the Creative Commons Attribution 3.0 License.
the Creative Commons Attribution 3.0 License.
Plant n-alkane production from litterfall altered the diversity and community structure of alkane degrading bacteria in litter layer in lowland subtropical rainforest in Taiwan
Tung-Yi Huang
Bing-Mu Hsu
Wei-Chun Chao
Cheng-Wei Fan
n-Alkane and alkane-degrading bacteria have long been used as crucial biological indicators of paleoecology, petroleum pollution, and oil and gas prospecting. However, the relationship between n-alkane and alkane-degrading bacteria in natural forests is still poorly understood. In this study, long-chain n-alkane (C14–C35) concentrations in litterfall, litter layer, and topsoil as well as the diversity and abundance of n-alkane-degrading bacterial communities in litter layers were investigated in three habitats across a lowland subtropical rainforest in southern Taiwan: ravine, windward, and leeward habitats in Nanjenshan. Our results demonstrate that the litterfall yield and productivity of long-chain n-alkane were highest in the ravine habitats. However, long-chain n-alkane concentrations in all habitats were decreased drastically to a similar low level from the litterfall to the bulk soil, suggesting a higher rate of long-chain n-alkane degradation in the ravine habitat. Operational taxonomic unit (OTU) analysis using next-generation sequencing data revealed that the relative abundances of microbial communities in the windward and leeward habitats were similar and different from that in the ravine habitat. Data mining of community amplicon sequencing using the NCBI database revealed that alkB-gene-associated bacteria (95 % DNA sequence similarity to alkB-containing bacteria) were most abundant in the ravine habitat. Empirical testing of litter layer samples using semi-quantitative polymerase chain reaction for determining alkB gene levels confirmed that the ravine habitat had higher alkB gene levels than the windward and leeward habitats. Heat map analysis revealed parallels in pattern color between the plant and microbial species compositions of the habitats, suggesting a causal relationship between the plant n-alkane production and microbial community diversity. This finding indicates that the diversity and relative abundance of microbial communities in the litter layer are affected by n-alkane plant composition in the litterfall.
- Article
(874 KB) - Full-text XML
-
Supplement
(1884 KB) - BibTeX
- EndNote
Alkanes are saturated hydrocarbons that account for a small fraction of the total organic carbon, with a concentration range in natural habitats of not more than a couple of thousand parts per million by weight (Rojo, 2010; Gomez-Coca et al., 2016). In non-contaminated habitats, long-chain n-alkanes with an odd/even predominance are produced by plants or algae to act as chemoattractants or agents that protect against microbial invasion or water loss (Koch et al., 2009; Feakins et al., 2016). Alkanes are exceptionally stable molecules that can survive in soil and sediment, which make them useful as biomarkers in paleoecology (Sachse et al., 2012). Because alkanes are the major components in petroleum, natural gas, and diesel fuel, the presence of environmental alkanes also serves as an index of oil contamination (Afzal et al., 2013). Although alkanes are very stable, most can be degraded by several microorganisms. Alkane-degrading bacteria are commonly upregulated when the concentration of alkanes is increased; it has thus been suggested that these bacteria be used as a biological indicator for oil and gas prospecting (Rasheed et al., 2012; Xu et al., 2013).
Extensive knowledge on the degradation of n-alkane by microorganisms has been acquired in the past decades. The first step of enzymatic reaction is oxidization of n-alkane to the corresponding primary alcohol by alkane terminal hydroxylases. This step is crucial because activation of the alkane molecule requires an enzyme system that is not widespread (Rojo, 2010). The products can be further oxidized into a corresponding aldehyde and fatty acid, which are digested by most microorganisms. Although fungi and yeasts can degrade alkanes, most research has focused on the role of bacteria in the degradation of alkanes (Rojo, 2009, 2010; Singh et al., 2012). To date, alkane monooxygenase gene (alkB) hydroxylases are the most commonly found alkane hydroxylases in both Gram-negative and Gram-positive bacteria. More than 60 genera of aerobic bacteria and 5 genera of anaerobic bacteria are known to degrade n-alkanes (Nie et al., 2014). Although alkanes can be metabolized anaerobically, the growth of anaerobic alkane degraders is significantly slower than that of aerobic ones, especially in the litter layer environment where the oxygen supply is adequate (Wentzel et al., 2007). The alkB-encoded membrane-bound homologous protein is an inducible integral membrane-bound alkane hydroxylase that plays a vital role in aerobic alkane degradation (Beilen et al., 2003). Evidence has revealed that the number of alkB bacteria dynamically changes in response to a fluctuation in environmental alkanes. In soil or water environments, when polluted with crude oil, the alkane-degrading bacteria were upregulated (Afzal et al., 2013). For example, a 10-fold increase in the relative abundance of oil degraders in contaminated versus clean sand was estimated for a Gulf of Mexico beach (Kostka et al., 2011). In oil and gas reservoirs, it has been shown that the abundance of alkB bacteria was substantially higher in the surface soils with no significant detection of soil alkane (Xu et al., 2013). The dynamic relationship between alkane and alkB bacteria in soil from an agriculture research farm and a laboratory maintained under controlled conditions has been previously studied. Giebler et al. (2013) reported that in a controlled environment, sizeable communities of alkane-degrading bacteria, especially Proteobacteria and Actinobacteria, were detected at the soil–litter interface when it was enriched with artificial alkane supplements. The researchers demonstrated that many of the alkane-degrading bacteria were not detected before the alkanes were supplemented, suggesting that some alkane degraders may have been present in very low copy numbers and below the limits of detection. It is reported that the substrate was discovered to modulate the biomass of microorganisms based on its initial concentration (Schmidt, 1992). Thus, different alkane yields of litterfall in various natural habitats may upregulate the growth of various alkane degraders. The relationship between alkB bacteria abundance and alkane substrate is more complicated in natural habitats. The dynamic changes in alkB-degrading bacteria populations were demonstrated to be driven by numerous factors, such as the original source of the alkane and soil type. Although knowledge of the regulatory mechanisms regarding environmental plant litter on soil bacterial levels and of the dynamics of alkane-degrading bacteria in natural habitats has been beneficial and important in many aspects, studies on their dynamic changes are currently lacking and should be conducted (Schulz et al., 2012).
The Nanjenshan Reserve, a lowland subtropical rainforest in southern Taiwan, consists of several dynamic forest plots for which forest structure, vegetation pattern, climate, and topography data have been collected at well-established permanent study sites. Four dynamic forest plots and a transect zone in the habitat have been documented since 1989 (Chen and Huang, 1986; Hsieh et al., 2000; Tsui et al., 2004; Fan et al., 2005; Chao et al., 2007, 2008; Chao et al., 2010). Among them, three plots captured our attention, namely plot I (120∘50′ 51 E, 22∘04′54 N), plot II (120∘50′ 36 E, 22∘04′52 N), and the Lanjenchi plot (120∘51′ 38 E, 22∘03′ 23 N). A ravine habitat is located across plots I and II, whereas leeward and windward habitats are located on plot III. Surveys conducted over the past decade have demonstrated that the annual yield of litterfall in the ravine habitat was higher than that in the windward and leeward habitats. Moreover, studies have shown that in the leeward habitat, plant height was higher and plant density was lower when compared with the windward habitat (8.41 ± 1.73 vs. 4.63 ± 0.88 m; 7505 vs. 20 065 trees ha−1). The plant height and density in the ravine habitat were 9.45 ± 1.35 m and 4257 trees ha−1 (Chin, 2008). In addition, the litterfall plays an essential role in sustaining the microorganism food chain in the forest ecosystem. The litter-leaf, the main component of litterfall, similarly provides the major source of plant-derived alkanes. The significant effects of litterfall derived alkanes on the bacterial alkane degradation potential have also been observed (Giebler et al., 2013 and Schulz et al., 2012). As the litterfall in the habitats of the Nanjenshan Reserve was different, investigation into the effects of litterfall on various habitats and microbial communities in these habitats is possible.
Environmental microbial communities are highly complex and diverse and difficult to culture under laboratory conditions. Next-generation sequencing (NGS) is a powerful tool for high throughput sequencing technologies. With this recent advent platform and computational methods, we can conduct genome studies of microbes in these habitats, which have numerous variables such as annual litterfall productivity and plant vegetation (Degnan and Ochman, 2012). We investigated the productivity of long-chain n-alkane in the pristine natural habitats of the Nanjenshan Reserve and explored their relationship with microbial communities, particularly emphasizing the correlation between n-alkane production and bacteria containing the alkB gene. During the present 1-year follow-up study, gas chromatography with a flame ionization detector (GC-FID) was used to determine n-alkane (C14–C35) concentrations. Bioinformatics analyses and community amplicon sequencing data mining were performed to identify microbial communities and read numbers of bacterial lineages that contained alkB genes in the three rainforest habitats. Semi-quantitative polymerase chain reaction (PCR) was performed to test the results of the bioinformatics analysis.
2.1 Sample description
A map of the sampling sites is provided in Fig. S1 in the Supplement. Twenty-two custom-made aluminum stands with frames (0.71 m × 0.71 m) covered by a nylon mesh (1 mm i.d.) were used to collect litterfall. Twelve stands were located in the ravine habitat, whereas 10 stands were located in the Lanjenchi plot. Of those on the Lanjenchi plot, six stands were placed in the windward habitat and four in the leeward habitat. The horizontal distance between stands was approximately 50 m. The number of stands in the three habitats of the Nanjenshan Reserve was mainly selected based on the stands available in this area. Each nylon mesh containing litterfall was collected and replaced monthly from early October 2012 to late September 2013. After their collection, the litterfall samples were sorted into litter-leaf, litter-branches, litter-flowers, litter-fruits, and miscellaneous fractions. The sorted samples were weighed after being oven-dried at 40 ∘C for 14 days (Conklin-Brittain et al., 2006). The litter-leaves were further separated into genus and species. Samples of the litter layer and underlying soils from the three habitats were collected in 2015 and 2016, respectively. The collected samples, including litter-leaf, litter layer, and soils, were subjected to n-alkane analysis, and litter layer samples from each habitat were further subjected to DNA extraction and consequential assays.
The annual yield (litterfall or litter-leaf) in each stand was used to calculate the productivity of each habitat. The annual litterfall and litter-leaf yields in each habitat were calculated by averaging the annual stand weights. Statistically significant differences between habitats were evaluated through Student's t test using the t test calculator (SD format).
2.2 Extraction of n-alkanes
All samples from the litter-leaf (42 plant species) and litter layer and all soils were dried and well homogenized. Approximately 0.5 g of sample powder was dissolved in dichloromethane (250 mL) and Soxhlet extracted for 16 h. A surrogate standard of 5-α-cholestane (Sigma) was added to each sample before extraction. The extracts were concentrated into 5–10 mL volumes using a rotary evaporator with a water bath and further concentrated to reduce the volume to approximately 1 mL, after which they were fractionated using silica gel chromatography (Silica gel 60, 3 %). The n-alkane fraction was eluted with 4 mL of hexane and reduced to a 1 mL volume under a stream of nitrogen. An internal standard of squalene (Acros) was added to the concentrated 1 mL of hexane fraction prior to instrumental GC analysis. Quantification of the n-alkanes was performed using a PerkinElmer Clarus 500 gas chromatograph equipped with an autosampler, PerkinElmer Elite-5 CB fused silica capillary column (30 m in length, 0.32 mm i.d., film thickness 0.25 µm), and flame ionization detector (FID). The GC oven temperature was programmed to increase from 70 to 310 ∘C at a rate of 4 ∘C min−1. The n-alkanes between C14 and C35 were identified and quantified using external standards that contained known concentrations of all n-alkanes of interest (Dr. Ehrenstorfer GmbH), 5-α-cholestane, and squalene.
The n-alkane concentrations in 42 litter-leaf samples were measured and then used to estimate the n-alkane concentration from the litter-leaf and to assess litter-leaf productivity in the three habitats. The n-alkane concentration in the litter-leaf from each stand (Cstand, in µg g−1) was summed by weighting the concentration in one species in the litter-leaf by that species' proportion of the total litter-leaf collected in the stand as in the following equation:
where Cstand is n-alkane concentration in the litter-leaf from each stand (µg g−1), Wi is the dry weight of litter-leaf of each species (g), Wt is the dry weight of total litter-leaf (g), and Ci is the n-alkane concentrations (µg g−1) of 42 litter-leaf samples.
Calculation of the litter-leaf n-alkane concentration in each habitat was then performed by averaging the n-alkane litter-leaf concentrations in all the stands. The annual n-alkane productivity in each stand was calculated by multiplying the n-alkane litter-leaf concentration in each stand by the annual yield of that stand. The n-alkane litter-leaf productivities of the stands in a habitat were then averaged to estimate the annual n-alkane productivity in the three habitats, which was evaluated using Student's t test.
2.3 DNA extraction
For DNA extraction in the litter layer, several pieces of leaves from the litter layer were randomly selected, cut into 2 mm × 2mm chips, and well blended. The DNA extraction procedure is described in detail in the user manual, Genomic DNA from soil (Macherey-Nagel). Briefly, a bulk mixture (approximately 0.1–0.2 g) was transferred to a tube containing ceramic beads. Lysis buffer (SL1, 700 µL) and enhancer SX (150 µL) were added to each sample and vortexed for 5 min at room temperature. Precipitate contaminants were removed through centrifugation for 2 min at 11 000 g. DNA supernatant was transferred to a new tube, 150 µL of SL3 was added, and the mixture was vortexed for 5 s before precipitation by centrifugation for 1 min at 11 000 g. The supernatant inhibitors were removed using an inhibitor removal column. The column that bound the DNA was washed four times and dried before DNA elution. DNA elution was performed by centrifugation for 30 s at 11 000 g after incubation with Buffer SE (50 µL). A final DNA extraction (approximately 30–100 ng µL−1 in 100 µL of Tris buffer) was eluted.
2.4 Sequencing using an Illumina MiSeq platform
The genomic DNA (12.5 ng) obtained from each habitat was used in amplicon PCR experiments, which were performed in triplicate. We performed PCR amplification of 16S rRNA gene sequences at the V3–V4 regions using Illumina's MiSeq system to create paired-end sequencing data. The experimental protocol was modified from the Illumina manual. The target sequence was amplified using PCR with mixed forward and reverse primers. The sequences of forward primers included an adapter overhang nucleotide sequence and V3–V4 primer pairs, which were 5'-TCGTC GGCAG CGTCA GATGT GTATA AGAGA CAGCC TACGG GNGGC WGCAG, 5'-TCGTC GGCAG CGTCA GATGT GTATA AGAGA CAGAC CTACG GGNGG CWGCA G, 5'-TCGTC GGCAG CGTCA GATGT GTATA AGAGA CAGTD CCTAC GGGNG GCWGC AG, and 5'-TCGTC GGCAG CGTCA GATGT GTATA AGAGA CAGGD RCCTA CGGGN GGCWG CAG. The sequences of the reverse primers were 5'-GTCTC GTGGG CTCGG AGATG TGTAT AAGAG ACAGG ACTAC HVGGG TATCT AATCC, 5'-GTCTC GTGGG CTCGG AGATG TGTAT AAGAG ACAGT GACTA CHVGG GTATC TAATC C, 5'-GTCTC GTGGG CTCGG AGATG TGTAT AAGAG ACAGA CGACT ACHVG GGTAT CTAAT CC, and 5'-GTCTC GTGGG CTCGG AGATG TGTAT AAGAG ACAGG TTGAC TACHV GGGTA TCTAA TCC. After separation using agarose gel electrophoresis, PCR products with expected sizes were purified from the matrix. The Illumina Nextera XT index kit was used in second-stage PCR for the addition of the index. Capillary electrophoresis followed by a fluorescence-based method was employed to qualify and quantify libraries, respectively. Nucleotides were sequenced using the MiSeq sequencer for 18 dark cycles and 350 read cycles in the forward read, and 18 dark cycles and 250 read cycles in the reverse read. The data of forward and reverse reads were aligned using the analysis platform from (Genomic Workbench v.8.5) CLC bio with Q20 as a threshold to generate output FASTA files.
2.5 The construction and analysis of community amplicon sequencing library
FASTA files were further processed using the sequence analysis tool, USEARCH. We merged all sequence files, removed duplicates, and clustered the sequences into operational taxonomic units (OTUs) at 97 % pairwise identity with a minimum cluster size of two to construct an OTU reference library. Then, a comparison between samples and the reference library at a 97 % sequence identity level was performed to create OTU tables, which contained the number of DNA sequence reads of each OTU. A 16S UTAX reference database was employed as a BLAST (Basic Local Alignment Search Tool) library. Finally, these data were combined to determine the relative abundances and subsequent visual patterns of a heat map. Principal coordinate analysis (PCoA) was used to identify the relative distance between OTUs. To plot PCoA figures, the calc_distmx command together with a phylip_lower_triangular parameter was used to calculate the distance of the OTUs. The axes file contained plotting coordinates and was derived from mothur using the PCoA command. The PCoA figures were plotted using the R program.
2.6 Proportion of alkB-gene-containing bacteria in the habitats estimated using the NCBI database
Data mining for community amplicon sequencing was performed to search for microbial communities that may have had alkB genes. Because bacteria in the same genus may have similar gene sequences and functions, nucleotide blasting was performed to search for homogeneous sequences in the OTUs. Bacteria with DNA sequences 95 % or more similar to a known gene were defined as lineage-like bacteria. The NCBI database was used to construct an alkB gene reference library. We collected representative 16s gene sequences of all known alkB-gene-containing bacteria in the phyla Actinobacteria and Proteobacteria from the current NCBI database to investigate the alkB gene family populations in different habitats. The alkB gene sequences in bacterial 16s rRNA were downloaded to a reference nucleotide file and combined with the OTU libraries of Actinobacteria and Proteobacteria.
To search the alkB family gene-like lineage in the current OTU database, sequence alignment and phylogenetic analysis of the alkB nucleotide sequences were conducted using the Molecular Evolutionary Genetics Analysis 7 (MEGA 7) program. MEGA 7 used parsimony, neighbor-joining, and maximum likelihood analyses to create a 16S rRNA phylogenetic gene tree. The NCBI nucleotide BLAST program was employed to test the similarity of DNA sequences between OTUs and adjacent references. DNA sequences with a similarity of more than 95 % were manually selected to determine the alkB-lineage OTU DNA sequence numbers read in the library. The Shannon–Wiener diversity index was used to calculate the plant diversity, overall bacteria communities, and alkB-lineage bacteria and was determined using the PRIMER-5 software (Plymouth Routines in Multivariate Ecological Research).
2.7 Semi-quantitative PCR for determining alkB gene levels in litter layer samples
Because alkB genes are highly abundant and diverse, a standard method for alkB gene quantification was not available. We employed semi-quantitative PCR for measuring environmental alkB in a method that was modified from previous studies because the alkB-targeting primer covers Proteobacteria and Actinobacteria (Kloos et al., 2006; Jurelevicius et al., 2013). Litter layer samples from three habitats in Nanjenshan were subjected to semi-quantitative PCR to quantify the DNA levels of alkB genes in the natural habitats. Litter layer samples from different stands in each habitat were randomly selected for sample collection at three time points. After collection, litter layer samples were sent immediately to the laboratory at room temperature. Litter layer patches were randomly selected and cut into approximately 2 mm × 2 mm chips. Samples (250 mg) were taken for DNA extraction. Following DNA elution, 100 µL of buffer solution and 2 µL of eluted DNA were taken for semi-quantitative PCR. The DNA was mixed with an alkB gene primer set (forward primer: 5'- AAY ACN GCN CAY GAR CTN GGN CAY AA -3'; reverse primer: 5'- GCR TGR TGR TCN GAR TGN CGY TG -3', 1 µL, 0.4 µM), 5 µL Fast-Run Taq Master Mix with Dye, and 16 µL ddH2O with the final reaction volume of 25 µL. Thermal cycling conditions for PCR were an initial temperature of 95 ∘C for 5 min, followed by 30, 27, or 24 cycles of 95 ∘C for 30 s, 55 ∘C for 30 s, 72 ∘C for 1 min, and finally 72 ∘C for 5 min. The expected amplicons of the PCR were 548 bps. Following PCR, aliquots (5 µL) of each amplicon were confirmed using electrophoresis on 1.5 % agarose gel followed by 0.5 µg mL−1 SYBR Safe DNA staining and imaging using an ultraviolet transillumination system. The average band density levels were compared with a windward habitat density setting of 100 %. Statistically significant differences were evaluated using Student's t test.
3.1 Quantitative estimates of annual litterfall in the Nanjenshan Reserve
The annual average litterfall was estimated from the litterfall at the sampling sites. The annual yields of litterfall in the three habitats in Nanjenshan are illustrated in Fig. 1. As shown in the records from the past decade, the litterfall was higher in the ravine habitat than in the other habitats. Our results on the litterfall of Nanjenshan indicated that the annual weight productivity in the ravine habitat was approximately 30 % higher than in the leeward or windward habitats, which is consistent with previous studies. According to records from 1999 to 2007, seasonal litterfall in Nanjenshan ranged from 2 to 7 t ha−1; the increases in litterfall were contributed by typhoons, precipitation, and the effects of monsoon seasons. In this study, the annual litterfall was discovered to be approximately 7–10 t ha−1, which is considered to be a high volume when compared with the records. The primary reason for the large amount of litterfall during this study was the occurrence of typhoons. Because typhoons increase litterfall in the three habitats equally, the ranking in annual litterfall volume was not altered by the advent of typhoons. One of the reasons that the ravine habitat had more annual litterfall may be the topography of the ravine habitat, which is more suitable for plant growth than that of the other habitats. The average height and diameter of cross sections of plants in the ravine habitat were the largest of those in all the habitats. Because litter-leaf was the major part of litterfall and average n-alkane resources, we focused on n-alkane levels in the litter-leaf in these habitats.
3.2 Example of n-alkane measurements in the Nanjenshan Reserve
The n-alkane concentrations in litter-leaf from 42 plants were measured using GC-FID. Figure S2 presents representative GC chromatograms of the Ilex rotunda leaves in each habitat. The recovery rates from the surrogate of 5-α-cholestane ranged from 70 to 108 % and averaged at 99 ± 7 %, suggesting satisfactory extraction efficiency. The n-alkane concentrations (ranging from 123 to 2694 µg g−1) calculated in this study were not corrected by the recovery rate. All samples exhibited an odd/even carbon number predominance, with maximums at C31, C29,, and C33. n-Alkanes of C14 to C26 were present in only relatively low amounts. Further distribution analyses of the n-alkane litter-leaf components, such as the carbon preference index (CPI) and C29 ∕ C33 ratio, for each species, were needed to further identify the relationships between plant species and n-alkane distribution patterns. This paper is the first to reveal the n-alkane levels of plant vegetation in Nanjenshan.
Table 1The resultant ranking of n-alkane concentration of 42 litter-leaf samples with the nomenclature and the semi-quantitative results of annual litter-leaf productivity in three habitats. The plus sign (+) was used to denote the annual productivity rankings of litter-leaf in each habitat. Five plus signs ( denote the litter-leaf productivity rankings from 1 to 5; four plus signs ( denote the productivity rankings from 6 to 10; three plus signs ( denote the rankings from 11 to 15; two plus signs ( denote the rankings from 16 to 20; one plus sign (+) denotes the rankings after 21. A blank space denotes no litter-leaf yield for the entire year in the habitat.
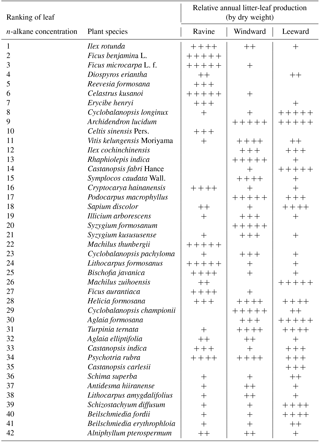
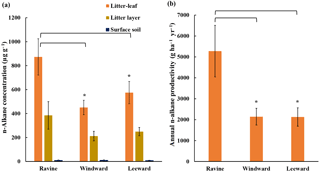
Figure 2(a) n-Alkane concentration in litter-leaf, litter layer, and surface soil. n-Alkane concentration in litter-leaf was higher in the ravine habitat than in the other two habitats (* p < 0.05). (b) Estimated annual n-alkane productivity generated by litter-leaf of litterfall in three habitats (* p < 0.05).
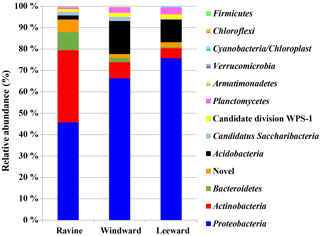
Figure 3Microbial community structure in the three habitats of Nanjenshan Reserve. Bacterial lineages were indicated by phylum.
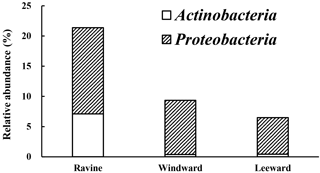
Figure 4The relative abundance in microbial community of alkB gene lineages. White block indicated the relative abundance of alkB gene in phylum Actinobacteria while the striped block was in Proteobacteria.
3.3 Annual litter-leaf productivity and n-alkane productivity rankings
A total of 42 plant species were identified in this study. Data from the plant species revealed the total amount of leaves in the annual litterfall, and the resultant ranking of leaf n-alkane concentrations in these plants is presented in Table 1. The leaf n-alkane concentration and dry weight data were employed to make a gross estimate of the n-alkane productivity of the litter-leaf in the Nanjenshan Reserve. The n-alkane concentration was discovered to change during leaf development and was affected by environmental parameters (Jetter and Schäffer, 2001; Kahmen et al., 2011; Hoffmann et al., 2013). For example, temperature and relative humidity affected the composition of n-alkanes in both Acacia and Eucalyptus in Australia (Hoffmann et al., 2013). In our pilot study, the n-alkane concentration variation among plant species was less than 15 % of the standard error, as determined through comparison of several samples from different stands and habitats for the same species. The ranges of leaf n-alkane concentrations between species were from 2694 (µg g−1) in Ilex rotunda to 123 (µg g−1) in Alniphyllum pterospermum. These data demonstrated that intraspecies variation in n−alkane concentration could be ignored compared with the interspecies variation. Therefore, using a representative plant n-alkane concentration was reasonable for estimating the overall litter-leaf n-alkane production in a habitat. When comparing the plant vegetation and leaf n-alkane concentration, the plants with leaves that had a high n-alkane concentration tended to grow in the ravine habitat; Ilex rotunda and Celastrus kusanoi, for example.
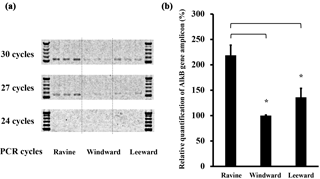
Figure 6Semi-quantitative PCR of alkB gene results from samples of three habitats in Nanjenshan Reserve. (a) Agarose electrophoresis of alkB genes in different amplified cycles. Standard markers were loaded at both sides of samples. The samples from different habitats were separated by dash line. (b) The statistic results of semi-quantitative PCR after amplifying 30 cycles. The read numbers of alkB gene were significantly higher in the ravine habitat than in the other two habitats (* p < 0.05).
3.4 Estimates of annual n-alkane productivity in the three habitats
Figure 2 presents the n-alkane concentrations from the litter-leaf to the bulk soil and the average litter-leaf n-alkane productivity in the three habitats. The average litter-leaf n-alkane concentration was highest in the ravine habitat. Moreover, the n-alkane concentration in different layers formed a steep gradient from the litterfall to the bulk soil. The n-alkane flux in the litterfall in the ravine habitat was approximately twice that in the other habitats. We concluded that the ravine habitat had higher n-alkane input than the other habitats. This characteristic offers us an example of higher n-alkane input in the natural habitat to investigate the consequence of microbial community.
The balance between litterfall yield and decomposition affects the development of organic carbon in soil layers. This study and previous reports have indicated that the litterfall in ravine habitats is higher than that in other habitats. However, a study of these habitats demonstrated that the total organic carbon in the litter layer and bulk soil in the ravine habitat was equal to or less than that in the windward and leeward habitats (Guo, 2010). Therefore, decomposition effects may be stronger in ravine habitats. Figure 2a reveals that the n-alkane concentration decreased significantly from litter-leaf to surface soil, which could be due to the effects of bacteria. It is plausible that other organic compounds were degraded in addition to the n-alkanes. Several effects such as leaching, erosion, and sediment transportation could play roles in governing organic compound accumulation; therefore, we cannot rule out the diffusion effects of other physical factors (Quinton et al., 2006; Kirkels et al., 2014). We performed NGS-based analysis and bioinformatics studies to discover the roles of microbial communities in n-alkane degradation.
3.5 Bacterial community composition in the Nanjenshan Reserve
NGS-based study was performed to reveal the microbial communities in the litter layer from the three habitats. Figure 3 shows the relative abundance in community amplicon sequencing data of OTUs that were grouped by phylum. More detailed information about the relative abundance in class, order, and family levels is presented in Fig. S5. Interestingly, a similarity was identified in microbial community structure between the windward and leeward habitats. Because the windward and leeward habitats are located on the same plot of a different orography, several parameters such as plant vegetation and soil properties were similar between the habitats. As mentioned previously, the most considerable differences between the windward and leeward habitats were the average height of plants and average number of trees per hectare. The physical parameters that affect plant growth in mountainous areas did not appear to affect the relative abundance of microbial communities. Conversely, the relative abundance of microbial communities in the ravine habitat was different from that in the windward and leeward habitats, with more members of the Actinobacteria phyla and fewer members of the Proteobacteria and Acidobacteria phyla. The more abundant microbial communities of the Actinobacteria phyla may have been because of higher n-alkane productivity in the ravine habitat. Although many phyla have been identified as carrying the alkB gene, our data revealed that the only microbial communities carrying the alkB gene in our study were Proteobacteria and Actinobacteria.
3.6 Prediction of bacteria carrying alkB genes in Nanjenshan Reserve
The relative abundance of bacteria containing the alkB gene in the Actinobacteria and Proteobacteria phyla from different habitats is illustrated in Fig. 4. The phylogenetic trees of OTUs in the Proteobacteria and Actinobacteria phyla with alkB reference strains are shown in Figs. S3 and S4. The nucleotide sequence similarities of OTUs and the adjacent alkB reference strains were manually blasted. Table S1 in the Supplement reveals the nomenclature of OTUs in Proteobacteria and Actinobacteria with nucleotide sequences that had similarity of higher than 95 % when compared with adjacent alkB reference strains. Figure 4 illustrates that a significant number of reads in the OTUs whose sequences were similar to those of alkB-gene-containing bacteria was identified for the ravine habitat. Although the relative abundance of Proteobacteria in the ravine habitat was 20 % less than that in the other habitats (Fig. 3), the number of DNA sequence reads of alkB-lineage-like bacteria in the ravine habitat was greater than 1.5 times those in the windward and leeward areas (Fig. 4). To summarize, there were twice as many DNA sequence reads of alkB-lineage-like bacteria in the ravine habitat than in the other habitats. Despite the relative abundance of DNA sequence reads in many OTUs of the alkB-lineage-like bacteria, Proteobacteria, and Actinobacteria phyla were limited in the leeward and windward habitats (Fig. 4); these two habitats had small amounts of alkB-lineage-like bacteria (approximately 0.1 % relative abundance) in most OTUs (Table S1). Our data provide evidence that there is a universal presence of alkane degraders in natural habitats.
3.7 PCoA of bacteria carrying alkB genes
PCoA was used to visualize the similarities of DNA sequences in Proteobacteria and Actinobacteria from the three habitats. The biodiversity index in OTUs of alkB-lineage-like bacteria and other sample types is shown in Table S2. Figure 5 displays the distribution of 240 OTUs in the Proteobacteria and Actinobacteria phyla. The majority of OTUs (> 90 %) are present in two or three habitats. An initial capital letter in Fig. 5 was used to denote the OTUs with the most read numbers for the habitats. The nomenclature of the alkB-lineage-like bacteria OTUs and relative locations are presented in the figure. Thirty-three OTUs contained alkB-lineage-like bacteria in this study. The numbers of OTUs that contained alkB-lineage-like bacteria in the ravine, windward, and leeward habitats were 30, 30, and 25, respectively. The Shannon–Wiener index of OTUs that contained alkB-lineage-like bacteria in the ravine, windward, and leeward habitats was 2.51, 2.39, and 2.46, respectively. Although the relative abundance of OTUs that contained alkB-lineage-like bacteria in the ravine habitat was more than twice that in the windward and leeward habitats (Fig. 4), the Shannon–Wiener indices of the three habitats were similar, suggesting that the effective species numbers of alkB-lineage-like bacteria were almost identical. The results indicate the pre-existence of diverse alkB-gene-containing bacteria in natural habitats, with some bacteria so small in number that they are undetectable using normal PCR. They could, however, proliferate in the habitats with the appropriate substrate supplements. This finding is consistent with the results from a previous study using a laboratory-controlled system and an agricultural research farm (Schulz et al., 2012; Giebler et al., 2013).
3.8 Empirical testing of bacteria carrying alkB gene
In the bacterial community amplicon sequencing study, equal amounts of DNA were amplified to estimate the bacterial communities in each habitat. To compare the productivity of the alkB-gene-containing bacteria in different habitats, we conducted semi-quantitative PCR to determine relative alkB gene concentrations. Figure 6a shows a triplicate study of DNA staining of amplicons from the three habitats after various semi-quantitative PCR cycles. No bands were detected if fewer than 24 amplification cycles were performed. Marginal PCR products could be detected by increasing the cycle number to 27. Figure 6b shows the statistical results regarding alkB gene levels in the three Nanjenshan habitats and confirms that bacterial alkB numbers were higher in the ravine habitat than in the other two habitats. We have thus provided evidence that litterfall and plant vegetation increase the abundance of alkane-degrading bacteria communities in natural habitats.
3.9 Exploring the relationship between plant vegetation and microbial communities using heat map analysis
Heat map analysis of plant species and read number of OTU versus habitat were performed to investigate the relationship between plant vegetation patterns and microbial communities in different habitats. The heat map presented in Fig. 7 was based on the microbial community abundance and plant productivity of litter-leaf from different habitats, with the color intensity indicating the number of reads or weight of litter-leaf (in logarithmic form). In Figure 7b, the color patterns of litter-leaf yields show that microbial abundance was similar in the windward and leeward habitats, demonstrating the similarity between the microbial community compositions in these two habitats. Furthermore, the litterfall data were also similar between the two habitats, which suggests a connection between plant vegetation species and microbial composition. The pattern similarities in the plant and bacterial species can be easily explained by substrate-specific-induced growth responses in the natural habitats. The data provided supporting evidence that diversity of bacteria in all OTUs was affected by plant vegetation, not only in quantity but also in species. In Figs. 2, 4, and 6, an increase in n-alkane productivity is demonstrated to increase the number of alkB-gene-containing bacteria. In Fig. 7, we showed that a substrate-specific relationship may exist between plant vegetation and microbial communities. Thus, a leaf in a natural habitat may upregulate some alkB-gene-containing bacteria while downregulating other types of alkB-gene-containing bacteria. A future study could include a litter bag study that would enable better understanding of the relationship between individual factors within plant and bacterial species; for instance, one could study the interaction of a specific leaf species with a particular genus of n-alkane-degrading bacteria or microbial community in different habitats.
We confirmed that the annual litterfall productivity of the ravine habitat was approximately 30 % higher than that of the windward and leeward habitats, whereas the long-chain n-alkane productivity in the ravine habitat was twice that in the other habitats in a lowland subtropical rainforest of Taiwan. Plant vegetation not only affects n-alkane productivity; it also changes litter layer microbial communities. In the ravine habitat, high litter-leaf n-alkane productivity resulted in an increase in the n-alkane-degrading bacteria in the litterfall. Increases in alkB-gene-containing bacteria were identified primarily in the Proteobacteria and Actinobacteria phyla, with NGS-based analysis and semi-quantitative PCR employed for determining alkB gene concentrations. Biodiversity analyses showed that the diversity of bacteria containing the alkB gene was similar among the different habitats, suggesting that natural habitats harbor diverse alkB-lineage-like bacteria with some species existing in very low copy numbers. Practical testing of alkB gene concentration by semi-quantitative PCR with primer coverage over Proteobacteria and Actinobacteria shows results consistent with the bioinformatics data, suggesting this method is helpful for future study on alkane bacteria activity assays from the environmental samples. Cross analyses regarding the quantity and quality of plant vegetation and structures of the microbial communities revealed that n-alkane-induced increases in alkB-gene-containing bacteria might have been substrate specific. To our knowledge, this is the first report on the regulation of n-alkane-degrading bacteria in litterfall in natural habitats.
Data presented in this study are available at https://doi.org/10.6084/m9.figshare.6026462 (Fan, 2018) and https://doi.org/10.6084/m9.figshare.6026159 (Huang, 2018).
The supplement related to this article is available online at: https://doi.org/10.5194/bg-15-1815-2018-supplement.
The authors declare that they have no conflict of interest.
The authors would like to express their gratitude to the anonymous reviewers
for their kind and useful criticism of the manuscript. The authors are
grateful to Chin Li, Cheng-Yu Wu, and Chun-Ping Huang for their
technical support. This work is sponsored by the Ministry of Science and
Technology of Taiwan (MOST 105-2116-M-194-013 and MOST 105-2116-M-194-014).
We would like to thank National Core Facility for Biopharmaceuticals (NCFB,
MOST 106-2319-B-492-002) and National Center for High-performance Computing (NCHC) of National Applied Research Laboratories (NARLabs) of Taiwan for
providing computational resources and storage resources. We also acknowledge
the technical services (support) provided by High-throughput Genome and Big
Data Analysis Core Facility of the National Yang-Ming University VGH Genome
Research Center (VYMGC). High-throughput Genome and Big Data Analysis Core
Facility is supported by National Core Facility Program for Biotechnology
(NCFPB), Ministry of Science and Technology.
Edited by: Denise Akob
Reviewed by: Boris Jansen and two anonymous referees
Afzal, M., Yousaf, S., Reichenauer, T. G., and Sessitsch, A.: Ecology of Alkane-Degrading Bacteria and Their Interaction with the Plant, Molecular Microbial Ecology of the Rhizosphere, 92, 975–989, https://doi.org/10.1002/9781118297674.ch92, 2013.
Beilen, J. B. V., Li, Z., Duetz, W. A., Smits, T. H. M., and Witholt, B.: Diversity of Alkane Hydroxylase Systems in the Environment, Oil Gas Sci. Technol., 58 427–440, 2003.
Chao, K.-J., Chao, W.-C., Chen, K.-M., and Hsieh, C.-F.: Vegetation Dynamics of a Lowland Rainforest at the Northern Border of the Paleotropics at Nanjenshan, Southern Taiwan, Taiwan Journal of Forest Science, 25, 29–40, 2010.
Chao, W.-C., Chao, K.-J., Song, G.-Z. M., and Hsieh, C.-F.: Species Composition and Structure of the Lowland Subtropical Rainforest at Lanjenchi, Southern Taiwan, Taiwania, 52, 253–269, 2007.
Chao, W.-C., Wu, S.-H., Fan, S.-W., Lin, H.-Y., Hsieh, C.-F., and Chao, K.-J.: Distribution Patterns of Tree Species in a Lowland Rainforest at Nanjen Lake, Southern Taiwan, Taiwania, 53, 124–133, 2008.
Chen, Y.-F. and Huang, T.-C.: Vegetation analysis in Nanjenshan area, Ann. Taiwan Mus., 29, 189–258, 1986.
Chin, C.-C.: Study on the Spatial and Temporal Change of Litterfall in Nanjenshan Lowland Rain Forest, master thesis, National Pingtung University of Science and Technology, Taiwan, 2008.
Conklin-Brittain, N. L., Knott, C. D., and Wrangham, R. W.: Feeding Ecology in Apes and Other Primates, Ecological, Physical and Behavioral Aspects, Energy intake by wild chimpanzees and orangutans: methodological considerations and a preliminary companson, 17, edited by: Hohmann, G., Robbins, M. M., and Boesch, C., Cambridge University Press, Cambridge, UK, 445–465, 2006.
Degnan, P. H. and Ochman, H.: Illumina-based analysis of microbial community diversity, ISME J., 6, 183–194, https://doi.org/10.1038/ismej.2011.74, 2012.
Fan, C.-W.: Litterfall and n-alkane, available at: https://doi.org/10.6084/m9.figshare.6026462, last access: 27 March 2018.
Fan, S.-W., Chao, W.-C., and Hsieh, C.-F.: Woody Floristic Composition, Size Class Distribution and Spatial Pattern of a Subtropical Lowland Rainforest at Nanjen Lake, Southernmost Taiwan, Taiwania, 50, 307–326, https://doi.org/10.6165/tai.2005.50(4).307, 2005.
Feakins, S. J., Peters, T., Wu, M. S., Shenkin, A., Salinas, N., Girardin, C. A. J., Bentley, L. P., Blonder, B., Enquist, B. J., Martin, R. E., Asner, G. P., and Malhi, Y.: Production of leaf wax n-alkanes across a tropical forest elevation transect, Org. Geochem., 100, 89–100, https://doi.org/10.1016/j.orggeochem.2016.07.004, 2016.
Giebler, J., Wick, L. Y., Chatzinotas, A., and Harms, H.: Alkane-degrading bacteria at the soil-litter interface: comparing isolates with T-RFLP-based community profiles, FEMS Microbiol. Ecol., 86, 45–58, https://doi.org/10.1111/1574-6941.12097, 2013.
Gomez-Coca, R. B., Perez-Camino Mdel, C., and Moreda, W.: Saturated hydrocarbon content in olive fruits and crude olive pomace oils, Food Addit. Contam. A, 33, 391–402, https://doi.org/10.1080/19440049.2015.1133934, 2016.
Guo, J. H.: The Estimation of Total Soil Organic Carbon Storage under three Habitat Types in Nanjenshan Lowland Rain Forest, master thesis, National Pingtung University of Science and Technology, Taiwan, available at: http://hdl.handle.net/11296/279pcy, 2010.
Hoffmann, B., Kahmen, A., Cernusak, L. A., Arndt, S. K., and Sachse, D.: Abundance and distribution of leaf wax n-alkanes in leaves of Acacia and Eucalyptus trees along a strong humidity gradient in northern Australia, Org. Geochem., 62, 62–67, https://doi.org/10.1016/j.orggeochem.2013.07.003, 2013.
Hsieh, C.-F. Sun, I.-F., and Yang, C.-C.: Species Composition and Vegetation Pattern of a Lowland Rain Forest at the Nanjenshan L TER Site, Southern Taiwan, Taiwania, 45, 107–119, https://doi.org/10.6165/tai.2000.45(1).107, 2000.
Huang, T.-Y.: Metagenomic fastq files from different habitats of subtropical rainforest, available at: https://doi.org/10.6084/m9.figshare.6026159, last access: 27 March 2018.
Jetter, R. and Schäffer, S. S.: Chemical Composition of the Prunus laurocerasus Leaf Surface. Dynamic Changes of the Epicuticular Wax Film during Leaf Development, Plant Physiol., 126, 1725–1737, https://doi.org/10.1104/pp.126.4.1725, 2001.
Jurelevicius, D., Alvarez, V. M., Peixoto, R., Rosado, A. S., and Seldin, L.: The Use of a Combination of alkB Primers to Better Characterize the Distribution of Alkane-Degrading Bacteria, PLoS One, 8, e66565, https://doi.org/10.1371/journal.pone.0066565, 2013.
Kahmen, A., Dawson, T. E., Vieth, A., and Sachse, D.: Leaf wax n-alkane deltaD values are determined early in the ontogeny of Populus trichocarpa leaves when grown under controlled environmental conditions, Plant Cell Environ., 34, 1639–1651, https://doi.org/10.1111/j.1365-3040.2011.02360.x, 2011.
Kirkels, F. M. S. A., Cammeraat, L. H., and Kuhn, N. J.: The fate of soil organic carbon upon erosion, transport and deposition in agricultural landscapes – A review of different concepts, Geomorphology, 226, 94–105, https://doi.org/10.1016/j.geomorph.2014.07.023, 2014.
Kloos, K., Munch, J. C., and Schloter, M.: A new method for the detection of alkane-monooxygenase homologous genes (alkB) in soils based on PCR-hybridization, J. Microbiol. Meth., 66, 486–496, https://doi.org/10.1016/j.mimet.2006.01.014, 2006.
Koch, K., Bhushan, B., and Barthlott, W.: Multifunctional surface structures of plants: An inspiration for biomimetics, Prog. Mater. Sci., 54, 137–178, https://doi.org/10.1016/j.pmatsci.2008.07.003, 2009.
Kostka, J. E., Prakash, O., Overholt, W. A., Green, S. J., Freyer, G., Canion, A., Delgardio, J., Norton, N., Hazen, T. C., and Huettel, M.: Hydrocarbon-degrading bacteria and the bacterial community response in gulf of Mexico beach sands impacted by the deepwater horizon oil spill, Appl. Environ. Microb., 77, 7962–7974, https://doi.org/10.1128/AEM.05402-11, 2011.
Nie, Y., Chi, C. Q., Fang, H., Liang, J. L., Lu, S. L., Lai, G. L., Tang, Y. Q., and Wu, X. L.: Diverse alkane hydroxylase genes in microorganisms and environments, Sci. Rep., 4, 4968, https://doi.org/10.1038/srep04968, 2014.
Quinton, J. N., Catt, J. A., Wood, G. A., and Steer, J.: Soil carbon losses by water erosion: Experimentation and modeling at field and national scales in the UK, Agriculture, Ecosyst. Environ., 112, 87–102, https://doi.org/10.1016/j.agee.2005.07.005, 2006.
Rasheed, M. A., Kalpana, M. S., Veena Prasanna, M., Lakshmi, M., Madhavi, T., Devleena Mani, T., Patil, D. J., Dayal, A. M., and Raju, S. V.: Geo-microbial and light gaseous hydrocarbon anomalies in the near surface soils of Deccan Syneclise Basin, India: Implications to hydrocarbon resource potential, J. Petrol. Sci. Eng., 84–85, 33–41, https://doi.org/10.1016/j.petrol.2012.01.010, 2012.
Rojo, F.: Degradation of alkanes by bacteria, Environ. Microbiol., 11, 2477–2490, https://doi.org/10.1111/j.1462-2920.2009.01948.x, 2009.
Rojo, F.: Enzymes for Aerobic Degradation of Alkanes, Handbook of Hydrocarbon and Lipid Microbiology, 2, 781–797, https://doi.org/10.1007/978-3-540-77587-4_59, 2010.
Sachse, D., Billault, I., Bowen, G. J., Chikaraishi, Y., Dawson, T. E., Feakins, S. J., Freeman, K. H., Magill, C. R., McInerney, F. A., van der Meer, M. T. J., Polissar, P., Robins, R. J., Sachs, J. P., Schmidt, H.-L., Sessions, A. L., White, J. W. C., West, J. B., and Kahmen, A.: Molecular Paleohydrology: Interpreting the Hydrogen-Isotopic Composition of Lipid Biomarkers from Photosynthesizing Organisms, Annu. Rev. Earth Pl. Sc., 40, 221–249, https://doi.org/10.1146/annurev-earth-042711-105535, 2012.
Schmidt, S. K.: A substrate-induced growth-response method for estimating the biomass of microbial functional groups in soil and aquatic systems, FEMS Microbiol. Ecol., 101, 197–206, https://doi.org/10.1111/j.1574-6941.1992.tb01656.x, 1992.
Schulz, S., Giebler, J., Chatzinotas, A., Wick, L. Y., Fetzer, I., Welzl, G., Harms, H., and Schloter, M.: Plant litter and soil type drive abundance, activity and community structure of alkB harbouring microbes in different soil compartments, ISME J., 6, 1763–1774, https://doi.org/10.1038/ismej.2012.17, 2012.
Singh, S. N., Kumari, B., and Mishra, S.: Microbial Degradation of Alkanes, 439–469, https://doi.org/10.1007/978-3-642-23789-8_17, 2012.
Tsui, C.-C., Chen, Z.-S., and Hsieh, C.-F.: Relationships between soil properties and slope position in a lowland rain forest of southern Taiwan, Geoderma, 123, 131–142, https://doi.org/10.1016/j.geoderma.2004.01.031, 2004.
Wentzel, A., Ellingsen, T. E., Kotlar, H. K., Zotchev, S. B., and Throne-Holst, M.: Bacterial metabolism of long-chain n-alkanes, Appl Microbiol. Biot., 76, 1209–1221, https://doi.org/10.1007/s00253-007-1119-1, 2007.
Xu, K., Tang, Y., Ren, C., Zhao, K., and Sun, Y.: Diversity and abundance of n-alkane-degrading bacteria in the near-surface soils of a Chinese onshore oil and gas field, Biogeosciences, 10, 2041–2048, https://doi.org/10.5194/bg-10-2041-2013, 2013.