the Creative Commons Attribution 3.0 License.
the Creative Commons Attribution 3.0 License.
Simultaneous shifts in elemental stoichiometry and fatty acids of Emiliania huxleyi in response to environmental changes
Rong Bi
Stefanie M. H. Ismar
Ulrich Sommer
Meixun Zhao
Climate-driven changes in environmental conditions have significant and complex effects on marine ecosystems. Variability in phytoplankton elements and biochemicals can be important for global ocean biogeochemistry and ecological functions, while there is currently limited understanding on how elements and biochemicals respond to the changing environments in key coccolithophore species such as Emiliania huxleyi. We investigated responses of elemental stoichiometry and fatty acids (FAs) in a strain of E. huxleyi under three temperatures (12, 18 and 24 ∘C), three N : P supply ratios (molar ratios 10:1, 24:1 and 63:1) and two pCO2 levels (560 and 2400 µatm). Overall, C : N : P stoichiometry showed the most pronounced response to N : P supply ratios, with high ratios of particulate organic carbon vs. particulate organic nitrogen (POC : PON) and low ratios of PON vs. particulate organic phosphorus (PON : POP) in low-N media, and high POC : POP and PON : POP in low-P media. The ratio of particulate inorganic carbon vs. POC (PIC : POC) and polyunsaturated fatty acid proportions strongly responded to temperature and pCO2, both being lower under high pCO2 and higher with warming. We observed synergistic interactions between warming and nutrient deficiency (and high pCO2) on elemental cellular contents and docosahexaenoic acid (DHA) proportion in most cases, indicating the enhanced effect of warming under nutrient deficiency (and high pCO2). Our results suggest differential sensitivity of elements and FAs to the changes in temperature, nutrient availability and pCO2 in E. huxleyi, which is to some extent unique compared to non-calcifying algal classes. Thus, simultaneous changes of elements and FAs should be considered when predicting future roles of E. huxleyi in the biotic-mediated connection between biogeochemical cycles, ecological functions and climate change.
- Article
(1535 KB) - Full-text XML
-
Supplement
(789 KB) - BibTeX
- EndNote
Climate change and intensive anthropogenic pressures have pronounced and diverse effects on marine ecosystems. Physical and chemical properties in marine ecosystems are changing simultaneously such as the concurrent shifts in temperature, CO2 and oxygen concentrations, and nutrient availability (Boyd et al., 2015). These changes have altered trophic interactions in both bottom-up and top-down directions and thus resulted in changes in community structure of different trophic levels and ecosystem functions (Doney et al., 2012). Phytoplankton are the base of marine food webs and major drivers of ocean biogeochemical cycling, and thus quantifying their responses to changing oceanic conditions is a major challenge in studies of food web structure and ocean biogeochemistry.
Coccolithophores are a key phytoplankton group in the ocean because of their production of calcified scales called coccoliths. They are not only important photosynthetic producers of organic matter (causing a drawdown of CO2 in the surface layer) but also play predominant roles in the production and export of calcium carbonate to deeper layers (causing a net release of CO2 into the atmosphere) (Rost and Riebesell, 2004). Owning to the determination of these two processes on ocean–atmosphere exchange of CO2, coccolithophores exhibit a complex and significant influence on the global carbon cycle (Rost and Riebesell, 2004). Of all coccolithophores, Emiliania huxleyi is the most widely distributed and the most abundant species (Winter et al., 2014), with the capacity to form spatially extensive blooms in mid- to high latitudes (Raitsos et al., 2006; Tyrrell and Merico, 2004). Evidence from in situ and satellite observations indicates that E. huxleyi has been increasingly expanding its range poleward in both hemispheres over the last two decades, and contributing factors to this poleward expansion may differ between regions and hemispheres (Winter et al., 2014). For example, warming and freshening have promoted E. huxleyi blooms in the Bering Sea since the late 1970s (Harada et al., 2012), while temperature and irradiance were best able to explain variability in E. huxleyi-dominated coccolithophore community composition and abundance across the Drake Passage (Southern Ocean) (Charalampopoulou et al., 2016). Hence, empirical data on the responses of E. huxleyi to different environmental drivers would be critical for fully understanding the roles of this prominent coccolithophore species in marine ecosystems.
Extensive experimental studies have shown highly variable responses of E. huxleyi to rising atmospheric CO2 (reviewed by Feng et al., 2017a; Meyer and Riebesell, 2015), while other studies focused on the influence of other environmental factors such as temperature (Rosas-Navarro et al., 2016; Sett et al., 2014; Sorrosa et al., 2005), light intensity (Nanninga and Tyrrell, 1996; Xing et al., 2015) and nutrient availability (Oviedo et al., 2014; Paasche, 1998). Responses of E. huxleyi to the interactions between these different factors have recently received more attention (De Bodt et al., 2010; Feng et al., 2008; Milner et al., 2016; Perrin et al., 2016; Rokitta and Rost, 2012). Many of these studies above focused on the physiological, calcification and photosynthetic responses of E. huxleyi due to its considerable role in the global carbon cycle. However, biogeochemical cycles of the major nutrient elements (nitrogen and phosphorus) and carbon are tightly linked (Hutchins et al., 2009), and thus variability in E. huxleyi C : N : P stoichiometry (cellular quotas and ratios of C, N and P) can also be important in ocean biogeochemistry. Moreover, elemental budgets in organisms are primarily determined by the physiology and biochemistry of biochemicals such as proteins and fatty acids (FAs) (Anderson et al., 2004; Sterner and Elser, 2002). Thus, studying simultaneous changes of elements and biochemicals enables the connection between climate change and ecosystem functions such as elemental cycles. However, shifts in resource nutrient content for consumers are often overlooked in climate change ecology (Rosenblatt and Schmitz, 2016). Recently, Bi et al. (2017) investigated responses of C : N : P stoichiometry and FAs to the interactions of three environmental factors in the diatom Phaeodactylum tricornutum and the cryptophyte Rhodomonas sp., showing dramatic effects of warming and nutrient deficiency, and modest effects of increased pCO2. However, for the key coccolithophore species E. huxleyi much less is known about the simultaneous changes in elemental stoichiometry and biochemicals in response to multiple environmental factor changes.
In the present study, we conducted semi-continuous cultures of E. huxleyi to disentangle potential effects of temperature, N : P supply ratios and pCO2 on E. huxleyi elemental stoichiometry and FAs. The elevated levels of temperature and pCO2 in our study are within the predicted ranges of future ocean scenarios. The interannual average temperature varied between 16 and 22 ∘C at the Azores (http://dive.visitazores.com/en/when-dive), the source region of our E. huxleyi strain, while annual mean sea surface temperature across the North Atlantic (0–60∘ N) is projected to reach 29.8 ∘C in 2100 according to the ocean general circulation model (Lewandowska et al., 2014). Considerable seasonal, depth and regional variations of pCO2 have been observed in the present-day ocean (Joint et al., 2011). In plankton-rich waters, respiration plus atmospheric CO2 enrichment can drive high regional pCO2 at times today, e.g., up to 900 µatm in August, with a minimum value of 192 µatm in April, in the Southern Bight of the North Sea (Schiettecatte et al., 2007). In future oceans, pCO2 is projected to increase with rising atmospheric CO2, with 851–1370 µatm by 2100 and 1371–2900 µatm by 2150 (RCP8.5 scenario of the IPCC report 2014) (IPCC, 2014). We tested the following hypotheses in the present study: (i) elemental stoichiometry and FAs in E. huxleyi show different sensitivity to considerable variations in temperature, N : P supply ratios and pCO2; (ii) the ratios of particulate organic carbon vs. particulate organic nitrogen (POC : PON), POC vs. particulate organic phosphorus (POC : POP), and particulate inorganic carbon vs. POC (PIC : POC) in E. huxleyi will reduce and the proportions of unsaturated fatty acids will increase under projected future ocean scenarios; and (iii) there are synergetic interactions between warming, nutrient deficiency and rising pCO2 on E. huxleyi elemental stoichiometry and FA composition.
2.1 Experimental setup
To address our questions on how multiple environmental drivers influence elemental and FA composition in E. huxleyi, we performed a semi-continuous culture experiment crossing three temperatures (12, 18 and 24 ∘C), three N : P supply ratios (molar ratios 10:1, 24:1 and 63:1) and two pCO2 levels (560 and 2400 µatm). The strain of E. huxleyi (internal culture collection reference code: A8) was isolated from waters off Terceira Island, Azores, North Atlantic (38∘39′22 N, 27∘14′08 W). Semi-continuous cultures, as a practical surrogate for fully continuous culture, have been successfully used to study the responses of phytoplankton stoichiometric and biochemical composition to environmental changes such as nutrient availability (Feng et al., 2017a; Lynn et al., 2000; Terry et al., 1985). Our temperature range setup was based on the study of Lewandowska et al. (2014), who chose a temperature increment of 6 ∘C, according to the ocean general circulation model under the IPCC SRES A1F1 scenario.
All cultures were exposed to a light intensity of 100 µmol photons m−2 s−1 at a 16:8 h light : dark cycle in temperature-controlled rooms. The culture medium was prepared with sterile filtered (0.2 µm pore size, Sartobran® P 300; Sartorius, Göttingen, Germany) North Sea water with a salinity of 37 psu. Macronutrients were added as sodium nitrate (NaNO3) and potassium dihydrogen phosphate (KH2PO4) to achieve three N : P supply ratios, i.e., 35.2 µmol L−1 N and 3.6 µmol L−1 P (10:1 mol mol−1), 88 µmol L−1 N and 3.6 µmol L−1 P (24:1 mol mol−1) and 88 µmol L−1 N and 1.4 µmol L−1 P (63:1 mol mol−1). Vitamins and trace metals were added based on the modified Provasoli culture medium (Ismar et al., 2008; Provasoli, 1963). Initial pCO2 of the culture medium was manipulated by bubbling with air containing the target pCO2. Three replicates were set up for each treatment, resulting in 54 experimental units. Each culture was kept in a sealed cell culture flask with 920 mL culture volume. Culture flasks were carefully rotated twice per day at a set time to minimize sedimentation.
First, batch culture experiments were performed to obtain an estimate of the observed maximal growth rate (μmax, d−1) under three temperatures, three N : P supply ratios and two pCO2 levels. μmax was calculated based on the changes of population cell density within exponential phase (Bi et al., 2012). Once batch cultures reached the early stationary phase, semi-continuous cultures were started with the algae from batch cultures. The gross growth rate (μ (d−1), resulting from the process of reproduction alone due to negligible mortality in cultures lacking predators; Lampert and Sommer, 2007) was applied as 20 % of μmax. Using percentage of μmax guarantees that the strength of nutrient deficiency is equal through all temperature and pCO2 treatments. A fixed value of μ would mean weak deficiency when μmax is low, and strong deficiency when it is high. Based on μ, the equivalent daily renewal rate (D, d−1) can be calculated according to the equation , where t is renewal interval (here t=1 day). The volume of the daily renewal incubation water can be calculated by multiplying D by the total volume of incubation water (920 mL). The incubation water was exchanged with freshly made seawater medium with the target N : P supply ratios, as well as pre-acclimated to the desired pCO2 level. To counterbalance the biological CO2 drawdown, the required amount of CO2-saturated seawater was also added. Renewal of the cultures was carried out at the same hour every day. The steady state in semi-continuous cultures was assessed based on the net growth rate (r (d−1), the difference between the gross growth rate and the loss rate (). When r was zero (at steady state), μ was equivalent to D.
2.2 Sample analysis
Sampling took place at steady state for the following parameters: cell density, dissolved inorganic carbon (DIC), total alkalinity (TA), pH, total particulate carbon (TPC), POC, PON, POP and FAs. Cell density was counted daily in batch and semi-continuous cultures (final cell density at steady state ranging between 1.50 × 105 and 17.8 × 105 cells mL−1, with an average value of 7.95 × 105 cells mL−1). pH measurements were conducted daily in semi-continuous cultures (Fig. S1 in the Supplement), and the electrode was calibrated using standard pH buffers (pH 4 and pH 7; WTW, Weilheim, Germany).
DIC water samples were gently filtered using a single-use syringe filter (0.2 µm, Minisart RC25; Sartorius, Göttingen, Germany) which was connected to the intake tube of a peristaltic pump. Samples were collected into 10 mL glass vials, and all vials were immediately sealed after filling. DIC was analyzed following Hansen et al. (2013) using a gas chromatographic system (8610C; SRI Instruments, California, USA). Samples for TA analysis were filtered through GF/F filters (Whatman GmbH, Dassel, Germany) and analyzed with the Tirino plus 848 (Metrohm, Filderstadt, Germany). The remaining carbonate parameter pCO2 was calculated using CO2SYS (Pierrot et al., 2006) and the constants supplied by Hansson (1973) and Mehrbach et al. (1973) that were refitted by Dickson and Millero (1987) (Table S1 in the Supplement).
TPC, POC, PON and POP samples were filtered onto pre-combusted and pre-washed (5–10 % HCl) GF/F filters (Whatman GmbH, Dassel, Germany). For POC samples, PIC was removed by exposing filters containing TPC to fuming hydrochloric acid for 12 h. Before analysis, filters were dried at 60 ∘C and stored in a desiccator. POC and PON were simultaneously determined by gas chromatography using an organic elemental analyzer (Thermo Flash, 2000; Thermo Fisher Scientific Inc., Schwerte, Germany) after Sharp (1974). POP was analyzed colorimetrically by converting organic phosphorus compounds to orthophosphate (Hansen and Koroleff, 1999). PIC was determined by subtracting POC from TPC. PIC and POC production were estimated by multiplying μ by cellular PIC and POC content, respectively. As the physiological (i.e., cellular) PIC and POC variations cannot directly be upscaled to total population response (Matthiessen et al., 2012), PIC and POC contents in our study were shown both on the cellular (as pg cell−1) and the population (as µg mL−1) levels.
Fatty acid samples were taken on pre-combusted and hydrochloric acid-treated GF/F filters (Whatman GmbH, Dassel, Germany) and stored at −80 ∘C before measurement. FAs were measured as fatty acid methyl esters (FAMEs) using a gas chromatograph (Trace GC-Ultra; Thermo Fisher Scientific Inc., Schwerte, Germany) according to the procedure described in detail in Arndt and Sommer (2014). The FAME 19:0 was added as internal standard and 21:0 as esterification control. The extracted FAs were dissolved with n-hexane to a final volume of 100 µL. Sample aliquots (1 µL) were given into the GC by splitless injection with hydrogen as the carrier gas. Individual FAs were integrated using Chromcard software (Thermo Fisher Scientific Inc., Schwerte, Germany) and identified with reference to the standards Supelco 37 component FAME mixture and Supelco Menhaden fish oil. FA data were expressed as a percentage of total fatty acids (TFAs) (FA proportion, % of TFAs) to better compare our results with those in previous studies. FAs were also quantified on a per unit biomass (µg mg C−1), which is an ideal approach when considering nutritional quality of phytoplankton for herbivores (Piepho et al., 2012).
2.3 Statistical analysis
Generalized linear mixed models (GLMMs) were applied to test the best model explaining the variations in μmax, elemental stoichiometry and FA composition, as this method is more appropriate for non-normal data than classical statistical procedures (Bolker et al., 2009). GLMMs combine the properties of two statistical models (linear mixed models and generalized linear models) (Bolker et al., 2009) and have been widely used in ecology (e.g., Bracewell et al., 2017; Frère et al., 2010; Jamil et al., 2014), in which data sets are often non-normally distributed. In our study, response variables included μmax, elemental stoichiometry (elemental cellular contents (as pg cell−1) and their molar ratios), POC and PIC population yield (as µg mL−1) and production (as pg cell−1 d−1), FA proportion (as % of TFAs) and contents (as µg mg C−1), with temperature, N : P supply ratios and pCO2 as fixed effects. Target distributions were tested and link functions were consequently chosen. The link function is a transformation of the target that allows estimation of the model (https://www.ibm.com/support/knowledgecenter/). For example, identity link function is appropriate with any distribution except for multinomial, while logit can be used only with the binomial or multinomial distribution. For all response variables, we tested models containing first-order effects, and second- and third-order interactions of the three factors. The model that best predicted targets was selected based on the corrected Akaike information criterion (AICc), i.e., a lower AICc value representing a better fit of the model. Changes of 10 units or more in AICc values were considered as a reasonable improvement in the fitting of GLMMs (Bolker et al., 2009). If AICc values were comparable (< 10 units difference), the simpler model was thus chosen, unless there were significant second- or third-order interactions detected. According to differences in AICc values, models containing only first-order effects of the three factors were selected as the best models for most response variables, while those also containing second-order interactions were chosen for cellular POC, PON, POP and PIC contents, and the proportions of saturated fatty acid (SFA) and docosahexaenoic acid (22:6n-3; DHA) (bold letters in Table S2). Models containing third-order interactions were not selected for any response variable.
Nested models were applied to test whether the response pattern to one factor (a nested factor) was significant within another factor if significant second-order interactions were detected in GLMMs. The question a nested model addresses is whether one factor plays a role under one (or several) configuration(s) of another factor, but not under all configurations of that factor equally. Also, the nature (antagonistic, additive, or synergistic) of significant second-order interactions was analyzed according to Christensen et al. (2006). The observed combined effect of two factors was compared with their expected net additive effect (e.g., (factor1 − control) + (factor2 − control)), which was based on the sum of their individual effects. If the observed combined effect exceeded their expected additive effect, the interaction was defined as synergism. In contrast, if the observed combined effect was less than the additive effect, the interaction was defined as antagonism.
All statistical analyses were conducted using SPSS 19.0 (IBM Corporation, New York, USA). Significance level was set to p< 0.05 in all statistical tests.
Table 1Results of the selected GLMMs testing for the effects of temperature, N : P supply ratios and pCO2 on the observed maximal growth rate (μmax), elemental stoichiometry and fatty acid proportions in Emiliania huxleyi. Significant p values are shown in bold; T: temperature; N : P: N : P supply ratios; TFAs: total fatty acids; SFA: saturated fatty acid; MUFA: monounsaturated fatty acid; PUFA: polyunsaturated fatty acid; DHA: docosahexaenoic acid. Results of AICc are shown in Table S2.
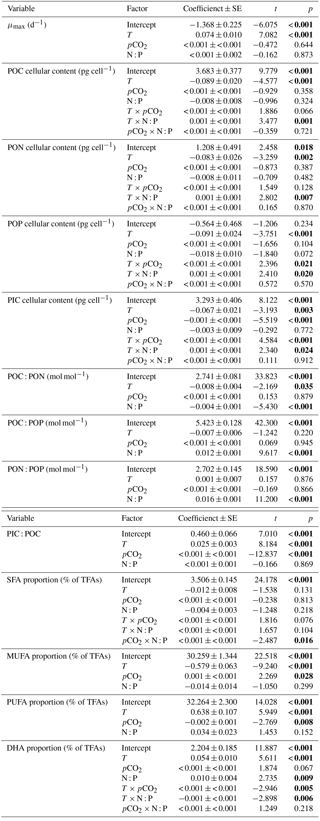
3.1 Maximal growth rate (μmax)
We observed a highly significant effect of temperature (bold letters in Table 1) and non-significant effects of N : P supply ratios and pCO2 on μmax in E. huxleyi. Increasing temperature stimulated μmax, causing μmax to be 2 to 3 times higher at the highest temperature than those at the lowest temperature (Fig. 1).
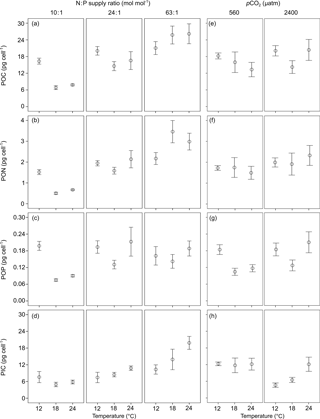
Figure 2Responses of cellular contents of (a, e) particulate organic carbon (POC), (b, f) particulate organic nitrogen (PON), (c, g) particulate organic phosphorus (POP) and (d, h) particulate inorganic carbon (PIC) (mean ± SE) to temperature, N : P supply ratios and pCO2 in Emiliania huxleyi. The selected models contain the first-order effects and second-order interactions of the three environmental factors for the four response variables, with the results of AICc shown in Table S2.
3.2 Elemental stoichiometry
GLMM results showed that cellular contents of POC, PON, POP and PIC responded significantly to temperature and the interactions between temperature and N : P supply ratios (bold letters in Table 1). Moreover, there were significant effects of pCO2 on cellular PIC content, and significant interactions between temperature and pCO2 on cellular POP and PIC contents. For cellular contents of POC, PON and POP, increasing temperature and nutrient deficiency showed synergistic interactions (Table S3), resulting in lower values at higher temperatures under N deficiency (N : P supply ratio = 10:1 mol mol−1) and increasing values with increasing temperature under P deficiency (N : P supply ratio = 63:1 mol mol−1) (Fig. 2a–c; nested model, p < 0.001). Synergistic interactions were also observed between increasing temperature and enhanced pCO2 on cellular POP content (Table S3), showing the lowest value at low pCO2 level and the highest one at enhanced pCO2 in response to increasing temperature (Fig. 2g; nested model, p=0.003). For cellular PIC content, increasing temperature and N deficiency had antagonistic interactions, while increasing temperature and P deficiency showed synergistic interactions (Table S3). As a result, cellular PIC content showed a slight decreasing trend with increasing temperature under N deficiency and an increasing trend under higher N : P supply ratios (Fig. 2d; nested model, p=0.030). Increasing temperature and enhanced pCO2 affected cellular PIC content synergistically (Table S3), with the negative response of cellular PIC content to enhanced pCO2 being significantly weaker as temperature increased (Fig. 2h; nested model, p< 0.001).
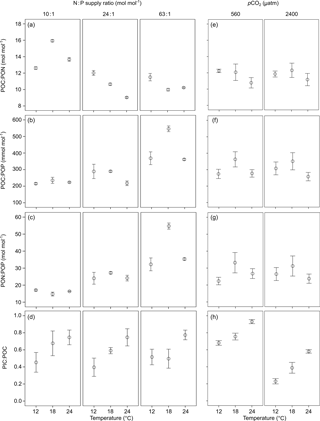
Figure 3The ratios of (a, e) particulate organic carbon vs. particulate organic nitrogen (POC : PON), (b, f) POC vs. particulate organic phosphorus (POC : POP), (c, g) PON vs. POP (PON : POP) and (d, h) particulate inorganic carbon vs. POC (PIC : POC) (mean ± SE) in response to temperature, N : P supply ratios and pCO2 in Emiliania huxleyi. The selected models contain only the first-order effects of the three environmental factors for the four response variables, with the results of AICc shown in Table S2.
POC : PON, POC : POP and PON : POP responded significantly to N : P supply ratios (bold letters in Table 1), while only POC : PON showed significant responses to temperature, with a non-significant effect of pCO2 detected. Increasing N : P supply ratios caused a decreasing trend in POC : PON (Fig. 3a) and an increase in POC : POP (Fig. 3b), resulting in a positive relationship between PON : POP and N : P supply ratios (Fig. 3c). The response of POC : PON to increasing temperature was complex, showing a hump-shaped response under N deficiency and negative responses under higher N : P supply ratios (Fig. 3a). PIC : POC responded significantly to temperature and pCO2, with a non-significant effect of N : P supply ratios detected (Table 1). PIC : POC increased with increasing temperature and decreased with enhanced pCO2 (Fig. 3d and h).
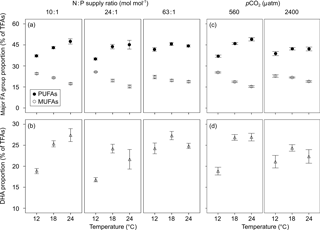
Figure 4Responses of the proportions of (a, c) monounsaturated fatty acids (MUFAs) and polyunsaturated fatty acids (PUFAs), and (b, d) docosahexaenoic acid (DHA) (mean ± SE) to temperature, N : P supply ratios and pCO2 in Emiliania huxleyi. For MUFA and PUFA proportions, the selected models contain only the first-order effects of the three environmental factors, and that for DHA proportion also contains second-order interactions, with the results of AICc shown in Table S2.
3.3 Fatty acids
The most abundant FA group was polyunsaturated fatty acids (PUFAs) (33–54 % of TFAs), followed by SFAs (22–46 %) and monounsaturated fatty acids (MUFAs) (13–27 %), across the entire tested gradients of temperature, N : P supply ratios and pCO2 (Table S4). The high proportion of PUFAs was predominantly caused by high amounts of DHA (12–31 %) and 18:4n-3 (3–13 %), and SFAs were mainly represented by 14:0 (13–23 %) and 16:0 (5–11 %). The major individual MUFA was 18:1n-9 (8–21 %).
GLMM results showed significant effects of temperature and pCO2 on the proportions of both MUFAs and PUFAs, and significant interactions between N : P supply ratios and pCO2 on SFAs (bold letters in Table 1). Increasing temperature caused a decrease in the proportion of MUFAs and an increase in PUFAs (Fig. 4a). In contrast, enhanced pCO2 resulted in an increase in MUFAs and a decrease in PUFAs at higher temperatures (Fig. 4c). Moreover, enhanced pCO2 and N (and P) deficiency affected SFA proportion synergistically (Table S3), with the unimodal response of SFA proportion to increasing N : P supply ratios being more pronounced at the high pCO2 (Fig. S2; nested model, p < 0.001).
The proportion of major individual PUFA (DHA) showed significant responses to temperature and N : P supply ratios, and the interactions between temperature and N : P supply ratios (and pCO2) (bold letters in Table 1). Increasing temperature and N : P supply ratios caused an overall increase in DHA (Fig. 4b). The interactions between increasing temperature and nutrient deficiency (and enhanced pCO2) affected DHA synergistically (Table S3), and the positive effect of temperature became more pronounced at lower N : P supply ratios (nested model, p < 0.001) and at low pCO2 (nested model, p < 0.001) (Fig. 4b and d).
Table 2The changes in cellular elemental contents (as pg cell−1), elemental molar ratios and the proportions of major fatty acid groups and docosahexaenoic acid (DHA) (as % of total fatty acids) in response to warming, N and P deficiency and enhanced pCO2 in Emiliania huxleyi. Here, only significant changes are shown based on GLMM results in Table 1. Red and blue arrows indicate a mean percent increase and decrease in a given response, respectively. T: temperature; N : P : N : P supply ratios.
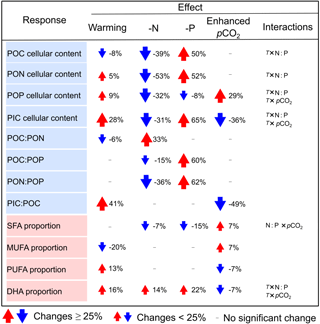
Our study scales the impacts of temperature, N : P supply ratios and pCO2 on elemental stoichiometry and FA composition of the ubiquitously important calcifier E. huxleyi, while accounting for their interactive effects. Overall, C : N : P stoichiometry changed markedly in response to N : P supply ratios, showing a maximum of 62 % changes under P deficiency (Table 2). Both PIC : POC and PUFA proportion increased with warming and decreased under high pCO2, indicating a partial compensation by pCO2 of a predominantly temperature-driven response. The overall response patterns of C : N : P stoichiometry in our study are consistent with those on a global scale (Martiny et al., 2013), and PUFA responses conform with the meta-analysis results on haptophytes (Hixson and Arts, 2016). In line with these studies, we also detected significant interactions between temperature, N : P supply ratios and pCO2 on certain response variables (e.g., cellular elemental contents and DHA proportion) (Table 1), indicating variable response patterns of elemental stoichiometry and FA composition in E. huxleyi under any given constellation of environmental factors. Our results thus underscore the important effects of multiple environmental drivers, demonstrating differential effects of the three environmental factors on elemental stoichiometry and FA composition in E. huxleyi.
4.1 Responses of maximal growth rate
Increasing temperature significantly accelerated μmax of E. huxleyi in our study (Fig. 1; Table 1). This positive correlation between increasing temperature and growth rate is typical for many E. huxleyi strains within the range of temperature 12 to 24 ∘C used in our study (Feng et al., 2008; Rosas-Navarro et al., 2016; Sett et al., 2014; van Bleijswijk et al., 1994). However, the extent to which growth rate of E. huxleyi increases with increasing temperature varies between E. huxleyi strains, which may contribute to specific biogeographic distribution of different strains (Paasche, 2002). For example, growth rate of E. huxleyi from the Gulf of Maine (∼ 42∘ N) was 1.2 times higher at 26 ∘C than that at 16 ∘C, while growth rate of E. huxleyi from the Sargasso Sea (∼ 20–35∘ N) was 1.6 times higher at the higher temperature (Paasche, 2002). In our study, μmax of E. huxleyi (from the Azores, ∼ 38∘ N) was 2 to 3 times higher at the highest temperature than that at the lowest temperature, showing a similar change pattern with that in the E. huxleyi strain from the Sargasso Sea. The results above suggest that the biogeographic origin of an E. huxleyi strain is important for their growth response to temperature.
4.2 Responses of C : N : P stoichiometry
N : P supply ratios showed highly significant effects on C : N : P stoichiometry (up to a 62 % increase in PON : POP under P deficiency) in E. huxleyi in our study, with a weaker effect of warming (a 6 % decrease in POC : PON) and a non-significant effect of pCO2 observed (Tables 1, 2). Similarly, previous lab experiments have also reported that nutrient availability played a more important role than temperature and pCO2 for C : N : P stoichiometry in different strains of E. huxleyi such as those from outer Oslofjord (Skau, 2015) and from the Chatham Rise, east of New Zealand (Feng et al., 2017b). Also, for marine phytoplankton community biomass on a global scale, nitrate concentration as a proxy of nutrient availability explained 36 and 42 % of variation in N : P and C : P, respectively, with the less variation explained by temperature (33 and 38 % of the variation in N : P and C : P, respectively) (Martiny et al., 2013).
N deficiency caused overall high POC : PON and low PON : POP, while P deficiency resulted in high POC : POP and PON : POP in E. huxleyi in this and most previous studies (Langer et al., 2013; Leonardos and Geider, 2005b; Perrin et al., 2016). An important biogeochemical question is the extent to which C : N : P stoichiometry changes in response to N and P deficiency. We found that the high percent change in PON : POP (a 62 % increase) under P deficiency was mainly due to a 60 % increase in POC : POP, associated with the higher percent change in cellular POC content (a 50 % increase) and the lower percent change in cellular POP content (a 8 % decrease) (Table 2). Under N deficiency, the 36 % decrease in PON : POP was driven by a 33 % increase in POC : PON and a 15 % decrease in POC : POP, along with similar percent changes in cellular elemental contents (32 to 53 % decrease). The more variable POC : POP under P deficiency and the less variable POC : PON under N deficiency in our study are consistent with the findings in global suspended particle measurements, which showed the high variability of P : C in response to changes in phosphate and the less variable N : C to changes in nitrate (Galbraith and Martiny, 2015). The consistence of C : N : P stoichiometric responses in our study with those on a global scale may reflect the capacity of E. huxleyi to thrive under a wide range of environmental conditions. This capacity was largely revealed by a pan-genome assessment, which distributed genetic traits variably between strains and showed a suit of core genes for the uptake of inorganic nitrogen and N-rich compounds such as urea (Read et al., 2013). In spite of strain diversity within E. huxleyi, a recent study suggested that the global physiological response of this species to nutrient environments is highly conserved across strains and may underpin its success under a variety of marine environments (Alexander, 2016).
Warming resulted in a significant, but slight decrease in POC : PON (−6 %), associated with a 8 % decrease in cellular POC content and a 5 % increase in cellular PON content, while non-significant responses of POC : POP or PON : POP were observed in E. huxleyi (Table 2). In the literature, variable changes of POC : PON to warming were observed in E. huxleyi, showing positive (Borchard and Engel, 2012), negative (Feng et al., 2008; Matson et al., 2016), and U-shaped responses (Rosas-Navarro et al., 2016). Similar to our study, Borchard and Engel (2012) also found that increasing temperature caused a stronger change in POC : PON than that in POC : POP at higher P conditions in the strain PML B92/11 from Bergen, Norway. The mechanism behind the stronger change in POC : PON compared to POC : POP with warming may be explained by the temperature-dependent physiology hypothesis, which shows that organisms in warmer conditions require fewer P-rich ribosomes, relative to N-rich proteins (Toseland et al., 2013).
The single effects of nutrient availability and temperature described above can be modulated by their interactions. We observed synergistic interactions between warming and nutrient deficiency on cellular contents of POC, PON and POP, and between warming and enhanced pCO2 on cellular POP content (Tables 1, S3). An overall synergistic effect was also observed across 171 studies on the responses of marine and coastal systems to multiple stressors (Crain et al., 2008). Furthermore, although a 29 % change emerged in cellular POP content with rising pCO2, we found a non-significant single effect of pCO2 on E. huxleyi C : N : P stoichiometry. Previous studies showed that rising pCO2 seems to change phytoplankton stoichiometry under specific conditions, e.g. at high light intensity (400 µmol photons m−2 s−1) (Feng et al., 2008) and low nutrient loads (500 µmol photons m−2 s−1 at N : P supply ratio ≤ 15 or N : P supply ratio ≥ 30) (Leonardos and Geider, 2005a). In our study, we used relatively lower light intensity (100 µmol photons m−2 s−1) than that in previous studies, and did not investigate irradiance effects. Additional research is required to assess the effects of other environmental factors such as irradiance and their interactions on C : N : P stoichiometry in our E. huxleyi strain.
Taken together, our results indicate that C : N : P stoichiometry in E. huxleyi largely reflected the changes in N : P supply ratios, across different temperatures and pCO2 levels. However, for two algal species from non-calcifying classes (the diatom P. tricornutum and the cryptophyte Rhodomonas sp.) temperature had the most consistent significant effect on stoichiometric ratios in our previous work (Bi et al., 2017). The results above are consistent with the ranking of environmental control factors in Boyd et al. (2010), which showed that temperature, nitrogen and phosphorus were ranked as important factors for major phytoplankton groups.
4.3 Responses of PIC : POC
Both pCO2 and temperature had highly significant effects on PIC : POC in our study, with enhanced pCO2 and warming resulting in an overall 49 % decrease and a 41 % increase in PIC : POC, respectively, while N : P supply ratios showed no significant effect (Tables 1, 2). This result is in agreement with rankings of the importance of environmental drivers on PIC : POC in a Southern Hemisphere strain of E. huxleyi (isolated from the Chatham Rise), showing the order of pCO2 (negative effect) > temperature (positive effect) and a non-significant effect of nitrate or phosphate (Feng et al., 2017b).
The negative effect of enhanced pCO2 on PIC : POC has been widely observed for different strains of E. huxleyi (Meyer and Riebesell, 2015, and references therein). The negative response of PIC : POC to rising pCO2 in our study was driven by the significant decrease in cellular PIC content (calcification), with cellular POC content (photosynthesis) showing non-significant changes (Tables 1, 2). Previous studies also showed a greater impact of ocean acidification on calcification than on photosynthesis in coccolithophores (De Bodt et al., 2010; Feng et al., 2017a; Meyer and Riebesell, 2015). Feng et al. (2017a) suggested that the decreased calcification in E. huxleyi may be caused by the increased requirement of energy to counteract intracellular acidification. The increased activity of carbonic anhydrase (CA) at low pCO2 may explain the lack of a significant effect of pCO2 on the photosynthetic or growth rate (Feng et al., 2017a), as up-regulation of CA at low DIC was previously observed (Bach et al., 2013).
Warming causes diverse responses of calcification and photosynthesis within E. huxleyi species (Rosas-Navarro et al., 2016, and references therein; present study). Overall, our study showed that the increase in PIC : POC at high temperatures was driven by a markedly increased cellular PIC content (28 %) and a decreased cellular POC content (−8 %) (Tables 1, 2), consistent with the responses of PIC : POC to warming in other E. huxleyi strains such as the strain PML B92/11 (Sett et al., 2014) and the strain CCMP3266 from the Tasman Sea (Matson et al., 2016). The positive response of PIC : POC to increasing temperature may be explained by the allocation of carbon to calcification rather than photosynthesis at high temperatures (Sett et al., 2014).
Significant interactions were observed between temperature and N : P supply ratios, and between temperature and pCO2 on cellular particulate carbon contents in our study (Table 1). For example, the negative relationship between cellular PIC content and enhanced pCO2 became weaker at higher temperatures (Fig. 2h). This result is in agreement with the modulating effect of temperature on the CO2 sensitivity of key metabolic rates in coccolithophores, due to the shift of the optimum CO2 concentration for key metabolic processes towards higher CO2 concentrations from intermediate to high temperatures (Sett et al., 2014). Specifically, the interactions between warming and nutrient deficiency (and high pCO2) synergistically affected both PIC and POC cellular contents in most cases in our study (Table S3), indicating that nutrient deficiency and high pCO2 are likely to enhance the effect of warming on E. huxleyi calcification and photosynthesis efficiency.
In summary, our results showed an overall reduced PIC : POC in E. huxleyi under future ocean scenarios of warming and higher pCO2 (Fig. 3h; Table 2), consistent with the reduced ratio of calcium carbon production to organic carbon during the E. huxleyi bloom in previous mesocosm experiments (Delille et al., 2005; Engel et al., 2005). It is worth noting that cellular PIC and POC contents are a measure for physiological response and cannot be directly used to infer population response, as different responses between cellular and population yields of PIC (and POC) (as µg mL−1) to environmental changes were evident in previous work (Matthiessen et al., 2012) and the present study (Tables S5, S6; Figs. S3, S4). Thus, scaling our results up to coccolithophores carbon export should consider these uncertainties.
4.4 Responses of fatty acids
Our study provides one of the first experimental demonstrations of the relative importance of temperature, N : P supply ratios and pCO2 on E. huxleyi FA composition. Both temperature and pCO2 had significant effects on the proportions of MUFAs and PUFAs, with warming causing larger changes in MUFAs and PUFAs than rising pCO2, while significant effects of N : P supply ratios were only observed for DHA proportion (Tables 1, 2).
Increasing temperature caused a 20 % decline in MUFA proportion and a 13 % increase in PUFA proportion in our study (Table 2). This result is consistent with the negative response of MUFA proportion and positive response of PUFA proportion to warming in other haptophytes based on a meta-analysis on 137 FA profiles (Hixson and Arts, 2016), showing an opposite response to general patterns of phytoplankton FAs to warming. Although warming is expected to have a negative effect on the degree of fatty acid unsaturation to maintain cell membrane structural functions (Fuschino et al., 2011; Guschina and Harwood, 2006; Sinensky, 1974), variable FA responses to warming were widely observed in different phytoplankton groups (Bi et al., 2017; Renaud et al., 2002; Thompson et al., 1992). Contradictory findings were even reported in meta-analyses on large FA profiles such as the absence (Galloway and Winder, 2015) or presence (Hixson and Arts, 2016) of the negative correlation between temperature and the proportion of long-chain EFAs in freshwater and marine phytoplankton. While the underling mechanisms of variable FA responses are still unclear, it is known that both phylogeny and environmental conditions determine phytoplankton FA composition (Bi et al., 2014; Dalsgaard et al., 2003; Galloway and Winder, 2015). In our study, we found significant interactions between temperature and pCO2 (and N : P supply ratios) on the individual FA component DHA, showing that pCO2 and nutrient availability may alter the effect of warming on E. huxleyi FA composition.
Enhanced pCO2 led to an overall 7 % increase in MUFAs and a 7 % decrease in PUFAs (Table 2), consistent with FA response patterns in the E. huxleyi strain PML B92/11 (Riebesell et al., 2000) and the strain AC472 from western New Zealand, South Pacific (Fiorini et al., 2010). Also in a natural plankton community (Raunefjord, southern Norway), PUFA proportion was reduced at high pCO2 level in the nano-size fraction, suggesting a reduced Haptophyta (dominated by E. huxleyi) biomass and a negative effect of high pCO2 on PUFA proportion (Bermúdez et al., 2016). To date, several mechanisms have been suggested to explain the reduced PUFAs at high pCO2 in green algae (Pronina et al., 1998; Sato et al., 2003; Thompson, 1996), with much less work conducted in other phytoplankton groups. One possible mechanism was demonstrated in a study on Chlamydomonas reinhardtii, showing that the repression of the CO2-concentrating mechanisms (CCMs) was associated with reduced FA desaturation at high CO2 concentration (Pronina et al., 1998). Our observed decrease in the proportion and content of PUFAs at higher pCO2 (Table S6) fits well with the mechanism proposed by Pronina et al. (1998), which may be attributed to the repression of CCMs at high pCO2 in E. huxleyi.
N and P deficiency caused no significant changes in the proportions of MUFAs and PUFAs, while a 14 to 22 % increase in DHA proportion was observed (Table 2). While nutrients often play a major role on phytoplankton lipid composition (Fields et al., 2014; Hu et al., 2008), the less pronounced effects of nutrient deficiency in our study indicate a unique lipid biosynthesis in E. huxleyi. Indeed, Van Mooy et al. (2009) suggested that E. huxleyi used non-phosphorus betaine lipids as substitutes for phospholipids in response to P scarcity. Genes are also present in the core genome of E. huxleyi for the synthesis of betaine lipids and unusual lipids used as nutritional/feedstock supplements (Read et al., 2013). Therefore, the lack of significant nutrient effects on most FA groups in E. huxleyi in our study may be caused by the functioning of certain lipid substitutions under nutrient deficiency.
In summary, our study showed stronger effects of pCO2 and temperature, and a weaker effect of N : P supply ratios on the proportions of unsaturated FAs in E. huxleyi. It should be noted that using different units to quantify FA composition may cause contradictory results, e.g., an increase in PUFA proportion (% of TFAs) but non-significant changes in PUFA contents per biomass (µg mg C−1) with increasing temperature in our study (Tables S5, S6). Moreover, PUFA contents per biomass in two species of non-calcifying classes (P. tricornutum and Rhodomonas sp.) showed a different response pattern from that observed in E. huxleyi in our study, i.e., a significant negative effect of enhanced pCO2 on PUFA contents in E. huxleyi (Table S6) but a non-significant effect of pCO2 on PUFA contents in P. tricornutum and Rhodomonas sp. (Bi et al., 2017). This different response between phytoplankton groups is in agreement with findings in mesocosm studies (Bermúdez et al., 2016; Leu et al., 2013), suggesting that changes in taxonomic composition can cause different relationships between PUFAs and pCO2 in natural phytoplankton community.
4.5 Implications for marine biogeochemistry and ecology
We observed an overall increase in POC : PON (with warming and N deficiency) and POC : POP (with N and P deficiency) in E. huxleyi, while enhanced pCO2 showed no significant effect (Table 2). This result indicates that nitrogen and phosphorus requirements in E. huxleyi are likely to reduce under projected future changes in temperature and nutrient deficiency, and will respond less strongly to changes in pCO2 than to temperature or nutrient regime shifts. Likewise, Hutchins et al. (2009) suggested negligible or minor effects of projected future changes in pCO2 on most phytoplankton phosphorus requirements. Moreover, the overall low PIC : POC under future ocean scenarios (warming and enhanced pCO2) indicates that carbon production by the strain E. huxleyi in our study acts as a carbon sink. This argument is consistent with the findings of the decreased calcification with increasing pCO2 in most coccolithophores (Beaufort et al., 2011; Hutchins and Fu, 2017), which may reduce vertical exported fluxes of sinking calcium carbonate and minimize calcification as a carbon source term, ultimately downsizing the ocean's biological carbon cycle (Hutchins and Fu, 2017).
Besides the overall increase in POC : PON and POC : POP, we found an overall increase in the proportions of PUFAs (with warming and enhanced pCO2) and DHA (with warming, N and P deficiency and enhanced pCO2) in E. huxleyi (Table 2), but a decrease in PUFA and DHA contents per biomass with enhanced pCO2 (Table S6). The relationship between changes in stoichiometry and FA composition in phytoplankton varies in a complex way with environmental conditions and algal taxonomy (Bi et al., 2014; Pedro Cañavate et al., 2017; Sterner and Schulz, 1998). For example, the correlation between PON : POC and PUFA contents per biomass was negative in Rhodomonas sp. and positive in P. tricornutum under N deficiency (Bi et al., 2014). Our findings thus indicate that elemental composition responses may be coupled with responses in essential FA composition in the strain of E. huxleyi studied under certain configurations of environmental drivers. Such a linkage between stoichiometric and FA composition is important in studies of food web dynamics, as the C : N and C : P stoichiometry and PUFAs both have been used as indicators of nutritional quality of phytoplankton, with high POC : PON (and POC : POP) and low contents in certain PUFAs often constraining zooplankton production by reducing trophic carbon transfer from phytoplankton to zooplankton (Hessen, 2008; Jónasdóttir et al., 2009; Müller-Navarra et al., 2000; Malzahn et al., 2016). In addition, other factors such as the cell size of phytoplankton and nutritional requirements of consumers can also influence trophic transfer efficiency (Anderson and Pond, 2000; Sommer et al., 2016). Nevertheless, studies on plant–herbivore interactions reported that changes in elemental and biochemical composition in phytoplankton can translate to higher trophic levels (Kamya et al., 2017; Malzahn et al., 2010; Rossoll et al., 2012) and refer to direct effects of environmental changes on low-trophic-level consumers, which can be modified by indirect bottom-up driven impacts through the primary producers (Garzke et al., 2016, 2017).
Our study shows that N : P supply ratios had the strongest effect on C : N : P stoichiometry, while temperature and pCO2 played more influential roles in PIC : POC and PUFA proportions in E. huxleyi. The specific response patterns of elemental ratios and FAs have important implications for understanding biogeochemical and ecological functioning of E. huxleyi. The observations presented here suggest differential responses of elements and FAs to rising temperature, nutrient deficiency and enhanced pCO2 in E. huxleyi, being to some extent unique compared to algal species from non-calcifying classes. Thus, the role of multiple environmental drivers under the biodiversity context should be considered to truly estimate the future functioning of phytoplankton in changing marine environments.
Data sets are available upon request by contacting Meixun Zhao (maxzhao@ouc.edu.cn, maxzhao04@yahoo.com).
The supplement related to this article is available online at: https://doi.org/10.5194/bg-15-1029-2018-supplement.
RB, SI, US and MZ designed the experiments and RB carried them out. RB prepared the manuscript with contributions from all co-authors.
The authors declare that they have no conflict of interest.
The authors thank Thomas Hansen, Cordula Meyer, Bente Gardeler and Petra Schulz for technical assistance. Birte Matthiessen,
Renate Ebbinhaus and Lena Eggers are acknowledged for providing the E. huxleyi strain.
The authors thank Dorthe Ozod-Seradj, Carolin Paul, Si Li, Xupeng Chi and Yong Zhang
for their assistance during the experiments, and Philipp Neitzschel,
Kastriot Qelaj and Jens Wernhöner for helping with DIC analysis. Jessica Garzke is acknowledged for her comments on the calculation of interaction
magnitude. Mandy Velthuis and one anonymous reviewer are gratefully acknowledged for helpful
comments. This study was funded by the National Natural Science Foundation
of China (grant no. 41630966; no. 41506086; no. 41521064), the Scientific
Research Foundation for the Returned Overseas Chinese Scholars, State
Education Ministry (grant no. [2015]1098), the “111” Project (B13030) and
GEOMAR Helmholtz-Zentrum für Ozeanforschung Kiel. This is MCTL
contribution 139.
Edited by: Wajih Naqvi
Reviewed by: Mandy Velthuis and one anonymous referee
Alexander, H.: Defining the ecological and physiological traits of phytplankton across marine ecosystems, Ph.D. thesis, Woods Hole Oceanographic Institution, Woods Hole, USA, 179 pp., 2016.
Anderson, T. R. and Pond, D. W.: Stoichiometric theory extended to micronutrients: Comparison of the roles of essential fatty acids, carbon, and nitrogen in the nutrition of marine copepods, Limnol. Oceanogr., 45, 1162–1167, https://doi.org/10.4319/lo.2000.45.5.1162, 2000.
Anderson, T. R., Boersma, M., and Raubenheimer, D.: Stoichiometry: linking elements to biochemicals, Ecology, 85, 1193–1202, https://doi.org/10.1890/02-0252, 2004.
Arndt, C. and Sommer, U.: Effect of algal species and concentration on development and fatty acid composition of two harpacticoid copepods, Tisbe sp. and Tachidius discipes, and a discussion about their suitability for marine fish larvae, Aquacult. Nutr., 20, 44–59, https://doi.org/10.1111/anu.12051, 2014.
Bach, L. T., Mackinder, L. C. M., Schulz, K. G., Wheeler, G., Schroeder, D. C., Brownlee, C., and Riebesell, U.: Dissecting the impact of CO2 and pH on the mechanisms of photosynthesis and calcification in the coccolithophore Emiliania huxleyi, New Phytol., 199, 121–134, https://doi.org/10.1111/nph.12225, 2013.
Beaufort, L., Probert, I., de Garidel-Thoron, T., Bendif, E. M., Ruiz-Pino, D., Metzl, N., Goyet, C., Buchet, N., Coupel, P., Grelaud, M., Rost, B., Rickaby, R. E. M., and de Vargas, C.: Sensitivity of coccolithophores to carbonate chemistry and ocean acidification, Nature, 476, 80–83, https://doi.org/10.1038/nature10295, 2011.
Bermúdez, J. R., Riebesell, U., Larsen, A., and Winder, M.: Ocean acidification reduces transfer of essential biomolecules in a natural plankton community, Sci. Rep.-UK, 6, 27749, https://doi.org/10.1038/srep27749, 2016.
Bi, R., Arndt, C., and Sommer, U.: Stoichiometric responses of phytoplankton species to the interactive effect of nutrient supply ratios and growth rates, J. Phycol., 48, 539–549, https://doi.org/10.1111/j.1529-8817.2012.01163.x, 2012.
Bi, R., Arndt, C., and Sommer, U.: Linking elements to biochemicals: effects of nutrient supply ratios and growth rates on fatty acid composition of phytoplankton species, J. Phycol., 50, 117–130, https://doi.org/10.1111/jpy.12140, 2014.
Bi, R., Ismar, S. M. H., Sommer, U., and Zhao, M.: Environmental dependence of the correlations between stoichiometric and fatty acid-based indicators of phytoplankton food quality, Limnol. Oceanogr., 62, 334–347, https://doi.org/10.1002/lno.10429, 2017.
Bolker, B. M., Brooks, M. E., Clark, C. J., Geange, S. W., Poulsen, J. R., Stevens, M. H. H., and White, J.-S. S.: Generalized linear mixed models: a practical guide for ecology and evolution, Trends Ecol. Evol., 24, 127–135, https://doi.org/10.1016/j.tree.2008.10.008, 2009.
Borchard, C. and Engel, A.: Organic matter exudation by Emiliania huxleyi under simulated future ocean conditions, Biogeosciences, 9, 3405–3423, https://doi.org/10.5194/bg-9-3405-2012, 2012.
Boyd, P. W., Strzepek, R., Fu, F., and Hutchins, D. A.: Environmental control of open-ocean phytoplankton groups: Now and in the future, Limnol. Oceanogr., 55, 1353–1376, https://doi.org/10.4319/lo.2010.55.3.1353, 2010.
Boyd, P. W., Lennartz, S. T., Glover, D. M., and Doney, S. C.: Biological ramifications of climate-change-mediated oceanic multi-stressors, Nature Climate Change, 5, 71–79, https://doi.org/10.1038/nclimate2441, 2015.
Bracewell, S. A., Johnston, E. L., and Clark, G. F.: Latitudinal variation in the competition-colonisation trade-off reveals rate-mediated mechanisms of coexistence, Ecol. Lett., 20, 947–957, https://doi.org/10.1111/ele.12791, 2017.
Charalampopoulou, A., Poulton, A. J., Bakker, D. C. E., Lucas, M. I., Stinchcombe, M. C., and Tyrrell, T.: Environmental drivers of coccolithophore abundance and calcification across Drake Passage (Southern Ocean), Biogeosciences, 13, 5717–5735, https://doi.org/10.5194/bg-13-5917-2016, 2016.
Christensen, M. R., Graham, M. D., Vinebrooke, R. D., Findlay, D. L., Paterson, M. J., and Turner, M. A.: Multiple anthropogenic stressors cause ecological surprises in boreal lakes, Glob. Change Biol., 12, 2316–2322, https://doi.org/10.1111/j.1365-2486.2006.01257.x, 2006.
Crain, C. M., Kroeker, K., and Halpern, B. S.: Interactive and cumulative effects of multiple human stressors in marine systems, Ecol. Lett., 11, 1304–1315, https://doi.org/10.1111/j.1461-0248.2008.01253.x, 2008.
Dalsgaard, J., St. John, M., Kattner, G., Müller-Navarra, D., and Hagen, W.: Fatty acid trophic markers in the pelagic marine environment, Adv. Mar. Biol., 46, 225–340, https://doi.org/10.1016/S0065-2881(03)46005-7, 2003.
De Bodt, C., Van Oostende, N., Harlay, J., Sabbe, K., and Chou, L.: Individual and interacting effects of pCO2 and temperature on Emiliania huxleyi calcification: study of the calcite production, the coccolith morphology and the coccosphere size, Biogeosciences, 7, 1401–1412, https://doi.org/10.5194/bg-7-1401-2010, 2010.
Delille, B., Harlay, J., Zondervan, I., Jacquet, S., Chou, L., Wollast, R., Bellerby, R. G. J., Frankignoulle, M., Borges, A. V., Riebesell, U., and Gattuso, J. P.: Response of primary production and calcification to changes of pCO2 during experimental blooms of the coccolithophorid Emiliania huxleyi, Global Biogeochem. Cy., 19, GB2023, https://doi.org/10.1029/2004gb002318, 2005.
Dickson, A. and Millero, F.: A comparison of the equilibrium constants for the dissociations of carbonic acid in seawater media, Deep-Sea Res., 34, 1733–1741, https://doi.org/10.1016/0198-0149(87)90021-5, 1987.
Doney, S. C., Ruckelshaus, M., Duffy, J. E., Barry, J. P., Chan, F., English, C. A., Galindo, H. M., Grebmeier, J. M., Hollowed, A. B., Knowlton, N., Polovina, J., Rabalais, N. N., Sydeman, W. J., and Talley, L. D.: Climate change impacts on marine ecosystems, Annu. Rev. Mar. Sci., 4, 11–37, https://doi.org/10.1146/annurev-marine-041911-111611, 2012.
Engel, A., Zondervan, I., Aerts, K., Beaufort, L., Benthien, A., Chou, L., Delille, B., Gattuso, J. P., Harlay, J., Heemann, C., Hoffmann, L., Jacquet, S., Nejstgaard, J., Pizay, M. D., Rochelle-Newall, E., Schneider, U., Terbrueggen, A., and Riebesell, U.: Testing the direct effect of CO2 concentration on a bloom of the coccolithophorid Emiliania huxleyi in mesocosm experiments, Limnol. Oceanogr., 50, 493–507, https://doi.org/10.4319/lo.2005.50.2.0493, 2005.
Feng, Y., Warner, M. E., Zhang, Y., Sun, J., Fu, F.-X., Rose, J. M., and Hutchins, D. A.: Interactive effects of increased pCO2, temperature and irradiance on the marine coccolithophore Emiliania huxleyi (Prymnesiophyceae), Eur. J. Phycol., 43, 87–98, https://doi.org/10.1080/09670260701664674, 2008.
Feng, Y., Roleda, M. Y., Armstrong, E., Boyd, P. W., and Hurd, C. L.: Environmental controls on the growth, photosynthetic and calcification rates of a Southern Hemisphere strain of the coccolithophore Emiliania huxleyi, Limnol. Oceanogr., 62, 519–540, https://doi.org/10.1002/lno.10442, 2017a.
Feng, Y., Roleda, M. Y., Armstrong, E., Law, C. S., Boyd, P. W., and Hurd, C. L.: Environmental controls on the elemental composition of a Southern Hemisphere strain of the coccolithophore Emiliania huxleyi, Biogeosciences Discuss., https://doi.org/10.5194/bg-2017-332, in review, 2017b.
Fields, M. W., Hise, A., Lohman, E. J., Bell, T., Gardner, R. D., Corredor, L., Moll, K., Peyton, B. M., Characklis, G. W., and Gerlach, R.: Sources and resources: importance of nutrients, resource allocation, and ecology in microalgal cultivation for lipid accumulation, Appl. Microbiol. Biot., 98, 4805–4816, https://doi.org/10.1007/s00253-014-5694-7, 2014.
Fiorini, S., Gattuso, J.-P., van Rijswijk, P., and Middelburg, J.: Coccolithophores lipid and carbon isotope composition and their variability related to changes in seawater carbonate chemistry, J. Exp. Mar. Biol. Ecol., 394, 74–85, https://doi.org/10.1016/j.jembe.2010.07.020, 2010.
Frère, C. H., Kruetzen, M., Mann, J., Connor, R. C., Bejder, L., and Sherwin, W. B.: Social and genetic interactions drive fitness variation in a free-living dolphin population, P. Natl. Acad. Sci. USA, 107, 19949–19954, https://doi.org/10.1073/pnas.1007997107, 2010.
Fuschino, J. R., Guschina, I. A., Dobson, G., Yan, N. D., Harwood, J. L., and Arts, M. T.: Rising water temperatures alter lipid dynamics and reduce N-3 essential fatty acid concentrations in Scenedesmus obliquus (Chlorophyta), J. Phycol., 47, 763–774, https://doi.org/10.1111/j.1529-8817.2011.01024.x, 2011.
Galbraith, E. D. and Martiny, A. C.: A simple nutrient-dependence mechanism for predicting the stoichiometry of marine ecosystems, P. Natl. Acad. Sci. USA, 112, 8199–8204, https://doi.org/10.1073/pnas.1423917112, 2015.
Galloway, A. W. E. and Winder, M.: Partitioning the relative importance of phylogeny and environmental conditions on phytoplankton fatty acids, Plos One, 10, e0130053, https://doi.org/10.1371/journal.pone.0130053, 2015.
Garzke, J., Hansen, T., Ismar, S. M. H., and Sommer, U.: Combined effects of ocean warming and acidification on copepod abundance, body size and fatty acid content, Plos One, 11, e0155952, https://doi.org/10.1371/journal.pone.0155952, 2016.
Garzke, J., Sommer, U., and Ismar, S. M. H.: Is the chemical composition of biomass the agent by which ocean acidification influences on zooplankton ecology?, Aquat. Sci., 79, 733–748, https://doi.org/10.1007/s00027-017-0532-5, 2017.
Guschina, I. A. and Harwood, J. L.: Mechanisms of temperature adaptation in poikilotherms, Febs Lett., 580, 5477–5483, https://doi.org/10.1016/j.febslet.2006.06.066, 2006.
Hansen, H. P. and Koroleff, F.: Determination of nutrients, in: Methods of Seawater Analysis, edited by: Grasshoff, K., Kremling, K., and Ehrhardt, M., WILEY-VCH, Weinheim, Germany, 159–228, 1999.
Hansen, T., Gardeler, B., and Matthiessen, B.: Technical Note: Precise quantitative measurements of total dissolved inorganic carbon from small amounts of seawater using a gas chromatographic system, Biogeosciences, 10, 6601–6608, https://doi.org/10.5194/bg-10-6601-2013, 2013.
Hansson, I.: A new set of acidity constants for carbonic acid and boric acid in seawater, Deep-Sea Res., 20, 661–678, https://doi.org/10.1016/0011-7471(73)90100-9, 1973.
Harada, N., Sato, M., Oguri, K., Hagino, K., Okazaki, Y., Katsuki, K., Tsuji, Y., Shin, K.-H., Tadai, O., Saitoh, S.-I., Narita, H., Konno, S., Jordan, R. W., Shiraiwa, Y., and Grebmeier, J.: Enhancement of coccolithophorid blooms in the Bering Sea by recent environmental changes, Global Biogeochem. Cy., 26, GB2036, https://doi.org/10.1029/2011gb004177, 2012.
Hessen, D. O.: Efficiency, energy and stoichiometry in pelagic food webs; reciprocal roles of food quality and food quantity, Freshwater Rev., 1, 43–57, https://doi.org/10.1608/frj-1.1.3, 2008.
Hixson, S. M. and Arts, M. T.: Climate warming is predicted to reduce omega-3, long-chain, polyunsaturated fatty acid production in phytoplankton, Glob. Change Biol., 22, 2744–2755, https://doi.org/10.1111/gcb.13295, 2016.
Hu, Q., Sommerfeld, M., Jarvis, E., Ghirardi, M., Posewitz, M., Seibert, M., and Darzins, A.: Microalgal triacylglycerols as feedstocks for biofuel production: perspectives and advances, Plant J., 54, 621–639, https://doi.org/10.1111/j.1365-313X.2008.03492.x, 2008.
Hutchins, D. A. and Fu, F.: Microorganisms and ocean global change, Nat. Microbiol., 2, 17058, https://doi.org/10.1038/nmicrobiol.2017.58, 2017.
Hutchins, D. A., Mulholland, M. R., and Fu, F.: Nutrient cycles and marine microbes in a CO2-enriched ocean, Oceanography, 22, 128–145, https://doi.org/10.5670/oceanog.2009.103, 2009.
IPCC: Climate change 2014: Synthesis report. Contribution of working groups I, II and III to the fifth assessment report of the intergovernmental panel on climate change, Geneva, Switzerland, 151 pp., 2014.
Ismar, S. M. H., Hansen, T., and Sommer, U.: Effect of food concentration and type of diet on Acartia survival and naupliar development, Mar. Biol., 154, 335–343, https://doi.org/10.1007/s00227-008-0928-9, 2008.
Jónasdóttir, S. H., Visser, A. W., and Jespersen, C.: Assessing the role of food quality in the production and hatching of Temora longicornis eggs, Mar. Ecol.-Prog. Ser., 382, 139–150, https://doi.org/10.3354/meps07985, 2009.
Jamil, T., Kruk, C., and ter Braak, C. J. F.: A unimodal species response model relating traits to environment with application to phytoplankton communities, Plos One, 9, e97583, https://doi.org/10.1371/journal.pone.0097583, 2014.
Joint, I., Doney, S. C., and Karl, D. M.: Will ocean acidification affect marine microbes?, ISME J., 5, 1–7, https://doi.org/10.1038/ismej.2010.79, 2011.
Kamya, P. Z., Byrne, M., Mos, B., Hall, L., and Dworjanyn, S. A.: Indirect effects of ocean acidification drive feeding and growth of juvenile crown-of-thorns starfish, Acanthaster planci, P. Roy. Soc. B-Biol. Sci., 284, 20170778, https://doi.org/10.1098/rspb.2017.0778, 2017.
Lampert, W. and Sommer, U.: Limnoecology: The ecology of lakes and streams, 2nd Edn., Oxford University Press, Oxford, UK, 2007.
Langer, G., Oetjen, K., and Brenneis, T.: Coccolithophores do not increase particulate carbon production under nutrient limitation: A case study using Emiliania huxleyi (PML B92/11), J. Exp. Mar. Biol. Ecol., 443, 155–161, https://doi.org/10.1016/j.jembe.2013.02.040, 2013.
Leonardos, N. and Geider, R. J.: Elemental and biochemical composition of Rhinomonas reticulata (Cryptophyta) in relation to light and nitrate-to-phosphate supply ratios, J. Phycol., 41, 567–576, https://doi.org/10.1111/j.1529-8817.2005.00082.x, 2005a.
Leonardos, N. and Geider, R. J.: Elevated atmospheric carbon dioxide increases organic carbon fixation by Emiliania huxleyi (Haptophyta), under nutrient-limited high-light conditions, J. Phycol., 41, 1196–1203, https://doi.org/10.1111/j.1529-8817.2005.00152.x, 2005b.
Leu, E., Daase, M., Schulz, K. G., Stuhr, A., and Riebesell, U.: Effect of ocean acidification on the fatty acid composition of a natural plankton community, Biogeosciences, 10, 1143–1153, https://doi.org/10.5194/bg-10-1143-2013, 2013.
Lewandowska, A. M., Boyce, D. G., Hofmann, M., Matthiessen, B., Sommer, U., and Worm, B.: Effects of sea surface warming on marine plankton, Ecol. Lett., 17, 614–623, https://doi.org/10.1111/ele.12265, 2014.
Lynn, S. G., Kilham, S. S., Kreeger, D. A., and Interlandi, S. J.: Effect of nutrient availability on the biochemical and elemental stoichiometry in the freshwater diatom Stephanodiscus minutulus (Bacillariophyceae), J. Phycol., 36, 510–522, https://doi.org/10.1046/j.1529-8817.2000.98251.x, 2000.
Malzahn, A. M., Hantzsche, F., Schoo, K. L., Boersma, M., and Aberle, N.: Differential effects of nutrient-limited primary production on primary, secondary or tertiary consumers, Oecologia, 162, 35–48, https://doi.org/10.1007/s00442-009-1458-y, 2010.
Malzahn, A. M., Doerfler, D., and Boersma, M.: Junk food gets healthier when it's warm, Limnol. Oceanogr., 61, 1677–1685, https://doi.org/10.1002/lno.10330, 2016.
Martiny, A. C., Pham, C. T. A., Primeau, F. W., Vrugt, J. A., Moore, J. K., Levin, S. A., and Lomas, M. W.: Strong latitudinal patterns in the elemental ratios of marine plankton and organic matter, Nat. Geosci., 6, 279–283, https://doi.org/10.1038/ngeo1757, 2013.
Matson, P. G., Ladd, T. M., Halewood, E. R., Sangodkar, R. P., Chmelka, B. F., and Iglesias-Rodriguez, D.: Intraspecific differences in biogeochemical responses to thermal change in the coccolithophore Emiliania huxleyi, Plos One, 11, e0162313, https://doi.org/10.1371/journal.pone.0162313, 2016.
Matthiessen, B., Eggers, S. L., and Krug, S. A.: High nitrate to phosphorus regime attenuates negative effects of rising pCO2 on total population carbon accumulation, Biogeosciences, 9, 1195–1203, https://doi.org/10.5194/bg-9-1195-2012, 2012.
Mehrbach, C., Culberson, C., Hawley, J., and Pytkowicz, R.: Measurement of the apparent dissociation constants of carbonic acid in seawater at atmospheric pressure, Limnol. Oceanogr, 18, 897–907, https://doi.org/10.4319/lo.1973.18.6.0897, 1973.
Meyer, J. and Riebesell, U.: Reviews and Syntheses: Responses of coccolithophores to ocean acidification: a meta-analysis, Biogeosciences, 12, 1671–1682, https://doi.org/10.5194/bg-12-1671-2015, 2015.
Milner, S., Langer, G., Grelaud, M., and Ziveri, P.: Ocean warming modulates the effects of acidification on Emiliania huxleyi calcification and sinking, Limnol. Oceanogr., 61, 1322–1336, https://doi.org/10.1002/lno.10292, 2016.
Müller-Navarra, D. C., Brett, M. T., Liston, A. M., and Goldman, C. R.: A highly unsaturated fatty acid predicts carbon transfer between primary producers and consumers, Nature, 403, 74–77, https://doi.org/10.1038/47469, 2000.
Nanninga, H. J. and Tyrrell, T.: Importance of light for the formation of algal blooms by Emiliania huxleyi, Mar. Ecol.-Prog. Ser., 136, 195–203, https://doi.org/10.3354/meps136195, 1996.
Oviedo, A. M., Langer, G., and Ziveri, P.: Effect of phosphorus limitation on coccolith morphology and element ratios in Mediterranean strains of the coccolithophore Emiliania huxleyi, J. Exp. Mar. Biol. Ecol., 459, 105–113, https://doi.org/10.1016/j.jembe.2014.04.021, 2014.
Paasche, E.: Roles of nitrogen and phosphorus in coccolith formation in Emiliania huxleyi (Prymnesiophyceae), Eur. J. Phycol., 33, 33–42, https://doi.org/10.1017/s0967026297001480, 1998.
Paasche, E.: A review of the coccolithophorid Emiliania huxleyi (Prymnesiophyceae), with particular reference to growth, coccolith formation, and calcification-photosynthesis interactions, Phycologia, 40, 503–529, https://doi.org/10.2216/i0031-8884-40-6-503.1, 2002.
Pedro Cañavate, J., Armada, I., and Hachero-Cruzado, I.: Common and species-specific effects of phosphate on marine microalgae fatty acids shape their function in phytoplankton trophic ecology, Microb. Ecol., 74, 623–639, https://doi.org/10.1007/s00248-017-0983-1, 2017.
Perrin, L., Probert, I., Langer, G., and Aloisi, G.: Growth of the coccolithophore Emiliania huxleyi in light- and nutrient-limited batch reactors: relevance for the BIOSOPE deep ecological niche of coccolithophores, Biogeosciences, 13, 5983–6001, https://doi.org/10.5194/bg-13-5983-2016, 2016.
Piepho, M., Arts, M. T., and Wacker, A.: Species-specific variation in fatty acid concentrations of four phytoplankton species: does phosphorus supply influence the effect of light intensity or temperature?, J. Phycol., 48, 64–73, https://doi.org/10.1111/j.1529-8817.2011.01103.x, 2012.
Pierrot, D., Lewis, E., and Wallace, D.: MS Excel program developed for CO2 system calculations: ORNL/CDIAC-105a, Carbon Dioxide Information Analysis Centre, Oak Ridge National Laboratory, US Department of Energy, Oak Ridge, TN, 2006.
Pronina, N. A., Rogova, N. B., Furnadzhieva, S., and Klyachko-Gurvich, G. L.: Effect of CO2 concentration on the fatty acid composition of lipids in Chlamydomonas reinhardtii cia-3, a mutant deficient in CO2-concentrating mechanism, Russ. J. Plant Physiol., 45, 447–455, 1998.
Provasoli, L.: Growing marine seaweeds, in: Proc. 4th Internatl, Seaweed Symp., edited by: De Virville, A. D. and Feldmann, J., Pergamon Press, Oxford, UK, 9–17, 1963.
Raitsos, D. E., Lavender, S. J., Pradhan, Y., Tyrrell, T., Reid, P. C., and Edwards, M.: Coccolithophore bloom size variation in response to the regional environment of the subarctic North Atlantic, Limnol. Oceanogr., 51, 2122–2130, https://doi.org/10.4319/lo.2006.51.5.2122, 2006.
Read, B. A., Kegel, J., Klute, M. J., Kuo, A., Lefebvre, S. C., Maumus, F., Mayer, C., Miller, J., Monier, A., Salamov, A., Young, J., Aguilar, M., Claverie, J. M., Frickenhaus, S., Gonzalez, K., Herman, E. K., Lin, Y. C., Napier, J., Ogata, H., Sarno, A. F., Shmutz, J., Schroeder, D., de Vargas, C., Verret, F., von Dassow, P., Valentin, K., Van de Peer, Y., Wheeler, G., Allen, A. E., Bidle, K., Borodovsky, M., Bowler, C., Brownlee, C., Cock, J. M., Elias, M., Gladyshev, V. N., Groth, M., Guda, C., Hadaegh, A., Iglesias-Rodriguez, M. D., Jenkins, J., Jones, B. M., Lawson, T., Leese, F., Lindquist, E., Lobanov, A., Lomsadze, A., Malik, S. B., Marsh, M. E., Mackinder, L., Mock, T., Mueller-Roeber, B., Pagarete, A., Parker, M., Probert, I., Quesneville, H., Raines, C., Rensing, S. A., Riano-Pachon, D. M., Richier, S., Rokitta, S., Shiraiwa, Y., Soanes, D. M., van der Giezen, M., Wahlund, T. M., Williams, B., Wilson, W., Wolfe, G., Wurch, L. L., Dacks, J. B., Delwiche, C. F., Dyhrman, S. T., Gloeckner, G., John, U., Richards, T., Worden, A. Z., Zhang, X. Y., and Grigoriev, I. V.: Pan genome of the phytoplankton Emiliania underpins its global distribution, Nature, 499, 209–213, https://doi.org/10.1038/nature12221, 2013.
Renaud, S. M., Thinh, L.-V., Lambrinidis, G., and Parry, D. L.: Effect of temperature on growth, chemical composition and fatty acid composition of tropical Australian microalgae grown in batch cultures, Aquaculture, 211, 195–214, https://doi.org/10.1016/S0044-8486(01)00875-4, 2002.
Riebesell, U., Revill, A. T., Holdsworth, D. G., and Volkman, J. K.: The effects of varying CO2 concentration on lipid composition and carbon isotope fractionation in Emiliania huxleyi, Geochim. Cosmochim. Ac., 64, 4179–4192, https://doi.org/10.1016/s0016-7037(00)00474-9, 2000.
Rokitta, S. D. and Rost, B.: Effects of CO2 and their modulation by light in the life-cycle stages of the coccolithophore Emiliania huxleyi, Limnol. Oceanogr., 57, 607–618, https://doi.org/10.4319/lo.2012.57.2.0607, 2012.
Rosas-Navarro, A., Langer, G., and Ziveri, P.: Temperature affects the morphology and calcification of Emiliania huxleyi strains, Biogeosciences, 13, 2913–2926, https://doi.org/10.5194/bg-13-2913-2016, 2016.
Rosenblatt, A. E. and Schmitz, O. J.: Climate change, nutrition, and bottom-up and top-down food web processes, Trends Ecol. Evol., 31, 965–975, https://doi.org/10.1016/j.tree.2016.09.009, 2016.
Rossoll, D., Bermúdez, R., Hauss, H., Schulz, K. G., Riebesell, U., Sommer, U., and Winder, M.: Ocean acidification-induced food quality deterioration constrains trophic transfer, Plos One, 7, e34737, https://doi.org/10.1371/journal.pone.0034737, 2012.
Rost, B. and Riebesell, U.: Coccolithophores and the biological pump: responses to environmental changes, in: Coccolithophores: From molecular processes to global impact, edited by: Thierstein, H. R. and Young, J. R., Springer, Heidelberg, Germany, 99–125, 2004.
Sato, N., Tsuzuki, M., and Kawaguchi, A.: Glycerolipid synthesis in Chlorella kessleri 11 h – Part II. Effect of the CO2 concentration during growth, BBA-Mol. Cell Biol. L., 1633, 35–42, https://doi.org/10.1016/s1388-1981(03)00070-2, 2003.
Schiettecatte, L. S., Thomas, H., Bozec, Y., and Borges, A. V.: High temporal coverage of carbon dioxide measurements in the Southern Bight of the North Sea, Mar. Chem., 106, 161–173, https://doi.org/10.1016/j.marchem.2007.01.001, 2007.
Sett, S., Bach, L. T., Schulz, K. G., Koch-Klavsen, S., Lebrato, M., and Riebesell, U.: Temperature modulates coccolithophorid sensitivity of growth, photosynthesis and calcification to increasing seawater pCO2, Plos One, 9, e88308, https://doi.org/10.1371/journal.pone.0088308, 2014.
Sharp, J.: Improved analysis for particulate organic carbon and nitrogen from seawater, Limnol. Oceanogr., 19, 984–989, https://doi.org/10.4319/lo.1974.19.6.0984, 1974.
Sinensky, M.: Homeoviscous adaptation – a homeostatic process that regulates the viscosity of membrane lipids in Escherichia coli, P. Natl. Acad. Sci. USA, 71, 522–525, https://doi.org/10.1073/pnas.71.2.522, 1974.
Skau, L. F.: Effects of temperature and phosphorus on growth, stoichiometry and size in three haptophytes, M.S. thesis, Centre for Ecological and Evolutionary Synthesis (CEES), Section for Aquatic Biology and Toxicology (AQUA), University of Oslo, Oslo, Norway, 64 pp., 2015.
Sommer, U., Peters, K. H., Genitsaris, S., and Moustaka-Gouni, M.: Do marine phytoplankton follow Bergmann's rule sensu lato?, Biol. Rev., 92, 1011–1026, https://doi.org/10.1111/brv.12266, 2016.
Sorrosa, J. M., Satoh, M., and Shiraiwa, Y.: Low temperature stimulates cell enlargement and intracellular calcification of Coccolithophorids, Mar. Biotechnol., 7, 128–133, https://doi.org/10.1007/s10126-004-0478-1, 2005.
Sterner, R. W. and Elser, J. J.: Ecological stoichiometry: The biology of elements from molecules to the biosphere, Princeton University Press, Princeton, USA, 2002.
Sterner, R. W. and Schulz, K.: Zooplankton nutrition: recent progress and a reality check, Aquat. Ecol., 32, 261–279, https://doi.org/10.1023/A:1009949400573, 1998.
Terry, K. L., Laws, E. A., and Burns, D. J.: Growth rate variation in the N : P requirement ratio of phytoplankton, J. Phycol., 21, 323–329, 1985.
Thompson, G. A.: Lipids and membrane function in green algae, BBA-Lipid Lipid Met., 1302, 17–45, https://doi.org/10.1016/0005-2760(96)00045-8, 1996.
Thompson, P. A., Guo, M.-X., Harrison, P. J., and Whyte, J. N. C.: Effects of variation in temperature, II. On the fatty acid composition of eight species of marine phytoplankton, J. Phycol., 28, 488–497, https://doi.org/10.1111/j.0022-3646.1992.00488.x, 1992.
Toseland, A., Daines, S. J., Clark, J. R., Kirkham, A., Strauss, J., Uhlig, C., Lenton, T. M., Valentin, K., Pearson, G. A., Moulton, V., and Mock, T.: The impact of temperature on marine phytoplankton resource allocation and metabolism, Nature Climate Change, 3, 979–984, https://doi.org/10.1038/nclimate1989, 2013.
Tyrrell, T. and Merico, A.: Emiliania huxleyi: bloom observations and the conditions that induce them, in: Coccolithophores: From molecular processes to global impact, edited by: Thierstein, H. R. and Young, J. R., Springer, Heidelberg, Germany, 75–97, 2004.
van Bleijswijk, J. D. L., Kempers, R. S., Veldhuis, M. J., and Westbroek, P.: Cell and growth characteristics of types A and B of Emiliania huxleyi (Prymnesiophyceae) as determined by flow cytometry and chemical analyses, J. Phycol., 30, 230–241, https://doi.org/10.1111/j.0022-3646.1994.00230.x, 1994.
Van Mooy, B. A. S., Fredricks, H. F., Pedler, B. E., Dyhrman, S. T., Karl, D. M., Koblizek, M., Lomas, M. W., Mincer, T. J., Moore, L. R., Moutin, T., Rappe, M. S., and Webb, E. A.: Phytoplankton in the ocean use non-phosphorus lipids in response to phosphorus scarcity, Nature, 458, 69–72, https://doi.org/10.1038/nature07659, 2009.
Winter, A., Henderiks, J., Beaufort, L., Rickaby, R. E. M., and Brown, C. W.: Poleward expansion of the coccolithophore Emiliania huxleyi, J. Plankton Res., 36, 316–325, https://doi.org/10.1093/plankt/fbt110, 2014.
Xing, T., Gao, K., and Beardall, J.: Response of growth and photosynthesis of Emiliania huxleyi to visible and UV irradiances under different light regimes, Photochem. Photobiol., 91, 343–349, https://doi.org/10.1111/php.12403, 2015.