kang et al., 2023). These observations align with previous findings in the shelf region, showing strong trophic coupling and minimal sinking of unused biomass, dominated by small phytoplankton (<5 µm) and balanced biomass levels of micro- and mesozooplankton (Negri et al., 2013).
Our findings showed active growth of heterotrophic bacteria across all stations, while phytoplankton exhibited low net growth or biomass yield, except at two stations undergoing developing bloom stages (14 and 21). Heterotrophic bacteria, despite having half the biomass of phytoplankton, was selectively preyed by protistan grazers, accounting for 72 % of bacterial production in mid-shelf stations and 83 % in the shelf break front (Fig. 9). This preference for bacteria likely stems from their higher growth rate compared to phytoplankton, as protistan grazing is primarily triggered by prey growth rate rather than biomass (Banse, 1982; Calbet and Landry, 2004; Chen et al., 2009). Regarding the low phytoplankton growth rates observed in this study, an additional factor beyond bloom timing may involve UV photoinhibition, which can affect some eukaryotic pigmented plankton when exposed to deck conditions. Although light conditions were carefully controlled during handling and the incubator was covered with a special net to prevent overexposure, continuous light exposure cannot fully replicate the natural mitigation of UV stress provided by vertical mixing in the water column (Häder et al., 2015). Laws et al. (2000) noted unrealistic growth estimates, attributing the negative effects of UV exposure to phytoplankton incubation at the surface. Simulating natural UV exposure remains a common challenge in most incubation experiments, where communities experience more stable light conditions.
Our results showed that bacteria exhibited growth advantages over phytoplankton across the examined environmental gradient but were concurrently more vulnerable to grazing pressure. This compensatory grazing on fast-growing bacteria has previously been observed in productive environments such as the California Current (Goericke, 2011; Taylor and Landry, 2018; Landry et al., 2023) and the oligotrophic Taiwan Warm Current (Chiang et al., 2014). The suggested mechanism underlying this trophic interaction posits that increased phytoplankton–DOM production fosters the growth of resource-efficient bacteria, as suggested by the tight coupling between bacteria and phytoplankton biomass. However, the heightened growth, coupled with a diminished allocation of energy to defensive skills, renders these bacteria susceptible to selective grazing (Landry et al., 2023).
The situation in the more productive shelf break front shifted toward a coupled predation upon both prokaryotes and phytoplankton (Fig. 9). Grazing accounted for 83 % of bacterial production and 154 % of phytoplankton production. Despite limited growth, phytoplankton faced substantial grazing pressure, possibly facilitated by shared predators with bacteria. This trophic interaction aligns with the enhanced microbial loop hypothesis (Taylor and Landry, 2018), suggesting that small phytoplankton are increasingly grazed as a byproduct of grazers actively preying on bacteria under conditions of rising productivity. Under this scenario, grazing on picophytoplankton is density-independent and occurs due to the presence of shared common grazers with bacteria. Overall, bacteria appeared to be positively regulated by commensalism with phytoplankton and negatively regulated by grazing, constituting a primary carbon source for protistan grazers regardless of the productivity level.
An additional factor contributing to grazing selectivity across productivity areas may be related to grazer community composition, which differed between the mid-shelf and the shelf break front, with ciliates more concentrated on the mid-shelf and heterotrophic dinoflagellates more associated with shelf break stations (especially at stations 12 and 13). In contrast, phytoplankton structure showed no distinct regional differences. Ciliates primarily select prey based on size, as their oral diameter limits ingestion. They can thrive on a diet composed exclusively of prey of uniform size, such as bacteria. In contrast, dinoflagellates employ diverse feeding strategies, allowing them access to a broader range of prey sizes. This trait positions dinoflagellates as key grazers in mesotrophic and eutrophic environments, such as upwelling areas (e.g. Hansen et al., 1997; Sherr and Sherr, 2007; Calbet, 2008), enabling them to effectively target both bacteria and larger phytoplankton.
4.3 Short-term DOM pathways
Throughout the experiments, substantial bacterial proliferation and minimal or no phytoplankton growth were observed to occur alongside the active biodegradation of organic matter, as evidenced by a decrease in the biological index (BIX) and an increase in the humification index (HIX). The general decreasing trend in BIX during the incubation and its similar behaviour in the absence of protist confirms a bacterial effect and suggests that bacteria are rapidly consuming recently produced phytoplankton exudates. Comparable observations have shown that bacteria produce humic-like substances from protistan plankton precursors in controlled environments absent of terrestrial influence (Gruber et al., 2006; Romera-Castillo et al., 2011; Lechtenfeld et al., 2015; Kinsey et al., 2018; Osburn et al., 2019), underscoring the pivotal role of microbial communities in converting DOM into refractory substances over brief timescales. The described carbon route partially explains why the region acts as a carbon sink throughout most of the year (Kahl et al., 2017), irrespective of the magnitude of primary productivity, and emphasizes the critical role of the microbial carbon pump (Jiao et al., 2010) as a key carbon sequestration pathway on the northern Patagonian Shelf. The moderate to high DOC concentration compared to other open waters (Hansell et al., 2009) appears to support sustained bacteria growth. While these findings highlight its importance, the seasonal relevance of this mechanism remains to be explored.
The accumulation of a fraction of DOM, as indicated by a254, was not limited to stations experiencing active phytoplankton growth, indicating that net DOM release is not necessarily tied to specific phenological stages, as previously suggested (Bachi et al., 2023). Moreover, accumulation occurred under diverse phytoplankton assemblages, implying a low level of specialization in the bacterial utilization of species-specific DOM substances, a trait that becomes apparent under conditions of high resource availability (Sarmento et al., 2016). It is worth noting that, while a254 is a reliable proxy for DOM quantity, representing 50 %–60 % of the carbon content, it primarily reflects aromatic compounds, which dominate humic substances in DOM. In contrast, aliphatic compounds contribute to a lesser extent. As a result, experimental results may overlook the production of simpler compounds.
The complexity of DOM, reflected by the prevalence of humic-like compounds (FDOMC, FDOMA, and FDOMM), emerged as a key factor influencing the bacterial utilization of DOM. A net consumption of the DOM fraction, as estimated by a254, was observed when the initial DOM pool contained a significant proportion of low-reactivity compounds, influenced by the phenological status of the sampling site, as indicated by satellite chlorophyll a trends. However, while stations 21 and 23 had high proportions of refractory compounds, they differed in labile compound content, leading to distinct experimental outcomes. Both stations had low pre-sampling chlorophyll a, indicating limited recent autochthonous DOM production, yet showed contrasting bacterial activity. At station 23, high bacterial activity increased DOM complexity and net consumption under both treatments, suggesting dependence on an initial labile DOM pool (FDOMT). In contrast, station 21 had low bacterial activity, with the a254 DOM fraction shifting from consumption to accumulation without protists, indicating bacterial reliance on phytoplankton DOM exudates when the DOM pool was predominantly refractory.
At station 13, the opposite pattern was observed, with the a254 DOM fraction shifting from weak accumulation in the presence of protists to consumption in the absence of protists. Here, a higher proportion of labile DOM facilitated bacterial activity. Low bacteria-to-phytoplankton biomass ratios indicated that bacterial DOM consumption was masked by phytoplankton biomass but became evident without protists. That is, at stations where bacterial biomass is low relative to phytoplankton, bacteria appear to rely more on phytoplankton-derived DOM than on other sources. This effect became apparent upon the removal of protists, implying the absence of newly produced DOM (Fig. 8b), in which a net a254 DOM fraction consumption was observed. Overall, the initial proportion of refractory compounds better predicted the net DOM production by providing insights on microbial succession trajectories. While changes in DOM production and consumption in protist-free bottles were mainly driven by heterotrophic bacteria, filtration likely allowed cyanobacteria and viruses to pass through, contributing additional DOM. Synechococcus, the dominant cyanobacterium in the Patagonian Shelf, releases bioavailable DOM that stimulates bacterial activity (Wang et al., 2022). Viral lysis also converts microbial biomass into DOM (Chen et al., 2022). These overlooked sources likely influenced the short-term DOM dynamics in the incubation bottles.
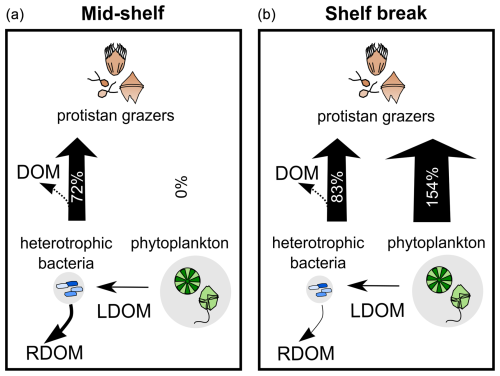
Figure 9Schematic representation of DOM pathways during the productive season on the Patagonian Shelf, contrasting the mid-shelf (low to moderate productivity) (a) with the shelf break front (high productivity) (b). Phytoplankton biomass (background circle size) was twice that of bacteria, with selective grazing on bacteria balancing their rapid growth across sampling areas. Grazing on phytoplankton increased under higher productivity, aligning with the enhanced microbial loop hypothesis. Heterotrophic bacteria were key in shaping DOM quality, utilizing labile DOM (LDOM) from phytoplankton and contributing to the storage of refractory DOM (RDOM), even without DOM-producing protists. RDOM production was more pronounced mid-shelf than at the shelf break. Bacterial grazing influenced the net production of the a254 DOM fraction, with intense grazing leading to DOM accumulation likely through reduced bacterial biomass and egested organic material. Arrow thickness represents grazing pressure, with percentages indicating daily grazed productivity.
The a254 DOM fraction also tended to accumulate under high bacterial mortality due to grazing. Our results evidenced a selective grazing on bacteria compensating for their fast growth rate. This group-specific grazing mortality aligns well with the grazing selectivity model, wherein grazers exhibit preferences against high-growth-rate organisms, establishing a tight coupling between growth advantages and grazing vulnerability across environmental gradients (Landry et al., 2023). Our experimental data not only support this hypothesis but also provide new insights into the repercussions of bacterial grazing on dissolved carbon stocks (Fig. 9). Specifically, our results suggest that grazing on bacteria can lead to an accumulation of DOM produced by phytoplankton by reducing the biomass of bacterial standing stock. In addition, protistan grazing may contribute to the DOM pool by releasing bacterial carbon (Taylor et al., 1985). However, bacteria may not immediately utilize this DOM source, as adapting their enzymatic machinery to target new compounds requires additional energy expenditure, resulting in less efficient resource utilization (Baña et al., 2014). Similar results were observed in polar waters, where high protistan bacterivory was associated with DOM accumulation (Lund Paulsen et al., 2019). Our observations carry biogeochemical implications, as intense bacterial grazing implies that bacterial biomass becomes available to higher trophic levels, thereby circumventing the DOM cycle. In other words, while most bacterial biomass is directed by protistan grazers toward higher trophic levels, it also partially diverts the production of DOM lysates by viral lysis (Suttle, 2005). Our experimental results indicate that the diversion of bacterial carbon from the DOM cycle by protistan grazing is only partial, as protistan grazing introduces additional DOM, while the reduction in bacterial abundance leaves a fraction of phytoplankton-derived DOM unutilized over short timescales.
Protistan grazing is widely recognized as the primary driver of phytoplankton mortality (Calbet and Landry, 2004) and is an important mortality source for heterotrophic bacteria (Weinbauer and Peduzzi, 1995). However, viral lysis can achieve comparable mortality rates in both phytoplankton and bacterial populations to those caused by protistan grazers. Discerning the viral impact within incubation bottles can be challenging, as viral activity may not only contribute to cell lysis but has also been observed to stimulate prey availability for protists, potentially through the release of cellular lysates or by reducing competition (Staniewski and Short, 2014). In our study, while protist grazing was considered the primary cause of bacterial and phytoplankton mortality, viral lysis may have also played an unrecognized but significant role in dilution experiments.
The fate of grazing-derived DOM remains uncertain in our experimental setup, as it could either serve as a potential source for bacterial utilization, thus establishing positive longer-term predator–prey feedback not captured in our 24 h experiment, or contribute to the complex DOM pool, feeding into the refractory fraction. Indeed, previous observations revealed that DOM derived from protistan grazers varies in its bioavailability (Taylor et al., 1985), and their complexity is further shaped by taxa composition (Nagata and Kirchman, 1992; Gruber et al., 2006). In our experiments, we did not observe a clear trend in DOM transformation between treatments with and without protists, indicating that bacteria remain the primary drivers of DOM quality. Overall, our findings revealed that, under the high bacterial growth rate that follows the onset of the productive season, protistan grazers not only channel carbon through remineralization but also foster the degree of DOM accumulation by reducing DOM-degrading bacterial stock and/or contributing with egestion substances. Additionally, instead of acting solely as a sink of carbon through mineralization of organic compounds, bacteria serve as a crucial link between assimilatory CO2 and higher trophic levels.
The data sets (López-Abbate et al., 2024) are open access under Attribution 4.0 International and available on Zenodo at https://doi.org/10.5281/zenodo.11662262.
MCLA conceptualized, designed, and carried out the experiments; analysed plankton samples; and acquired funding. JEGC, AMM, and ASG analysed DOC, optical properties of DOM, and nutrient samples and interpreted the results. JEGC performed the PARAFAC multivariate algorithm and calculated fluorescence indices. RS analysed plankton samples and performed biomass calculations. JCM contributed to the numerical methodology design and the conceptualization of overarching goals. LARE analysed and interpreted CTD data and Moderate Resolution Imaging Spectroradiometer (MODIS) Aqua images of chlorophyll a. RJL coordinated responsibilities for the planning and execution of research activities, acquired funding, and contributed to the conceptualization of overarching goals. MCLA prepared the article with contributions from all co-authors.
The contact author has declared that none of the authors has any competing interests.
Publisher’s note: Copernicus Publications remains neutral with regard to jurisdictional claims made in the text, published maps, institutional affiliations, or any other geographical representation in this paper. While Copernicus Publications makes every effort to include appropriate place names, the final responsibility lies with the authors.
We are thankful to the crew of the RV Dr. Bernardo Houssay of Prefectura Naval Argentina for their support in field activities and sampling. The authors also wish to thank the anonymous reviewers for their insightful comments on this article. This study was supported by the Argentine Oceanographic Institute (IADO, CONICET-UNS). The authors acknowledge the use of ChatGPT for language and spelling improvements (powered by OpenAI's language model, GPT-3; http://openai.com, last access: July 2024) in an earlier draft of this article.
This research has been supported by the Fondo para la Investigación Científica y Tecnológica (grant nos. PICT 0467–2010 and PICT 2386-2017) and the Consejo Nacional de Investigaciones Científicas y Técnicas (grant no. PIP 11220200102681CO).
This paper was edited by Yuan Shen and reviewed by three anonymous referees.
Armengol, L., Calbet, A., Franchy, G., Rodríguez-Santos, A., and Hernández-León, S.: Planktonic food web structure and trophic transfer efficiency along a productivity gradient in the tropical and subtropical Atlantic Ocean, Sci. Rep., 9, 2044, https://doi.org/10.1038/s41598-019-38507-9, 2019.
Bachi, G., Morelli, E., Gonnelli, M., Balestra, C., Casotti, R., Evangelista, V., Repeta, D. J., and Santinelli, C.: Fluorescent properties of marine phytoplankton exudates and lability to marine heterotrophic prokaryotes degradation, Limnol. Oceanogr., 68, 982–1000, https://doi.org/10.1002/lno.12325, 2023.
Bai, Y., Su, R., Han, X., Zhang, C., and Shi, X.: Investigation of seasonal variability of CDOM fluorescence in the southern Changjiang river estuary by EEM-PARAFAC, Acta Oceanol. Sin., 34, 1–12, https://doi.org/10.1007/s13131-015-0714-8, 2015.
Baltar, F., Palovaara, J., Unrein, F., Catala, P., Horňák, K., Šimek, K., Vaqué, D., Massana, R., Gasol, J. M., and Pinhassi, J.: Marine bacterial community structure resilience to changes in protist predation under phytoplankton bloom conditions, ISME J., 10, 568–581, https://doi.org/10.1038/ismej.2015.135, 2016.
Baña, Z., Ayo, B., Marrasé, C., Gasol, J. M., and Iriberri, J.: Changes in bacterial metabolism as a response to dissolved organic matter modification during protozoan grazing in coastal Cantabrian and Mediterranean waters, Environ. Microbiol., 16, 498–511, https://doi.org/10.1111/1462-2920.12274, 2014.
Banse, K.: Cell volumes, maximal growth rates of unicellular algae and ciliates, and the role of ciliates in the marine pelagial, Limnol. Oceanogr., 27, 1059–1071, https://doi.org/10.4319/lo.1982.27.6.1059, 1982.
Behrenfeld, M. J. and Boss, E. S.: Student's tutorial on bloom hypotheses in the context of phytoplankton annual cycles, Glob. Change Biol., 24, 55–77, https://doi.org/10.1111/gcb.13858, 2018.
Berden, G., Charo, M., Möller, O. O., and Piola, A. R.: Circulation and Hydrography in the Western South Atlantic Shelf and Export to the Deep Adjacent Ocean: 30° S to 40° S, J. Geophys. Res.-Oceans, 125, e2020JC016500, https://doi.org/10.1029/2020JC016500, 2020.
Berglund, J., Müren, U., Båmstedt, U., and Andersson, A.: Efficiency of a phytoplankton-based and a bacterial-based food web in a pelagic marine system, Limnol. Oceanogr., 52, 121–131, https://doi.org/10.4319/lo.2007.52.1.0121, 2007.
Brandstetter, A., Sletten, R. S., Mentler, A., and Wenzel, W. W.: Estimating dissolved organic carbon in natural waters by UV absorbance (254 nm), Z. Pflanz. Bodenkunde, 159, 605–607, https://doi.org/10.1002/jpln.1996.3581590612, 1996.
Calbet, A.: The trophic roles of microzooplankton in marine systems, ICES J., 65, 325–331, https://doi.org/10.1093/icesjms/fsn013, 2008.
Calbet, A. and Landry, M. R.: Phytoplankton growth, microzooplankton grazing, and carbon cycling in marine systems, Limnol. Oceanogr.-Meth., 49, 51–57, https://doi.org/10.4319/lo.2004.49.1.0051, 2004.
Calbet, A. and Saiz, E.: How much is enough for nutrients in microzooplankton dilution grazing experiments?, J. Plankton Res., 40, 109–117, https://doi.org/10.1093/plankt/fbx070, 2018.
Chen, B., Liu, H., Landry, M. R., DaI, M., Huang, B., and Sune, J.: Close coupling between phytoplankton growth and microzooplankton grazing in the western South China Sea, Limnol. Oceanogr., 54, 1084–1097, https://doi.org/10.4319/lo.2009.54.4.1084, 2009.
Chen, X., Wei, W., Xiao, X., Wallace, D., Hu, C., Zhang, L., Batt, J., Liu, J., Gonsior, M., Zhang, J., LaRoche, J., Hill, P., Xu, D., Wang, J., Jiao, N., and Zhang, R.: Heterogeneous viral contribution to dissolved organic matter processing in a long-term macrocosm experiment, Environ. Int., 158, 106950, https://doi.org/10.1016/j.envint.2021.106950, 2022.
Chiang, K.-P., Tsai, A.-Y., Tsai, P.-J., Gong, G.-C., Huang, B.-Q., and Tsai, S.-F.: The influence of nanoflagellates on the spatial variety of picoplankton and the carbon flow of the microbial food web in the oligotrophic subtropical pelagic continental shelf ecosystem, Cont. Shelf Res., 80, 57–66, https://doi.org/10.1016/j.csr.2014.02.019, 2014.
Coble, P. G.: Characterization of marine and terrestrial DOM in seawater using excitation-emission matrix spectroscopy, Mar. Chem., 51, 325–346, https://doi.org/10.1016/0304-4203(95)00062-3, 1996.
Delgado, A. L., Hernández-Carrasco, I., Combes, V., Font-Muñoz, J., Pratolongo, P. D., and Basterretxea, G.: Patterns and Trends in Chlorophyll-a Concentration and Phytoplankton Phenology in the Biogeographical Regions of Southwestern Atlantic, J. Geophys. Res.-Oceans, 128, e2023JC019865, https://doi.org/10.1029/2023JC019865, 2023.
Drozdova, A. N., Krylov, I. N., Nedospasov, A. A., Arashkevich, E. G., and Labutin, T. A.: Fluorescent signatures of autochthonous dissolved organic matter production in Siberian shelf seas, Front. Mar. Sci., 9, 872557, https://https://doi.org/10.3389/fmars.2022.872557, 2022.
Ferronato, C., Berden, G., Rivarossa, M., and Guinder, V. A.: Wind-driven currents and water masses shape spring phytoplankton distribution and composition in hydrologically complex, productive shelf waters, Limnol. Oceanogr., 68, 2195–2210, https://doi.org/10.1002/lno.12413, 2023.
Garzón-Cardona, J. E., Guinder, V. A., Alonso, C., Martínez, A. M., Pantoja-Gutiérrez, S., Kopprio, G. A., Krock, B., and Lara, R. J.: Chemically unidentified dissolved organic carbon: A pivotal piece for microbial activity in a productive area of the Northern Patagonian shelf, Mar. Environ. Res., 167, 105286, https://doi.org/10.1016/j.marenvres.2021.105286, 2021.
Goericke, R.: The structure of marine phytoplankton communities-patterns, rules and mechanisms, Cal. Coop. Ocean. Fish., 52, 182–197, 2011.
Görs, S., Rentsch, D., Schiewer, U., Karsten, U., and Schumann, R.: Dissolved organic matter along the eutrophication gradient of the Darβ–Zingst Bodden Chain, Southern Baltic Sea: I. Chemical characterisation and composition, Mar. Chem., 104, 125–142, https://doi.org/10.1016/j.marchem.2006.10.009, 2007.
Gruber, D. F., Simjouw, J.-P., Seitzinger, S. P., and Taghon, G. L.: Dynamics and characterization of refractory dissolved organic matter produced by a pure bacterial culture in an experimental predator-prey system, Appl Environ. Microbiol., 72, 4184–4191, https://doi.org/10.1128/AEM.02882-05, 2006.
Hach, P. F., Marchant, H. K., Krupke, A., Riedel, T., Meier, D. V., Lavik, G., Holtappels, M., Dittmar, T., and Kuypers, M. M. M.: Rapid microbial diversification of dissolved organic matter in oceanic surface waters leads to carbon sequestration, Sci. Rep., 10, 13025, https://doi.org/10.1038/s41598-020-69930-y, 2020.
Häder, D. P., Williamson, C. E., Wängberg, S. Å., Rautio, M., Rose, K. C., Gao, K., Helbling, W. E., Sinha, R. P., and Worrest, R.: Effects of UV radiation on aquatic ecosystems and interactions with other environmental factors, Photochem. Photobiol. Sci., 14, 108–126, https://https://doi.org/10.1039/c4pp90035a, 2015.
Hansell, D. A., Carlson, C. A., Repeta, D. J., and Schlitzer, R.: Dissolved organic matter in the ocean: A controversy stimulates new insights, Oceanography, 22, 202–211, https://doi.org/10.5670/oceanog.2009.109, 2009.
Hansen, P. J., Bjørnsen, P. K., and Hansen, B. W.: Zooplankton grazing and growth: Scaling within the 2–2,000-µm body size range, Limnol. Oceanogr., 42, 687–704, https://doi.org/10.4319/lo.1997.42.4.0687, 1997.
Hillebrand, H., Dürselen, C., Kirschtel, D., Pollingher, U., and Zohary, T.: Biovolume calculation for pelagic and benthic microalgae, J. Phycol., 35, 403–424, https://doi.org/10.1046/j.1529-8817.1999.3520403.x, 1999.
Hozbor, M. C., Hernández, D. R., Cucchi Colleoni, A. D., Costagliola, M. C., and Peressutti, S. R.: Biomasa y distribución espacial del bacterioplancton en el sector norte de la plataforma continental argentina (34° S–41° S), Revista de Investigación y Desarrollo Pesquero, 23, 145–160, 2013.
Huguet, A., Vacher, L., Relexans, S., Saubusse, S., Froidefond, J. M., and Parlanti, E.: Properties of fluorescent dissolved organic matter in the Gironde Estuary, Org. Geochem., 40, 706–719, https://doi.org/10.1016/j.orggeochem.2009.03.002, 2009.
Irigoien, X., Flynn, K. J., and Harris, R. P.: Phytoplankton blooms: a `loophole' in microzooplankton grazing impact?, J. Plankton Res., 27, 313–321, https://doi.org/10.1093/plankt/fbi011, 2005.
Jeffrey, S. W. and Humphrey, G. F.: New spectrophotometric equations for determining chlorophylls a, b, c1 and c2 in higher plants, algae and natural phytoplankton, Biochem. Physiol. Pfl., 167, 191–194, https://doi.org/10.1016/S0015-3796(17)30778-3, 1975.
Jiao, N., Herndl, G. J., Hansell, D. A., Benner, R., Kattner, G., Wilhelm, S. W., Kirchman, D. L., Weinbauer, M. G., Luo, T., Chen, F., and Azam, F.: Microbial production of recalcitrant dissolved organic matter: long-term carbon storage in the global ocean, Nat. Rev. Microbiol., 8, 593–599, https://doi.org/10.1038/nrmicro2386, 2010.
Jørgensen, L., Stedmon, C. A., Kragh, T., Markager, S., Middelboe, M., and Søndergaard, M.: Global trends in the fluorescence characteristics and distribution of marine dissolved organic matter, Mar. Chem., 126, 139–148, https://doi.org/10.1016/j.marchem.2011.05.002, 2011.
Kahl, L. C., Bianchi, A. A., Osiroff, A. P., Pino, D. R., and Piola, A. R.: Distribution of sea-air CO2 fluxes in the Patagonian Sea: Seasonal, biological and thermal effects, Cont. Shelf Res., 143, 18–28, https://doi.org/10.1016/j.csr.2017.05.011, 2017.
Kanayama, T., Kobari, T., Suzuki, K., Yoshie, N., Honma, T., Karu, F., and Kume, G.: Impact of microzooplankton grazing on the phytoplankton community in the Kuroshio of the East China sea: A major trophic pathway of the Kuroshio ecosystem, Deep-Sea Res. I, 163, 103337, https://doi.org/10.1016/j.dsr.2020.103337, 2020.
Kang, H. C., Jeong, H. J., Ok, J. H., Lim, A. S., Lee, K., You, J. H., Park, S. A., Eom, S. H., Lee, S. Y., Lee, K. H., Jang, S. H., Yoo, Y. Du, Lee, M. J., and Kim, K. Y.: Food web structure for high carbon retention in marine plankton communities, Sci. Adv., 9, eadk0842, https://doi.org/10.1126/sciadv.adk0842, 2023.
Kattner, G. and Becker, H.: Nutrients and organic nitrogenous compounds in the marginal ice zone of the Fram Strait, J. Mar. Syst., 2, 385–394, https://doi.org/10.1016/0924-7963(91)90043-T, 1991.
Kinsey, J. D., Corradino, G., Ziervogel, K., Schnetzer, A., and Osburn, C. L.: Formation of chromophoric dissolved organic matter by bacterial degradation of phytoplankton-derived aggregates, Front. Mar. Sci., 4, https://doi.org/10.3389/fmars.2017.00430, 2018.
Kujawinski, E. B., Del Vecchio, R., Blough, N. V., Klein, G. C., and Marshall, A. G.: Probing molecular-level transformations of dissolved organic matter: insights on photochemical degradation and protozoan modification of DOM from electrospray ionization Fourier transform ion cyclotron resonance mass spectrometry, Mar. Chem., 92, 23–37, https://doi.org/10.1016/j.marchem.2004.06.038, 2004.
Landry, M. R. and Hassett, R. P.: Estimating the grazing impact of marine micro-zooplankton, Mar. Biol., 67, 283–288, https://doi.org/10.1007/BF00397668, 1982.
Landry, M. R., Stukel, M. R., Selph, K. E., and Goericke, R.: Coexisting picoplankton experience different relative grazing pressures across an ocean productivity gradient, P. Natl. Acad. Sci. USA, 120, e2220771120, https://doi.org/10.1073/pnas.2220771120, 2023.
Laruelle, G. G., Cai, W.-J., Hu, X., Gruber, N., Mackenzie, F. T., and Regnier, P.: Continental shelves as a variable but increasing global sink for atmospheric carbon dioxide, Nat. Commun., 9, 454, https://doi.org/10.1038/s41467-017-02738-z, 2018.
Laws, E. A, Landry, M. R., Barber, R. T., Campbell, L., Dickson, M.-L., and Marra J.: Carbon cycling in primary production bottle incubations: Inferences from grazing experiments and photosynthetic studies using 14C and 18O in the Arabian Sea, Deep-Sea Res. Pt. II, 47, 1339–1352, https://doi.org/10.1016/S0967-0645(99)00146-0, 2000.
Lechtenfeld, O. J., Hertkorn, N., Shen, Y., Witt, M., and Benner, R.: Marine sequestration of carbon in bacterial metabolites, Nat. Commun., 6, 6711, https://doi.org/10.1038/ncomms7711, 2015.
Lee, M.-H., Osburn, C. L., Shin, K.-H., and Hur, J.: New insight into the applicability of spectroscopic indices for dissolved organic matter (DOM) source discrimination in aquatic systems affected by biogeochemical processes, Water Res., 147, 164–176, https://doi.org/10.1016/j.watres.2018.09.048, 2018.
Lehahn, Y., d'Ovidio, F., and Koren, I.: A Satellite-Based Lagrangian View on Phytoplankton Dynamics, Annu. Rev. Mar. Sci., 10, 99–119, https://doi.org/10.1146/annurev-marine-121916-063204, 2018.
Lønborg, C., Álvarez-Salgado, X. A., Davidson, K., Martínez-García, S., and Teira, E.: Assessing the microbial bioavailability and degradation rate constants of dissolved organic matter by fluorescence spectroscopy in the coastal upwelling system of the Ría de Vigo, Mar. Chem., 119, 121–129, https://doi.org/10.1016/j.marchem.2010.02.001, 2010.
Lønborg, C., Yokokawa, T., Herndl, G. J., and Antón Álvarez-Salgado, X.: Production and degradation of fluorescent dissolved organic matter in surface waters of the eastern north Atlantic ocean, Deep-Sea Res. Pt. I, 96, 28–37, https://doi.org/10.1016/j.dsr.2014.11.001, 2015.
López-Abbate, M. C., Garzón-Cardona, J. E., Silva, R. I., Molinero, J. C., Ruiz-Etcheverry, L. A., Martínez, A. M., Gilabert, A. S., and Lara, R. J.: Dilution experiments – H0917, Zenodo [data set], https://doi.org/10.5281/zenodo.11662262, 2024.
Lucas, A. J., Guerrero, R. A., Mianzán, H. W., Acha, E. M., and Lasta, C. A.: Coastal oceanographic regimes of the Northern Argentine Continental Shelf (34–43° S), Estuar. Coast. Shelf Sci., 65, 405–420, https://doi.org/10.1016/j.ecss.2005.06.015, 2005.
Lund Paulsen, M., Müller, O., Larsen, A., Møller, E. F., Middelboe, M., Sejr, M. K., and Stedmon, C.: Biological transformation of Arctic dissolved organic matter in a NE Greenland fjord, Limnol. Oceanogr., 64, 1014–1033, https://doi.org/10.1002/lno.11091, 2019.
Martínez-Pérez, A. M., Nieto-Cid, M., Osterholz, H. Catalá, T.S., Reche, I., Dittmar, T., and Álvarez-Salgado, J. A.: Linking optical and molecular signatures of dissolved organic matter in the Mediterranean Sea, Sci. Rep. 7, 3436, https://doi.org/10.1038/s41598-017-03735-4, 2017.
McCauley, D. J., Gellner, G., Martinez, N. D., Williams, R. J., Sandin, S. A., Micheli, F., Mumby, P. J., and McCann, K. S.: On the prevalence and dynamics of inverted trophic pyramids and otherwise top-heavy communities, Ecol. Lett., 21, 439–454, https://doi.org/10.1111/ele.12900, 2018.
McKnight, D. M., Boyer, E. W., Westerhoff, P. K., Doran, P. T., Kulbe, T., and Andersen, D. T.: Spectrofluorometric characterization of dissolved organic matter for indication of precursor organic material and aromaticity, Limnol. Oceanogr., 46, 38–48, https://doi.org/10.4319/lo.2001.46.1.0038, 2001.
Moran, M. A., Ferrer-González, F. X., Fu, H., Nowinski, B., Olofsson, M., Powers, M. A., Schreier, J. E., Schroer, W. F., Smith, C. B., and Uchimiya, M.: The Ocean's labile DOC supply chain, Limnol. Oceanogr., 67, 1007–1021, https://doi.org/10.1002/lno.12053, 2022.
Nagata, T. and Kirchman, D.: Release of macromolecular organic complexes by heterotrophic marine flagellates, Mar. Ecol. Prog. Ser., 83, 233–240, https://doi.org/10.3354/meps083233, 1992.
Negri, R. M., Silva, R. I., Segura, V., and Cucchi Colleoni, A. D.: Estructura de la comunidad del fitoplancton en el área de El Rincón, Mar Argentino (febrero 2011), Revista Investigación Desarrollo Pesquero, 23, 7–22, 2013.
Negri, R. M., Molinari, G., Carignan, M., Ortega, L., Ruiz, M. G., Cozzolino, E., Cucchi-Colleoni, A. D., Lutz, V. A., Costagliola, M., Garcia, A. B., Izzo, S., Jurquiza, V., Salomone, A., Odizzio, M., La Torre, S., Sanabria, A., Hozbor, M. C., Peressutti, S. R., Méndez, S. M., Silva, R., Martínez, A., Cepeda, G. D., Viñas, M. D., Diaz, M. V., Pajaro, M., Mattera, M. B., Montoya, N. G., Berghoff, C., and Leonarduzzi, E.: Ambiente y plancton en la zona Común de pesca argentino-uruguaya en un escenario de cambio climático (marzo, 2014), Publicaciones de la Comisión Técnica Mixta del Frente Marítimo, 251–316, https://www.ctmfm.org/upload/biblioteca/201708/cg_enlace-16-150211225210.pdf (last access: May 2025), 2016.
Orselli, I. B. M., Kerr, R., Ito, R. G., Tavano, V. M., Mendes, C. R. B., and Garcia, C. A. E.: How fast is the Patagonian shelf-break acidifying?, J. Mar. Syst., 178, 1–14, https://doi.org/10.1016/j.jmarsys.2017.10.007, 2018.
Osburn, C. L., Kinsey, J. D., Bianchi, T. S., and Shields, M. R.: Formation of planktonic chromophoric dissolved organic matter in the ocean, Mar. Chem., 209, 1–13, https://doi.org/10.1016/j.marchem.2018.11.010, 2019.
Paparazzo, F. E., Bianucci, L., Schloss, I. R., Almandoz, G. O., Solís, M., and Esteves, J. L.: Cross-frontal distribution of inorganic nutrients and chlorophyll-a on the Patagonian Continental Shelf of Argentina during summer and fall, Rev. Biol. Mar. Oceanog., 45, 107–119, https://doi.org/10.4067/S0718-19572010000100010, 2010.
Porter, K. G. and Feig, Y. S.: The use of DAPI for identifying and counting aquatic microflora1, Limnol. Oceanogr., 25, 943–948, https://doi.org/10.4319/lo.1980.25.5.0943, 1980.
Redfield, A. C., Ketchum, B. H., and Richards, F. A.: The influence of organisms on the composition of sea-water, in: The sea: ideas and observations on progress in the study of the seas, vol. 2, edited by: Hill, M. N., Wiley Interscience, New York, 26–77, ISBN 9780674017283, 1963.
Romera-Castillo, C., Sarmento, H., Álvarez-Salgado, X. A., Gasol, J. M., and Marraséa, C.: Production of chromophoric dissolved organic matter by marine phytoplankton, Limnol. Oceanogr., 55, 446–454, https://doi.org/10.4319/lo.2010.55.1.0446, 2010.
Romera-Castillo, C., Sarmento, H., Álvarez-Salgado, X. A., Gasol, J. M., and Marrasé, C.: Net production and consumption of fluorescent colored dissolved organic matter by natural bacterial assemblages growing on marine phytoplankton exudates, Appl. Environ. Microbiol., 77, 7490–7498, https://doi.org/10.1128/AEM.00200-11, 2011.
Romero, S. I., Piola, A. R., Charo, M., and Garcia, C. A. E.: Chlorophyll-a variability off Patagonia based on SeaWiFS data, J. Geophys. Res., 111, C05021, https://doi.org/10.1029/2005JC003244, 2006.
Sanders, R., Caron, D., and Berninger U, G.: Relationships between bacteria and heterotrophic nanoplankton in marine and fresh waters: an inter-ecosystem comparison, Mar. Ecol. Prog. Ser., 86, 1–14, https://doi.org/10.3354/meps086001, 1992.
Saraceno, M., Bodnariuk, N., Ruiz-Etcheverry, L. A., Berta, M., Simionato, C. G., Beron-Vera, F. J., and Olascoaga, M. J.: Lagrangian characterization of the southwestern Atlantic from a dense surface drifter deployment, Deep-Sea Res. Pt. I, 208, 104319, https://doi.org/10.1016/j.dsr.2024.104319, 2024.
Sarmento, H., Morana, C., and Gasol, J. M.: Bacterioplankton niche partitioning in the use of phytoplankton-derived dissolved organic carbon: quantity is more important than quality, ISME J., 10, 2582–2592, https://doi.org/10.1038/ismej.2016.66, 2016.
Sherr, E. B. and Sherr, B. F.: Heterotrophic dinoflagellates: a significant component of microzooplankton biomass and major grazers of diatoms in the sea, Mar. Ecol. Prog. Ser., 352, 187–197, https://https://doi.org/10.3354/meps07161, 2007.
Sieburth, J. M., Smetacek, V., and Lenz, J.: Pelagic ecosystem structure: Heterotrophic compartments of the plankton and their relationship to plankton size fractions, Limnol. Oceanogr., 23, 1256–1263, https://doi.org/10.4319/lo.1978.23.6.1256, 1978.
Silva, R., Negri, R., and Lutz, V.: Summer succession of ultraphytoplankton at the EPEA coastal station (Northern Argentina), J. Plankton Res., 31, 447–458, https://doi.org/10.1093/plankt/fbn128, 2009.
Simon, M. and Azam, F.: Protein content and protein synthesis rates of planktonic marine bacteria, Mar. Ecol. Prog. Ser., 51, 201–213, https://doi.org/10.3354/meps051201, 1989.
Skoog, A., Thomas, D., Lara, R., and Richter, K.-U.: Methodological investigations on DOC determinations by the HTCO method, Mar. Chem., 56, 39–44, https://doi.org/10.1016/S0304-4203(96)00084-9, 1997.
Song, H., Marshall, J., Follows, M. J., Dutkiewicz, S., and Forget, G.: Source waters for the highly productive Patagonian shelf in the southwestern Atlantic, J. Mar. Syst., 158, 120–128, https://doi.org/10.1016/j.jmarsys.2016.02.009, 2016.
Spencer, R. G. M., Pellerin, B. A., Bergamaschi, B. A., Downing, B. D., Kraus, T. E. C., Smart, D. R., Dahlgren, R. A., and Hernes, P. J.: Diurnal variability in riverine dissolved organic matter composition determined by in situ optical measurement in the San Joaquin River (California, USA), Hydrol. Process., 21, 3181–3189, https://doi.org/10.1002/hyp.6887, 2007.
Staniewski, M. A. and Short, S. M.: Potential viral stimulation of primary production observed during experimental determinations of phytoplankton mortality, Aquat. Microb. Ecol., 71, 239–256, https://doi.org/10.3354/ame01679, 2014.
Stedmon, C. A. and Bro, R.: Characterizing dissolved organic matter fluorescence with parallel factor analysis: a tutorial, Limnol. Oceanogr.-Meth., 6, 572–579, https://doi.org/10.4319/lom.2008.6.572, 2008.
Stedmon, C. A., Markager, S., and Bro, R.: Tracing dissolved organic matter in aquatic environments using a new approach to fluorescence spectroscopy, Mar. Chem., 82, 239–254, https://doi.org/10.1016/S0304-4203(03)00072-0, 2003.
Suttle, C. A.: Viruses in the sea, Nature, 437, 356–361, https://doi.org/10.1038/nature04160, 2005.
Taylor, A. and Landry, M.: Phytoplankton biomass and size structure across trophic gradients in the southern California Current and adjacent ocean ecosystems, Mar. Ecol. Prog. Ser., 592, 1–17, https://doi.org/10.3354/meps12526, 2018.
Taylor, G. T., Iturriaga, R., and Sullivan, C. W.: Interactions of bactivorous grazers and heterotrophic bacteria with dissolved organic matter, Mar. Ecol. Prog. Ser., 60, 1884–1888, https://doi.org/10.3354/meps023129, 1985.
Tremaine, S. C. and Mills, A. L.: Tests of the critical assumptions of the dilution method for estimating bacterivory by microeucaryotes, Appl. Environ. Microbiol., 53, 2914–2921, https://doi.org/10.1128/aem.53.12.2914-2921.1987, 1987.
Urban-Rich, J., McCarty, J. T., and Shailer, M.: Effects of food concentration and diet on chromophoric dissolved organic matter accumulation and fluorescent composition during grazing experiments with the copepod Calanus finmarchicus, ICES J. Mar. Sci., 61, 542–551, https://doi.org/10.1016/j.icesjms.2004.03.024, 2004.
Wang, Y., Xie, R., Shen, Y., Cai, R., He, C., Chen, Q., Guo, W., Shi, Q., Jiao, N., and Zheng, Q.: Linking microbial population succession and DOM molecular changes in Synechococcus-derived organic matter addition incubation, Microbiol. Spectr., 10, e0230821, https://doi.org/10.1128/spectrum.02308-21, 2022.
Weinbauer, M. G. and Peduzzi, P.: Significance of viruses versus heterotrophic nanoflagellates for controlling bacterial abundance in the northern Adriatic Sea, J. Plankton Res., 17, 1851–1856, https://doi.org/10.1093/plankt/17.9.1851, 1995.