the Creative Commons Attribution 4.0 License.
the Creative Commons Attribution 4.0 License.
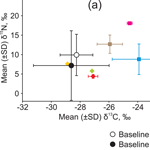
Immediate increase in isotopic enrichment in small mammals following the expansion of a great cormorant colony
Raminta Skipitytė
Marius Jasiulionis
Laima Balčiauskienė
Vidmantas Remeikis
Colonies of great cormorants (Phalacrocorax carbo) impact terrestrial ecosystems through the transport of nutrients from aquatic to terrestrial ecosystems. Deposited guano overload the ecosystem with N and P, change soil pH and damage vegetation. The ways in which small mammals are impacted, however, are little known. We aimed to evaluate the effects of an expanding great cormorant colony, testing if the expansion immediately increased the input of biogens into the forest ecosystem and, further, if the growing influence of the colony was reflected in basal resources (plants and invertebrates) and the hair of small mammals. δ15N and δ13C signatures were analyzed in granivorous yellow-necked mice (Apodemus flavicollis), omnivorous bank voles (Myodes glareolus) and basal resources of animal and plant origin from the territory of a colony of great cormorants situated near the Baltic Sea in west Lithuania. We found that biogens transferred by great cormorants to the terrestrial ecosystem affected the potential foods of the small mammals and led to highly elevated and variable δ15N values. An increase of the size of the colony in 2015 resulted in isotopic enrichment of the small mammals in the zone of expansion in comparison to levels in 2014. The increase of δ15N in A. flavicollis was 7.5 % (p < 0.05) in the ecotone and 5.7 % in the expansion zone. The decrease in δ13C signatures in A. flavicollis was 4.5 % (p < 0.1) in the expansion zone and 3.1 % (p < 0.001) in the colony. In M. glareolus, the decrease in δ13C signatures was 8.5 % in the expansion zone, 3.3 % (p < 0.1) in the control zone and 2.6 % in the ecotone. Isotopic niches (central ellipses) of A. flavicollis in the colony and between the control and expansion zones were separated in 2014 and 2015, while they partially overlapped in the ecotone. The isotopic niches of M. glareolus in 2014 and 2015 were separated in the ecotone and had a small overlap in the colony. For most of the resources tested, the isotopic signatures in the established colony area were significantly higher than in the rest of cormorant-inhabited area. In the colony, the δ15N values in plants (16.9 ± 1.1 ‰) were higher than in invertebrates (13.6 ± 0.4 ‰). In the ecotone, the δ15N values were 12.0 ± 1.4 in plants and 14.7 ± 0.04 ‰ in invertebrates, while in the expansion zone they were 7.2 ± 3.0 and 9.9 ± 3.8 ‰, respectively. δ15N-rich resources led to increased δ15N values in the hair of A. flavicollis and M. glareolus. Thus, biogens from the great cormorant colony immediately affected small mammals through their food sources.
- Article
(752 KB) - Full-text XML
-
Supplement
(504 KB) - BibTeX
- EndNote
Great cormorants (Phalacrocorax carbo) have one of the greatest impacts on the terrestrial ecosystems of all birds breeding in colonies and transporting nutrients from aquatic to terrestrial ecosystems (Klimaszyk et al., 2015). Cormorant excreta change soil pH, N and P levels and damage vegetation (Kameda et al., 2006; Klimaszyk and Rzymski, 2016), decreasing the diversity of plants (Boutin et al., 2011) and affecting seed germination (Žółkoś and Meissner, 2008). Cormorant faeces may cover up to 80 % of vegetation, with as many as 70 % of plant species disappearing in the established colonies, the rest being dominated by nitrophilous plants, such as elder (Sambucus nigra), common nettle (Urtica dioica), woodland groundsel (Senecio sylvaticus) and greater celandine (Chelidonium majus; Goc et al., 2005; Klimaszyk et al., 2015). The abundance and diversity of herbivorous insects also decreases, while other arthropod groups may be abundant (Kolb et al., 2012).
With regard to great cormorant colonies and small mammals, investigations are scarce. In previous studies, we have discovered that the great cormorant colony in Juodkrantė, Lithuania, changes the ecology of the small mammals inhabiting the territory, decreasing their diversity and abundance (Balčiauskienė et al., 2014), as well as changing population structure and fitness (Balčiauskas et al., 2015) – these changes indicate poor habitat and heightening values of δ13C and δ15N in their hair (Balčiauskas et al., 2016). Unexpectedly, the number of breeding cormorant pairs in the colony increased in 2015 due to the absence of deterrent measures. Cormorants built nests in formerly uninhabited territories, giving a unique opportunity to evaluate the immediate effect of colony formation. As a bigger number of nests should be related to increased biological pollution, we hypothesized that stable isotope values will also increase in small mammal hair.
We analyzed the isotope composition in small mammal hair during the period of cormorant colony growth, comparing the isotopic signatures in samples of small mammal hair obtained in 2014 and 2015. The aim was to evaluate the effects of the transfer of biogens from the aquatic to terrestrial ecosystem by the expanding great cormorant colony. We tested (i) if the expansion of the great cormorant colony immediately increases the input of biogens to the forest ecosystem, and (ii) if the influence of the great cormorant colony is reflected in the basal resources (plants and invertebrates) and the hair of small mammals. The novelty of our investigation was in evaluating the immediacy of the impact of the great cormorant colony on small mammals. The results for the first time showed how fast biogenic pollution is transferred and what the consequences are to small mammal ecology. The immediacy of the impact of the new nests has a practical implication, specifically illustrating potential negative consequences if bird scaring is deployed and the colony moves to a new territory.
2.1 Study site
In 2015, small mammals were trapped and samples of their possible foods were collected in the territory of the biggest colony of great cormorants (Phalacrocorax carbo) in Lithuania (Fig. 1), situated near the settlement of Juodkrantė (WGS 55∘31′14.22 N, 21∘6′37.74 E). The colony is in Kuršių Nerija National Park, which has been a UNESCO World Heritage site since 2000.
The colony existed in the 19th century, but due to persecution disappeared in 1887. Returning only after 100 years, breeding cormorants were again registered in 1989 and thereafter the colony rapidly expanded – reaching 1000 breeding pairs by 1999 and 2800 pairs in 2004. In the same year, measures to limit breeding success at the Juodkrantė great cormorant colony were implemented (Knyva, unpublished) with the aim of limiting colony expansion. Regardless, over 3500 nests have been recorded in the colony each year since 2010, with the exception of 2014 when, due to stringent control measures (firing petards in the nesting period), the number of successful pairs was under 2000. In 2015, measures were not applied, resulting in colony growth. In that year, nests appeared in an area that had been free of cormorants in 2014 and had been used as control zone in Balčiauskas et al. (2016).
Three zones were defined for this study, namely the colony, ecotone and expansion zone. The colony included the area with the highest concentration of nests and the area of former active influence with dead trees. The ecotone (position of the trap lines shown in Fig. 1a) was situated between the colony and forest not used by cormorants (shown in darker green in Fig. 1b). Its position did not change in 2011–2014, but a small number of nests did appear in the ecotone in 2015. Also in 2015, the area of the colony expanded by about 3 ha northward (Fig. 1b), the expansion zone. Nests and droppings appeared in the zone, but trees showed no visible influence of the birds. In 2013–2014, this expansion zone was used as trapping control (Balčiauskas et al., 2015, 2016); thus we were able to compare results to find colony influence after a single year of cormorant breeding in a formerly unaffected territory
2.2 Small mammal sampling
Small mammals were trapped in September 2015, using lines of 25 snap traps placed every 5 m. The expansion and ecotone zones had two such lines each, and six lines were located in the great cormorant colony (Fig. 1a). Baited with bread and sunflower oil, the traps were left for 3 days and checked every morning. Trapping effort was equal to 750 trap days and 125 individuals were trapped. Individuals were measured, and sex and age were recorded during dissection as described elsewhere (Balčiauskas et al., 2015). The study was conducted in accordance with the principles of Lithuanian legislation for animal welfare and wildlife.
The dominant species were yellow-necked mice (Apodemus flavicollis) and bank voles (Myodes glareolus), while harvest mice (Micromys minutus), root voles (Microtus oeconomus) and short-tailed voles (M. agrestis) were also trapped in very low numbers. Two shrew species, common (Sorex araneus) and pygmy shrews (S. minutus), were trapped occasionally. The trapped rodent species differ in food preferences, ranging from herbivory in Microtus to granivory in Apodemus and Micromys, and omnivory in Myodes (Butet and Delettre, 2011; Čepelka et al., 2014). In general though, small mammals are mostly omnivorous (Nakagawa et al., 2007), though Sorex typically consume invertebrates (Makarov and Ivanter, 2016).
The numbers of rodents trapped in the different zones of the territory (control/expansion, ecotone and the colony itself) in 2014 and 2015 are presented in Table 1, while the age and sex composition of the two dominant species in 2015 are shown in Table S1. No small mammals were trapped in the areas of the great cormorant colony that contained the highest concentration of nests and had experienced long-term influence. In the expansion part of the colony, only A. flavicollis was trapped in significant numbers in 2015.
2.3 Baseline sampling
Isotopic signatures were evaluated and isotopic baselines were established from possible dietary items. In 2015, we collected samples of the possible food items at the locations where the small mammals were trapped or at the closest available place. In the most affected zones, plant diversity was extremely restricted, with just a few nitrophilous species present. In total, 45 plant and 9 invertebrate samples were collected. Five litter samples and seven samples of great cormorant feathers and eggshells were also collected.
Plant samples included leaves of greater celandine (Chelidonium majus), sedges (Carex sp.), raspberry (Rubus idaeus), rush (Juncus sp.), blackberry (Rubus fruticosus) and bilberry (Vaccinium myrtillus), leaves and berries of elder (Sambucus nigra), alder buckthorn (Rhamnus frangula) and European barberry (Berberis vulgaris), and oak (Quercus robur) acorns. Invertebrate samples included coprophagous dung beetle (Geotrupes stercorarius), herbivorous dark bush-cricket (Pholidoptera griseoaptera), predatory ground beetle (Carabus sp.) and omnivorous land slug (Deroceras sp.). Quantifying of the different foods by volume was not done. Unfortunately, we have no data on the isotopic signatures in basal resources from the pre-expansion period in 2014.
2.4 Stable isotope analysis
We used the hair of the rodents as metabolically inert samples, these preserving the isotopic record of the animal's diet (Crawford et al., 2008; Bauduin et al., 2013). Hair samples were taken with scissors from between the shoulders of most of the trapped specimens of A. flavicollis and M. glareolus (88 and 29 individuals; see Table S1 in the Supplement). Individuals contaminated by fly larvae or predators were not sampled for stable isotopes. Each sample was placed separately in a bag and stored dry. Samples were weighed with a microbalance and packed in tin capsules. As few individuals of these species survive longer than 1 year, with most over-wintering individuals being autumn born (Bobek, 1969), our samples thus represent the influence of the cormorants in the year that the rodents were trapped.
Environmental samples (including plants, litter, invertebrates and great cormorant feathers and eggs) were stored in a freezer at below −20 ∘C prior to preparation and analysis. Samples were dried in an oven at 60 ∘C to a constant weight for 24–48 h and then homogenized to a fine powder (using mortar and pestle and a Retsch mixer mill MM 400). Pretreatment of hair and other samples was not done, as after testing it produced no change of results. Feathers were cleaned with acetone and deionized water prior to measurements. Feather samples were clipped from the vane avoiding the rachis.
Stable isotope ratios (δ13C and δ15N) were measured using an elemental analyzer (EA) coupled to an isotope ratio mass spectrometer (Flash EA1112; Thermo Delta V Advantage, Thermo Scientific, USA). Stable isotope data are reported as δ values, according to the formula δX= [] × 103, where Rsample=13C/12C or 15N/14N of the sample, Rstandard= 13C/12C or 15N/14N of the standard.
5 % of samples were run in duplicate. The equipment parameters and measurement quality control are detailed elsewhere (Balčiauskas et al., 2016).
2.5 Statistical analysis
The normality of distribution of δ13C and δ15N values was tested using the Kolmogorov–Smirnov D. As not all values of δ13C and δ15N were distributed normally, the influences of species and the zone of the colony on the carbon and nitrogen stable isotope values in the mammal hair were tested using nonparametric Kruskal–Wallis ANOVA. Independent groups were compared with the same Kruskal–Wallis multiple comparisons procedure (StatSoft, 2013). Differences in δ13C and δ15N ratios between 2014 (data from Balčiauskas et al., 2016) and 2015 were tested by multivariate Hotelling T2 test. The minimum significance level was set at p < 0.05. Calculations were performed using Statistica for Windows, version 6.0.
Environmental samples were analyzed by object group (cormorant, litter, invertebrates, plants) and by the zone (expansion, ecotone, colony). Isotopic baselines were calculated using basal resources as possible foods for rodents grouped according to their origin. Reported values are arithmetic means with SE of the δ15N and δ13C for all basal resources mentioned above.
The isotopic niches of species, as central ellipses, were calculated using SIBER (Jackson et al., 2011) under R version 3.5.0 (https://cran.r-project.org/bin/windows/base/rdevel.html, last access: 2 June 2018) for A. flavicollis and M. glareolus in the zones, where five or more individuals were investigated for δ15N and δ13C.
3.1 δ13C and δ15N values in the hair of small mammals inhabiting the great cormorant colony
The distribution of both δ13C and δ15N values in the hair of A. flavicollis and the distribution of δ15N values in M. glareolus were not normal, though the distribution δ13C in M. glareolus values corresponded to normal. Outliers from the normal distribution were values registered in the expansion zone (Fig. S1 in the Supplement). Kruskal–Wallis ANOVA demonstrated that the distribution of stable isotope values was influenced not only by zone, but also by the species of small mammal. These factors together significantly influenced the distribution of δ15N (r2= 0.31) and δ13C (r2= 0.26, F both p < 0.0001).
In 2015, the influence of the zone (both species pooled) was significant for the distribution of δ15N (Kruskal–Wallis ANOVA, H 18.62, p= 0.0001) and δ13C (H 6.30, p= 0.043). Between-species differences in the stable isotope values in the hair of the rodents in the colony area were highly significant for δ13C (H 21.69, p < 0.0001) and for δ15N (H 6.67, p= 0.01). δ15N values were highest in the hair of M. glareolus trapped in the ecotone and colony zones, while highest in A. flavicollis in the expansion zone. δ13C signatures in the hair of A. flavicollis were higher than in M. glareolus in all territories, including the expansion zone (Table 2).
With the expansion of the great cormorant colony in 2015, the isotopic signatures of δ15N in dominant small mammal hair grew in comparison to 2014, though not all differences are significant (Table 2). In A. flavicollis, δ15N values increased in all zones (the 7.5 % increase in the ecotone zone is significant at p < 0.05). The increase in the colony was ∼ 1 %, while the expansion zone compared to former control zone was 5.7 %. All are correlated with colony growth and expansion. In M. glareolus, δ15N increased by 2.3 % in the ecotone zone and ∼ 1 % in the colony.
Table 2Central position (mean ± SE, ‰) of stable isotope ratios in the hair of Apodemus flavicollis and Myodes glareolus trapped in the Juodkrantė colony of great cormorants. Data for 2014 recalculated from Balčiauskas et al. (2016). Significance of differences according to the Hotelling T2 multivariate test, * p < 0.1, p < 0.05, p < 0.001.
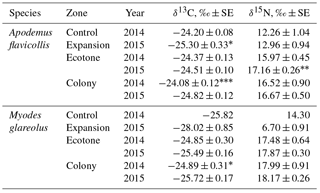
δ13C signatures in the hair of A. flavicollis in 2015 decreased in all zones. The decrease in the expansion zone compared to 2014 control zone was 4.5 % (p < 0.1), in the colony zone 3.1 % (p < 0.001) and in the ecotone zone 0.5 %. In the hair of M. glareolus, the decrease of δ13C signatures was even stronger – 8.5 % in the expansion zone, 3.3 % (p < 0.1) in the control zone and 2.6 % in the ecotone (Table 2). We suppose that no other factor other than colony growth could account for these changes.
Isotopic niches of A. flavicollis in 2014 and 2015 (shown as central ellipses) were separated in the colony and between the control and expansion zones, while they partially overlapped in the ecotone (Fig. S2a). The isotopic niches of M. glareolus in 2014 and 2015 were separated in the ecotone and had a small overlap in the colony (Fig. S2b). Insufficient sample size did not allow analysis in the control and expansion zones for this species (see Table 1).
3.2 Basal resources
Comparing average baseline data of plants and invertebrates between the expansion zone, ecotone and colony (Table 3), considerable differences were noted in the δ15N of plants (Kruskal–Wallis ANOVA, H2,45 = 13.89, p= 0.001) but not in invertebrates (H 2.76, p= 0.25). In plants, δ15N values were highest in the colony (difference from expansion zone, p < 0.002; difference from ecotone, p= 0.062). δ13C values showed no significant differences between zones in either plants or invertebrates (Table 3).
Out of ten plant species, the most 15N enriched were as follows: Chelidonium majus, δ15N = 19.6 ± 2.0 ‰ (from 8.4 in the expansion zone to 25.7 ‰ in the colony); Sambucus nigra, δ15N = 16.9 ± 2.6 ‰ (from 9.2 in the ecotone to 22.5 ‰ in the colony); Juncus sp., δ15N = 13.8 ± 4.7 ‰; and Rhamnus frangula, δ15N = 13.2 ± 1.8 ‰ (from 8.1 ‰ in the expansion zone to 16.3 ‰ in the colony). δ15N values in Carex sp. (average δ15N = 12.3 ± 0.9 ‰) did not differ significantly across zones.
The most δ13C-enriched plants were Carex sp. with δ13C = −26.7 ± 0.7 ‰ and Sambucus nigra with δ13C = −27.5 ± 0.6 ‰, values in the expansion zone and colony did not differ. Chelidonium majus was among the least δ13C-enriched plants, δ13C = −29.5 ± 0.4 ‰.
Of the investigated invertebrates, the most δ15N enriched were Carabus sp. and slugs, δ15N = 14.9 and 14.0 ‰, respectively. Dung beetles showed the highest variation of δ15N, being 2 times lower in the expansion zone than in the colony (6.2 ‰ versus 13.3 ‰). The stable carbon isotope ratio in slugs was about 1 ‰ higher than in arthropods.
δ15N values in the litter were highest in the colony (δ15N = 16.3 ‰), followed by the ecotone and expansion zones (δ15N = 8.2 and 7.3 ‰, respectively). δ15N values in the cormorant eggshells and feathers were lower than in rodent hair and did not depend on zone (Fig. S3).
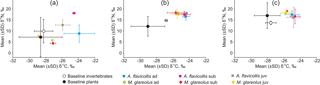
Figure 2Isotopic signatures of potential animal and plant foods compared with isotopic signatures in the hair of age groups of Apodemus flavicollis and Myodes glareolus, trapped in the expansion (a) and ecotone (b) zones and Juodkrantė great cormorant colony (c) in 2015. Ad – adults, sub – subadult animals, juv – juveniles.
3.3 Comparison of isotopic signatures in the hair of small mammals and in possible diet resources
Comparison of isotopic signatures in the hair of A. flavicollis and M. glareolus with baseline δ13C and δ15N values in possible food sources from the expansion, ecotone and colony zones showed that noted differences were related to bird influence.
In the expansion and ecotone zones, invertebrate isotopic signatures were higher than in plants in terms of both δ13C and δ15N. In the great cormorant colony, most plants were highly enriched in 15N due to over-enrichment and tended to have δ15N values well above the invertebrate δ15N range (Fig. 2).
Table 4Nitrogen (Δδ15N) and carbon (Δδ13C) trophic fractionation between Apodemus flavicollis and Myodes glareolus and their possible food sources in the Juodkrantė great cormorant colony.
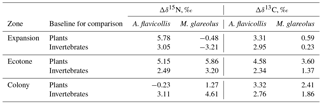
Compared to average plant and invertebrate baseline values, the higher average δ15N and δ13C values in the hair of A. flavicollis and M. glareolus were related to the zones where the rodents were trapped (Table 4). For A. flavicollis trapped in the expansion and ecotone zones, the average δ15N was over 5 ‰ higher than the plant baseline, but the plant baseline in the colony was higher than the δ15N value in the hair. Compared to the invertebrate baseline, enrichment of 15N in the rodent hair was 2.5–3 ‰. Concerning M. glareolus, 15N enrichment was highest in the ecotone when compared to the plant baseline, but highest in the colony zone when compared to the invertebrate baseline. As for 13C, enrichment was highest in the ecotone zone for both A. flavicollis and M. glareolus (Table 4).
4.1 Immediacy of the great cormorant colony impact
Our main finding showed that great cormorants influenced small mammals in the very first year of the appearance of breeding colony, and possible food objects (plants and invertebrates) also were subjected to increased δ15N and decreased δ13C concentrations. Moreover, in 2015 – the year of colony increase and expansion – we found that the increased influence of the great cormorant colony already limited small mammal distribution. Small mammals were not trapped in the area with the highest concentration of nests. By contrast, representatives of three small mammal species had been trapped in the same place in earlier years when the number of nests was lower due to scaring measures. In the expansion part of the colony, a single individual of M. glareolus was trapped in 2015, while only a single species, A. flavicollis, was trapped in significant numbers. In 2014, before colony expansion, four small mammal species were trapped in the same area.
4.2 Biogenic pollution disclosed by stable isotope concentration in the hair of small mammals
Investigations into the influence of great cormorant colonies have recently received more attention (see Ishida, 1996; Goc et al., 2005; Kameda et al., 2006; Nakamura et al., 2010; Klimaszyk et al., 2015; Klimaszyk and Rzymski, 2016). However, the impact of such colonies on plant and animal species has not been sufficiently investigated (see Bostrom et al., 2012; Kolb et al., 2012). Small mammal ecology in the colonies was recently investigated for the first time in Lithuania (Balčiauskienė et al., 2014; Balčiauskas et al., 2015) and some aspects of isotopic enrichment of small mammals in great cormorant colonies were reviewed in Balčiauskas et al. (2016). We found that biogenic pollution resulting from the birds reach the dominant species of small mammals. Stable isotope ratios in their hair depend on the degree of cormorant influence, being strongest in the area with cormorant nests (see data in Table 2) and also on the colony size. However, we had no data regarding stable isotopes in basal resources and we were not aware how fast the influence could be.
4.3 Stable isotopes showing small mammal diet differences in various zones of cormorant colony
Stable isotope analysis (SIA) can be used to investigate the trophic structure of food webs and various aspects of animal diet (Boecklen et al., 2011; Koike et al., 2016). SIA using mammal hair is a method suitable for diet analysis and for comparing intrapopulation groups as well as different species. When analyzing diet, hair isotopic signatures are compared with the signatures of possible food sources (Bauduin et al., 2013). In identifying diet sources, carbon and nitrogen isotope ratios are most widely used. The tissues of animals do differ in isotopic composition as a result of differences in their diet. Nitrogen values help in identifying the trophic position, since these values increase by 3–5 ‰ between levels of the food chain (Kelly, 2000; Smiley et al., 2016). The stable isotope ratio in the hair reflects the dietary isotope composition and trophic level, depending on the ingested food (Cassaing et al., 2007).
As the hair of the rodents trapped in the Juodkrantė colony, ecotone and expansion zone differed in δ15N and δ13C values, rodents obviously consumed foods with different isotopic signatures, the more diverse diet being in the expansion zone as reflected by much higher variance of δ13C values. Enrichment of plants and invertebrates was strongest in the territory of the colony (see Fig. 2).
Species-related differences in the isotopic signatures of the two dominant rodent species may be explained by diet differences and microhabitat use, both supporting coexistence (Stenseth et al., 2002; Cassaing et al., 2013). Previous experience with live-trapping and marking of A. flavicollis in the cormorant colony (Jasiulionis, unpublished) allowed us to conclude that movement between zones was very limited: we did not find any marked animals using multiple zones during the same year. In the resource-scarce territory of the great cormorant colony, any spatial segregation could lead to changes in the diet of the rodents. Considering the isotopic signatures in the hair of the small mammals as dietary proxies (according to Fernandes et al., 2014) reflecting the proteins of the food sources (Perkins et al., 2014), we found that diets differed in the various zones of the colony and also depended on the small mammal species. Therefore, differential exploitation of resources minimized competition (according Bauduin et al., 2013).
4.4 Pathways of small mammal enrichment in stable isotopes
There is a slight possibility that the observed rodent enrichment in δ15N was a result of eating cormorant tissues. Dead chicks, broken eggs and eggshells are constantly present on the ground underneath the nests in the breeding season, and so could serve as food source. Moreover, δ15N values in great cormorant eggshells and feathers were lower than the δ15N values in the hair of rodents from the same zone (see Fig. S2). Furthermore, there are observations of Microtus and Myodes voles eating auklets' eggs and chicks (Drever et al., 2000). In our case however, the difference in δ15N values between the cormorants and rodents was not great, questioning the possibility of consumption of cormorant tissue in any significant amount. Thus we support the opinion of Millus and Stapp (2008), that cormorant influence on small mammals is not direct, but is mediated through influence on their food resources.
The two possible pathways of marine nitrogen are (1) through guano-fertilized plants or (2) invertebrates that have fed on guano, guano-fertilized plants or cormorant remains (Harper, 2007). According to Szpak et al. (2012), 15N enrichment of plants may range from 11.3 to 20 ‰ after fertilization by guano of seabirds. Plants enriched in guano 15N may occur at distances exceeding 100 m from nesting sites and colonies (Millus and Stapp, 2008). A few plant samples (Carex and Sambucus) were highly enriched in 15N in the expansion zone of Juodkrantė colony in 2015, the first year of the presence of cormorant nests.
Rodents usually eat foods that are most abundant (Bauduin et al., 2013) or have preferences characteristic to the species (Fisher and Türke, 2016; Schneider et al., 2017). However, choices in the Juodkrantė great cormorant colony are limited to several plant species (mainly nitrophilous) and invertebrates. Nitrophilous plants usually grow abundantly, being the food source for herbivores living in the territory of a colony (Cassaing et al., 2007). Even after birds cease to use a territory, the isotopic signatures of the litter and plants remain high (Kameda et al., 2006). Enrichment of plants by 15N is a result of uptake of nitrogen from bio-polluted soil enriched by marine-derived N from great cormorant excreta. In such a situation, δ15N is not a straightforward indicator of the trophic level (Drever at al., 2000). On seabird islands, herbivores often exhibit heightened δ15N signatures (Stapp et al., 1999; Drever et al., 2000).
Typically, diet–tissue fractionation is from 2.5 to 3.4 ‰ for nitrogen (Perkins et al., 2014). Trophic fractionation from 3 to 5 ‰ for nitrogen occurs at every trophic level in seabird colonies (Cassaing et al., 2007). However, in Juodkrantė, it may exceed 5 ‰ in comparison to plants. Trophic fractionation for carbon of A. flavicollis was at a predictable level, up to 4.6 ‰ in comparison to plants, and up to 3 ‰, compared to the invertebrate baseline. Enrichment in 13C of M. glareolus was lower, up to 3.6 ‰ compared to plants and up to 1.9 ‰, compared to the invertebrate baseline (see Table 4). These values are similar to or even higher than those observed by Sponheimer et al. (2003), with a mean diet–hair fractionation of +3.2 ‰ and a range of +2.7 to +3.5 ‰ in mammalian herbivores.
This study seeks to understand how the influence of biological pollution from the great cormorant colony reaches small mammals and how fast this influence is registered after birds appear in the territory. The general conclusion is that in Juodkrantė, Lithuania, the great cormorant colony affected the terrestrial ecosystem starting from the autotrophs and ending with the consumers (two species of rodents). An increase in the number of breeding pairs in 2015 led to increased δ15N and decreased δ13C values in the hair of Apodemus flavicollis in the territory of the colony, ecotone and expansion zone. In the expansion zone, the influence was visible after a single year of nest appearance. In the resource-limited territory under the great cormorant nests, differences in isotopic signatures were related to the species of rodent, pointing to differences in their diet. We conclude that the influence of the nutrient transport from water to land ecosystems by great cormorants is indirect, resulting from the biological pollution of guano on rodent foods. Our results show that scaring cormorants from colonies may have a negative consequence – displaced birds may build their nest in other habitats and, as such, a negative impact of an emerging new colony could spread through the entire ecosystem and impact small mammals in the first year. The immediacy of the impact from new nests has practical implications, indicating the potential benefit of deterring birds from the nests during the actual breeding period as a management measure to limit growth in numbers, rather than scaring them before breeding, which may result in colony fragmentation and the moving to new territories.
Data used in this paper are available upon request from the corresponding author.
The supplement related to this article is available online at: https://doi.org/10.5194/bg-15-3883-2018-supplement.
MJ, LiB and LaB trapped small mammals and collected baseline data. MJ and RS analyzed stable isotopes. LiB analyzed data statistically. LiB and LaB wrote the first draft. All authors provided substantial input to the design of the study and discussion of the results.
The authors declare that they have no conflict of interest.
We thank Associate Editor Sébastien Fontaine and all reviewers for
helpful comments, which greatly improved the quality of the paper. We also
thank Gintautas Vaitonis for help with graphics, Andrius Kučas for help
with SIBER and Jos Stratford for polishing the language of the manuscript.
Edited by: Sébastien Fontaine
Reviewed by: two anonymous referees
Balčiauskas, L., Balčiauskienė, L., and Jasiulionis, M.: Mammals under the colony of great cormorants: population structure and body condition of the yellow-necked mice, Turk. J. Zool., 39, 941–948, https://doi.org/10.3906/zoo-1407-27, 2015.
Balčiauskas, L., Skipitytė, R., Jasiulionis, M., Trakimas, G., Balčiauskienė, L., and Remeikis, V.: The impact of Great Cormorants on biogenic pollution of land ecosystems: Stable isotope signatures in small mammals, Sci. Total Environ., 565, 376–383, https://doi.org/10.1016/j.scitotenv.2016.04.185, 2016.
Balčiauskienė, L., Jasiulionis, M., and Balčiauskas, L.: Loss of Diversity in a Small Mammal Community in a Habitat Influenced by a Colony of Great Cormorants, Acta Zool. Bulgar., 66, 229–234, 2014.
Bauduin, S., Cassaing, J., Issam, M., and Martin, C.: Interactions between the short-tailed mouse (Mus spretus) and the wood mouse (Apodemus sylvaticus): diet overlap revealed by stable isotopes, Can. J. Zool., 91, 102–109, https://doi.org/10.1139/cjz-2012-0286, 2013.
Bobek, B.: Survival, turnover and production of small rodents in a beech forest, Acta Theriol., 14, 191–210, https://doi.org/10.4098/AT.arch.69-15, 1969.
Boecklen, W. J., Yarnes, C. T., Cook, B. A., and James, A. C.: On the use of stable isotopes in trophic ecology, Annu. Rev. Ecol. Evol. S., 42, 411–440, https://doi.org/10.1146/annurev-ecolsys-102209-144726, 2011.
Bostrom, M. K., Ostman, O., Bergenius, M. A. J., Mikaela, A. J., and Lunneryd, S. G.: Cormorant diet in relation to temporal changes in fish communities, ICES J. Mar. Sci., 69, 175–183, https://doi.org/10.1093/icesjms/fss002, 2012.
Boutin, C., Dobbie, T., Carpenter, D., and Hebert, C. E.: Effect of Double Crested Cormorant on island vegetation, seedbank and soil chemistry: evaluating island restoration potential, Restor. Ecol., 19, 720–727, https://doi.org/10.1111/j.1526-100X.2010.00769.x, 2011.
Butet, A. and Delettre, Y. R.: Diet differentiation between European arvicoline and murine rodents, Acta Theriol., 56, 297–304, https://doi.org/10.1007/s13364-011-0049-6, 2011.
Cassaing, J., Derré, C., Moussa, I., and Cheylan, G.: Diet variability of Mediterranean insular populations of Rattus rattus studied by stable isotope analysis, Isot. Environ. Healt. S., 43, 197–213, https://doi.org/10.1080/10256010701562919, 2007.
Cassaing, J., Riviere, B. L. P. D. L., Donno, F. D., Marlinez-Garcia, E., and Thomas, C.: Interactions between 2 Mediterranean rodent species: Habitat overlap and use of heterospecific cues, Ecoscience, 20, 137–147, https://doi.org/10.2980/20-2-3560, 2013.
Čepelka, L., Heroldová, M., Jánová, E., and Suchomel, J.: The dynamics of nitrogenous substances in rodent diet in a forest environment, Mammalia, 78, 327–333, https://doi.org/10.1515/mammalia-2013-0017, 2014.
Crawford, K., Mcdonald, R. A., and Bearhop, S.: Applications of stable isotope techniques to the ecology of mammals, Mammal Rev., 38, 87–107, https://doi.org/10.1111/j.1365-2907.2008.00120.x, 2008.
Drever, M. C., Blight, L. K., Hobson, K. A., and Bertram, D. F.: Predation on seabird eggs by Keen's mice (Peromyscus keeni): using stable isotopes to decipher the diet of a terrestrial omnivore on a remote offshore island, Can. J. Zool., 78, 2010–2018, 2000.
Fernandes, R., Millard, A. R., Brabec, M., Nadeau, M. J., and Grootes, P.: Food reconstruction using isotopic transferred signals (FRUITS): a Bayesian model for diet reconstruction, PloS One, 9, e87436, https://doi.org/10.1371/journal.pone.0087436, 2014.
Fischer, C. and Türke, M.: Seed preferences by rodents in the agri-environment and implications for biological weed control, Ecol. Evol., 6, 5796–5807, https://doi.org/10.1002/ece3.2329, 2016.
Goc, M., Iliszko, L., and Stempniewicz, L.: The largest European colony of great cormorant on the Vistula spit (N Poland)—an impact of the forest ecosystem, Ecological Questions, 6, 93–103, 2005.
Harper, G. A.: Detecting predation of a burrow-nesting seabird by two introduced predators, using stable isotopes, dietary analysis and experimental removals, Wildlife Res., 34, 443–453, https://doi.org/10.1071/WR07037, 2007.
Ishida, A.: Effects of the common cormorant, Phalacrocorax carbo, on overgreen forests in two nest sites at Lake Biwa, Japan, Ecol. Res., 11, 193–200, https://doi.org/10.1007/BF02347685, 1996.
Jackson, A. L., Inger, R., Parnell, A. C., and Bearhop, S.: Comparing isotopic niche widths among and within communities: SIBER–Stable Isotope Bayesian Ellipses in R, J. Anim. Ecol., 80, 595–602, https://doi.org/10.1111/j.1365-2656.2011.01806.x, 2011.
Kameda, K., Koba, K., Hobara, S., Osono, T., and Terai, M.: Pattern of natural 15N abundance in lakeside forest ecosystem affected by cormorant-derived nitrogen, Hydrobiologia, 567, 69–86, https://doi.org/10.1007/s10750-006-0052-0, 2006.
Kelly, J. F.: Stable isotopes of carbon and nitrogen in the study of avian and mammalian trophic ecology, Can. J. Zool., 78, 1–27, https://doi.org/10.1139/z99-165, 2000.
Klimaszyk, P. and Rzymski, P.: The complexity of ecological impacts induced by great cormorants, Hydrobiologia, 771, 13–30, https://doi.org/10.1007/s10750-015-2618-1, 2016.
Klimaszyk, P., Brzeg, A., Rzymski, P., and Piotrowicz, R.: Black spots for aquatic and terrestrial ecosystems: impact of a perennial cormorant colony on the environment, Sci. Total Environ., 517, 222–231, https://doi.org/10.1016/j.scitotenv.2015.02.067, 2015.
Koike, S., Nakashita, R., Kozakai, C., Nakajima, A., Nemoto, Y., and Yamazaki, K.: Baseline characterization of the diet and stable isotope signatures of bears that consume natural foods in central Japan, Eur. J. Wildlife Res., 62, 23–31, https://doi.org/10.1007/s10344-015-0969-6, 2016.
Kolb, G., Jerling, L., Essenberg, C., Palmborg, C., and Hambäck, P. A.: The impact of nesting cormorants on plant and arthropod diversity, Ecography, 35, 726–740, https://doi.org/10.1111/j.1600-0587.2011.06808.x, 2012.
Makarov, A. M. and Ivanter, E. V.: Dimensional characteristics of prey and their role in the diet of shrews (Sorex L.), Russ. J. Ecol., 47, 315–319, https://doi.org/10.1134/S1067413616030073, 2016.
Millus, S. A. and Stapp, P.: Interactions between seabirds and endemic deer mouse populations on Santa Barbara Island, California, Can. J. Zool., 86, 1031–1041, https://doi.org/10.1139/Z08-081, 2008.
Nakagawa, M., Hyodo, F., and Nakashizuka, T.: Effect of forest use on trophic levels of small mammals: an analysis using stable isotopes, Can. J. Zool., 85, 472–478, https://doi.org/10.1139/Z07-026, 2007.
Nakamura, M., Yabe, T., Ishii, Y., Kido, K., and Aizaki, M.: Extreme eutrophication in a small pond adjacent to a forest colonized by great cormorant (Phalacrocorax carbo), Jap. J. Limnol., 71, 19–26, https://doi.org/10.3739/rikusui.71.19, 2010.
Perkins, M. J., McDonald, R. A., van Veen, F. F., Kelly, S. D., Rees, G., and Bearhop, S.: Application of nitrogen and carbon stable isotopes (δ15N and δ13C) to quantify food chain length and trophic structure, PloS One, 9, e93281, https://doi.org/10.1371/journal.pone.0093281, 2014.
Schneider, S., Steeves, R., Newmaster, S., and MacDougall, A. S.: Selective plant foraging and the top-down suppression of native diversity in a restored prairie, J. Appl. Ecol., 54, 1496–1504, https://doi.org/10.1111/1365-2664.12886, 2017.
Smiley, T. M., Cotton, J. M., Badgley, C., and Cerling, T. E.: Small-mammal isotope ecology tracks climate and vegetation gradients across western North America, Oikos, 125, 1100–1109, https://doi.org/10.1111/oik.02722, 2016.
Sponheimer, M., Robinson, T., Ayliffe, L., Passey, B., Roeder, B., Shipley, L., Lopez, E., Cerling, T., Dearing, D., and Ehleringer, J.: An experimental study of carbon-isotope fractionation between diet, hair, and feces of mammalian herbivores, Can. J. Zool., 81, 871–876, https://doi.org/10.1139/z03-066, 2003.
Stapp, P., Polis, G. A., and Piñero, F. S.: Stable isotopes reveal strong marine and El Nino effects on island food webs, Nature, 401, 467–469, https://doi.org/10.1038/46769, 1999.
StatSoft, Inc.: Electronic Statistics Textbook, available at: http://www.statsoft.com/textbook/ (last access: 20 July 2017), 2013.
Stenseth, N. C., Vljugrein, H., Jędrzejewski, W., Mysterud, A., and Pucek, Z.: Population dynamics of Clethrionomys glareolus and Apodemus flavicollis: seasonal components of density dependence and density independence, Acta Theriol., 47, 39–67, https://doi.org/10.1007/BF03192479, 2002.
Szpak, P., Millaire, J. F., White, C. D., and Longstaffe, F. J.: Influence of seabird guano and camelid dung fertilization on the nitrogen isotopic composition of field-grown maize (Zea mays), J. Archaeol. Sci., 39, 3721–3740, https://doi.org/10.1016/j.jas.2012.06.035, 2012.
Žółkoś, K. and Meissner, W.: The effect of grey heron (Ardea cinerea L.) colony on the surrounding vegetation and the biometrical features of three undergrowth species, Pol. J. Ecol., 56, 65–74, 2008.