the Creative Commons Attribution 4.0 License.
the Creative Commons Attribution 4.0 License.
Mn∕Ca intra- and inter-test variability in the benthic foraminifer Ammonia tepida
Jassin Petersen
Christine Barras
Antoine Bézos
Carole La
Lennart J. de Nooijer
Filip J. R. Meysman
Aurélia Mouret
Caroline P. Slomp
Frans J. Jorissen
The adaptation of some benthic foraminiferal species to low-oxygen conditions provides the prospect of using the chemical composition of their tests as proxies for bottom water oxygenation. Manganese may be particularly suitable as such a geochemical proxy because this redox element is soluble in reduced form (Mn2+) and hence can be incorporated into benthic foraminiferal tests under low-oxygen conditions. Therefore, intra- and inter-test differences in foraminiferal Mn∕Ca ratios may hold important information about short-term variability in pore water Mn2+ concentrations and sediment redox conditions. Here, we studied Mn∕Ca intra- and inter-test variability in living individuals of the shallow infaunal foraminifer Ammonia tepida sampled in Lake Grevelingen (the Netherlands) in three different months of 2012. The deeper parts of this lake are characterized by seasonal hypoxia/anoxia with associated shifts in microbial activity and sediment geochemistry, leading to seasonal Mn2+ accumulation in the pore water. Earlier laboratory experiments with similar seawater Mn2+ concentrations as encountered in the pore waters of Lake Grevelingen suggest that intra-test variability due to ontogenetic trends (i.e. size-related effects) and/or other vital effects occurring during calcification in A. tepida (11–25 % relative SD, RSD) is responsible for part of the observed variability in Mn∕Ca. Our present results show that the seasonally highly dynamic environmental conditions in the study area lead to a strongly increased Mn∕Ca intra- and inter-test variability (average of 45 % RSD). Within single specimens, both increasing and decreasing trends in Mn∕Ca ratios with size are observed. Our results suggest that the variability in successive single-chamber Mn∕Ca ratios reflects the temporal variability in pore water Mn2+. Additionally, active or passive migration of the foraminifera in the surface sediment may explain part of the observed Mn∕Ca variability.
- Article
(2233 KB) - Full-text XML
-
Supplement
(373 KB) - BibTeX
- EndNote
In many coastal ecosystems, high summer temperatures and eutrophication lead to seasonally occurring hypoxia ([O2]<63 µM; Rabalais et al., 2002; Diaz and Rosenberg, 2008), linked to the emergence of water column stratification in combination with lower oxygen solubility and higher respiration rates in warmer waters (e.g. Keeling et al., 2010). On the seafloor, oxygen is consumed by respiration of marine biota, thereby coupling bottom water oxygenation (BWO), benthic ecosystem functioning and organic carbon cycling (Altabet et al., 1995; Levin et al., 2009; Koho et al., 2013).
Most of the organic matter (OM) deposited on the seafloor is mineralized in surface sediments by respiration processes, involving oxygen, nitrate, manganese, and iron oxides or sulfate as electron acceptors (Froelich et al., 1979). If Fe and Mn (hydr)oxides are sufficiently abundant, their reduction can be relevant for the total OM decomposition in the sediment (Aller, 1990; Burdige, 1993; Canfield, 1993; Vandieken et al., 2006). When the bottom waters of coastal water bodies are oxygenated, Mn oxides are present in the oxic surface layer of the sediment and are reduced to soluble Mn2+ in the deeper anoxic sediment layers. Dissolved Mn2+ can then diffuse upwards in the pore water across the oxic–anoxic boundary, where it precipitates again in the form of Mn oxides, leading to a continuous cycling of Mn within the upper sediment (Aller, 1994; Slomp et al., 1997). The sedimentation rate and the bioturbation intensity are important factors controlling the cycling of various reactive compounds in sediments, such as metal oxides (van de Velde and Meysman, 2016). Macrofaunal bioturbation may introduce Mn oxides in the deeper, anoxic sediment, where these minerals are subsequently reduced (Mouret et al., 2009; Thibault de Chanvalon et al., 2016). When eutrophication and stratification of the water column lead to (seasonally) hypoxic bottom water conditions, the oxygen penetration depth is reduced, causing an upward movement of the Mn redox front and diminishing the possibility that pore water Mn2+ is oxidized. In extreme cases, Mn2+ may diffuse from the pore water into the water column (Sundby and Silverberg, 1985; Thamdrup et al., 1994; Dellwig et al., 2007; Konovalov et al., 2007; Pakhomova et al., 2007; Kowalski et al., 2012).
Sedimentary records of manganese may reflect a variety of past environmental conditions, including bottom water redox state, continental runoff, surface water productivity and bottom water current dynamics (Reichart et al., 1997; van der Weijden et al., 2006; Lenz et al., 2015). The use of sedimentary manganese as a proxy is complicated because of its complex biogeochemical dynamics, including the remobilization of once precipitated manganese oxides, and subsequent diagenetic overprinting (Schenau et al., 2002). Mn incorporated into the calcite of benthic foraminifera potentially represents a more reliable proxy of redox conditions, since these marine protists build their shells (tests) in the upper sediment layer, where the presence of Mn2+ may be a function of bottom water oxygenation, and Mn2+ can be permanently incorporated into the tests. However, the ecology of the various foraminiferal species is crucial and can influence manganese incorporation into the shell, since different adaptation strategies to changes in the availability of OM and of oxygen lead to different microhabitats and probably also to different calcification depths and periods (Jorissen et al., 1995; Van der Zwaan et al., 1999; Koho et al., 2015). So far, few studies have investigated the potential of using Mn∕Ca ratios in benthic foraminifera as a proxy for bottom water redox state. These studies show that benthic foraminifera register environmental Mn2+ concentrations in their tests (Reichart et al., 2003; Munsel et al., 2010; Glock et al., 2012; Groeneveld and Filipsson, 2013; Koho et al., 2015, 2017; McKay et al., 2015; Barras et al., 2018). For example, Koho et al. (2015, 2017) demonstrated that foraminiferal species occupying a relatively deep microhabitat display higher Mn∕Ca ratios than those living near the sediment surface. Furthermore, by using microanalytical techniques, capable of measuring elemental concentrations in single foraminiferal chambers, short-term variability in oxygenation may be unravelled. Recent studies have shown the potential of intra-test variability in Mn∕Ca to resolve vertical migration in the sediment and/or seasonal changes in oxygenation (Glock et al., 2012; McKay et al., 2015).
In order to apply Mn∕Ca ratios in benthic foraminiferal tests as a quantifiable proxy of palaeo-redox conditions, it is necessary to assess the presence of ontogenetic trends (i.e. size-related effects) and/or other vital effects occurring during calcification; the variability due to such biological processes will hereafter be termed “intrinsic (intra-test) variability”. This intrinsic part of the total variability (which can be assessed only in culturing studies) determines the threshold value above which the variability within a single specimen can be recognized successfully as a response to environmental conditions. In the case of the total intra-test variability being greater than the intrinsic variability, the added part can be ascribed to environmental factors and/or active or passive migration of the foraminifera in the sediment surface layer. In such cases, single-chamber measurements could provide information about the temporal variability in the Mn dynamics. A first culturing study of Ammonia tepida with different concentrations of seawater Mn2+ (in the range of naturally occurring concentrations in seawater, which is up to 3 orders of magnitude lower than concentrations found in pore waters of our coastal study area) was performed by Munsel et al. (2010). A more recent culturing study of Ammonia tepida and Bulimina marginata evidences a species-specific linear relationship between Mn∕Ca ratios of the tests and a range of dissolved Mn2+ concentrations commonly found in pore waters of coastal areas ( ± 0.001 Mn∕Caseawater for A. tepida, R2=0.96, p<0.001; Barras et al., 2018). This culturing study found that Mn∕Ca intrinsic variability varied between 11 and 25 % for A. tepida for a range of seawater Mn2+ concentrations similar to those found in the pore water of surface sediments in our study area.
Here, we investigate Mn∕Ca intra- and inter-test variability in the same benthic foraminifer, A. tepida, as studied by Barras et al. (2018; culturing experiments) from field samples of Lake Grevelingen (the Netherlands). The shallow infaunal A. tepida represents a species complex including several pseudo-cryptic species (Hayward et al., 2004; Schweizer et al., 2011; Saad and Wade, 2016). In Lake Grevelingen, A. tepida is almost exclusively represented by the T6 genotype. Ammonia tepida is abundant in coastal areas of temperate climate zones, tolerating diverse biological and environmental stress factors, including low-oxygen conditions (Moodley and Hess, 1992; Sen Gupta et al., 1996; Geslin et al., 2014; Nardelli et al., 2014; Thibault de Chanvalon et al., 2015; Cesbron et al., 2016). The salty bottom waters of Lake Grevelingen, an artificial lake created after the closure of a branch of the Rhine–Meuse–Scheldt estuary, are characterized by seasonal hypoxia ([O2]<63 µM) and anoxia ([O2]< detection limit of 1 µM) (Hagens et al., 2015; Seitaj et al., 2017). Therefore, this site provides a suitable location to study short-term environmental variability (on a timescale of weeks to months) in relation to elemental incorporation into benthic foraminiferal tests. However, one complicating factor is that the activity of cable bacteria strongly influences the seasonal pattern of sediment geochemical cycles in Lake Grevelingen. These electrogenic bacteria induce strong shifts in the sediment geochemistry (Seitaj et al., 2015, 2017; Sulu-Gambari et al., 2016a, b) and lead to substantial Mn2+ mobilization in pore waters (Rao et al., 2016; Sulu-Gambari et al., 2016b; van de Velde et al., 2016). These cable bacteria tend to have a highly patchy distribution, which hence could complicate the interpretation (Sulu-Gambari et al., 2016b; Seitaj et al., 2017).
Our approach is to investigate the Mn∕Ca intra- and inter-test variability in A. tepida by measuring individual chambers with a laser ablation inductively coupled plasma mass spectrometer (LA-ICP-MS) for selected living specimens sampled in three different months of 2012. We will compare the obtained Mn∕Ca intra-test variability with results on strictly intrinsic intra-test variability for the same species, measured for specimens from laboratory experiments by Barras et al. (2018), to find out whether the seasonal variability in BWO and pore water Mn2+ concentrations has left an imprint on benthic foraminiferal Mn∕Ca ratios.
2.1 Study area
Sediment samples were recovered in Lake Grevelingen at a single location (51∘44.956′ N, 03∘53.826′ E; water depth 23.1 m; Fig. S1). The sampling site experiences seasonal hypoxia; for the year 2012, monthly recordings of temperature, salinity and water column oxygen concentrations (Hagens et al., 2015), sedimentary microbial community composition (Seitaj et al., 2015), pore water geochemistry (Sulu-Gambari et al., 2016a, b), and benthic O2 uptake rates (Seitaj et al., 2017) are available. In 2012, BWO started to decrease in April and attained a minimum of about 20 µM (∼8 % saturation) in August (Fig. S2). After homogenization of the water column in September/October, BWO quickly rose to values of ∼200 µM (∼80 % saturation). Pore water Mn2+ showed highest concentrations (up to 310 µM) in winter and early spring, followed by considerably lower concentrations in summer and autumn (Sulu-Gambari et al., 2016b; Fig. S3).
2.2 Samples of living benthic foraminifera
Living specimens of Ammonia tepida were sampled in Lake Grevelingen in March, July and September 2012. In all three months, surface sediments of the sampling site were inhabited by dense populations of A. tepida. Living specimens were recognized by CellTracker Green (CTG; Bernhard et al., 2006). CTG was applied on board R/V Luctor within 1 h of retrieval (Langlet, 2014). Adult specimens (size fraction 150–315 µm) from the sediment depth 0–0.5 cm were selected for LA-ICP-MS measurements. For March, July and September, 10, 16 and 18 specimens were analysed, respectively. Prior to LA-ICP-MS analyses, all specimens were cleaned to remove sediment adherences (Barker et al., 2003) by rinsing them three times with ultra-pure water in 200 µL tubes, followed by one rinse in methanol and three final rinses in ultra-pure water. During each rinse, the samples were gently agitated with a vortex machine.
2.3 LA-ICP-MS operating conditions and instrument calibration
For all specimens several consecutive test chambers were measured individually with a LA-ICP-MS (Fig. 1). The analyses were performed with an ArF excimer laser (193 nm, Analyte G2, Teledyne Photon Machines) coupled to a quadrupole inductively coupled plasma mass spectrometer (ICP-MS; Varian Bruker 820-MS) at the Laboratory of Planetology and Geodynamics, Université de Nantes (France). Ablations were conducted in a HelEx II two-volume cell with He as a carrier gas, a laser energy density of 0.91 J cm−2 and a repetition rate of 4 Hz. To maximize the amount of ablated material, spot sizes were adapted to the chamber size and varied typically between 40 and 85 µm in diameter. The LA-ICP-MS operating conditions are summarized in Table 1 and the isotope masses selected for analyses were 24Mg, 27Al, 43Ca, 55Mn, 57Fe, 66Zn, 88Sr and 137Ba.
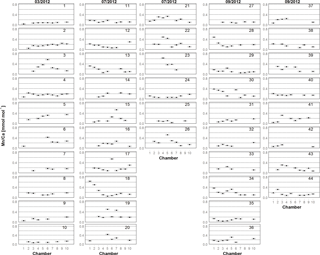
Figure 2Mn∕Ca for each chamber in living specimens of A. tepida from Lake Grevelingen, collected in March (03/2012), July (07/2012) and September (09/2012) 2012 (specimens labelled from 1 to 44 as in Table A1). One plot represents one specimen. Numbers on x axis indicate chambers: 1 – penultimate chamber; 2 – antepenultimate chamber, etc.; 10 – central part of test. Error bars represent the Mn∕Ca SD of multiple analyses of the foraminiferal carbonate standard NFHS (see Sect. 2.3 and 2.4).
Table 2Evaluation of external reproducibility of laser ablation ICP-MS analyses for Mg∕Ca, Mn∕Ca and Sr∕Ca determined on the NFHS, USGS MACS-3 and NIST SRM 610. For Mn∕Ca and Sr∕Ca, all values are calibrated against NIST SRM 612 and are given as average with SDs from all sessions (in bold). For Mg∕Ca, all values are calibrated against USGS MACS-3. Reference values with SD are given according to Jochum et al. (2011), Jochum et al. (2012), USGS (S. Wilson, USGS, unpublished) and Pearce et al. (1997). Values for NFHS are in mmol mol−1 for better comparison with results from samples (SD of standard applied as error bar on samples) as well as in ppm because reference values are given in this unit (information obtained from the NIOZ, 2017) and for comparison with concentrations of other reference materials. Values for USGS MACS-3 and NIST SRM 610 are in ppm for comparison with published reference values.
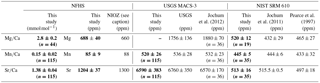
Prior to each analytical session, the ICP-MS was tuned with the NIST SRM 612 reference material to minimize oxide formation ( %) and elemental fractionation (U∕Th close to 1), as well as to optimize the signal-to-noise ratio for Mn. The typical laser ablation profile of a foraminiferal chamber includes 30 s of data acquisition of the background signal (laser turned off) followed by the ablation of the chamber wall until it was completely pierced (the laser was shut down after a visual control of the ablation), and data acquisition was stopped after another measuring interval of the background signal. The NIST SRM 612 glass reference material was analysed every 10 measurements and the NIST SRM 610 (silicate glass reference material), USGS MACS-3 (carbonate reference material) and the NFHS (NIOZ, Netherlands Institute of Sea Research, foraminifera in-house standard; Mezger et al., 2016) were analysed every 20 analytical spots. All reference materials have been analysed in raster mode, with the same laser energy as for the samples, a spot size of 65 µm and a scan speed of 10 µm s−1. The choice of raster mode for reference materials allows us to minimize elemental fractionation linked to crater depth (Eggins et al., 1998). For the ablation of chambers of A. tepida such fractionation is probably negligible because data acquisition was in general short (∼10 s for spot analyses). All foraminiferal ablation profiles were normalized to 43Ca as an internal standard, and element concentrations were calculated assuming 40 % wt for the CaCO3. The NIST SRM 612 glass served as calibration standard for Mn∕Ca and Sr∕Ca of the foraminiferal samples, using the recommended values of Jochum et al. (2011). The results obtained from NIST SRM 610, USGS MACS-3 and NFHS were also normalized to the NIST SRM 612 glass to evaluate the long-term reproducibility of our analyses (Table 2). For each element and analytical session we have calculated the limit of detection (LOD = background signal ; σ=SD calculated on the background signal) and the limit of quantification (LOQ = background signal ) and have discarded all data below the LOQ (Long and Winefordner, 1983; Longerich et al., 1996; Bettencourt da Silva and Williams, 2015). As a result of the low-energy laser ablation conditions, 24Mg profiles of NIST SRM 612 were below the LOQ for some datasets. However, the USGS MACS-3 has high Mg concentrations (1880±70 ppm), resulting in signals above the LOQ, and thus Mg∕Ca ratios of foraminiferal samples, NFHS and NIST SRM 610 were normalized to USGS MACS-3 using the recommended values of Jochum et al. (2012).
2.4 Data treatment
All laser ablation profiles for each element and each sample or reference material were carefully examined and processed with the GLITTER software. Firstly, the integration interval was based on constant raw counts of 44Ca as an indicator of calcite ablation and on constant Sr∕Ca ratios, as the intra-shell heterogeneity of Sr∕Ca in foraminifera in general and of A. tepida in particular is known to be relatively small (e.g. Eggins et al., 2003; de Nooijer et al., 2014a). Secondly, each laser ablation profile was screened for peaks in elements that may indicate surface contamination (27Al, 57Fe, 66Zn). Typically, high concentrations of Mn on the outer and inner shell surfaces are considered an indicator of contamination (Marr et al., 2011; de Nooijer et al., 2014a; Leduc et al., 2014; Koho et al., 2015). In our case, where Mn is the element of interest, whenever peaks of Mn on outer and inner parts of profiles corresponded to peaks of other contaminant elements, they were discarded from our data. All integration intervals containing less than 10 data points or results with count rates below the LOQ were removed from our dataset.
The external reproducibility of our Mn∕Ca analyses was 2 % (calculated as 2 × SE, relative standard error) when determined on the NFHS foraminiferal carbonate standard (data for Mn∕Ca, Mg∕Ca and Sr∕Ca in Table 2). This standard is adequate for this purpose since it has Mn concentrations comparable to our samples and the same matrix. The results for the USGS MACS-3 carbonate and NIST SRM 610 glass reference materials (Table 2) agree with the recommended values (published values have RSDs of 4 and 1 %, respectively; Table 2). Additionally, we analysed the GJR in-house calcite standard four times, and the calculated concentrations for Mn and Sr (107±1 ppm and 190±3 ppm, respectively) compared well with published values (106 ± 7 ppm and 184±15 ppm, respectively) from Wit et al. (2010).
2.5 Statistical analyses
Statistical analyses were carried out using R (R Core Team, 2016) and the package ggplot2 for graphical representation (Wickham, 2009). To verify whether data were normally distributed we used the Shapiro–Wilk test. For normally distributed data we used an analysis of variance (ANOVA) and t tests with the Bonferroni adjustment as a post hoc test. In all other cases we used a Kruskal–Wallis test and a Wilcoxon–Mann–Whitney test as a post hoc test. When comparing Mn∕Ca ratios between chambers, in order to check for ontogenetic trends, we used Spearman rank correlation. In all cases, a p value below 0.05 was considered significant.
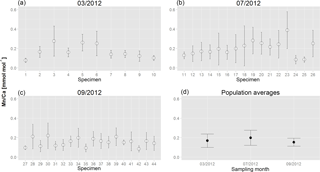
Figure 3Average Mn∕Ca values and SD for all measured chambers of each analysed specimen of A. tepida for the three sampling campaigns (a: March; b: July; c: September). (d) Population averages with SD calculated on the basis of mean values per specimen of all analysed specimens for each of the three sampling months.
3.1 Average Mn∕Ca ratios per specimen
For all specimens of A. tepida investigated, between four and nine chambers per specimen fulfilled our profile selection criteria for reliable Mn∕Ca measurements (as defined in Sect. 2.4). Mn∕Ca ratios of individual chambers ranged from 0.03 to 0.57 mmol mol−1 for the 10 specimens sampled in March 2012 (Fig. 2 (03/2012); Table A1), from 0.04 to 0.63 mmol mol−1 for the 16 specimens collected in July 2012 (Fig. 2 (07/2012); Table A1) and from 0.04 to 0.48 mmol mol−1 for the 18 specimens sampled in September 2012 (Fig. 2 (09/2012); Table A1). Average values for Mn∕Ca per specimen were between 0.08±0.02 and 0.28±0.15 mmol mol−1 in March, between 0.08±0.04 and 0.39±0.19 mmol mol−1 in July, and between 0.09±0.03 and 0.22±0.13 mmol mol−1 in September (Fig. 3a–c; Table A1). There are no significant differences in average Mn∕Ca ratios per specimen between the three sampled months (Fig. 3d; ANOVA, Table 3). However, for the SDs per specimen, the values from July 2012 are significantly larger than those of the two other months (ANOVA, Table 3).
3.2 Intra-test variability
The intra-test variability defined as RSD per specimen is on average 45 % (±15 % SD for all specimens) for Mn∕Ca, 49 % (±24 %) for Mg∕Ca and 9 % (±4 %) for Sr∕Ca. (Tables A1–A3). The specimens with the highest Mn∕Ca RSD (three specimens with RSD >70 %) were sampled in July (specimens 15, 17 and 18; Fig. 2). Out of these three specimens, two had one chamber with much higher Mn∕Ca compared to the other chambers (specimens 15 and 17), whereas one specimen had an increase in Mn∕Ca ratios from older to younger chambers (specimen 18).
To further investigate the variability in Mn∕Ca ratios within single specimens, we calculated the range (maximal minus minimal chamber value) for each measured individual. The so-defined Mn∕Ca intra-test variability shows a different pattern for all specimens from each of the three sampling campaigns (Fig. 4; data in Table A1). For instance, in March, 7 out of 10 specimens had a range smaller than 0.2 mmol mol−1, whereas the remaining 3 had a maximum Mn∕Ca intra-test variability of up to 0.5 mmol mol−1. Yet, for the specimens sampled in July, 12 out of 16 had a range larger than 0.2 mmol mol−1, with 1 specimen showing a range of 0.5–0.6 mmol mol−1. Most specimens collected in September had a Mn∕Ca range within specimens of 0.1–0.2 mmol mol−1, with no specimen exceeding a difference in Mn∕Ca of 0.4 mmol mol−1. Because the range histogram for March clearly shows a non-normal distribution (p value =0.041) and the sample size per sampling date is small (n between 10 and 18), we investigated the differences with a non-parametric test (Kruskal–Wallis test and a Wilcoxon–Mann–Whitney test as a post hoc test) and obtained a significant difference between July and the other two months. This result confirms the higher Mn∕Ca intra-test variability in July compared to March and September (Table 3).
3.3 Ontogenetic trends of Mn∕Ca ratios
To investigate the presence of ontogenetic (i.e. size-related) trends, we distinguished between four types: (a) specimens with a trend towards lower values in later chambers (n=7; specimens 1, 2, 5, 6, 9, 14 and 31 in Fig. 2); (b) specimens that tended to have higher Mn∕Ca ratios in later chambers (n=6; specimens 18, 23, 28, 30, 34 and 39 in Fig. 2); (c) specimens with only one or two values deviating from generally rather constant Mn∕Ca ratios in all other chambers (n=2; specimens 17 and 19 in Fig. 2); and (d) specimens with no apparent trend (the remaining 29 specimens). Most specimens of the first group were sampled in March 2012; in fact half of the specimens from March showed a tendency towards lower Mn∕Ca ratios in later chambers. Most individuals with increasing values towards later chambers were collected in September 2012. In the sample of July 2012, no dominant trend was observed.
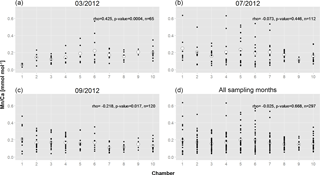
Figure 5Mn∕Ca of all measured specimens as a function of chamber succession. (a) March; (b) July; (c) September; (d) all combined. Numbers on x axis indicate chambers: 1 – penultimate chamber; 2 – antepenultimate chamber, etc.; 10 – central part of test. Small black dots represent Mn∕Ca of single measurements; larger white circles represent average values per chamber, when all specimens are combined. Trends were tested for statistical significance with a Spearman rank correlation (correlation coefficient rho, p value and n (total number of data points) are indicated).
To check for the existence of persistent ontogenetic trends, we combined for each successive chamber the values of all measured specimens and tested for statistically significant trends (Fig. 5). As expected, for March 2012 there is a significant trend towards lower values in later chambers (Fig. 5a). In July 2012 no significant trend is found (Fig. 5b), whereas in September 2012 there was a significant trend of slightly increasing values towards later chambers (Fig. 5c). If all specimens from the three sampled months are combined (Fig. 5d), there is no significant relation with chamber stage.
4.1 Comparison of Mn∕Ca ratios between benthic foraminiferal species from coastal and deep-sea ecosystems
Recently, Mn∕Ca ratios in benthic foraminiferal tests have been proposed as a potential palaeo-proxy for BWO. This suggestion is based on several observations made in recent continental margin and deep-sea ecosystems (Reichart et al., 2003; Glock et al., 2012; Groeneveld and Filipsson, 2013; Koho et al., 2015, 2017). Furthermore, the application of foraminiferal Mn∕Ca ratios as a proxy for dissolved Mn has been tested in laboratory conditions, where calibration studies show a linear relation between seawater dissolved Mn2+ concentrations and foraminiferal Mn∕Ca ratios, though with species-dependent partition coefficients (Munsel et al., 2010; Barras et al., 2018). The use of foraminiferal Mn∕Ca ratios as a palaeo-proxy for BWO has further been explored in several studies of deep-sea sediment records (Klinkhammer et al., 2009; Ní Fhlaithearta et al., 2010; McKay et al., 2015).
Our study is the first to investigate benthic foraminiferal Mn∕Ca ratios in a coastal ecosystem. The results for A. tepida show an average Mn∕Ca ratio of 0.17±0.08 mmol mol−1 and a range of 0.08±0.04 to 0.39±0.19 mmol mol−1 for the average Mn∕Ca per specimen (Table A1). This range is comparable to that found in living specimens of some deep-sea infaunal species from the NE Japan margin (E. batialis, B. spissa, U. cf. graciliformis, U. akitaensis, N. labradorica, C. fimbriata; 0.0020 to 0.277 mmol mol−1; Koho et al., 2017) but is elevated compared to Mn∕Ca ratios measured in single tests of living benthic foraminifera from the Peruvian oxygen minimum zone (OMZ) (B. spissa; 0.0021 to 0.010 mmol mol−1; Glock et al., 2012). This latter difference can be explained by the much higher pore water Mn2+ concentrations in our study (< 310 µmol L−1) and in the study of Koho et al. (2017; < 5 µmol L−1) compared to the study of Glock et al. (2012; < 0.1 µmol L−1). Despite the different pore water Mn2+ concentrations in our study compared to Koho et al. (2017), we found rather similar foraminiferal Mn∕Ca ratios, and this contrast could be partly resolved by higher partition coefficients in deep-sea species compared to coastal species (Barras et al., 2018). For A. tepida, the range measured in specimens from Lake Grevelingen in our study compares well with measured average Mn∕Ca ratios in the study of Barras et al. (2018; 0.13 and 0.86 mmol mol−1) for a similar range of pore water Mn2+ concentrations compared to the concentration of dissolved Mn in the seawater of the culturing experiments (10 to 100 µmol L−1).
4.2 Intra-test variability in elemental ratios in benthic foraminiferal tests
Intra-test variability is less well documented for Mn∕Ca ratios than for other elemental ratios (el ∕ Ca; e.g. Mg∕Ca, Sr∕Ca), and it has not yet been established what portion of the total variability can be attributed to either intrinsic (i.e. intra-test variability due to ontogenetic trends or other vital effects) or environmental factors (e.g. seasonality of Mn cycling in the surface sediments, microhabitat effects). In fact, it is essential to know what degree of intrinsic variability can be expected in a population having experienced the same environmental conditions and above what threshold changes in Mn∕Ca ratios can be ascribed to environmental factors. When using a LA-ICP-MS, or other microanalytical techniques, Mn∕Ca ratios are measured on small parts of the foraminiferal test, making knowledge of intra-test variability even more crucial for the interpretation of the measurements. In the case of a high intrinsic variability, independent of environmental parameters, more spot measurements will be necessary to obtain a reliable mean value for one specimen or several specimens from the same stable environment (Sadekov et al., 2005; de Nooijer et al., 2014a). Although contamination is an important issue in Mn∕Ca measurements (Boyle, 1983; Barker et al., 2003; Pena et al., 2005, 2008), pre-treatment cleaning and a precise targeting of the measurement interval (when using secondary ion mass spectrometry, SIMS, or a LA-ICP-MS), should largely eliminate the potential influence of contaminant phases and/or diagenetic overgrowths (Glock et al., 2012; Koho et al., 2015; McKay et al., 2015). Therefore, the Mn∕Ca intra-test variability should ideally not have a diagenetic contribution.
The results for all specimens measured in this study showed an average Mn∕Ca intra-test variability for A. tepida of 45±15 % (RSD average for all chambers measured on a single specimen; Table A1), which is comparable to that reported in some previous studies (30–50 %; Glock et al., 2012; McKay et al., 2015; Koho et al., 2017). Our results from the different sampling campaigns showed more specimens with higher variability in July compared to March and September (Figs. 3 and 4). In order to investigate if this significant difference can be attributed to environmental factors, our results will be compared to variability reported in other elements.
4.2.1 Approach for estimating (intrinsic) intra-test variability in Mn∕Ca, Mg∕Ca and Sr∕Ca
The average Mn∕Ca intra-test variability observed for A. tepida (45 %) is comparable to that measured for Mg∕Ca (49 %; Table A2) but is larger than for Sr∕Ca (9 %; Table A3). Similarly, in other field studies of benthic foraminifera, average intra-test variability was ∼20–50 % RSD for Mg∕Ca (Allison and Austin, 2003; Curry and Marchitto, 2008; Raitzsch et al., 2011) and 5 % RSD for Sr∕Ca (Allison and Austin, 2003). In these studies it could not be determined how much of the observed variability was due to intrinsic and environmental factors. In contrast to field studies, the use of cultured foraminifera offers the advantage that specimens have grown under exactly the same stable physico-chemical conditions (no environmental variability); thus, intra-test variability in elemental ratios is entirely due to biological processes. These culture studies suggest that when only intrinsic intra-test variability is considered, the RSD is ∼30 % for Mg∕Ca, compared to ∼8 % for Sr∕Ca (values for all chambers analysed with a LA-ICP-MS; de Nooijer et al., 2014a). This difference between intrinsic intra-test variability in Mg∕Ca and Sr∕Ca was explained by the fact that during biomineralization processes, unlike Sr, Mg is strongly discriminated against in the calcifying fluid, and that these processes may vary over time and/or position in the test (Bentov and Erez, 2005, 2006; Nehrke et al., 2013; de Nooijer et al., 2014b). In some field studies (Allison and Austin, 2003; Curry and Marchitto, 2008), values for Mg∕Ca intra-test variability were similar to those observed in the culturing experiment, whereas only a single field study (Raitzsch et al., 2011) reported substantially higher Mg∕Ca intra-test variability, with an RSD of 51 %. In the case of our A. tepida specimens, the intra-test variability in Mg∕Ca was similarly high (49 %). Contrastingly, the Sr∕Ca RSD of A. tepida was 9 %, comparable to variability in Sr∕Ca from culturing studies.
4.2.2 Intrinsic intra-test variability in Mn∕Ca and ontogenetic trends
Similarly to Mg∕Ca ratios, it is possible to estimate the amount of the intrinsic intra-test variability in Mn∕Ca based on results from cultured benthic foraminifera. Culture experiments performed with A. tepida in controlled and stable conditions show 85 % of variability (RSD) for very low Mn2+ concentrations of 2 µmol L−1 (all chambers calcified under laboratory conditions, measured with a LA-ICP-MS, Barras et al., 2018). However, for seawater Mn2+ concentrations of 12 and 101 µmol L−1, comparable to the pore water concentration at our site, the variability (RSD) in Mn∕Ca was 25 and 11 %, respectively (Barras et al., 2018). For even higher seawater Mn2+ concentrations of 595 µmol L−1 the Mn∕Ca RSD was 17 % (Barras et al., 2018). Despite analytical considerations, the large RSD observed for the lowest Mn2+ concentration was partly due to the presence of a clear decrease in Mn∕Ca during the ontogeny. At higher concentrations, no such ontogenetic tendencies were found (Barras et al., 2018). In our material there were no systematic ontogenetic trends either (for all specimens of all investigated months combined; Figs. 2 and 5d), so that the 11–25 % RSD range measured for Mn2+ concentrations of ∼10–100 µM should be representative of the intrinsic intra-test variability in our specimens. This range is much lower than the average total intra-test variability of 45 % found for living A. tepida in our study of Lake Grevelingen.
Consequently, it appears that at most, about half of the total variability in Mn∕Ca can be attributed to intrinsic factors. The remaining part of the variability in Mn∕Ca should be due to changing pore water Mn2+ concentrations in the calcification environment of the foraminifera. This may be due to environmental changes during the lifespan of the individuals or, alternatively, to active or passive vertical foraminiferal migration through different biogeochemical micro-environments.
4.2.3 Seasonality of environmental factors as explanation for Mn∕Ca intra-test variability
As explained in the previous paragraph, it appears that half or more of the measured Mn∕Ca intra-test variability in A. tepida (total intra-test variability was 45 % RSD compared to 11–25 % RSD intrinsic variability) can be attributed to environmental parameters. Here, we will consider which specific factors may be responsible for this variability. First of all, the sampling site of A. tepida in Lake Grevelingen at 23.1 m water depth shows strong seasonal fluctuations in bottom water oxygenation (Sect. 2.1, Fig. S2; Hagens et al., 2015). In theory, under oxic conditions the zone of manganese reduction should lie below the microhabitat of A. tepida, which is situated close to the sediment–water interface (SWI) (Thibault de Chanvalon et al., 2015; Cesbron et al., 2016), and chambers calcified in this condition should show low Mn∕Ca ratios. When bottom water oxygen concentrations decrease (for instance due to enhanced fluxes of organic matter to the seafloor or the stagnation of bottom waters), the zone of manganese reduction will migrate upward; under hypoxic conditions the Mn redox front will be situated closer to the SWI and more Mn2+ should be incorporated into chambers of A. tepida. However, in extreme cases, the upward migration of the Mn redox front can lead to high amounts of Mn2+ diffusing into the water column (Sundby and Silverberg, 1985; Konovalov et al., 2007; Pakhomova et al., 2007), and in the case of seafloor anoxia, Mn2+ may almost entirely seep out of the sediment into the bottom water (Slomp et al., 1997). Thus, foraminiferal Mn∕Ca ratios from chambers calcified under anoxic conditions should be nominal. At our station in Lake Grevelingen, which experiences hypoxic but not anoxic events in summer, these events lead to the dissolution of Mn oxides in surface sediments, and suspended-matter Mn in the water column (sampled ∼500 m away from our station) shows maximum values in July 2012 (Sulu-Gambari et al., 2016b, 2017). Therefore, we would expect higher Mn2+ concentrations below the SWI in summer than in winter, coinciding with higher Mn∕Ca ratios in chambers of A. tepida calcified in summer. However, contrary to these theoretical expectations, observations showed maximum pore water Mn2+ concentrations in the topmost centimetres from February to April 2012, whereas Mn2+ concentrations remained relatively low during the rest of 2012 (Fig. S3, Sulu-Gambari et al., 2016a, b). Recently, similar seasonal Mn2+ pore water patterns at a nearby but slightly deeper station (∼500 m away, depth 34 m) in Lake Grevelingen were explained by the presence of cable bacteria in winter and early spring, which are capable of performing electrogenic sulfur oxidation resulting in the dissolution of FeS (Seitaj et al., 2015; Sulu-Gambari et al., 2016a, b). Consequently, upward-diffusing Fe2+ is oxidized by manganese oxides, which produces an accumulation of Mn2+ in the pore water in winter (Sulu-Gambari et al., 2016a, b). This process would be responsible for a temporal offset of ∼4–6 months between minimum BWO and maximum Mn2+ concentrations. Although cable bacteria were not detected at our sampling station with fluorescence in situ hybridization (FISH), which may be due to their patchy distribution (Sulu-Gambari et al., 2016b; Seitaj et al., 2017), the similarity in pore water data between the two stations strongly suggests that, also at our sampling station, cable bacteria activity is responsible for the observed strong seasonality in pore water Mn2+. Therefore, it seems probable that A. tepida, sampled in March, July and September 2012 were confronted with strongly different pore water Mn2+ concentrations, and may also have experienced important fluctuations in Mn2+ in their micro-environment during their lifespan.
The foraminiferal lifespan generally varies from 3 months to about 2 years (Boltovskoy and Lena, 1969; Murray, 1983). No precise data are available for A. tepida, although on the basis of field evidence, Goldstein and Moodley (1993) and Morvan et al. (2006) concluded that their longevity should be at least 1 year. However, during the lifespan, chamber formation is probably not a continuous process and could be faster in juvenile stages (on the basis of lower metabolic rates in later ontogenetic stages, inferred from increasing δ13C ratios; e.g. Schmiedl et al., 2004; Mackensen, 2008; Schumacher et al., 2010; Raitzsch et al., 2011). Under experimental conditions, growth rates of A. tepida showed a decrease with ontogeny from 1 chamber per day to 1 chamber per week during a 3-week period (de Nooijer et al., 2014a). It has been suggested (e.g. Jorissen, 1988) that at later ontogenetic stages, calcification could be limited to short periods with favourable conditions, such as abundant food supply. In view of the rather scarce information about the timing and duration of calcification, we assume that A. tepida from Lake Grevelingen calcifies during several successive seasons and that the specimens of our three samples (March, July and September 2012) have each recorded a different part of the yearly pore water Mn2+ cycle. The different degrees of intra-test variability between sampled months (Figs. 3 and 4) could be explained by the fact that some specimens mainly calcify during periods with stable sediment Mn2+ concentrations (i.e. a large part of specimens sampled in March and September), whereas others have calcified during periods with rapid changes, such as in winter, when cable bacteria activity and Mn2+ concentrations rapidly increase, or in spring, when these two parameters decrease again (i.e. a large part of specimens sampled in July). This temporal aspect of Mn∕Ca variability in relation to environmental changes can be further studied by considering all penultimate (and antepenultimate, etc.) chambers from the same sampling (Fig. 5a–c). However, the comparison of Mn∕Ca ratios in the successive chambers of different individuals of the same sample shows important differences (Figs. 2 and 5a–c). This suggests that even within the same sample, all individuals have slightly different calcification histories and have not registered exactly the same time window.
4.2.4 Foraminiferal vertical migration as an explanation for Mn∕Ca intra-test variability
Ammonia tepida is a shallow infaunal taxon, preferring a microhabitat close to the SWI, implying that the chemical composition of its test in principle reflects conditions in the superficial sediment layer (Thibault de Chanvalon et al., 2015; Cesbron et al., 2016). Observations made in laboratory experiments show that specimens of this species introduced into deeper anoxic sediments rapidly decrease their activity (Langlet et al., 2013; Maire et al., 2016), and, according to a field study from the Loire estuary, only specimens from the top 2 cm appear to be capable of regaining their preferred niche at the sediment surface (Thibault de Chanvalon et al., 2015). However, Geslin et al. (2014) and Barras et al. (2018) have shown that several foraminiferal species (among which are A. tepida) repeatedly calcify new chambers in hypoxic conditions, whereas Nardelli et al. (2014) showed that even in anoxic conditions, A. tepida is capable of producing at least one new chamber. These data suggest that individuals which have actively (through vertical migration) or passively (being transported by burrowing macrofauna) moved to deeper sediment layers, with higher Mn2+ levels (such as in February and March 2012, when the oxygen penetration depth was ∼0.2–0.3 cm below the SWI; Seitaj et al., 2017) could incidentally calcify a single chamber with a much higher Mn∕Ca ratio. This could be the explanation for the patterns observed, for instance, in specimens 15, 17 and 19 sampled in July 2012 (Fig. 2). This phenomenon could be responsible for a small contribution to increased Mn∕Ca intra-test variability (specimens 15 and 17 have high RSD values; Table A1). In conclusion, we propose that a large part of the overall Mn∕Ca intra-test variability can be explained by a different timing of calcification events with respect to the seasonal cycle of pore water Mn2+ concentrations, whereas extremely high values in single chambers could be due to occasional chamber formation in deeper sediment layers.
4.3 Relevance of benthic foraminiferal Mn∕Ca ratios for coastal environments and implications for palaeoceanographic studies
Concerning the reliability of single-chamber measurements, in view of the large intra-test variability in the very dynamic environment of Lake Grevelingen, it is necessary to measure several chambers to obtain reliable average Mn∕Ca ratios for a single specimen. In this specific context, bulk measurements could be a more practical solution to study the long-term evolution of BWO. However, the large intra-test variability may include very useful information about the seasonal variability in pore water dynamics driven by redox conditions and sedimentation rates as well as microbial activity. Such information can only be obtained by spot measurements of individual chambers. In fact, a comparison of Mn∕Ca ratios of successive individual chambers can potentially inform us about the extent of these seasonal changes and, implicitly, about cable bacteria activity. If the calcification season of the foraminifera could be determined more precisely (for instance by using single specimen stable isotope analyses; e.g. Diz et al., 2009), it would potentially even be possible to reconstruct the annual cycle of pore water dynamics.
Tests of the coastal benthic foraminifer A. tepida, sampled in three different months at 23.1 m depth in the seasonally hypoxic/anoxic Lake Grevelingen show Mn∕Ca ratios with a range of 0.08±0.04 to 0.39±0.19 mmol mol−1 (for average values per specimen), associated with a very large intra-test variability (average RSD =45 %). This high intra-test variability may partly represent intrinsic factors (due to biological processes), although no systematic ontogenetic trends could be identified (for all specimens from the three sampled months combined). However, we ascribed the larger part of the Mn∕Ca intra-test variability to the substantial temporal variability in environmental parameters and to the different timing of the calcification of the analysed specimens. We suggest that the strong seasonal dynamics of pore water Mn induced by seasonal hypoxia and cable bacteria activity, leading to variations in absolute Mn2+ concentrations and/or migration of the redox front in the sediment, is the main factor responsible for this large intra-test variability. Differences in the timing of calcification could explain the different degrees of intra-test variability observed for the three sampled months, whereas differences in individual life history (between the individuals found in a single sample) could even explain inter-test differences observed in each of our three samples. Some individual chambers with exceptionally high Mn∕Ca ratios could be due to active or passive migration to, and calcification in, slightly deeper sediment layers. In conclusion, in environments with strong seasonal changes in redox conditions and microbial activity, Mn∕Ca measurements of successive chambers of individual tests may provide a powerful proxy to reconstruct the seasonal variability in these parameters.
Datasets (including raw data) are given in Tables A1, A2 and A3. Additionally, the raw data can be accessed under https://issues.pangaea.de/browse/PDI-16596.
Table A1Results of Mn∕Ca measurements for different samplings of living specimens of A. tepida from Lake Grevelingen, station ST2. All data are normalized to NIST SRM 612. Values are calculated per specimen (numbers of specimens according to Fig. 2). The total number of chambers included is indicated in column “n”. Calculations were performed using all decimals.
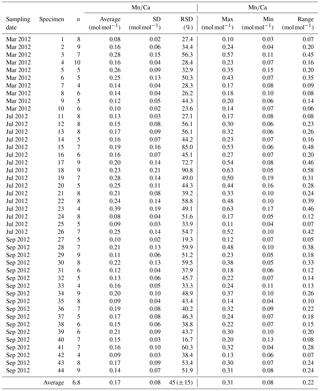
Table A2Results of Mg∕Ca measurements for different samplings of living specimens of A. tepida from Lake Grevelingen, station ST2. All data are normalized to USGS MACS-3. Values are calculated per specimen (numbers of specimens according to Fig. 2). The total number of chambers included is indicated in column “n”. Calculations were performed using all decimals.
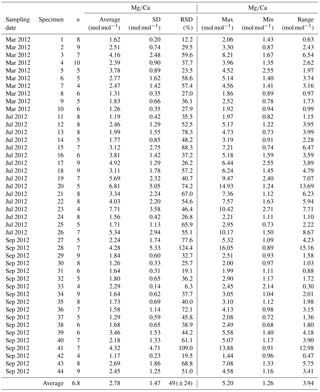
Table A3Results of Sr∕Ca measurements for different samplings of living specimens of A. tepida from Lake Grevelingen, station ST2. All data are normalized to NIST SRM 612. Values are calculated per specimen (numbers of specimens according to Fig. 2). The total number of chambers included is indicated in column “n”. Calculations were performed using all decimals.
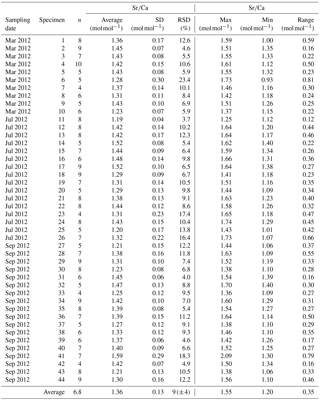
The supplement related to this article is available online at: https://doi.org/10.5194/bg-15-331-2018-supplement.
The authors declare that they have no conflict of interest.
This research was financially supported by the European Research Council
under the European Union's Seventh Framework Programme (FP/2007-2013) through
ERC Grant 306933 to Filip J. R. Meysman. The Region Pays de la Loire is thanked
for financing the MADONA project, including the PhD allocation of
Jassin Petersen. We are grateful to the SCIAM laboratory (University of Angers)
for the foraminiferal SEM image.
Edited by: Markus Kienast
Reviewed by: two anonymous referees
Aller, R. C.: Bioturbation and manganese cycling in hemipelagic sediments, Philos. T. R. Soc. A, 331, 51–68, 1990.
Aller, R. C.: The sedimentary Mn cycle in Long Island Sound: Its role as intermediate oxidant and the influence of bioturbation, O2, and Corg flux on diagenetic reaction balances, J. Mar. Res., 52, 259–295, https://doi.org/10.1357/0022240943077091, 1994.
Allison, N. and Austin, W. E. N.: The potential of ion microprobe analysis in detecting geochemical variations across individual foraminifera tests, Geochem. Geophy. Geosy., 4, 8403, https://doi.org/10.1029/2002GC000430, 2003.
Altabet, M. A., Francois, R., Murray, D. W., and Prell, W. L.: Climate-related variations in denitrification in the Arabian Sea from sediment 15N∕14N ratios, Nature, 373, 506–509, 1995.
Barker, S., Greaves, M., and Elderfield, H.: A study of cleaning procedures used for foraminiferal Mg∕Ca paleothermometry, Geochem. Geophy. Geosy., 4, 8407, https://doi.org/10.1029/2003GC000559, 2003.
Barras, C., Mouret, A., Nardelli, M. P., Metzger, E., Petersen, J., La, C., Filipsson, H. L., and Jorissen, F. J.: Experimental calibration of Mn incorporation in foraminiferal calcite, Geochim. Cosmochim. Ac., in review, 2018.
Bentov, S. and Erez, J.: Novel observations on biomineralization processes in foraminifera and implications for Mg∕Ca ratio in the shells, Geology, 33, 841–844, https://doi.org/10.1130/G21800.1, 2005.
Bentov, S. and Erez, J.: Impact of biomineralization processes on the Mg content of foraminiferal shells: A biological perspective, Geochem. Geophy. Geosy., 7, Q01P08, https://doi.org/10.1029/2005GC001015, 2006.
Bernhard, J. M., Ostermann, D. R., Williams, D. S., and Blanks, J. K.: Comparison of two methods to identify live benthic foraminifera: A test between Rose Bengal and CellTracker Green with implications for stable isotope paleoreconstructions, Paleoceanography, 21, PA4210, https://doi.org/10.1029/2006PA001290, 2006.
Bettencourt da Silva, R. and Williams, A. (Eds.): Eurachem/CITAC Guide: Setting and Using Target Uncertainty in Chemical Measurement, 1st edn., Eurachem and CITAC (Cooperation on International Traceability in Analytical Chemistry), 2015.
Boltovskoy, E. and Lena, H.: Seasonal occurrences, standing crop and production in benthic foraminifera of Puerto Deseado, Contrib. from Cushman Found. Foraminifer. Res., 20, Part 3, 87–95, 1969.
Boyle, E. A.: Manganese carbonate overgrowths on foraminifera tests, Geochim. Cosmochim. Ac., 47, 1815–1819, 1983.
Burdige, D. J.: The biogeochemistry of manganese and iron reduction in marine sediments, Earth-Sci. Rev., 35, 249–284, 1993.
Canfield, D. E.: Organic matter oxidation in marine sediments, in: Interactions of C, N, P and S Biogeochemical Cycles and Global Change, edited by: Wollast, R., Mackenzie, F. T., Chou, L., Springer, Berlin Heidelberg, Germany, 333–363, 1993.
Cesbron, F., Geslin, E., Jorissen, F. J., Delgard, M. L., Charrieau, L., Deflandre, B., Jézéquel, D., Anschutz, P., and Metzger, E.: Vertical distribution and respiration rates of benthic foraminifera: contribution to aerobic remineralization in intertidal mudflats covered by Zostera noltei meadows, Estuar. Coast. Shelf S., 179, 23–38, https://doi.org/10.1016/j.ecss.2015.12.005, 2016.
Curry, W. B. and Marchitto, T. M.: A secondary ionization mass spectrometry calibration of Cibicidoides pachyderma Mg∕Ca with temperature, Geochem. Geophy. Geosy., 9, Q04009, https://doi.org/10.1029/2007GC001620, 2008.
Dellwig, O., Bosselmann, K., Kölsch, S., Hentscher, M., and Hinrichs, J.: Sources and fate of manganese in a tidal basin of the German Wadden Sea, J. Sea Res., 57, 1–18, https://doi.org/10.1016/j.seares.2006.07.006, 2007.
de Nooijer, L. J., Hathorne, E. C., Reichart, G. J., Langer, G., and Bijma, J.: Variability in calcitic Mg∕Ca and Sr∕Ca ratios in clones of the benthic foraminifer Ammonia tepida, Mar. Micropaleontol., 107, 32–43, https://doi.org/10.1016/j.marmicro.2014.02.002, 2014a.
de Nooijer, L. J., Spero, H. J., Erez, J., Bijma, J., and Reichart, G. J.: Biomineralization in perforate foraminifera, Earth-Sci. Rev., 135, 48–58, https://doi.org/10.1016/j.earscirev.2014.03.013, 2014b.
Diaz, R. J. and Rosenberg, R.: Spreading dead zones and consequences for marine ecosystems, Science, 321, 926–929, https://doi.org/10.1126/science.1156401, 2008.
Diz, P., Jorissen, F. J., Reichart, G. J., Poulain, C., Dehairs, F., Leorri, E., and Paulet, Y.-M.: Interpretation of benthic foraminiferal stable isotopes in subtidal estuarine environments, Biogeosciences, 6, 2549–2560, https://doi.org/10.5194/bg-6-2549-2009, 2009.
Eggins, S. M., Kinsley, L. P. J., and Shelley, J. M. G.: Deposition and element fractionation processes during atmospheric pressure laser sampling for analysis by ICP-MS, Appl. Surf. Sci., 127–129, 278–286, https://doi.org/10.1016/S0169-4332(97)00643-0, 1998.
Eggins, S. M., De Deckker, P., and Marshall, J.: Mg∕Ca variation in planktonic foraminifera tests: Implications for reconstructing palaeo-seawater temperature and habitat migration, Earth Planet. Sc. Lett., 212, 291–306, https://doi.org/10.1016/S0012-821X(03)00283-8, 2003.
Froelich, P. N., Klinkhammer, G. P., Bender, M. L., Luedtke, N. A., Heath, G. R., Cullen, D., Dauphin, P., Hammond, D., Hartman, B., and Maynard, V.: Early oxidation of organic-matter in pelagic sediments of the eastern equatorial Atlantic – suboxic diagenesis, Geochim. Cosmochim. Ac., 43, 1075–1090, https://doi.org/10.1016/0016-7037(79)90095-4, 1979.
Geslin, E., Barras, C., Langlet, D., Nardelli, M. P., Kim, J.-H., Bonnin, J., Metzger, E., and Jorissen, F. J.: Survival, reproduction and calcification of three benthic foraminiferal species in response to experimentally induced hypoxia, in: Approaches to Study Living Foraminifera: Collection, Maintenance and Experimentation, edited by: Kitazato, H. and Bernhard, J. M., Springer, Japan, 163–193, 2014.
Glock, N., Eisenhauer, A., Liebetrau, V., Wiedenbeck, M., Hensen, C., and Nehrke, G.: EMP and SIMS studies on Mn∕Ca and Fe ∕ Ca systematics in benthic foraminifera from the Peruvian OMZ: a contribution to the identification of potential redox proxies and the impact of cleaning protocols, Biogeosciences, 9, 341–359, https://doi.org/10.5194/bg-9-341-2012, 2012.
Goldstein, S. T. and Moodley, L.: Gametogenesis and the life cycle of the foraminifer Ammonia beccarii (Linné) forma tepida (Cushman), J. Foramin. Res., 23, 213–220, 1993.
Groeneveld, J. and Filipsson, H. L.: Mg/Ca and Mn∕Ca ratios in benthic foraminifera: the potential to reconstruct past variations in temperature and hypoxia in shelf regions, Biogeosciences, 10, 5125–5138, https://doi.org/10.5194/bg-10-5125-2013, 2013.
Hagens, M., Slomp, C. P., Meysman, F. J. R., Seitaj, D., Harlay, J., Borges, A. V., and Middelburg, J. J.: Biogeochemical processes and buffering capacity concurrently affect acidification in a seasonally hypoxic coastal marine basin, Biogeosciences, 12, 1561–1583, https://doi.org/10.5194/bg-12-1561-2015, 2015.
Hayward, B. W., Holzmann, M., Grenfell, H. R., Pawlowski, J., and Triggs, C. M.: Morphological distinction of molecular types in Ammonia – towards a taxonomic revision of the world's most commonly misidentified foraminifera, Mar. Micropaleontol., 50, 237–271, https://doi.org/10.1016/S0377-8398(03)00074-4, 2004.
Jochum, K. P., Weis, U., Stoll, B., Kuzmin, D., Yang, Q., Raczek, I., Jacob, D. E., Stracke, A., Birbaum, K., Frick, D. A., Günther, D., and Enzweiler, J.: Determination of reference values for NIST SRM 610-617 glasses following ISO guidelines, Geostand. Geoanal. Res., 35, 397–429, https://doi.org/10.1111/j.1751-908X.2011.00120.x, 2011.
Jochum, K. P., Scholz, D., Stoll, B., Weis, U., Wilson, S. A., Yang, Q., Schwalb, A., Börner, N., Jacob, D. E., and Andreae, M. O.: Accurate trace element analysis of speleothems and biogenic calcium carbonates by LA-ICP-MS, Chem. Geol., 318–319, 31–44, https://doi.org/10.1016/j.chemgeo.2012.05.009, 2012.
Jorissen, F. J.: Benthic Foraminifera from the Adriatic Sea; Principles of Phenotypic Variation, Utrecht Micropaleontoligac Bullettin, Utrecht University, vol. 37, 174 pp., 1988.
Jorissen, F. J., de Stigter, H., and Widmark, J. G. V: A conceptual model explaining benthic foraminiferal microhabitats, Mar. Micropaleontol., 26, 3–15, 1995.
Keeling, R. F., Körtzinger, A., and Gruber, N.: Ocean deoxygenation in a warming world, Annu. Rev. Mar. Sci., 2, 199–229, https://doi.org/10.1146/annurev.marine.010908.163855, 2010.
Klinkhammer, G. P., Mix, A. C., and Haley, B. A.: Increased dissolved terrestrial input to the coastal ocean during the last deglaciation, Geochem. Geophy. Geosy., 10, Q03009, https://doi.org/10.1029/2008GC002219, 2009.
Koho, K. A., Nierop, K. G. J., Moodley, L., Middelburg, J. J., Pozzato, L., Soetaert, K., van der Plicht, J., and Reichart, G.-J.: Microbial bioavailability regulates organic matter preservation in marine sediments, Biogeosciences, 10, 1131–1141, https://doi.org/10.5194/bg-10-1131-2013, 2013.
Koho, K. A., de Nooijer, L. J., and Reichart, G. J.: Combining benthic foraminiferal ecology and shell Mn∕Ca to deconvolve past bottom water oxygenation and paleoproductivity, Geochim. Cosmochim. Ac., 165, 294–306, https://doi.org/10.1016/j.gca.2015.06.003, 2015.
Koho, K. A., de Nooijer, L. J., Fontanier, C., Toyofuku, T., Oguri, K., Kitazato, H., and Reichart, G.-J.: Benthic foraminiferal Mn∕Ca ratios reflect microhabitat preferences, Biogeosciences, 14, 3067–3082, https://doi.org/10.5194/bg-14-3067-2017, 2017.
Konovalov, S. K., Luther, G. W., and Yücel, M.: Porewater redox species and processes in the Black Sea sediments, Chem. Geol., 245, 254–274, https://doi.org/10.1016/j.chemgeo.2007.08.010, 2007.
Kowalski, N., Dellwig, O., Beck, M., Grunwald, M., Dürselen, C., Badewien, T. H., Brumsack, H.-J., van Beusekom, J. E. E., and Böttcher, M. E.: A comparative study of manganese dynamics in the water column and sediments of intertidal systems of the North Sea, Estuar. Coast. Shelf S., 100, 3–17, https://doi.org/10.1016/j.ecss.2011.03.011, 2012.
Langlet, D.: Réponse des foraminifères benthiques à l'anoxie dans les milieux côtiers: études in situ de l'écologie des foraminifères benthiques, expériences en laboratoire et analyse du métabolisme anaérobie, PhD Thesis, Université Nantes Angers Le Mans (UNAM), Université d'Angers, France, 2014.
Langlet, D., Geslin, E., Baal, C., Metzger, E., Lejzerowicz, F., Riedel, B., Zuschin, M., Pawlowski, J., Stachowitsch, M., and Jorissen, F. J.: Foraminiferal survival after long-term in situ experimentally induced anoxia, Biogeosciences, 10, 7463–7480, https://doi.org/10.5194/bg-10-7463-2013, 2013.
Leduc, G., Garbe-Schönberg, D., Regenberg, M., Contoux, C., Etourneau, J., and Schneider, R.: The late Pliocene Benguela upwelling status revisited by means of multiple temperature proxies, Geochem. Geophy. Geosy., 15, 475–491, https://doi.org/10.1002/2013GC004940, 2014.
Lenz, C., Jilbert, T., Conley, D. J., and Slomp, C. P.: Hypoxia-driven variations in iron and manganese shuttling in the Baltic Sea over the past 8 kyr, Geochem. Geophy. Geosy., 16, 3754–3766, https://doi.org/10.1002/2015GC005960, 2015.
Levin, L. A., Whitcraft, C. R., Mendoza, G. F., Gonzalez, J. P., and Cowie, G.: Oxygen and organic matter thresholds for benthic faunal activity on the Pakistan margin oxygen minimum zone (700–1100 m), Deep-Sea Res.-Pt. II, 56, 449–471, https://doi.org/10.1016/j.dsr2.2008.05.032, 2009.
Long, G. L. and Winefordner, J. D.: Limit of detection: a closer look at the IUPAC definition, Anal. Chem., 55, 712A–724A, 1983.
Longerich, H. P., Jackson, S. E., and Günther, D.: Inter-laboratory note. Laser ablation inductively coupled plasma mass spectrometric transient signal data acquisition and analyte concentration calculation, J. Anal. Atom. Spectrom., 11, 899–904, https://doi.org/10.1039/JA9961100899, 1996.
Mackensen, A.: On the use of benthic foraminiferal δ13C in palaeoceanography: constraints from primary proxy relationships, Geol. Soc. Spec. Publ., 303, 121–133, https://doi.org/10.1144/SP303.9, 2008.
Maire, O., Barras, C., Gestin, T., Nardelli, M. P., Romero-Ramirez, A., Duchêne, J.-C., and Geslin, E.: How does macrofaunal bioturbation influence the vertical distribution of living benthic foraminifera?, Mar. Ecol.-Prog. Ser., 561, 83–97, 2016.
Marr, J. P., Baker, J. A., Carter, L., Allan, A. S. R., Dunbar, G. B., and Bostock, H. C.: Ecological and temperature controls on Mg∕Ca ratios of Globigerina bulloides from the southwest Pacific Ocean, Paleoceanography, 26, PA2209, https://doi.org/10.1029/2010PA002059, 2011.
McKay, C. L., Groeneveld, J., Filipsson, H. L., Gallego-Torres, D., Whitehouse, M. J., Toyofuku, T., and Romero, O. E.: A comparison of benthic foraminiferal Mn∕Ca and sedimentary Mn/Al as proxies of relative bottom-water oxygenation in the low-latitude NE Atlantic upwelling system, Biogeosciences, 12, 5415–5428, https://doi.org/10.5194/bg-12-5415-2015, 2015.
Mezger, E. M., de Nooijer, L. J., Boer, W., Brummer, G. J. A., and Reichart, G. J.: Salinity controls on Na incorporation in Red Sea planktonic foraminifera, Paleoceanography, 31, 1562–1582, https://doi.org/10.1002/2016PA003052, 2016.
Moodley, L. and Hess, C.: Tolerance of infaunal benthic foraminifera for low and high oxygen concentrations, Biol. Bull., 183, 94–98, https://doi.org/10.2307/1542410, 1992.
Morvan, J., Debenay, J. P., Jorissen, F., Redois, F., Bénéteau, E., Delplancke, M., and Amato, A. S.: Patchiness and life cycle of intertidal foraminifera: Implication for environmental and paleoenvironmental interpretation, Mar. Micropaleontol., 61, 131–154, https://doi.org/10.1016/j.marmicro.2006.05.009, 2006.
Mouret, A., Anschutz, P., Lecroart, P., Chaillou, G., Hyacinthe, C., Deborde, J., Jorissen, F. J., Deflandre, B., Schmidt, S., and Jouanneau, J. M.: Benthic geochemistry of manganese in the Bay of Biscay, and sediment mass accumulation rate, Geo-Mar. Lett., 29, 133–149, https://doi.org/10.1007/s00367-008-0130-6, 2009.
Munsel, D., Kramar, U., Dissard, D., Nehrke, G., Berner, Z., Bijma, J., Reichart, G.-J., and Neumann, T.: Heavy metal incorporation in foraminiferal calcite: results from multi-element enrichment culture experiments with Ammonia tepida, Biogeosciences, 7, 2339–2350, https://doi.org/10.5194/bg-7-2339-2010, 2010.
Murray, J. W.: Population dynamics of benthic foraminifera; results from the Exe Estuary, England, J. Foramin. Res., 13, 1–12, https://doi.org/10.2113/gsjfr.13.1.1, 1983.
Nardelli, M. P., Barras, C., Metzger, E., Mouret, A., Filipsson, H. L., Jorissen, F., and Geslin, E.: Experimental evidence for foraminiferal calcification under anoxia, Biogeosciences, 11, 4029–4038, https://doi.org/10.5194/bg-11-4029-2014, 2014.
Nehrke, G., Keul, N., Langer, G., de Nooijer, L. J., Bijma, J., and Meibom, A.: A new model for biomineralization and trace-element signatures of Foraminifera tests, Biogeosciences, 10, 6759–6767, https://doi.org/10.5194/bg-10-6759-2013, 2013.
Ní Fhlaithearta, S., Reichart, G. J., Jorissen, F. J., Fontanier, C., Rohling, E. J., Thomson, J., and De Lange, G. J.: Reconstructing the seafloor environment during sapropel formation using benthic foraminiferal trace metals, stable isotopes, and sediment composition, Paleoceanography, 25, PA4225, https://doi.org/10.1029/2009PA001869, 2010.
Pakhomova, S. V, Hall, P. O. J., Yu, M., Rozanov, A. G., Tengberg, A., and Vershinin, A. V: Fluxes of iron and manganese across the sediment – water interface under various redox conditions, Mar. Chem., 107, 319–331, https://doi.org/10.1016/j.marchem.2007.06.001, 2007.
Pearce, N. J. G., Perkins, W. T., Westgate, J. A., Gorton, M. P., Jackson, S. E., Neal, C. R., and Chenery, S. P.: A compilation of new and published major and trace element data for NIST SRM 610 and NIST SRM 612 glass reference materials, Geostandard. Newslett., 21, 115–144, https://doi.org/10.1111/j.1751-908X.1997.tb00538.x, 1997.
Pena, L. D., Calvo, E., Cacho, I., Eggins, S., and Pelejero, C.: Identification and removal of Mn-Mg-rich contaminant phases on foraminiferal tests: Implications for Mg∕Ca past temperature reconstructions, Geochem. Geophy. Geosy., 6, Q09P02, https://doi.org/10.1029/2005GC000930, 2005.
Pena, L. D., Cacho, I., Calvo, E., Pelejero, C., Eggins, S., and Sadekov, A.: Characterization of contaminant phases in foraminifera carbonates by electron microprobe mapping, Geochem. Geophy. Geosy., 9, Q07012, https://doi.org/10.1029/2008GC002018, 2008.
Rabalais, N. N., Turner, R. E., and Wiseman, W. J.: Gulf of Mexico Hypoxia, a.k.a. “The Dead Zone,” Annu. Rev. Ecol. Syst., 33, 235–263, https://doi.org/10.1146/annurev.ecolsys.33.010802.150513, 2002.
R Core Team: R: A Language and Environment for Statistical Computing, R Foundation for Statistical Computing, Vienna, Austria, 2016.
Raitzsch, M., Hathorne, E. C., Kuhnert, H., Groeneveld, J., and Bickert, T.: Modern and late pleistocene B∕Ca ratios of the benthic foraminifer Planulina wuellerstorfi determined with laser ablation ICP-MS, Geology, 39, 1039–1042, https://doi.org/10.1130/G32009.1, 2011.
Rao, A. M. F., Malkin, S. Y., Hidalgo-Martinez, S., and Meysman, F. J. R.: The impact of electrogenic sulfide oxidation on elemental cycling and solute fluxes in coastal sediment, Geochim. Cosmochim. Ac., 172, 265–286, https://doi.org/10.1016/j.gca.2015.09.014, 2016.
Reichart, G. J., den Dulk, M., Visser, H. J., van Der Weijden, C. H., and Zachariasse, W. J.: A 225 kyr record of dust supply, paleoproductivity and the oxygen minimum zone from the Murray Ridge (Northern Arabian Sea), Palaeogeogr. Palaeocl., 134, 149–169, https://doi.org/10.1016/S0031-0182(97)00071-0, 1997.
Reichart, G. J., Jorissen, F., Anschutz, P., and Mason, P. R. D.: Single foraminiferal test chemistry records the marine environment, Geology, 31, 355–358, https://doi.org/10.1130/0091-7613(2003)031<0355:SFTCRT>2.0.CO;2, 2003.
Saad, S. A. and Wade, C. M.: Biogeographic distribution and habitat association of Ammonia genetic variants around the coastline of Great Britain, Mar. Micropaleontol., 124, 54–62, https://doi.org/10.1016/j.marmicro.2016.01.004, 2016.
Sadekov, A. Y., Eggins, S. M., and De Deckker, P.: Characterization of Mg∕Ca distributions in planktonic foraminifera species by electron microprobe mapping, Geochem. Geophy. Geosy., 6, Q12P06, https://doi.org/10.1029/2005GC000973, 2005.
Schenau, S. J., Reichart, G. J., and De Lange, G. J.: Oxygen minimum zone controlled Mn redistribution in Arabian Sea sediments during the late Quaternary, Paleoceanography, 17, 1058, https://doi.org/10.1029/2000PA000621, 2002.
Schmiedl, G., Pfeilsticker, M., Hemleben, C., and Mackensen, A.: Environmental and biological effects on the stable isotope composition of recent deep-sea benthic foraminifera from the western Mediterranean Sea, Mar. Micropaleontol., 51(1–2), 129–152, https://doi.org/10.1016/j.marmicro.2003.10.001, 2004.
Schumacher, S., Jorissen, F. J., Mackensen, A., Gooday, A. J., and Pays, O.: Ontogenetic effects on stable carbon and oxygen isotopes in tests of live (Rose Bengal stained) benthic foraminifera from the Pakistan continental margin, Mar. Micropaleontol., 76(3–4), 92–103, https://doi.org/10.1016/j.marmicro.2010.06.002, 2010.
Schweizer, M., Polovodova, I., Nikulina, A., and Schönfeld, J.: Molecular identification of Ammonia and Elphidium species (Foraminifera, Rotaliida) from the Kiel Fjord (SW Baltic Sea) with rDNA sequences, Helgoland Mar. Res., 65, 1–10, https://doi.org/10.1007/s10152-010-0194-3, 2011.
Seitaj, D., Schauer, R., Sulu-Gambari, F., Hidalgo-Martinez, S., Malkin, S. Y., Burdorf, L. D. W., Slomp, C. P., and Meysman, F. J. R.: Cable bacteria generate a firewall against euxinia in seasonally hypoxic basins, P. Natl. Acad. Sci. USA, 112, 13278–13283, https://doi.org/10.1073/pnas.1510152112, 2015.
Seitaj, D., Sulu-Gambari, F., Burdorf, L. D. W., Romero-Ramirez, A., Maire, O., Malkin, S. Y., Slomp, C. P., and Meysman, F. J. R.: Sedimentary oxygen dynamics in a seasonally hypoxic basin, Limnol. Oceanogr., 62, 452–473, https://doi.org/10.1002/lno.10434, 2017.
Sen Gupta, B. K., Turner, R. E., and Rabalais, N. N.: Seasonal oxygen depletion in continental-shelf waters of Louisiana?: Historical record of benthic foraminifers, Geology, 24, 227–230, 1996.
Slomp, C. P., Malschaert, J. F. P., Lohse, L., and van Raaphorst, W.: Iron and manganese cycling in different sedimentary environments on the North Sea continental margin, Cont. Shelf Res., 17, 1083–1117, 1997.
Sulu-Gambari, F., Seitaj, D., Meysman, F. J. R., and Schauer, R.: Cable bacteria control iron-phosphorus dynamics in sediments of a coastal hypoxic basin, Environ. Sci. Technol., 50, 1227–1233, https://doi.org/10.1021/acs.est.5b04369, 2016a.
Sulu-Gambari, F., Seitaj, D., Behrends, T., Banerjee, D., Meysman, F. J. R., and Slomp, C. P.: Impact of cable bacteria on sedimentary iron and manganese dynamics in a seasonally-hypoxic marine basin, Geochim. Cosmochim. Ac., 192, 49–69, https://doi.org/10.1016/j.gca.2016.07.028, 2016b.
Sulu-Gambari, F., Roepert, A., Jilbert, T., Hagens, M., Meysman, F. J. R., and Slomp, C. P.: Molybdenum dynamics in sediments of a seasonally-hypoxic coastal marine basin, Chem. Geol., 466(July), 627–640, https://doi.org/10.1016/j.chemgeo.2017.07.015, 2017.
Sundby, B. and Silverberg, N.: Manganese fluxes in the benthic boundary layer, Limnol. Oceanogr., 30, 372–381, https://doi.org/10.4319/lo.1985.30.2.0372, 1985.
Thamdrup, B., Fossing, H., and Jørgensen, B. B.: Manganese, iron, and sulfur cycling in a coastal marine sediment, Aarhus Bay, Denmark, Geochim. Cosmochim. Ac., 58, 5115–5129, 1994.
Thibault de Chanvalon, A., Metzger, E., Mouret, A., Cesbron, F., Knoery, J., Rozuel, E., Launeau, P., Nardelli, M. P., Jorissen, F. J., and Geslin, E.: Two-dimensional distribution of living benthic foraminifera in anoxic sediment layers of an estuarine mudflat (Loire estuary, France), Biogeosciences, 12, 6219–6234, https://doi.org/10.5194/bg-12-6219-2015, 2015.
Thibault de Chanvalon, A., Mouret, A., Knoery, J., Geslin, E., Péron, O., and Metzger, E.: Manganese, iron and phosphorus cycling in an estuarine mudflat, Loire, France, J. Sea Res., 118, 92–102, https://doi.org/10.1016/j.seares.2016.10.004, 2016.
van de Velde, S. and Meysman, F. J. R.: The influence of bioturbation on iron and sulphur cycling in marine sediments: a model analysis, Aquat. Geochem., 22, 469–504, https://doi.org/10.1007/s10498-016-9301-7, 2016.
van de Velde, S., Lesven, L., Burdorf, L. D. W., Hidalgo-Martinez, S., Geelhoed, J. S., Van Rijswijk, P., Gao, Y., and Meysman, F. J. R.: The impact of electrogenic sulfur oxidation on the biogeochemistry of coastal sediments: A field study, Geochim. Cosmochim. Ac., 194, 211–232, https://doi.org/10.1016/j.gca.2016.08.038, 2016.
van der Weijden, C. H., Reichart, G.-J., and van Os, B. J. H.: Sedimentary trace element records over the last 200 kyr from within and below the northern Arabian Sea oxygen minimum zone, Mar. Geol., 231, 69–88, https://doi.org/10.1016/j.margeo.2006.05.013, 2006.
Van der Zwaan, G. J., Duijnstee, I. A. P., den Dulk, M., Ernst, S. R., Jannink, N. T., and Kouwenhoven, T. J.: Benthic foraminifers: proxies or problems? A review of paleocological concepts, Earth-Sci. Rev., 46, 213–236, 1999.
Vandieken, V., Nickel, M., and Jørgensen, B. B.: Carbon mineralization in Arctic sediments northeast of Svalbard: Mn(IV) and Fe(III) reduction as principal anaerobic respiratory pathways, Mar. Ecol.-Prog. Ser., 322, 15–27, https://doi.org/10.3354/meps322015, 2006.
Wickham, H.: ggplot2: Elegant Graphics for Data Analysis, Springer New York, New York, 2009.
Wit, J. C., Reichart, G. J., Jung, S. J. A., and Kroon, D.: Approaches to unravel seasonality in sea surface temperatures using paired single-specimen foraminiferal δ18O and Mg∕Ca analyses, Paleoceanography, 25, PA4220, https://doi.org/10.1029/2009PA001857, 2010.