the Creative Commons Attribution 4.0 License.
the Creative Commons Attribution 4.0 License.
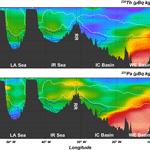
Evolution of 231Pa and 230Th in overflow waters of the North Atlantic
Feifei Deng
Gideon M. Henderson
Maxi Castrillejo
Fiz F. Perez
Reiner Steinfeldt
Many palaeoceanographic studies have sought to use the 231Pa∕230Th ratio as a proxy for deep ocean circulation rates in the North Atlantic. As of yet, however, no study has fully assessed the concentration of, or controls on, 230Th and 231Pa in waters immediately following ventilation at the start of Atlantic meridional overturning. To that end, full water-column 231Pa and 230Th concentrations were measured along the GEOVIDE section, sampling a range of young North Atlantic deep waters. 230Th and 231Pa concentrations in the water column are lower than those observed further south in the Atlantic, ranging between 0.06 and 12.01 µBq kg−1 and between 0.37 and 4.80 µBq kg−1, respectively. Both 230Th and 231Pa profiles generally increase with water depth from surface to deep water, followed by decrease near the seafloor, with this feature most pronounced in the Labrador Sea (LA Sea) and Irminger Sea (IR Sea). Assessing this dataset using extended optimum multi-parameter (eOMP) analysis and CFC-based water mass age indicates that the low values of 230Th and 231Pa in water near the seafloor of the LA Sea and IR Sea are related to the young waters present in those regions. The importance of water age is confirmed for 230Th by a strong correlation between 230Th and water mass age (though this relationship with age is less clear for 231Pa and the 231Pa∕230Th ratio). Scavenged 231Pa and 230Th were estimated and compared to their potential concentrations in the water column due to ingrowth. This calculation indicates that more 230Th is scavenged (∼80 %) than 231Pa (∼40 %), consistent with the relatively higher particle reactivity of 230Th. Enhanced scavenging for both nuclides is demonstrated near the seafloor in young overflow waters. Calculation of the meridional transport of 230Th and 231Pa with this new GEOVIDE dataset enables a complete budget for 230Th and 231Pa for the North Atlantic. Results suggest that net transport southward of 230Th and 231Pa across GEOVIDE is smaller than transport further south in the Atlantic, and indicate that the flux to sediment in the North Atlantic is equivalent to 96 % of the production of 230Th and 74 % of the production for 231Pa. This result confirms a significantly higher advective loss of 231Pa to the south relative to 230Th and supports the use of 231Pa∕230Th to assess meridional transport at a basin scale.
- Article
(4391 KB) - Full-text XML
-
Supplement
(486 KB) - BibTeX
- EndNote
Several palaeoceanographic proxies have been proposed that rely on the 231Pa∕230Th ratio in marine sediments; one of which is that 231Pa∕230Th may record the rate of deep water circulation, particularly in the North Atlantic. Both 231Pa and 230Th are produced in seawater at a constant rate by the decay of uranium, but they have decay activities much lower than their parent uranium isotopes due to rapid removal by adsorption onto sinking marine particles. Both nuclides are also reversibly scavenged, leading to particularly low concentrations at the surface and increasing concentrations with depth (Nozaki et al., 1981). Advection of surface waters to depth transports water with low concentrations of 231Pa and 230Th into the deep ocean, where their concentrations subsequently increase towards an equilibrium value at a rate dependent on the residence time of the nuclide. The longer residence time of 231Pa relative to 230Th (∼130 years versus ∼20 years; Henderson and Anderson, 2003) means that the equilibrium concentration of 231Pa is closer to that expected from uranium decay, and that the time taken to reach this equilibrium is longer.
This oceanic behaviour of 231Pa and 230Th suggests that their measurement in marine sediments may reveal information about the past environment, with one common use being a recorder of deep water circulation, particularly in the North Atlantic (e.g. Gherardi et al., 2005, 2009; McManus et al., 2004; Roberts et al., 2014; Yu et al., 1996). The interpretation of sedimentary 231Pa∕230Th ratios for such past ocean circulation is based on two end-member conceptual models.
-
Basin-scale advection. The longer residence time of 231Pa than 230Th means that deep water contains more 231Pa than 230Th relative to production from decay. Advection of deep waters out of the North Atlantic therefore removes more 231Pa than 230Th, leaving sediments in the basin with a 231Pa∕230Th ratio below the production ratio. If deep water ventilation ceases, 231Pa removal from the North Atlantic also ceases, and sedimentary 231Pa∕230Th values reach their production ratio. This approach was first proposed by Yu et al. (1996), who measured 231Pa∕230Th in Holocene and Last Glacial Maximum (LGM) sediments from many core-top samples from the Atlantic and Southern Ocean. They found similar Holocene and LGM values at a basin scale, suggesting broadly similar overturning during the two periods. Subsequent application to sediments from Heinrich Stadial 1, initially in a single core (McManus et al., 2004) and progressively in a geographical range of cores (Bradtmiller et al., 2014), revealed reduced advection of 231Pa out of the basin at that time, suggesting decreased overturning.
-
Water mass evolution. The longer residence time of 231Pa means that, following ventilation, it takes longer for deep water 231Pa concentrations to reach equilibrium with respect to scavenging than is the case for 230Th. This leads to a systematic evolution of 231Pa∕230Th with age of the water. Sediments capture this ratio (with a fractionation due to different scavenging coefficients for the two nuclides), thus capturing information about the age of the water. Simple models suggest an increase in 231Pa∕230Th with age of over about 400 years (e.g. several residence times of 231Pa). This approach to interpreting sedimentary 231Pa∕230Th allows for the possibility of calculating flow rates for a single water mass and from a single core, rather than at a basin scale. It has been pursued by Negre et al. (2010) to assess deep water flow in both southerly and northerly directions by comparing sediments in the North and South Atlantic, and allowed these authors to apply a simple model (Thomas et al., 2007) to calculate flow rates.
Recent water-column measurements of 231Pa and 230Th on GEOTRACES cruises shed new light on the chemical behaviour and controls on these isotopes in seawater and provided evidence to assess the validity of the models underlying the use of sedimentary 231Pa∕230Th as a proxy for deep water circulation. These measurements have indicated that there is considerably more net advection of 231Pa than 230Th out of the North Atlantic (Deng et al., 2014), supporting the basin-scale advection model for 231Pa∕230Th. But these measurements also have suggested that there is no simple relationship between increasing 231Pa∕230Th and age of water, as would be expected for the water mass evolution model (e.g. Deng et al., 2014). Studies using 2-D and 3-D ocean models (e.g. Marchal et al., 2010; Siddall et al., 2007) have also supported the use of sedimentary 231Pa∕230Th to constrain deep water circulation at a basin scale and suggested that the relationship between 231Pa∕230Th and water mass age is more complex than assumed in earlier studies (e.g. Luo et al., 2010).
Observations and model studies of 231Pa and 230Th have also suggested that other controls complicate 231Pa∕230Th as a dynamic tracer of deep water circulation, such as the effect of boundary scavenging at seafloor and ocean margins (e.g. Anderson et al., 1994; Deng et al., 2014; Rempfer et al., 2017) and the influence of particle flux and composition (e.g. Chase et al., 2002; Hayes et al., 2014; Siddall et al., 2005).
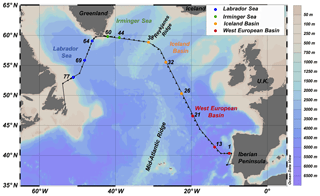
Figure 1Map showing GEOVIDE cruise track (black line) and station locations (black dots). Colour bars indicate water depth. Sampling locations for water-column 231Pa and 230Th in this study are shown by coloured dots, with colours representing the oceanic regions they are located in.
To fully assess the behaviour of 231Pa∕230Th, and its potential as a dynamic tracer of deep water circulation, knowledge of the concentrations and variations of these isotopes as deep waters form and enter the deep Atlantic is required. Some measurements have placed initial constraints on 231Pa and 230Th values in young North Atlantic deep waters (e.g. Moran et al., 1997, 2002; Rutgers van der Loeff and Berger, 1993), but there has not yet been a systematic study of the composition of waters in the far North Atlantic. The GEOVIDE cruise allowed waters to be collected for such a study, along a line where significant other data are available, from both that cruise and from previous occupations of OVIDE. GEOVIDE provided an ideal opportunity to understand 231Pa and 230Th at the start of the ocean meridional overturning circulation, and to assess the hypotheses underlying the use of 231Pa∕230Th as a palaeo-proxy for the rate of deep water circulation.
Seawater samples were collected during the GEOVIDE cruise aboard the R/V Pourquoi Pas? from 15 May to 30 June 2014 as part of GEOTRACES Section GA01. The cruise sampled four regions in the North Atlantic between 40 and 60∘ N: the Labrador Sea (LA Sea), Irminger Sea (IR Sea), Iceland Basin (IC Basin), and West European Basin (WE Basin) (Fig. 1).
Full-depth water-column 231Pa and 230Th for this study were collected from 11 stations (Fig. 1). Sampling followed the procedure suggested by GEOTRACES intercalibration work (Anderson et al., 2012). Briefly, seawater samples of 5 L were directly filtered from Niskin bottles mounted on the stainless steel CTD rosette through AcroPak™ capsules with Supor® membrane (0.45 µm pore size). Filtered seawater samples were collected into acid-cleaned HDPE plastic bottles and sealed with a screw cap and Parafilm to reduce evaporation and contamination. Samples were then double bagged for storage in boxes for transport back to the shore-based lab for analysis.
Once returned to the laboratory in Oxford, samples were weighed and then acidified with quartz-distilled concentrated HCl to pH ∼1.7, shaken, and left for at least 4 days to ensure that Pa and Th was desorbed from the walls of the bottle. A mixed 229Th–236U spike and a 233Pa spike were then added to each sample to allow measurement of Th, U (for another study), and Pa by isotope-dilution multi-collector inductively coupled plasma-mass spectrometry (MC-ICP-MS). The 233Pa spike was freshly made by milking from 237Np (following Regelous et al., 2004) and calibrated against a known 236U solution after the complete decay of 233Pa to 233U, i.e. four to five half-lives of 233Pa ( days; Usman and MacMahon, 2000) after spike production (Robinson et al., 2004); 50 mg of pure Fe as a chloride solution was also added to each water sample. Samples were left overnight to allow for spike equilibrium, after which the pH was raised to ∼8.5 using distilled NH4OH to co-precipitate the actinides with insoluble Fe-oxyhydroxides. At least 48 h were allowed for scavenging of the actinides onto Fe-oxyhydroxides. The precipitate was centrifuged and rinsed, and Th, Pa, and U were separated using anion exchange chromatography following Thomas et al. (2006).
After chemical separation, Pa and Th were measured on a Nu instrument (MC-ICP-MS) at the University of Oxford. Mass discrimination and ion-counter gain were assessed with the measurement of a U standard, CRM-145 U, before each sample measurement. The use of a U standard for this purpose minimizes memory problems that might be caused by use of a Th or Pa standard (Thomas et al., 2006). Measurements were also made 0.5 mass units either side of masses of interest to allow accurate correction for the effect of abundance sensitivity on small 231Pa and 230Th beams, and a correction for a small 232ThH interference on the 233Pa beam is made from assessment of the hydride formation rate on a 232Th standard. Concentrations of 231Pa and 230Th together with 232Th were obtained from the precise MC-ICP-MS measurement of 231Pa∕233Pa, 230Th∕229Th, and 232Th∕229Th ratios together with well-calibrated concentrations of 233Pa and 229Th–236U spikes.
Chemistry blanks were assessed by conducting the complete chemical procedure on ∼100 ml of Milli-Q water with each batch of samples. Based on six blank measurements, the average blanks for dissolved 231Pa, 230Th, and 232Th are 0.21±0.14 fg, 1.59±0.60 fg and 5.13±1.47 pg, respectively (uncertainties are 2 standard errors). Blank contributions account for 2 %–22 %, 2 %–26 %, and 0.2 %–16 % of the dissolved 231Pa, 230Th, and 232Th, respectively (with the higher values being for surface samples due to their low concentrations).
Measured 230Th and 231Pa concentrations were corrected for blanks, ingrowth from U in seawater since the time of sample collection, and detrital U-supported 230Th and 231Pa concentrations. Measured and corrected concentrations of 230Th, 231Pa, and 232Th, along with details of corrections, are provided in the Supplement Sect. S1. Although analysis was conducted in terms of fg kg−1, results are converted to the SI units adopted by GEOTRACES data product, i.e. µBq kg−1 for 230Th and 231Pa and pmol kg−1 for 232Th. This conversion uses half-lives for 231Pa, 230Th, and 232Th of 32 760, 75 584, and 1.405×1010 years, respectively (Cheng et al., 2013; Holden, 1990; Robert et al., 1969). Uncertainties were propagated, including the contribution from sample weighing, spike calibration, impurities in the spikes, blank corrections, and mass spectrometric measurement, and are reported as 2 standard errors (2 SE). Average total uncertainties for 231Pa, 230Th, and 232Th are ±0.17 µBq kg−1, ±0.17 µBq kg−1, and ±0.0032 pmol kg−1, respectively. Vertical profiles showing the results of corrected 230Th and 231Pa concentrations in the water column are plotted by region in Fig. 2.
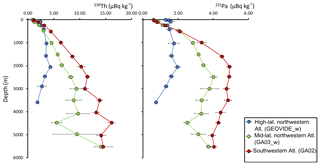
Figure 3Vertical profiles of 230Th and 231Pa from high-latitude northwestern Atlantic (west section of GEOVIDE), mid-latitude northwestern Atlantic (GA03_w), and southwestern Atlantic (GA02). Data from all stations were sorted by water depth, and averages for depth and 230Th and 231Pa concentrations were taken for surface, 25, 100, 250, and 500 m, and every 500 m interval below. Error bars on 230Th and 231Pa concentration averages reflect standard deviation of the mean of measurements.
230Th concentrations in the water column range between 0.06 and 12.01 µBq kg−1, and initially generally increase with water depth from surface to deep water. Towards the seafloor, 6 of the 11 stations show a prominent decrease in 230Th, with this feature most pronounced in the LA and IR seas.
231Pa concentrations in the water column range between 0.37 and 4.80 µBq kg−1 and also increase with water depth, but less rapidly than 230Th. 231Pa profiles also often exhibit a decrease near the seafloor at stations showing a 230Th decrease. Station 38 at the Reykjanes Ridge distinguishes itself from other 231Pa profiles, in that an increase in 231Pa concentrations from low concentrations at 1000 m is observed, continuing towards the bottom.
Observed 230Th and 231Pa values at GEOVIDE are lower than those observed in intercalibrated GEOTRACES data from further south in the Atlantic. Figure 3 compares average depth profiles for 230Th and 231Pa in the western Atlantic, covering the high-latitude northwestern Atlantic (from GEOVIDE, west of the Mid-Atlantic Ridge), mid-latitude northwestern Atlantic (GEOTRACES section GA03_w, Hayes et al., 2015), and southwestern Atlantic (GEOTRACES section GA02, Deng et al., 2014). A southward increase in both 230Th and 231Pa concentrations is observed below 1000 m.
Early studies of water-column 230Th and 231Pa reported a linear increase in both nuclides with water depth (e.g. Anderson et al., 1983b; Nozaki et al., 1981), and introduced a reversible scavenging model with the exchange of both nuclides between their dissolved and particulate phases. Later studies observed a deviation of 230Th and 231Pa profiles from this reversible scavenging model, with the expected increase with depth often inverting near the seafloor (e.g. Anderson et al., 1983a; Bacon and Anderson, 1982). This feature has been further investigated in more recent studies. Rutgers van der Loeff and Berger (1993) observed that 230Th concentrations decrease in the bottom water in the South Atlantic south of the Antarctic Polar Front and interpreted this as the influence of relatively young bottom water in the region. Okubo et al. (2012) also found decreasing 230Th values near the seafloor in the North Pacific and, in the absence of ventilation in the area, interpreted these as being due to bottom scavenging. Deng et al. (2014) observed low concentrations of both 230Th and 231Pa in near-bottom water coinciding with the presence of the nepheloid layer, and interpreted the low values as a result of enhanced scavenging by resuspended particles in the nepheloid layer.
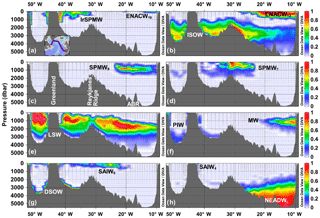
Figure 4Results of extended optimum multi-parameter (eOMP) analysis for the GEOVIDE section (García-Ibáñez et al., 2018). Colours reflect the fraction of water at each location assigned to the water mass shown in that panel: ENACW16 and ENACW12 represent the East North Atlantic Central Water of 16 and 12 ∘C; SPMW8, SPMW7, and IrSPMW represent the Subpolar Mode Water of 8 ∘C, 7 ∘C, and of the Irminger Sea; SAIW6 and SAIW4 represent Subarctic Intermediate Water of 6 and 4 ∘C; MW represents Mediterranean Water; PIW represents Polar Intermediate Water; ISOW represents Iceland–Scotland Overflow Water; LSW represents Labrador Sea Water; DSOW represents Denmark Strait Overflow Water; NEADWL represents Lower North East Atlantic Deep Water; and ABR represents the Azores–Biscay Rise.
In this study, recently ventilated overflow waters are sampled at depth, particularly in the Labrador and Irminger seas. Low values of 230Th and 231Pa near the seafloor might be expected to relate to these young waters, but the effects of scavenging must also be considered.
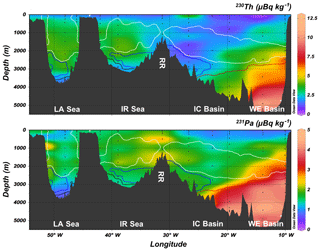
Figure 5Distribution of 230Th and 231Pa along the GEOVIDE section. Water masses were indicated by contours (black: DSOW; blue: ISOW; white: LSW; red: NEADW) based on 50 % level percentage composition of source water types from eOMP analysis.
4.1 Water mass distribution and influence
The presence of multiple water masses sampled by the GEOVIDE Section allows the influence of water mass (and age) on 230Th and 231Pa to be assessed. Extended optimum multi-parameter (eOMP) analysis (García-Ibáñez et al., 2018) for the GEOVIDE section maps the presence of 10 water mass end-members in the section (Fig. 4), including three recently ventilated waters in the GEOVIDE section.
- i.
Labrador Sea Water (LSW) is formed by deep convection (Talley and McCartney, 1982). It is the dominant deep water along the section, extending from 1000 to 2500 m depth in the east and from surface to 3500 m in the west of the section.
- ii.
Iceland–Scotland Overflow Water (ISOW) is formed in the Norwegian Sea and subsequently entrains overlying warmer and saltier waters. This water mass initially flows along the eastern flank of the Reykjanes Ridge before spreading back northwards, after crossing the Charlie-Gibbs Fracture Zone, into the Irminger and Labrador seas (Dickson and Brown, 1994; Saunders, 2001). A pronounced layer of this water mass is observed immediately below the LSW and extends as deep as 4000 m west of 20∘ W.
- iii.
Denmark Strait Overflow Water (DSOW) is formed after the Nordic Sea deep waters overflow and entrain Atlantic waters (SPMW and LSW) (Yashayaev and Dickson, 2008) with dense Greenland shelf water cascading down to the DSOW layer in the Irminger Sea (Falina et al., 2012; Olsson et al., 2005; Tanhua et al., 2005). This water occupies the deepest part of the IR and LA seas.
In the east of the section, deep waters consist of the much older Lower North East Atlantic Deep Water (NEADWL), which is formed with a significant southern component from Antarctic Bottom Water. A number of other water masses are also observed at shallow depths, including Mediterranean Water and various mode waters.
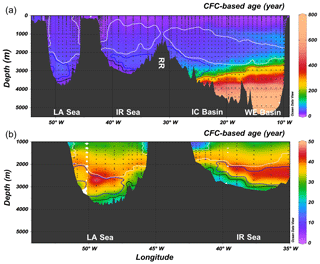
Figure 6Water mass age based on CFC data along the GEOVIDE section. (a) full water-column data for the entire section, showing waters from 10 to 800 years in age; (b) a rescaled version of panel (a) omitting the upper 1000 m and the older waters east of 35∘ W to show age variation in recently ventilated deep waters. Water masses were indicated by contours (black: DSOW; blue: ISOW; white: LSW; red: NEADW) based on 50 % level percentage composition of source water types from eOMP analysis.
Some control of water mass on 230Th and 231Pa concentrations is evident in nuclide section plots (Fig. 5), particularly relatively low 230Th and 231Pa concentrations in DSOW and high values in the old NEADW. In other places, the impact of water mass is less apparent. The challenge with these nuclides is that they are not conservative tracers of water mass, but evolve significantly during transport and water aging. In the GEOVIDE section, we can analyse this evolution, because the ages of the water masses can be assessed from CFC data.
CFC measurements are not available from the GEOVIDE cruise itself. De la Paz et al. (2017), however, measured CFC concentrations along the east of the same section (covering the WE Basin, IC Basin, and IR Sea) in 2012 (OVIDE/CATARINA cruise). This allowed the computing of CFC-based age with the transit time distribution (TTD) method. Using the water mass distribution along GEOVIDE given by García-Ibáñez et al. (2018) and the distribution for the same water masses in 2012 (García-Ibáñez et al., 2015), we derived CFC-based ages for GEOVIDE waters (Fig. 6; further details in Supplement Sect. S2). Uncertainties (1 standard error) associated with CFC-based age calculated with this approach range between 11 % and 40 %.
CFC-based water mass ages range from ≈10 years, observed in DSOW at the bottom of the LA Sea, to ≈800 years, observed for NEADW at the bottom of the WE Basin. Because this study focuses on understanding controls on 231Pa and 230Th in recently ventilated waters, we omit detailed consideration of the upper 1 km in subsequent discussion, and restrict our analysis to water sampled west of 35∘ W of the section, where young waters (<50 years) dominate. A rescaled version of the CFC age section indicates the variation in age of ventilated waters (Fig. 6b). DSOW, occupying the deepest LA and IR seas, is the youngest water mass in this region, with an average age of ∼19 years. ISOW and LSW are slightly older, with ages ranging from 26 to 45 years and 32 to 40 years, respectively.
4.2 Evolution of 230Th and 231Pa with water age
The presence of recently ventilated deep waters with constrained CFC ages allows analysis of the rates at which 230Th and 231Pa concentrations increase during transport and the rates of scavenging of these nuclides. To conduct this analysis, we define five components in the budget of 230Th and 231Pa.
- i.
Preformed component. The 230Th or 231Pa transported from the surface into the interior. For this analysis, in the absence of measurements for the exact location of deep water formation during winter convection, we assume the same preformed value for all water masses and set this as the average of concentrations measured in surface waters <100 m depth along GEOVIDE section. This gives preformed concentrations of 1.66 µBq kg−1 for 230Th and 1.31 µBq kg−1 for 231Pa. We recognize that true preformed values may differ from these values and between water masses, and discuss the implications of uncertainty in preformed values in the following section. Preformed 230Th and 231Pa will decrease due to radioactive decay during transport. Although we take this decay into account in the following analysis, it is insignificant given the ages of waters involved and the much longer half-lives of 230Th and 231Pa.
- ii.
Ingrown component. The ingrown 230Th or 231Pa from the radioactive decay of U since the water was last in contact with the surface. This component increases as the water mass ages. The concentration of this component in a water mass of age t can be calculated as
where 230ThIngrown and 231PaIngrown are the 230Th and 231Pa ingrown from their U parents, respectively; 234U and 235U are activities of 234U, and 235U in seawater (45 551.2 µBq kg−1 or 2801.4 dpm 1000 L−1, and 1823.8 µBq kg−1 or 112.2 dpm 1000 L−1, respectively, assuming a constant seawater 238U activity of 39 609.8 µBq kg−1 or 2436 dpm 1000 L−1 at salinity 35 psu, seawater 234U∕238U activity ratio of 1.15 and a natural 238U∕235U abundance ratio of 137.88); λ230 and λ231 are decay constants of 230Th and 231Pa ( and yr−1, respectively).
- iii.
Potential total component. The 230Th and 231Pa expected in the water due to the combination of preformed and ingrown components, if there is no removal by scavenging.
- iv.
Observed component. The 230Th and 231Pa observed in the water column, i.e. dissolved 230Th and 231Pa measured in this study (after correction for detritus and ingrowth from U since sample collection).
- v.
Scavenged component. The net 230Th or 231Pa removed from the water since it left the surface due to scavenging. For each depth, this component is the net of nuclide added from above by desorption from settling particles and the removal downwards by scavenging.
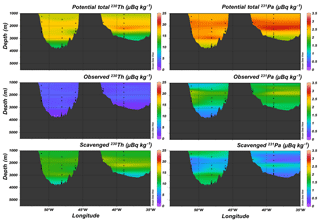
Figure 7Potential total, observed, and scavenged components of 230Th and 231Pa in waters >1000 m water depth and west of 35∘ W.
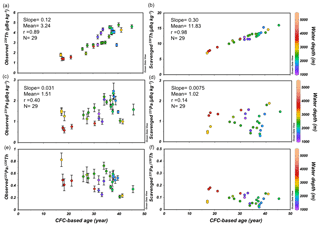
Figure 8Relationship between water mass age and the observed and scavenged components of 230Th, 231Pa, and 231Pa∕230Th (colour coded by water depth). Least-square-fitting statistics were also given, i.e. slope and correlation coefficient r of the least square line, mean value, and number of the data points. Note the increase in observed concentrations for both nuclides with age. Comparison of average values indicates that about three quarters of 230Th produced by decay is scavenged, compared with about half of the 231Pa.
These components are related to each other as follows:
The difference between the potential total and the observed concentration of 230Th (or 231Pa) therefore provides a measure of the amount of nuclide scavenged since the water left the surface (Fig. 7).
We examine the evolution of both the observed and scavenged components of 230Th and 231Pa with water mass age (Fig. 8). Both 230Th and 231Pa show an increase in observed concentration with age of water, with the increase for 230Th much more regular than for 231Pa. This strong 230Th relationship, regardless of depth of the sample (Fig. 8a), indicates a primary control of water mass age on the increase in 230Th in these younger waters.
For 230Th, the rate of increase with age (i.e. slope in Fig. 8a) indicates that about one quarter of the 230Th formed from U decay remains in the water, with the other three quarters being removed by scavenging. This ratio is consistent with the average 230Th for these waters, which requires about 3 times more 230Th than remains in water to be removed by scavenging (Fig. 8a, b). The scatter between 231Pa and age (Fig. 8c) precludes the use of the slope to assess the relative proportion of scavenged 231Pa, but the average values (Fig. 8c, d) indicate that about half of the 231Pa remains in the water, while the other half is removed by scavenging. The relative behaviour of 230Th and 231Pa is consistent with previous expectations, with a higher fraction of scavenging for 230Th than 231Pa.
The hypothesis that 231Pa∕230Th ratios increase monotonically as water mass ages forms the foundation of the water mass evolution model for interpretation of sedimentary 231Pa∕230Th in terms of the rate of deep water circulation. For these young waters, however, there is neither a clear relationship between observed 231Pa∕230Th and age (Fig. 8e), nor between the 231Pa∕230Th value scavenged to the sediment and age (Fig. 8f), calling the water mass evolution model into question.
4.3 The importance of preformed 230Th and 231Pa in young waters
To assess the controls on 230Th, 231Pa, and particularly the resulting 231Pa∕230Th ratio, we apply a simple scavenging-mixing model following Moran et al. (1997). This model was first created to assess the evolution of 230Th in a 1-D water column as it ages following ventilation. Here we adopt it by modelling the nuclide evolution with age for each depth and by also modelling 231Pa. This assumes that waters have remained at the same depth since ventilation, which, though not correct in detail, still allows the model to provide insights about controls on these nuclides.
Following Moran et al. (1997), dissolved concentration of 230Th and 231Pa is given by
where cd is the dissolved concentration of the nuclide; P is the production rate of 230Th and 231Pa, 0.42 µBq kg−1 yr−1 ( dpm 1000 L−1 yr−1) and 0.039 µBq kg−1 yr−1 ( dpm 1000 L−1 yr−1), respectively; Kd is the distribution coefficient of the nuclide; λ is the decay constant of the nuclide; Cpre,t is the preformed total concentration of 230Th (or 231Pa); SPM is the suspended particle concentration; S is the particle settling speed, which represents the net effect of particle sinking, disaggregation, and aggregation; τw is water mass age; and z is the water depth.
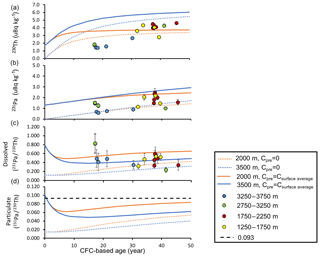
Figure 9Results from a scavenging-mixing model of 230Th, 231Pa, dissolved 231Pa∕230Th, and particulate 231Pa∕230Th compared to observations. Preformed concentration (Cpre) were set at 0 (dashed line) and at the average surface concentration (Csurface average) from GEOVIDE section (solid line), i.e. 230Th = 1.66 µBq kg−1 and 231Pa = 1.31 µBq kg−1. A version of this figure extending to older waters is available in Fig. S2.
The model requires values for four parameters: particle settling speed (S), suspended particle concentration (SPM), and distribution coefficients for 230Th () and 231Pa (). We select these parameters to give a good fit to the 230Th and 231Pa observations at an open ocean station, Station 13, on the east of the section (i.e. a station sampling older waters, which are close to equilibrium) and use these values to interpret the younger waters to the west. Best fits to Station 13 suggested S=800 m yr−1, SPM = 25 µg L−1, mL g−1, and mL g−1 (the first three of these are close to those of Moran et al., 1997). A fuller description of the model is given in Supplement Sect. S3.
We show two sets of output from the model, one with a preformed component (Cpre) equal to the nuclide concentrations observed in the upper 100 m of the GEOVIDE section (as in Sect. 4.2 above), and one with the preformed component set to zero for both nuclides. For both cases, the modelled evolution of nuclide concentrations with age between 0 and 50 years at 2000 and 3500 m water depths is plotted in Fig. 9, and compared to data. As expected, modelled 230Th and 231Pa concentrations increase with age, with deeper waters having higher concentrations and 230Th increasing more rapidly initially (Fig. 9), but the preformed concentration is seen to be important in setting total nuclide concentration for several decades after ventilation. The fit of the model to observations in young waters from GEOVIDE is improved in the model run with zero preformed nuclide, particularly for 230Th. This is surprising, given that surface-water 230Th, and 231Pa values, are generally non-zero, and are typically close to the values observed in the GEOVIDE surface waters. For 230Th in young deep waters, even the model with zero preformed nuclide overestimates the observed value, possibly indicating additional scavenging from these waters close to the seafloor, or as a result of differing biological productivity and particle fluxes between stations.
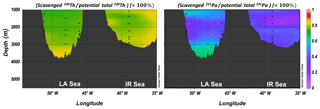
Figure 10Ratio of scavenged component to potential total components for 230Th and 231Pa, providing an assessment of the relative importance of scavenging for the two nuclides and of the location of scavenging.
The most striking effect of changing the assumed preformed values in the model is on 231Pa∕230Th (Fig. 9c). When preformed values are set at zero, 231Pa∕230Th ratios always increase with water age, but when set at the average surface value from GEOVIDE, 231Pa∕230Th ratios initially decrease before increasing. The impact of preformed concentrations has a long-lasting impact on water-column and scavenged 231Pa∕230Th, lasting for hundreds of years following ventilation (Fig. S2c, d in the Supplement). This indicates that knowledge of the nuclide concentration at the site of deep water formation is critical to understanding the early evolution of 231Pa∕230Th in waters and their underlying sediments.
4.4 Scavenging of 230Th and 231Pa
Knowledge of the CFC ages of the waters analysed on the GEOVIDE cruise allows an assessment of the scavenging rates of 230Th and 231Pa. To do so, we compare the scavenged component to the potential total component (as defined in Sect. 4.2). The percentage of the scavenged component relative to the potential total component is higher for 230Th, at an average of 80 %, than for 231Pa, at an average of 40 % (Fig. 10), which is consistent with the relatively higher particle reactivity of 230Th. For both nuclides, there is a higher fraction of scavenging in samples from near the seafloor, particularly those from DSOW in the deepest LA Sea. Bottom scavenging has been indicated in previous studies (e.g. Bacon and Anderson, 1982; Deng et al., 2014; Okubo et al., 2012), but this study indicates that this enhanced nuclide scavenging occurs even in the very young overflow waters at the start of the meridional circulation.
4.5 Uncertainty analysis of the assessment of the scavenging of 230Th and 231Pa
Uncertainty in both CFC-based ages and in preformed values of 230Th and 231Pa contributes to uncertainty when calculating the scavenging of 230Th and 231Pa. Uncertainties associated with CFC-based age range between 11 % and 40 % (1 standard error). These uncertainties lead to an average uncertainty of 23 % and 13 % in potential total 230Th and 231Pa, respectively, corresponding to an average uncertainty of 30 % in the scavenged component of 230Th and 40 % in the scavenged component of 231Pa.
A 2-fold increase in preformed values results in an increase by a factor of 1.2 and 1.6 in the total potential 230Th and 231Pa, respectively, leading to an increase by a factor of 1.2 in the scavenged component of 230Th and of 2.6 in the scavenged component of 231Pa. The impact of preformed uncertainty is less significant when comparing the scavenging to the potential total components. The ratio of scavenged component to potential total component increases by factors of 1.1 and 1.4 for 230Th and 231Pa, respectively. This sensitivity analysis indicates that a better knowledge of preformed values will benefit the assessment of the scavenging of both nuclides.
4.6 Meridional transport of 230Th and 231Pa in the North Atlantic
Previous calculations have indicated removal of 230Th and 231Pa from the North Atlantic by meridional transport southward. Deng et al. (2014) calculated net southward transport of 6 % of the 230Th and 33 % of 231Pa, relative to production of these nuclides in the water column. That calculation, however, did not provide a complete budget for 230Th and 231Pa for the North Atlantic, because observations at the time did not constrain input of these nuclides from the north. Data in this study allow this calculation, and therefore a more complete budget for the modern North Atlantic.
García-Ibáñez et al. (2018) calculated volume transports for the Portugal–Greenland section of the GEOVIDE section by combining the water mass fractions from eOMP analysis with the absolute geostrophic velocity field calculated using inverse model constrained by Doppler current profiler velocity measurements (Zunino et al., 2017). They separated northward flowing upper limbs and southward flowing lower limbs of the Atlantic Meridional Overturning Circulation (AMOC) at isopycnal σ1 (potential density referenced to 1000 dbar) = 32.15 kg m−3, with and Sv flowing across the section above and below this value (positive value indicates northward transport). With average 230Th and 231Pa concentrations in the upper limb (σ1<32.15 kg m−3) of 1.60 and 1.32 µBq kg−1, respectively, northward transport of 230Th is 3.07×1010 and of 231Pa is 2.53×1010 µBq s−1. Average 230Th and 231Pa concentrations in the lower limb (σ1>32.15 kg m−3) are 3.44 and 2.07 µBq kg−1, respectively, indicating that transports of 230Th and 231Pa are and µBq s−1, respectively.
Net transport of 230Th and 231Pa across GEOVIDE is therefore to the south, and supplies 3.15×1010 µBq s−1 230Th and 1.21×1010 µBq s−1 231Pa to the North Atlantic (Fig. 11). This is a smaller net transport than further south in the Atlantic (Fig. 11), due to the lower 230Th and 231Pa concentrations in the water column close of the site of deep water formation.
The budget for these nuclides for the North Atlantic consists of the following: production in the water column, addition by advection from the north, loss by advection to the south, and removal to the sediment. The data from this study allow this budget to be fully assessed, and indicate that the flux to the sediment is equivalent to 96 % of the production of 230Th, and 74 % of the production for 231Pa (Table S4). For both nuclides, these fluxes are higher than in previous calculations (Deng et al., 2014), which ignored advective fluxes from the north. There is, however, still a significantly higher advective loss of 231Pa relative to 230Th. At a basin scale, therefore, 231Pa∕230Th in the sediment must be lower than the production ratio. This lower value is generated by the meridional transport of the North Atlantic and likely to be sensitive to changes in this transport.
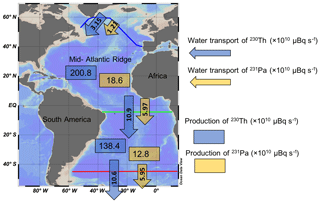
Figure 11Fluxes of 230Th (blue arrow) and 231Pa (yellow arrow) across the GEOVIDE section (blue solid line), 4.5∘ S (green solid line), and 45∘ S (red solid line). Also shown is production of 230Th (blue box) and 231Pa (yellow box) in the North Atlantic (between GEOVIDE section and 4.5∘ S) and in the South Atlantic (between 4.5 and 45∘ S), based on calculation in Deng et al. (2014). These fluxes indicate that 4 % of the 230Th and 26 % of the 231Pa produced in the North Atlantic is exported southward by ocean circulation.
Using the basin-scale advection model to interpret sedimentary 231Pa∕230Th to assess meridional transport, as initially proposed by Yu et al. (1996), is therefore still supported by a full modern North Atlantic budget for these nuclides.
Measurement of 230Th and 231Pa in waters from GEOVIDE shows some control of water mass on 230Th and 231Pa concentrations, particularly low concentrations in DSOW and high values in the old NEADW. There is, however, no close mapping of nuclide concentration to water mass.
With the availability of CFC-based ages in this section, the evolution of 230Th and 231Pa concentration with age is possible. A systematic increase in 230Th concentration is observed over the first 50 years following ventilation, and a similar though more scattered relationship is seen for 231Pa. There is no clear relationship between the 231Pa∕230Th ratio and age for these young waters. The long-term evolution of 231Pa∕230Th is found from a simple model to be highly dependent on the preformed concentrations for these nuclides. These results complicate the interpretation of sedimentary 231Pa∕230Th as a palaeo-proxy for deep water circulation based on the systematic evolution of water 231Pa∕230Th with age, and point to the importance of a better knowledge of preformed 230Th and 231Pa concentrations to improve interpretation. This analysis of the 230Th and 231Pa concentration relative to the age of the water not only demonstrates the influence of water mass aging on 231Pa and 230Th but also points to the influence of scavenging. Scavenged 230Th is much more extensive than 231Pa, as expected, and enhanced removal of both nuclides is seen immediately above the seafloor, particularly for young waters.
Calculation of the meridional transport of 230Th and 231Pa indicates a southward net transport of both nuclides across the GEOVIDE section. This advection is smaller than that further south in the Atlantic as a result of lower 230Th and 231Pa concentrations at GEOVIDE. Calculation of the flux across GEOVIDE allows a more complete budget for the North Atlantic to be constructed and demonstrates a significantly higher advective loss of 231Pa to the south, relative to 230Th, with 26 % of the 231Pa produced advected southward (relative to only 4 % for 230Th). This calculation supports the interpretation of sedimentary 231Pa∕230Th measurements as a proxy for overturning circulation, when based on the advective loss of 231Pa at a basin scale.
GEOVIDE 231Pa, 230Th and 232Th data are available at British Oceanographic Data Centre – Natural Environment Research Council, UK: https://doi.org/10.5285/7a150d33-956b-0fec-e053-6c86abc0b35c (Deng et al., 2018).
The supplement related to this article is available online at: https://doi.org/10.5194/bg-15-7299-2018-supplement.
GMH conceptualized and acquired funding for the research. MC collected seawater samples for 231Pa, 230Th, and 232Th at sea and provided expertise of other measurements on the cruise. FD conducted the chemical analysis and MC-ICP-MS measurement of 231Pa, 230Th, and 232Th. FFP and RS conducted the analysis, calculation, and interpretation of CFC ages. FD conducted the interpretation and analysis of 231Pa, 230Th, and 232Th data, with an extensive contribution from GMH. FD wrote the paper and prepared all figures, with GMH contributing extensively and contributions from the other co-authors.
The authors declare that they have no conflict of interest.
This article is part of the special issue “GEOVIDE, an international GEOTRACES study along the OVIDE section in the North Atlantic and in the Labrador Sea (GA01)”. It is not associated with a conference.
Géraldine Sarthou and Pascale Lherminier are thanked for leading the
GEOVIDE cruise, along with the captain, Gilles Ferrand, and crew of the R/V
Pourquoi Pas?. We would like to give a special thanks to
Pierre Branellec, Floriane Desprez de Gésincourt, Michel Hamon,
Catherine Kermabon, Philippe Le Bot, Stéphane Leizour,
Olivier Ménage, Fabien Pérault, and Emmanuel de Saint Léger for
their technical expertise and to Catherine Schmechtig for the GEOVIDE
database management. This work was supported by the French National Research
Agency (ANR-13-BS06-0014, ANR-12-PDOC-0025-01), the French National Centre
for Scientific Research (CNRS-LEFE-CYBER), the LabexMER (ANR-10-LABX-19), and
Ifremer. It was supported for the logistics by DT-INSU and GENAVIR. Thank you
also to Yi Tang, who helped with sampling during the cruise. We are grateful
to Mercedes de la Paz Arandiga, and Pascale Lherminier for providing valuable
input during analysis of water masses and ages, and to
Maribel García-Ibáñez for the early provision of the eOMP
analysis presented elsewhere in this volume. We also thank Yves Plancherel
and Jerry McManus for valuable insight during discussion of the results
presented. We thank Roger Francois, two anonymous reviewers, and the editor
Gilles Reverdin for their constructive comments on the paper.
Edited by: Gilles Reverdin
Reviewed by: Roger
Francois and two anonymous referees
Anderson, R. F., Bacon, M. P. and Brewer, P. G.: Removal of 230Th and 231Pa at ocean margins, Earth Planet. Sc. Lett., 66, 73–90, https://doi.org/10.1016/0012-821X(83)90127-9, 1983a.
Anderson, R. F., Bacon, M. P. and Brewer, P. G.: Removal of 230Th and 231Pa from the open ocean, Earth Planet. Sc. Lett., 62, 7–23, https://doi.org/10.1016/0012-821X(83)90067-5, 1983b.
Anderson, R. F., Fleisher, M. Q., Biscaye, P. E., Kumar, N., Dittrich, B., Kubik, P., and Suter, M.: Anomalous boundary scavenging in the Middle Atlantic Bight: evidence from 230Th, 231Pa, 10Be and 210Pb, Deep-Sea Res. Pt. II, 41, 537–561, https://doi.org/10.1016/0967-0645(94)90034-5, 1994.
Anderson, R. F., Fleisher, M. Q., Robinson, L. F., Edwards, R. L., Hoff, J. A., Moran, S. B., Rutgers van der Loeff, M. R., Thomas, A. L., Roy-Barman, M., and Francois, R.: GEOTRACES intercalibration of 230Th, 232Th, 231Pa, and prospects: For 10Be, Limnol. Oceanogr.-Meth., 10, 179–213, https://doi.org/10.4319/lom.2012.10.179, 2012.
Bacon, M. P. and Anderson, R. F.: Distribution of thorium isotopes between dissolved and particulate forms in the deep sea, J. Geophys. Res., 87, 2045, https://doi.org/10.1029/JC087iC03p02045, 1982.
Bradtmiller, L. I., McManus, J. F., and Robinson, L. F.: 231Pa∕230Th evidence for a weakened but persistent Atlantic meridional overturning circulation during Heinrich Stadial 1, Nat. Commun., 5, 5817, https://doi.org/10.1038/ncomms6817, 2014.
Chase, Z., Anderson, R. F., Fleisher, M. Q., and Kubik, P. W.: The influence of particle composition and particle flux on scavenging of Th, Pa and Be in the ocean, Earth Planet. Sc. Lett., 204, 215–229, doi10.1016/S0012-821X(02)00984-6, 2002.
Cheng, H., Edwards, L. R., Shen, C.-C., Polyak, V. J., Asmerom, Y., Woodhead, J., Hellstrom, J., Wang, Y., Kong, X., Spötl, C., Wang, X., and Calvin, A. E.: Improvements in 230Th dating, 230Th and 234U half-life values, and U–Th isotopic measurements by multi-collector inductively coupled plasma mass spectrometry, Earth Planet. Sc. Lett., 371, 82–91, https://doi.org/10.1016/j.epsl.2013.04.006, 2013.
de la Paz, M., García-Ibáñez, M. I., Steinfeldt, R., Ríos, A. F., and Pérez, F. F.: Ventilation versus biology: What is the controlling mechanism of nitrous oxide distribution in the North Atlantic?, Global Biogeochem. Cy., 31, 745–760, https://doi.org/10.1002/2016GB005507, 2017.
Deng, F., Thomas, A. L., Rijkenberg, M. J. A., and Henderson, G. M.: Controls on seawater 231Pa, 230Th and 232Th concentrations along the flow paths of deep waters in the Southwest Atlantic, Earth Planet. Sc. Lett., 390, 93–102, https://doi.org/10.1016/j.epsl.2013.12.038, 2014.
Deng, F., Henderson, G., Castrillejo, M., and Browning, T. J.: GEOTRACES GA01 (cruise GEOVIDE) 231Pa, 230Th and 232Th activities, British Oceanographic Data Centre – Natural Environment Research Council, UK, https://doi.org/10.5285/7a150d33-956b-0fec-e053-6c86abc0b35c, 2018.
Dickson, R. R. and Brown, J.: The production of North Atlantic Deep Water: Sources, rates, and pathways, J. Geophys. Res.-Oceans, 99, 12319–12341, https://doi.org/10.1029/94JC00530, 1994.
Falina, A., Sarafanov, A., Mercier, H., Lherminier, P., Sokov, A., and Daniault, N.: On the Cascading of Dense Shelf Waters in the Irminger Sea, J. Phys. Oceanogr., 42, 2254–2267, https://doi.org/10.1175/JPO-D-12-012.1, 2012.
García-Ibáñez, M. I., Pardo, P. C., Carracedo, L. I., Mercier, H., Lherminier, P., Ríos, A. F., and Pérez, F. F.: Structure, transports and transformations of the water masses in the Atlantic Subpolar Gyre, Prog. Oceanogr., 135, 18–36, https://doi.org/10.1016/j.pocean.2015.03.009, 2015.
García-Ibáñez, M. I., Pérez, F. F., Lherminier, P., Zunino, P., Mercier, H., and Tréguer, P.: Water mass distributions and transports for the 2014 GEOVIDE cruise in the North Atlantic, Biogeosciences, 15, 2075–2090, https://doi.org/10.5194/bg-15-2075-2018, 2018.
Gherardi, J.-M., Labeyrie, L., McManus, J. F., Francois, R., Skinner, L. C., and Cortijo, E.: Evidence from the Northeastern Atlantic basin for variability in the rate of the meridional overturning circulation through the last deglaciation, Earth Planet. Sc. Lett., 240, 710–723, https://doi.org/10.1016/j.epsl.2005.09.061, 2005.
Gherardi, J. M., Labeyrie, L., Nave, S., Francois, R., McManus, J. F., and Cortijo, E.: Glacial-interglacial circulation changes inferred from 231Pa∕230Th sedimentary record in the North Atlantic region, Paleoceanography, 24, PA2204, https://doi.org/10.1029/2008PA001696, 2009.
Hayes, C. T., Anderson, R. F., Fleisher, M. Q., Serno, S., Winckler, G., and Gersonde, R.: Biogeography in 231Pa∕230Th ratios and a balanced 231Pa budget for the Pacific Ocean, Earth Planet. Sc. Lett., 391, 307–318, https://doi.org/10.1016/j.epsl.2014.02.001, 2014.
Hayes, C. T., Anderson, R. F., Fleisher, M. Q., Huang, K. F., Robinson, L. F., Lu, Y., Cheng, H., Edwards, R. L., and Moran, S. B.: 230Th and 231Pa on GEOTRACES GA03, the U.S. GEOTRACES North Atlantic transect, and implications for modern and paleoceanographic chemical fluxes, Deep-Sea Res. Pt. II, 116, 29–41, https://doi.org/10.1016/j.dsr2.2014.07.007, 2015.
Henderson, G. M. and Anderson, R. F.: The U-series Toolbox for Paleoceanography, Rev. Mineral. Geochem., 52, 493–531, https://doi.org/10.2113/0520493, 2003.
Holden, N. E.: Total half-lives for selected nuclides, Pure Appl. Chem., 62, 941–958, https://doi.org/10.1351/pac199062050941, 1990.
Luo, Y., Francois, R., and Allen, S. E.: Sediment 231Pa∕230Th as a recorder of the rate of the Atlantic meridional overturning circulation: insights from a 2-D model, Ocean Sci., 6, 381–400, https://doi.org/10.5194/os-6-381-2010, 2010.
Marchal, O., François, R., Stocker, T. F., and Joos, F.: Ocean thermohaline circulation and sedimentary 231Pa∕230Th ratio, Paleoceanography, 15, 625–641, https://doi.org/10.1029/2000PA000496, 2010.
McManus, J. F., Francois, R., Gherardi, J.-M., Keigwin, L. D., and Brown-Leger, S.: Collapse and rapid resumption of Atlantic meridional circulation linked to deglacial climate changes, Nature, 428, 834–837, https://doi.org/10.1038/nature02494, 2004.
Moran, S. B., Charette, M. A., Hoff, J. A., and Edwards, R. L.: Distribution of 230Th in the Labrador Sea and its relation to ventilation, Earth Planet. Sc. Lett., 150, 151–160, 1997.
Moran, S. B., Shen, C., Edmonds, H. N., Weinstein, S. E., Smith, J. N., and Edwards, R. L.: Dissolved and particulate 231Pa and 230Th in the Atlantic Ocean?: constraints on intermediate/deep water age, boundary scavenging, and 231Pa∕230Th fractionation, Earth Planet. Sc. Lett., 203, 999–1014, 2002.
Negre, C., Zahn, R., Thomas, A. L., Masqué, P., Henderson, G. M., Martínez-Méndez, G., Hall, I. R., and Mas, J. L.: Reversed flow of Atlantic deep water during the Last Glacial Maximum, Nature, 468, 84–88, https://doi.org/10.1038/nature09508, 2010.
Nozaki, Y., Horibe, Y., and Tsubota, H.: The water column distributions of thorium isotopes in the western North Pacific, Earth Planet. Sc. Lett., 54, 203–216, https://doi.org/10.1016/0012-821X(81)90004-2, 1981.
Okubo, A., Obata, H., Gamo, T., and Yamada, M.: 230Th and 232Th distributions in mid-latitudes of the North Pacific Ocean: Effect of bottom scavenging, Earth Planet. Sc. Lett., 339–340, 139–150, https://doi.org/10.1016/j.epsl.2012.05.012, 2012.
Olsson, K. A., Jeansson, E., Anderson, L. G., Hansen, B., Eldevik, T., Kristiansen, R., Messias, M.-J., Johannessen, T., and Watson, A. J.: Intermediate water from the Greenland Sea in the Faroe Bank Channel: spreading of released sulphur hexafluoride, Deep-Sea Res. Pt. I, 52, 279–294, https://doi.org/10.1016/j.dsr.2004.09.009, 2005.
Regelous, M., Turner, S. P., Elliott, T. R., Rostami, K., and Hawkesworth, C. J.: Measurement of Femtogram Quantities of Protactinium in Silicate Rock Samples by Multicollector Inductively Coupled Plasma Mass Spectrometry, Anal. Chem., 76, 3584–3589, https://doi.org/10.1021/ac030374l, 2004.
Rempfer, J., Stocker, T. F., Joos, F., Lippold, J., and Jaccard, S. L.: New insights into cycling of 231Pa and 230Th in the Atlantic Ocean, Earth Planet. Sc. Lett., 468, 27–37, https://doi.org/10.1016/j.epsl.2017.03.027, 2017.
Robert, J., Miranda, C. F., and Muxart, R.: Mesure de la période du protactinium 231 par microcalorimétrie, Radiochim. Acta, 11, 104–108, https://doi.org/10.1524/ract.1969.11.2.104, 1969.
Roberts, N. L., McManus, J. F., Piotrowski, A. M., and McCave, I. N.: Advection and scavenging controls of Pa/Th in the northern NE Atlantic, Paleoceanography, 29, 668–679, https://doi.org/10.1002/2014PA002633, 2014.
Robinson, L. F., Belshaw, N. S., and Henderson, G. M.: U and Th concentrations and isotope ratios in modern carbonates and waters from the Bahamas, Geochim. Cosmochim. Ac., 68, 1777–1789, https://doi.org/10.1016/j.gca.2003.10.005, 2004.
Rutgers van der Loeff, M. M. and Berger, G. W.: Scavenging of 230Th and 231Pa near the antarctic polar front in the South Atlantic, Deep-Sea Res. Pt. I, 40, 339–357, https://doi.org/10.1016/0967-0637(93)90007-P, 1993.
Saunders, P, M.: The dense northern overflows, in: Ocean Circulation and Climate, vol. 77, chaap. 5.6, edited by: Siedler, G., Church, J., and Gould, J., Academic Press, 401–417, 2001.
Siddall, M., Henderson, G. M., Edwards, N. R., Frank, M., Müller, S. A., Stocker, T. F., and Joos, F.: 231Pa∕230Th fractionation by ocean transport, biogenic particle flux and particle type, Earth Planet. Sc. Lett., 237, 135–155, https://doi.org/10.1016/j.epsl.2005.05.031, 2005.
Siddall, M., Stocker, T. F., Henderson, G. M., Joos, F., Frank, M., Edwards, N. R., Ritz, S. P., and Müller, S. A.: Modeling the relationship between 231Pa∕230Th distribution in North Atlantic sediment and Atlantic meridional overturning circulation, Paleoceanography, 22, PA2214, https://doi.org/10.1029/2006PA001358, 2007.
Talley, L. D. and McCartney, M. S.: Distribution and Circulation of Labrador Sea Water, J. Phys. Oceanogr., 12, 1189–1205, https://doi.org/10.1175/1520-0485(1982)012<1189:DACOLS>2.0.CO;2, 1982.
Tanhua, T., Olsson, K. A., and Jeansson, E.: Formation of Denmark Strait overflow water and its hydro-chemical composition, J. Marine Syst., 57, 264–288, https://doi.org/10.1016/j.jmarsys.2005.05.003, 2005.
Thomas, A. L., Henderson, G. M., and Robinson, L. F.: Interpretation of the 231Pa/ 230Th paleocirculation proxy: New water-column measurements from the southwest Indian Ocean, Earth Planet. Sc. Lett., 241, 493–504, https://doi.org/10.1016/j.epsl.2005.11.031, 2006.
Thomas, A. L., Henderson, G. M., and McCave, I. N.: Constant bottom water flow into the Indian Ocean for the past 140 ka indicated by sediment 231Pa∕230Th ratios, Paleoceanography, 22, PA4210, https://doi.org/10.1029/2007PA001415, 2007.
Usman, K. and MacMahon, T. D.: Determination of the half-life of 233Pa, Appl. Radiat. Isot., 52, 585–589, https://doi.org/10.1016/S0969-8043(99)00214-6, 2000.
Yashayaev, I. and Dickson, B.: Transformation and Fate of Overflows in the Northern North Atlantic BT – Arctic–Subarctic Ocean Fluxes: Defining the Role of the Northern Seas in Climate, edited by: Dickson, R. R., Meincke, J., and Rhines, P., Springer Netherlands, Dordrecht, the Netherlands, 505–526, 2008.
Yu, E.-F., Francois, R., and Bacon, M. P.: Similar rates of modern and last-glacial ocean thermohaline circulation inferred from radiochemical data, Nature, 379, 689–694, https://doi.org/10.1038/379689a0, 1996.
Zunino, P., Lherminier, P., Mercier, H., Daniault, N., García-Ibáñez, M. I., and Pérez, F. F.: The GEOVIDE cruise in May–June 2014 reveals an intense Meridional Overturning Circulation over a cold and fresh subpolar North Atlantic, Biogeosciences, 14, 5323–5342, https://doi.org/10.5194/bg-14-5323-2017, 2017.