the Creative Commons Attribution 4.0 License.
the Creative Commons Attribution 4.0 License.
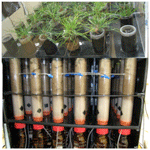
Biological enhancement of mineral weathering by Pinus sylvestris seedlings – effects of plants, ectomycorrhizal fungi, and elevated CO2
Nicholas P. Rosenstock
Patrick A. W. van Hees
Petra M. A. Fransson
Roger D. Finlay
Anna Rosling
Better understanding and quantifying the relative influence of plants, associated mycorrhizal fungi, and abiotic factors such as elevated CO2 on biotic weathering is essential to constraining weathering estimates. We employed a column microcosm system to examine the effects of elevated CO2 and Pinus sylvestris seedlings, with or without the ectomycorrhizal fungi Piloderma fallax and Suillus variegatus, on rhizosphere soil solution concentrations of low-molecular-weight organic acids (LMWOAs) and on the weathering of primary minerals. Seedlings significantly increased mineral weathering, as estimated from elemental budgets of Ca, K, Mg, and Si. Elevated CO2 increased plant growth and LMWOA concentrations but had no effect on weathering. Colonization by ectomycorrhizal fungi, particularly P. fallax, showed some tendency to increase weathering. LMWOA concentrations correlated with seedling biomass across both CO2 and mycorrhizal treatments but not with total weathering. We conclude that nutrient uptake, which reduces transport limitation to weathering, is the primary mechanism by which plants enhanced weathering in this system. While the experimental system used departs from conditions in forest soils in a number of ways, these results are in line with weathering studies performed at the ecosystem, macrocosm, and microcosm scale, indicating that nutrient uptake by plants and microbes is an important biological mechanism by which mineral weathering is enhanced.
- Article
(1138 KB) - Full-text XML
- BibTeX
- EndNote
Accurate estimates of net primary productivity of forests are critically important to global carbon models, and forest productivity is predicted to increase due to elevated CO2 (Lindner et al., 2010; Ainsworth and Long, 2005). The extent of this response to elevated CO2 levels is largely dependent on the ability of forest trees to meet their increased carbon availability and water use efficiency with increased nutrient uptake (Pinkard et al., 2010; Norby et al., 1999). As the effects of anthropogenic nitrogen deposition continue to accumulate, large areas of forest are limited by mineral-derived nutrients (Naples and Fisk, 2010; Baribault et al., 2010; Jonard et al., 2015). The ability of forest ecosystems to replenish pools of base cations and phosphorus through dissolution of minerals may thus become increasingly important. In coniferous trees, elevated CO2 has been shown to increase the root : shoot biomass ratio (Alberton et al., 2007; Janssens et al., 2005) and allocation to mycorrhizal symbionts (Fransson et al., 2010; Compant et al., 2010) indicating an increased exploration of the soil environment to obtain nutrients. Studies in Swedish (Almeida et al., 2018) and Czech (Rosenstock et al., 2016) Norway spruce forests have observed increased belowground allocation to fine roots and ectomycorrhizal hyphal growth and greater preference for growth around apatite (a mineral source of Ca and P) mineral grains under phosphorus-limiting conditions.
Most forest trees of the temperate and boreal biomes are dependent on ectomycorrhizal fungi for their survival (Smith and Read, 2008). Ectomycorrhizal fungi (EMF) are mutualistic symbionts that form intimate associations with the fine roots of trees and some woody shrubs, and they colonize mineral and organic substrates in the soil with their extraradical mycelia. Increased nutrient uptake is generally considered to be the most beneficial effect of EMF on forest trees (Smith and Read, 2008), and the host plant transfers significant amounts of photosynthetically fixed carbon to their associated EMF. A review by Hobbie (2006) suggests that an average of approximately 15 % of total fixed carbon is allocated to EMF symbionts, but some studies show that more than 60 % of recent carbon assimilation (Rosling et al., 2004) and net primary production (Godbold et al., 2006) can be allocated to EMF. As a result of their central role in tree nutrition and their ability to stimulate mineral weathering (Finlay et al., 2009, 2019), EMF are acknowledged to be instrumental in forest biogeochemistry. Many studies have found that EMF influence weathering, and the proposed mechanisms include acidification (Balogh-Brunstad et al., 2008; Rosling et al., 2004), nutrient uptake (Wallander, 2000; van Hees et al., 2004), production of siderophores (Ochs et al., 1993; Watteau and Berthelin, 1994), production of low-molecular-weight organic acids (Paris et al., 1996, van Schöll et al., 2006; Schmalenberger et al., 2015), and biomechanical forcing (Bonneville et al., 2009, 2016). Despite numerous potential mechanisms by which EMF may directly influence weathering rates, little is known about their quantitative importance for the process of mineral weathering in soil.
In this experiment we use a column microcosm system to quantify nutrient uptake by Scots pine seedlings and determine the effect of their associated EMF, elevated CO2, and soil solution low-molecular-weight organic acid (LMWOA) concentrations on system nutrient budgets. The experiment was designed to test the hypothesis that elevated CO2 would drive plants to allocate more carbon below ground, to roots, EMF, and exudation, thereby increasing mineral weathering through quantitative and qualitative effects on nutrient release and acquisition from the mineral substrate. We specifically asked the following questions:
-
What is the contribution of plants, in comparison to EMF, to mineral weathering?
-
Will elevated CO2 increase mineral weathering?
-
How are LMWOA concentrations affected by mycorrhizal colonization and elevated CO2?
-
If observed, what are the potential mechanisms of biological enhancement of weathering?
2.1 Experimental overview
We used column microcosm systems filled with a mineral mix mimicking a podzol E horizon. Treatments were factorial with ±CO2, ±seedling, and ±EMF (two species). Non-mycorrhizal and non-planted treatments were performed in four replicates and EMF treatments in five; in total there were 36 columns, 28 of which were planted with 6-month-old seedlings. A bacterial suspension was added to all the columns to enable bacterial–mycorrhizal interactions. Following planting, the experiment was run for 9 months and watered with nutrient solution. Organic acid concentrations were measured in rhizosphere soil solution, and elemental concentrations and pH were measured in column leachate collected during the experiment. Upon harvest, the ion-exchangeable element pool (Ec) of the mineral mix after 9 months of incubation as well as mineral mix set aside at planting before the 9-month incubation was quantified in addition to the dry weight and elemental contents of seedlings, and the chitin contents of the roots and mineral mix. Amounts of elements added with nutrient solution taken up by plants and leached though the columns were used to construct whole column elemental budgets for silica, calcium, potassium, and magnesium.
2.2 Plant and mycorrhizal pre-culture and bacterial inoculation
Ectomycorrhizal and non-mycorrhizal Pinus sylvestris L. seedlings were prepared in petri dishes containing peat / vermiculite / modified Melin-Norkrans media (, ) as detailed by Fransson and Johansson (2010). The ectomycorrhizal fungal species Suillus variegatus (Sw. : Fr.) O. Kuntze (isolate code UP597, GenBank accession no. EF493256) and Piloderma fallax (Liberta) Stalpers (UP113, DQ179125), growing on half-strength, modified Melin-Norkrans medium (Marx, 1969) were used as EMF inoculum. After 12 weeks growth (300 µmol m−2 s−1 photosynthetic photon flux density (PPFD); 16 h light at 18 ∘C and 8 h dark at 15 ∘C) seedlings were removed from the petri dishes and planted in 10 cm × 10 cm × 10 cm pots filled with a 1:10 v∕v sterilized, autoclaved peat / quartz sand mixture. These pots were maintained under the conditions detailed above for 6 months and watered three times per week with ∼20 mL of nutrient solution. The composition of this nutrient solution was 600 µM NH4NO3, 140 µM K2HP04, 150 µM Ca(NO3)2, 80 µM K2SO4, 15 µM H3BO3, 0.3 µM Na2MoO4, 0.3 µM ZnSO4, 0.3 µM CuSO4, and 50 µM Mg(NO3)2.
In order to incorporate bacterial–ectomycorrhizal interactions, which may be important to mineral weathering (Uroz et al., 2007, 2009), experimental columns were inoculated with a fungus-free bacterial suspension extracted on April 2008 from E horizon soil and humus collected from a local P. sylvestris boreal forest (59.785∘ N, 17.683∘ E; Lunsen forest, Uppsala, Sweden). The collected soil was subjected to sequential centrifugation in Winogradsky salt solution (Faegri et al., 1977), followed by Nycodenz (Medinor AB, Stockholm, Sweden) extraction at ultra-high speed (Courtois et al., 2001). The resulting bacterial suspension was tested for the presence of culturable fungi by plating on potato dextrose agar, which yielded no observable fungal colonies. The bacterial suspension was used for inoculation within 2 d of extraction and was stored at 4–8 ∘C until application.
2.3 Soil column system and growth conditions
The pre-grown seedlings were transplanted from pots into a sand culture system with opaque plexiglass tubes (4 cm diameter, height 30 cm) serving as vertical growth columns (van Hees et al., 2006). Each column was filled with 405 g (dry weight) of a mineral mix (which mimicked the E horizon of a local boreal forest soil) comprised of 50 % quartz sand, 28 % oligoclase, 18 % microcline, 1.8 % hornblende, 0.9 % vermiculite, and 0.9 % biotite. The quartz sand was acid washed (10 % HCl w∕v) overnight and washed with deionized water until the solution pH was > 6 before being mixed with the other minerals. The complete mix was approximately 40 % silt size class (70–100 µm) and 60 % sand size class (100–500 µm). The columns drained into opaque 250 mL glass bottles via a ceramic lysimeter cup (655X01, Soilmoisture Equipment Corp., Santa Barbara, CA) at the base of the columns. Suction was applied to the bottles to induce drainage. After packing the mineral mixture into the columns, and before seedlings were planted into the columns, the watering regime and drainage efficiency were established over 3 weeks. Rhizon SMS–MOM suction lysimeter samplers (0.3 cm diameter, 3 cm length; Rhizosphere Research Products, Wageningen) were inserted horizontally 10 cm below the soil surface to extract the rhizosphere soil solution and measure LMWOA concentrations within. One seedling was planted in each column (except for the eight non-planted controls). At the time of planting three seedlings from each treatment (non-mycorrhizal, P. fallax, and S. variegatus) were dried for future analysis. One week after planting, each column was inoculated with 5 mL of the fungus-free bacterial inoculum described above.
The columns were incubated in two adjacent, climate-controlled chambers (16 h light at 20 ∘C and 8 h dark at 15 ∘C), and in one chamber CO2 levels were maintained at 330–380 ppm (ambient) and in the other 700–750 ppm (elevated). Light was supplied by a high-pressure sodium lamp with an intensity of 300 PPFD at the seedling tops. The columns were watered three times per week with nutrient solution (72–108 mL per column per week). The watering solution was 33 µM (NH4)2HPO4, 407 µM NH4NO3, 27.5 µM K2HP04, 55 µM Ca(NO3)2, 27.5 µM K2SO4, 5.5 µM H3BO3, 1 µM FeCl3, 0.1 µM Na2MoO4, 0.1 µM ZnSO4, 0.1 µM CuSO4, and 55 µM Mg(NO3)2, and the pH was adjusted to 5.0. Four hours after watering, suction was applied for 1 hour to the bottom of the columns via the ceramic lysimeters, and the column leachate was collected in the glass bottles beneath each column.
2.4 Sampling and chemical analysis
Column leachate was sampled from drainage bottles beneath each column every 3–4 weeks throughout the experiment (in total 11 times). At each sampling, solution volume was measured, and duplicate 15 mL aliquots from each column were collected and frozen for future elemental analysis. The pH was measured for the leachate of each column at seven of the sampling dates. Rhizosphere soil solution was extracted for low-molecular-weight organic acid (LMWOA) analysis by applying suction to lysimeter samplers 24–36 h after watering. Rhizosphere soil solution samples were collected five times from each column at 5, 6, 7, 8, and 9 months post planting, and samples were immediately frozen at −20 ∘C for subsequent analysis.
At harvest, seedlings were removed from the mineral mix, and adhering mineral particles were classified as the rhizosphere soil fraction and collected by dry-shaking the roots. Rhizosphere and bulk mineral mix fractions were collected, weighed, dried, and stored for future analysis. Seedling roots were washed with deionized water and classified as abundantly colonized (> 50 % of root tips colonized), moderately colonized (50 % > colonization > 5 %), or sparsely colonized (< 5 % colonized) by ectomycorrhizal fungi. Each seedling was separated into roots, stem, and needles and dried at 60 ∘C for 72 h after which the dry weight (DW) was measured.
Concentrations of LMWOAs in rhizosphere soil solution samples were determined by capillary electrophoresis using the method of Dahlén et al. (2000). Briefly, LMWOAs were analyzed on an Agilent 3DCE capillary electrophoresis system (Agilent Technologies, Santa Clara). The concentrations of 12 different LMWOAs were analyzed: acetate, butyrate, citrate, formate, fumarate, lactate, malate, malonate, oxalate, propionate, succinate, and shikimate. LMWOA data are presented in micromoles per liter (µmol L−1) solution collected from rhizosphere lysimeters and as micromoles per liter per gram (µmol L−1 g−1) plant DW.
To measure elemental contents, acid digestion of plant material was undertaken following the procedure of Zarcinas et al. (1987) as follows: 0.1 g of each seedling component (needles, stems, and roots), pre-ground on a Wiley® mill (Thomas Scientific; Swedesboro, USA), was separately digested at room temperature overnight in 2 mL concentrated HNO3 (10 N), heated up to and refluxed at 130 ∘C with a funnel lid for 5 to 7 h, and subsequently diluted with 12–15 mL deionized water.
Ammonium-acetate-extractable elements (Ec) were measured in the post-harvest mineral mix for each column as well as for nine replicates of the pre-experimental mineral mix. Extractions were performed in a 1:10 (m∕v) mineral mix : 1 M NH4Ac suspension by shaking for 5 h at 100 rpm at room temperature. The supernatant was separated by centrifugation (3000 g) and filtered through a pre-washed 0.45 µm Na acetate filter syringe. The pre-experimental mineral mix was equilibrated with the nutrient solution 3 × 12 h to mimic the pre-planting treatment of minerals in columns.
Plant digests, Ec extracts, and column leachate were all analyzed for elemental contents of Al, Ca, Fe, K, Mg, Mn, Na, P, S, and Si on a PerkinElmer (Waltham, USA) atomic optical emission inductively coupled plasma emission spectrometer (ICP–OES). A set of four standards was established based on preliminary analysis for each sample type. In addition to rerunning standards hourly, duplicates and an internal scandium standard were run to ensure an accuracy of elemental contents to ±1 %. Total elemental loss (µmol) through column leachate was calculated from the leachate concentration and the total volume of leachate at each sampling time.
Plant roots and mineral mix were assayed for chitin content post harvest to assess fungal biomass. Chitin was extracted and analyzed by HPLC at the Department of Forest Ecology and Management, SLU (Sweden), according to the method of Ekblad and Näsholm (1996). The chitin concentration of roots, rhizosphere mineral mix, and bulk mineral mix were multiplied by the mass of that fraction, and these sums were added to obtain total chitin content per column. To relate fungal biomass to plant biomass, the total chitin content (root + rhizosphere + mineral mix) was divided by the total plant biomass in each column.
2.5 Construction of elemental budgets to estimate weathering
Elemental budgets of Ca, K, Mg, and Si were constructed to estimate weathering, using the four pools: (1) nutrients added (Na); (2) seedling uptake (Su: final elemental content in plants – pre-experimental plant contents); (3) elemental leaching (Lt: total leachate); and (4) ammonium-acetate-extractable elements (Ec). All values were calculated in micromoles and minimum weathering (Wm) was represented by the following equation:
We use the term minimum weathering to account for possible secondary minerals which may have formed during the experimental period and which were not extracted with the ammonium acetate extraction. ΔEc is the change in ammonium-acetate-extractable elements over the course of the experimental period, and it is calculated as follows:
Transpiration by the seedlings was calculated as the % water lost from the total solution added as
where leachatenp is the total solution volume (mean liters per column) collected from column flow through in non-planted treatments, leachatep is the total column flow through in planted treatments, MCnp is the total solution volume remaining in non-planted columns (dry mineral mass × % moisture determined by drying; mean liters per column) upon harvest, and MCp is the total solution volume remaining in planted columns upon harvest. In total, 3.4 L of nutrient solution was added to the columns over the course of the experiment.
To evaluate the potential for nutrient uptake to stimulate weathering via reducing solution concentrations of weathering products, the effect of plants on column solution concentrations of weathering products was estimated as the portion of weathered and added elements (in nutrient solution) which plants removed from solution as
which can also be expressed as
2.6 Statistical analysis
Except where explicitly stated otherwise, all data are presented as the mean per column for each treatment ± standard error of the mean (SE). LMWOA and chitin are also presented as mean per column per unit seedling mass. Two different independent variables were investigated: CO2 (ambient and elevated) and biological treatment (non-planted, non-mycorrhizal, P. fallax and S. variegatus). For some comparisons only the planted treatments (non-mycorrhizal, S. bovinus, and P. fallax) were examined and are henceforth referred to as the “planted” treatments. First, two-way ANOVA was used to determine treatment and interaction effects. When looking at the effects of the planted treatments the two CO2 treatments (ambient and elevated) were combined, and when examining the effects of CO2, the planted treatments (non-mycorrhizal, P. fallax, and S. variegatus) were combined. Statistical analysis was performed using JMP software version 5.01a (SAS Institute, Inc., Cary, NC, USA) and R v. 3.1.2 (R Core Team, 2015). Significant differences between CO2 treatments were assessed with Student's t test, and significant differences between biological treatments with Tukey's HSD test, both using a one-way ANOVA.
The chemical speciation and equilibria model Visual MINTEQ (Gustafsson, 2007) was used to examine the possibility of formation of secondary minerals within a range of chemical conditions based on data collected in our experiment. The model was run across a range of LMWOA concentrations (0–10 × observed), with pH values (pH 5 − observed) and leachate elemental concentrations (0–2 × observed) as model inputs.
3.1 Seedling growth and mycorrhizal colonization
Elevated CO2 significantly increased the biomass of seedlings (P<0.04), but had no significant effect on the root : shoot ratio. Mycorrhizal inoculation had no significant effect on growth or the root : shoot ratio (Table 1). There was no interactive effect of mycorrhizal treatment and CO2.
Table 1Seedling biomass, root : shoot mass ratio, and seedling chitin contents (mg chitin g−1 seedling dry weight) by treatment (mean per column). Values in parentheses are standard errors of the mean; values paired with different letters are significantly different (p<0.05) either between ambient and elevated CO2 (left half of table) or among planted treatments (right half of table).

The root systems extended 12–21 cm down the 30 cm columns, but the majority of roots were densely clustered in the top 10 cm. Mycorrhizal colonization was highly variable. Of the 20 seedlings in the mycorrhizal inoculated treatments, 6, 4, and 10 seedlings were abundantly, moderately, and sparsely colonized, respectively, at the time of harvest. No mycorrhizae were observed deeper than 6 cm. In the non-mycorrhizal inoculated treatment, turgid, smooth, black root tips were observed which may have been thelephoroid mycorrhiza. Chitin content in non-planted controls was negligible and significantly higher in planted treatments (Fig. 1) (P< 0.002). Non-inoculated treatments had, on average, half as much chitin per column as the mycorrhizal-inoculated treatments. Inoculated treatments had significantly more chitin per gram seedling biomass (Table 1 and Fig. 2) compared to the non-mycorrhizal inoculated treatment. In the P. fallax treatments elevated CO2 was associated with significantly higher chitin per unit seedling biomass (Fig. 2).
3.2 Low-molecular-weight organic acids
Formic, lactic, and acetic acids made up the majority of measured LMWOAs, comprising 82 %, 12 %, and 4 % of total measured LMWOAs, respectively. Much smaller amounts of malonic, oxalic, fumaric, and succinic acids were occasionally detected, but their occurrence in measurable quantities was not associated with any specific treatment. Planted columns had significantly higher LMWOA concentrations compared to non-planted columns (P<0.001), while P. fallax columns had significantly lower LMWOA concentrations (P<0.05) than either the non-inoculated or S. variegatus columns (Fig. 3a). Formic acid concentrations and total LMWOA concentrations were significantly higher (P<0.01) in the elevated CO2 treatments compared to ambient conditions (Fig. 3b).
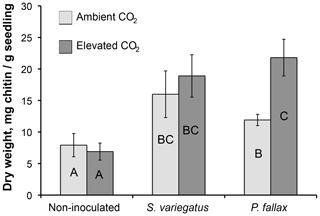
Figure 2Total chitin content per unit seedling mass (mg g−1) for planted treatments by CO2 treatment. Error bars are equivalent to the standard error of the mean in length. Bars that do not share letters are significantly different (p<0.05).
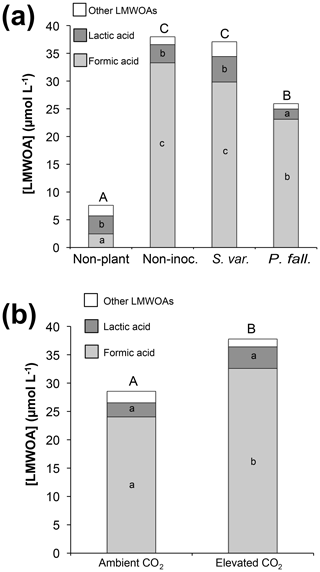
Figure 3Effects of (a) biological treatment and (b) CO2 treatment (planted columns only) on concentrations of low-molecular-weight organic acids (LMWOA) in rhizosphere soil solution collected with lysimeters (µmol L−1). Values are the average of five sampling occasions. Bars that do not share letters are significantly different (p<0.05). Capital letters atop each bar refer to total LMWOA.
3.3 Seedling transpiration, leachate pH, and elemental losses
Seedlings transpired on average 23 % (±2 % SEM) of the water added to each column. Leachate pH was consistently alkaline (pH 7.0–9.4) despite the added nutrient solution being acid at pH 5. There was no significant difference in leachate pH between elevated and ambient CO2 treatments or among planted treatments. On five of the seven sampling dates leachate of the non-planted controls had significantly lower pH (P<0.001) than that of planted treatments (Fig. 4). At the first time point (43 d after planting) both planted and non-planted columns had an average pH of 8.8 (±0.04 SEM). Over the course of the experiment the pH of the leachate was stable in planted columns (8.6±0.2 SEM), while the leachate from the non-planted columns decreased (∼0.8 pH units), suggesting that seedlings stabilized the columns at the initial alkaline conditions. Under elevated CO2, less K, Ca, Mg, and Si was lost from the columns in leachate compared to the ambient CO2 treatment. More K, Ca, and Mg leached from non-planted treatment than for the planted treatments, while the opposite was true for Si (Table 2). Concentrations of K, Ca, Mg, and Si in leachate were relatively steady over time (data not shown).
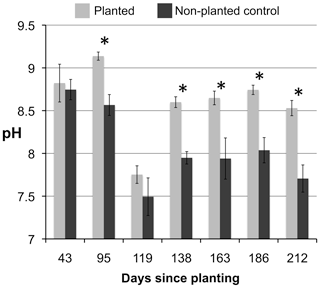
Figure 4pH of leachate for planted (n=28) and non-planted columns (n=8) on seven different sampling dates. Error bars are equivalent to the standard error of the mean in length. Pairs of columns topped with the * symbol represent significant differences (p<0.001).
Table 2Mean total elemental losses in column leachate (total µmol per column). Values in parentheses are standard errors of the mean; values paired with different letters are significantly different (p<0.05) either between planted columns with ambient and elevated CO2 (left half of table) or between biological treatments (right half of table).

3.4 Extractable elements
Overall, CO2 had no effect on Ec elements. For Ca and Si, planted columns had significantly higher Ec than non-planted columns, while mycorrhizal inoculation, but not seedlings alone, increased the Ec for Mg and K (Table 3). For some elements and treatments, the Ec was considerably higher before the experiment than after (Table 3). In particular, a large decrease in Ec for Ca was observed. Decreased Ec was also observed for Mg and K in non-inoculated treatments and for P. fallax in the case of Mg.
Table 3Change in surface ammonium-acetate-extractable elements ΔEc (Ec_final–Ec_initial: mean µmol per column). Values paired with different letters are significantly different (p<0.05) either between planted columns with ambient and elevated CO2 (left half of table) or biological treatments (right half of table).

3.5 Seedling elemental contents
The needle concentrations of Ca, K, Mg, Fe, and P were all at or above sufficiency thresholds for P. sylvestris (Breakke, 1994; Bargagli, 1998) (data not shown). Total seedling contents of Ca, K, or Mg did not vary significantly between the CO2 treatments (Table 4), despite significant differences in seedling biomass between CO2 treatments (Table 1). Mycorrhizal inoculated was not associated with a significant difference in total seedling contents of Ca, K, or Mg, but EMF colonization, particularly with P. fallax, increased seedling Mg concentration (Table 4).
3.6 Estimating weathering using an elemental budget
Whole column elemental budgets for the elements Si, Ca, K, and Mg show no effect of CO2 on element pools (Table 5). The presence of seedlings, mycorrhizal or not, significantly enhanced weathering compared to non-planted controls (Table 5). Mycorrhizal inoculation had no significant effect on weathering for any of the elements examined. Compared to the non-mycorrhizal and S. variegatus treatments, more Si (23 %) and Mg (27 %) were weathered in columns planted with P. fallax-inoculated seedlings, but these differences were not statistically significant (Table 5). The major sink for weathered products Mg, K, and Ca in the seedling treatments was seedling uptake, particularly for Mg (Fig. 5b–d). For Si, seedling uptake was negligible (Fig. 5a). Seedlings took up ∼5 % of the total dissolved Si, 68 % of the total dissolved K (±3 % SE), 54 % of the total dissolved Ca (±3 % SE), and 91 % of the total dissolved Mg (±3 % SE). After subtracting the nutrients added from the elemental budgets, the net weathering of Ca, K, and Mg in the non-planted treatment was negative or only slightly positive (Table 5). No formation of precipitates was predicted using the chemical speciation and equilibria model Visual MINTEQ (Gustafsson, 2007).
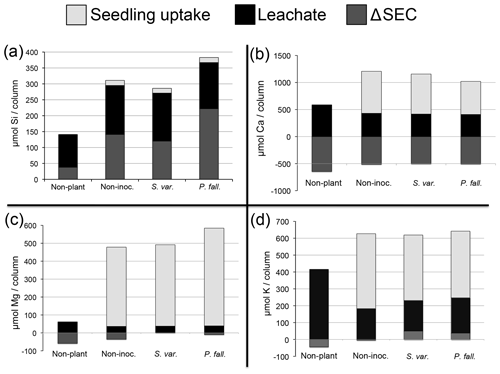
Figure 5Total elemental (µmol) losses for (a) Si, (b) Ca, (c) Mg, and (d) K (average per column). Non-plant represents non-planted columns. Non-myc represents non-mycorrhizal columns. P. fall. represents columns with seedlings colonized by P. fallax. S. var. represents columns with seedlings colonized by S. variegatus. Negative values for ΔEc indicate that Ec decreased over the course of the experiment.
Table 5Total weathering (µmol) losses for Ca, K, Mg, and Si (average per column). Total weathering = leachate + ΔEc + seedling uptake − nutrient additions. Values paired with different letters are significantly different (p<0.05) either between planted columns with ambient and elevated CO2 (left half of table) or between biological treatments (right half of table).

4.1 Growth and organic acid concentrations
Elevated CO2 increased the biomass of the P. sylvestris seedlings. Other studies of coniferous seedlings have generally found a growth stimulation with elevated CO2 (reviews by Ceulemans and Mousseau, 1994; Norby et al., 2005), including several studies of mycorrhizal P. sylvestris seedlings (Gorissen and Kuyper, 2000; Alberton et al., 2007), though other studies have also found no plant growth effect of elevated CO2 (Gorissen and Kuyper, 2000; Fransson and Johansson, 2010; Fransson et al., 2007). Plant growth stimulation from elevated CO2 is often accompanied by an increase in the root : shoot ratio (Gorissen and Kuyper, 2000; Janssens et al., 2005; Alberton et al., 2007). While we did not detect any change in root : shoot ratio, elevated CO2 was associated with significantly more chitin in both roots and bulk mineral mix and especially for P. fallax, possibly indicting higher belowground allocation to fungi under elevated CO2. Fransson and Johansson (2010) found P. fallax to be the most responsive to elevated CO2 among the five fungal species examined. Increased growth of mycorrhizal fungi in response to elevated CO2 treatments (see reviews by Alberton et al., 2005; Compant et al., 2010) is highly species specific (Fransson et al., 2007; Gorissen and Kuyper, 2000; Parrent and Vilgalys, 2007).
While EMF are often mentioned in the literature to produce significant amounts of LMWOAs, our findings seem to fall in line with the majority of studies examining the EMF role in LMWOA production, which fail to find an increase in LMWOA production when comparing EMF and non-EMF seedlings (van Schöll et al., 2006; van Hees et al., 2005). However, many of these studies have found that EMF significantly alter the composition of LMWOAs produced and particularly increase oxalic acid concentrations (van Schöll, 2006; van Hees et al., 2006; Ahonen-Jonnarth, 2000), which we did not observe. However, a number of studies in ectomycorrhizal systems have also observed higher soil solution concentrations of formic, acetic, and lactic acids compared to the weathering-promoting citric and oxalic acids (Strobel et al., 2001; van Hees et al., 2002, 2006; Ray and Adholeya, 2009). Studies that find particularly high concentrations of oxalic acid, are typically pure culture studies with high nitrogen availability (Rosling, 2009), in which rapid microbial degradation of LMWOAs is excluded. Despite the fact that our rhizosphere lysimeters were placed in the area of highest root density, it is possible that the organic acid profile we observed is primarily the product of microbial activity, following partial decomposition of plant exudates and other soil organic matter. It is, however, interesting to note that the planted treatments all had far higher LMWOA concentrations, and LMWOA concentrations were correlated with seedling biomass.
Elevated CO2 was associated with significantly higher total LMWOA concentrations, potentially indicating greater carbon allocation to root exudation by seedlings under elevated CO2, which is in agreement with previous studies (Fransson and Johansson, 2010). However this stimulation by elevated CO2 appeared to be primarily a function of larger seedlings, as the elevated CO2 treatment was not associated with higher LMWOA concentrations per unit seedling biomass. Similar to Fransson and Johansson (2009), we did not find that elevated CO2 increased LMWOA production beyond its effect on seedling biomass.
4.2 Column nutrient budgets
After subtracting the nutrients added from the elemental budgets, the net weathering of Ca, K, and Mg in the non-planted treatment was negative or only slightly positive (Table 5), suggesting a “missing sink” for weathering products in these treatments or possibly calling into question the negative ΔEc values. As stated previously, ΔEc was negative for Ca and slightly negative for K and Mg in some treatments. Negative ΔEc arises from the mineral mix having higher ammonium-acetate-extractable elements at the beginning of the growth period than at the end. Alternatively, it is possible that the equilibration with nutrient solution that occurred over 3 weeks in the columns prior to planting was not accurately replicated by the flushing steps we subjected the pre-mineral mix to in preparation for measuring the Ec on the “before planting” mineral mix. We think it is likely that significant elemental losses may have occurred during this 3-week flushing phase before planting, but this would have been the same for all treatments and thus should not affect our interpretation of biological enhancement of weathering. Alternatively, it is possible that secondary precipitates formed during the experimental period that we were not able to completely extract with the ammonium acetate extraction. Using a broad range of solution chemistry we were not able to predict the formation of precipitates using the chemical speciation and equilibria model Visual MINTEQ (Gustafsson, 2007). In addition, at the pH values we observed, we would expect higher pH to be associated with greater secondary mineral formation, and we observed that the planted treatments had both higher pH and higher estimated total weathering.
4.3 Biotic enhancement of weathering
Biotic enhancement of weathering was observed in the present study as the presence of seedlings had a significant effect on every individual elemental flux. For K, Mg, and Ca increased weathering products were primarily taken up by the seedlings, while for Si increased weathering products were recovered from the leachate and mineral matrix. Elevated CO2 had no significant effect on the weathering of Ca, K, Mg, or Si despite increased plant growth in this treatment. Biological uptake of Si was likely underestimated due to the inadequacy of aqua regia as a solvent for biological Si. Considering a total seedling biomass of 4–5 g and a wide range of potential SI concentrations (0.01 %–0.5 %; taken from measurements of Douglas fir, black pine, and Norway spruce plant biomass from a mature forest; Cornelis et al., 2010), plant Si pools could vary between 14 and 700 µmol per seedling; the contents we observed after aqua regia digestion averaged 15.2 µmol per seedling. The potentially incomplete digestion of biogenic Si from seedling biomass means that we have likely underestimated biotic enhancement of Si weathering.
There was, however, limited evidence for ectomycorrhizal stimulation of mineral weathering. The amounts of ammonium-acetate-extractable K and Mg in the mineral mix were found to be greater in the mycorrhizal inoculated treatments, and P. fallax appeared to solubilize more Si and Mg (23 % and 27 % greater mobilization, respectively, than non-mycorrhizal inoculated treatments, although not significantly) and take up significantly more Mg than either S. variegatus or the non-mycorrhizal treatment. Overall, ectomycorrhizal inoculation did not cause significantly greater weathering rates. It is possible that had our mycorrhizal treatments been more consistently colonized, and had the non-mycorrhizal inoculated treatment remained entirely free of mycorrhiza, we might have seen a clearer effect of mycorrhizal treatment on mineral weathering. However, half of the mycorrhizal treatment seedlings, and none of the non-inoculated seedlings, were heavily or moderately colonized at harvest. Further, when we examine seedling chitin content as an explanatory factor, there is no significant relationship between ectomycorrhizal colonization and the weathering fluxes of any element. Another reason for the lack of mycorrhizal stimulation of mineral weathering may be the limited rooting volume to which the seedlings were confined. The upper half of the columns had very dense rooting, and thus the ability for ectomycorrhizal fungi to greatly increase the exploitable soil volume of a plant was not appreciably incorporated into this experimental arena.
Soil biota is capable of directly stimulating weathering of alumina silicate minerals by four distinct mechanisms: proton promotion, via biological proton exudation; ligand promotion: via organic ligand exudation; reduction of transport limitation via nutrient uptake; and physical disruption via physical forcing of minerals by hyphae or root hairs. There is considerable debate as to which of these four mechanisms dominates the biotic influence on weathering (see reviews by Drever, 1994; Hinsinger et al., 2006; Lucas, 2001; Harley and Gilkes, 2000, and articles by Hinsinger et al., 2001; Bonneville et al., 2009). We have discounted physical disruption as a major contributing process to the biological stimulation of weathering due to both our use of freshly ground primary minerals without pre-existing fissures and cracks for hyphae to exploit and the relatively short time span of the experiment, and this excluded the potential for significant increase in available mineral surface area by biomechanical forcing (Pawlik et al., 2016). In the present study, seedling uptake of nutrient cations, and the resulting reduction in transport limitation to weathering, stands out as the most likely mechanism of biological enhancement of weathering through which the presence of P. sylvestris caused the observed elevated weathering. Removal of transport limitation may occur if seedlings significantly reduce the concentrations of weathering products at or near mineral surfaces and/or significantly alter solution flow rates. By transpiring 23 % of column moisture and taking up 54 %–91 % of the major nutrient cations from the solution, seedlings exerted considerable influence on both column hydrology and elemental concentration gradients in this system.
In light of the alkaline pH of the leachate, proton promotion was probably not a likely mechanism by which seedlings enhanced weathering. The high pH of the column leachate (7.0–9.4) may not reflect the pH of the rooting zone since leachate pH was collected after 30 cm of vertical percolation through ground primary minerals, while ectomycorrhizae and most roots were restricted to the uppermost portions of the columns. However, the pH from planted treatments was significantly higher than for unplanted columns and remained high while unplanted columns became increasingly neutral over the course of the experiment. This trend is the opposite of what would be expected if biotic acidification (proton-promoted dissolution) is an important mechanism by which seedlings enhance weathering in this system.
Ligand-promoted dissolution appears unlikely to have contributed significantly to biological enhancement of weathering since concentrations of the most weathering-enhancing organic acids were in the order of micromolar concentrations in the present study and not in millimolar concentrations which are required to significantly increase weathering (Drever and Stillings, 1997; Drever, 1994; Pokrovsky et al., 2009). The stimulatory effect of LMWOAs on mineral dissolution increases significantly at near-neutral and slightly alkaline pH values, as the relative rate of proton-promoted dissolution drops sharply (Welch and Ullman, 1993; van Hees et al., 2002; Stillings et al., 1996). However, the majority of LMWOAs detected in this experiment were the mono-carboxylic acids (formic, lactic, and acetic acids) and not the di- and tri-carboxylic acids, such as citric and oxalic acids, that have been shown to strongly increase weathering rates (Neaman et al., 2006; Drever and Stillings, 1997). In soil systems LMWOA concentrations are a result of production, microbial uptake rates, and adsorption (van Hees et al., 2005). LMWOA concentrations may have been considerably higher near mineral surfaces and under biofilms than those we measured; however the rhizosphere samplers were placed in the zone of highest root activity. LMWOAs represent one of a few possible weathering-promoting ligands of biotic origin and commonly comprise less than 10 % of dissolved organic carbon (Strobel, 2001). Organic compounds that are generally found in much lower concentrations than LMWOAs may be key ligand-promoted weathering agents in soil, such as siderophores (Lierman et al., 2000; Reichard et al., 2007; Watteau and Berthelin, 1994), which are exuded by EMF (van Hees et al., 2006) and bacteria (Liermann et al., 2000). We did not find detectable levels of iron or aluminum in column leachate (< 0.1 µM of Al and Fe vs. > 50 µM of Si, data not shown), and thus siderophores were not likely a significant contributor to biotic weathering enhancement in our system.
The findings presented here are in line with a growing body of evidence that identify transport limitation as the predominant process governing weathering rates of minerals in soil (Harley and Gilkes, 2000; Maher, 2010). Experiments using column reactors, which unlike batch reactors are not saturated and well mixed, have shown that element transport away from mineral surfaces is the rate-limiting step for weathering (Evans and Banwart, 2006; van Grinsven and van Riemsdijk, 1991). In a review of ectomycorrhizal weathering studies, Rosenstock (2009) also identified increased nutrient uptake as the main mechanism by which ectomycorrhizal fungi stimulate weathering. By taking up nutrients and stimulating solution flow, plant growth exerts a major influence on solution composition around mineral surfaces. While the actual weathering mechanism is likely ligand promotion or proton promotion, the weathering rate is controlled by nutrient uptake by plants and soil microorganisms.
In this experiment seedlings significantly increased weathering rates, while ectomycorrhizal colonization had limited effects; P. fallax showed some potential to enhance weathering rates, particularly with respect to Mg. The lack of a mycorrhizal effect may have been due to low mycorrhizal colonization and a lack of mycorrhizal plant growth promotion. Enhancement of weathering by seedling growth is most likely explained by nutrient uptake through its influence on transport limitation. Elevated CO2 significantly increased plant growth and soil solution LMWOA concentrations but did not enhance total weathering. Caution is advised when relating results from ectomycorrhizal weathering experiments in containers with primary minerals and low organic content to phenomena occurring in natural forests. Studies on biotic weathering should separate biotic influences on weathering mechanisms (proton promotion, ligand promotion) from biotic influences on weathering rate-limiting factors (transport limitation).
No data sets were used in this article.
PAWvH, AR, PMAF, RDF, and NPR designed the experiment. AR, PMAF, and NPR conducted the experiment. NPR analyzed the data. NPR prepared the paper with assistance and contributions from AR, PMAF, PAWvH, and RDF.
The authors declare that they have no conflict of interest.
This article is part of the special issue “Quantifying weathering rates for sustainable forestry (BG/SOIL inter-journal SI)”. It is not associated with a conference.
We thank Torgny Näsholm, SLU, for performing the chitin analysis and thank Paul Brooks, UC Berkeley, for assistance with ICP analysis.
This research has been supported by the Swedish Research Council (bioweathering project).
This paper was edited by Nobuhito Ohte and reviewed by two anonymous referees.
Ahonen-Jonarth, U., van Hees, P. A. W., Lundstrom, U., and Finlay, R. D.: Organic acids produced by mycorrhizal Pinus sylvestris exposed to elevated aluminium and heavy metal concentrations, New Phytol., 146, 557–567, 2000.
Ainsworth, E. A. and Long, S. P.: What have we learned from 15 years of free-air CO2 enrichment (FACE)? A meta-analytic review of the responses of photosynthesis, canopy properties and plant production to rising CO2, New Phytol., 165, 351–372, 2005.
Alberton, O., Kuyper, T. W., and Gorissen, A.: Taking mycocentrism seriously: mycorrhizal fungal and plant responses to elevated CO2, New Phytol., 167, 859–868, 2005.
Alberton, O., Kuyper, T. W., and Gorissen, A.: Competition for nitrogen between Pinus sylvestris and ectomycorrhizal fungi generates potential for negative feedback under elevated CO2, Plant Soil, 296, 159–172, 2007.
Almeida, J. P., Rosenstock, N. P., Forsmark, B., Bergh, J., and Wallander, H.: Ectomycorrhizal community composition and function in a spruce forest transitioning between nitrogen and phosphorus limitation, Fungal Ecol., 40, 20–31, https://doi.org/10.1016/j.funeco.2018.05.008, 2018.
Balogh-Brunstad, Z., Keller, C. K., Dickinson, J. T., Stevens, F., Li, C. Y., and Bormann, B. T.: Biotite weathering and nutrient uptake by ectomycorrhizal fungus, Suillus tomentosus, in liquid-culture experiments, Geochim. Cosmochim. Ac., 72, 2601–2618, 2008.
Bargagli, R.: Trace Elements in Terrestrial Plants: an Ecophysiological Approach to Biomonitoring and Biorecovery, Springer, 324 pp., 1998.
Baribault, T. W., Kobe, R. K., and Rothstein, D. E.: Soil calcium, nitrogen, and water are correlated with aboveground net primary production in northern hardwood forests, Forest Ecol. Manag., 260, 723–733, 2010.
Bonneville, S., Smits, M. M., Brown, A., Harrington, J., Leake, J. R., Brydson, R., and Benning, L. G.: Plant-driven fungal weathering: Early stages of mineral alteration at the nanometer scale, Geology 37, 615–618, 2009.
Bonneville, S., Bray, A. W., and Benning, L. G.: Structural Fe(II) oxidation in biotite by an ectomycorrhizal fungi drives mechanical forcing, Environ. Sci. Technol., 50, 5589–5596, 2016.
Breakke, F. H.: Diagnostic levels of nutrient elements in Norway spruce and Scots pine needles, Aktuelt Skogforsk, 15, 1–11, 1994.
Ceulemans, R. and Mousseau, M.: Tansley Review No. 71 Effects of elevated atmospheric CO2 on woody plants, New Phytol., 127, 425–446, 1994.
Compant, S., van der Heijden, M. G. A., and Sessitsch, A.: Climate change effects on beneficial plant microorganism interactions, FEMS Microb. Ecol., 73, 197–214, 2010.
Cornelis, J. T., Ranger, J., Iserentant, A., and Delvaux, B.: Tree species impact the terrestrial cycle of silicon through various uptakes, Biogeochemistry, 97, 231–245, 2010.
Courtois, S., Frostegard, A., Goransson, P., Depret, G., Jeannin, P., and Simonet, P.: Quantification of bacterial subgroups in soil: comparison of DNA extracted directly from soil or from cells previously released by density gradient centrifugation, Environ. Microbiol., 3, 431–439, 2001.
Dahlen, J., Hagberg, J., and Karlsson, S.: Analysis of low molecular weight organic acids in water with capillary zone electrophoresis employing indirect photometric detection, Fresenius J. Anal. Chem., 366, 488–493, 2000.
Drever, J. I.: The effect of land plants on weathering rates of silicate minerals, Geochim. Cosmochim. Ac., 58, 2325–2332, 1994.
Drever, J. I. and Stillings, L. L.: The role of organic acids in mineral weathering, Colloid. Surface. A, 120, 167–181, 1997.
Ekblad, A. and Näsholm, T.: Determination of chitin in fungi and mycorrhizal roots by an improved HPLC analysis of glucosamine, Plant Soil, 178, 29–35, 1996.
Evans, K. A. and Banwart, S. A.: Rate controls on the chemical weathering of natural polymineralic material. I. Dissolution behaviour of polymineralic assemblages determined using batch and unsaturated column experiments, Appl. Geochem., 21, 352–376, 2006.
Faegri, A., Torsvik, V. L., and Goksoyr, J.: Bacterial and fungal activities in soil – Separation of bacteria and fungi by a rapid fractionated centrifugation technique, Soil Biol. Biogeochem., 9, 105–112, 1977.
Finlay, R., Wallander, H., Smits, M., Holmström, S., van Hees, P. A. W., Lian, B., and Rosling, A.: The role of fungi in biogenic weathering in boreal forest soils, Fungal Biol. Rev., 23, 101–106, 2009.
Finlay, R. D., Mahmood, S., Rosenstock, N., Bolou-Bi, E. B., Köhler, S. J., Fahad, Z., Rosling, A., Wallander, H., Belyazid, S., Bishop, K., and Lian, B.: Biological weathering and its consequences at different spatial levels – from nanoscale to global scale, Biogeosciences Discuss., https://doi.org/10.5194/bg-2019-41, in review, 2019.
Fransson, P. M. A. and Johansson, E. M.: Elevated CO2 and nitrogen influence exudation of soluble organic compounds by ectomycorrhizal root systems, FEMS Microb. Ecol., 71, 186–196, 2010.
Fransson, P. M. A., Anderson, I. C., and Alexander, I. J.: Does carbon partitioning in ectomycorrhizal pine seedlings under elevated CO2 vary with fungal species?, Plant Soil, 291, 323–333, 2007.
Godbold, D. L., Hoosebeek, M. R., Lukac, M., Cotrufo, M. F., Janssens I. A., Ceulemans, R., Polle, A., Velthorst, E. J., Scarascia-Mugnozza, G., De Angelis, P., Miglietta, F., and Peressotti, A.: Mycorrhizal hyphal turnover as a dominant process for carbon input into soil organic matter, Plant Soil, 281, 15–24, 2006.
Gorissen, A. and Kuyper, T. W.: Fungal species-specific responses of ectomycorrhizal Scots pine (Pinus sylvestris) to elevated [CO2], New Phytol., 146, 163–168, 2000.
Gustafsson, J. P.: Visual MINTEQ version 2.53, available at: https://vminteq.lwr.kth.se/ (last access: 23 September 2019), 2007.
Harley, A. D. and Gilkes, R. J.: Factors influencing the release of plant nutrient elements from silicate rock powders: a geochemical overview, Nut. Cyc. Agroeco., 56, 11–36, 2000.
Hinsinger, P., Barros, O. N. F., Benedetti, M. F., and Callot, G.: Plant-induced weathering of a basaltic rock: Experimental evidence, Geochim. Cosmochim. Ac., 65, 137–152, 2001.
Hinsinger, P., Plassard, C., and Jaillard, B.: Rhizosphere: A new frontier for soil biogeochemistry, J. Geochem. Explor., 88, 210–213, 2006.
Hobbie, E. A.: Carbon allocation to ectomycorrhizal fungi correlates with belowground allocation in culture studies, Ecology, 87, 563–569, 2006.
Janssens, I. A., Medlyn, B., Gielen, B., Laureysens, I., Jach, M. E., van Hove, D., and Ceulemans, R.: Carbon budget of Pinus sylvestris saplings after four years of exposure to elevated atmospheric carbon dioxide concentration, Tree Physiol., 25, 325–337, 2005.
Jonard, M., Fürst, A., Verstraeten, A., Thimonier, A., Timmermann,V., Potocǐc, N., Waldner, P., Benham, S., Hansen, K., Merila, P., Ponette, Q., de la Cruz, A. C., Roskams, P., Nicolas, M., Croise, L., Ingerslev, M., Matteucci, G., Decinti, B., Bascietto, M., and Rautio, P.: Tree mineral nutrition is deteriorating in Europe, Glob. Change Biol., 21, 418–430, 2015.
Liermann, L. J., Kalinowski, B. E., Brantley, S. L., and Ferry, J. G.: Role of bacterial siderophores in dissolution of hornblende, Geochim. Cosmochim. Ac., 64, 587–602, 2000.
Lindner, M., Maroschek, M., Netherer, S., Kremer, A., Barbati, A., Garcia-Gonzalo, J., Seidl, R., Delzon, S., Corona, P., Kolstrom, M., Lexer, M. J., and Marchetti, M.: Climate change impacts, adaptive capacity, and vulnerability of European forest ecosystems, Forest Ecol. Manag., 259, 698–709, 2010.
Lucas, Y.: The role of plants in controlling rates and products of weathering: Importance of biological pumping, Annu. Rev. Earth Planet. Sc., 29, 13–63, 2001.
Maher, K.: The dependence of chemical weathering rates on fluid residence time, Earth Planet. Sc. Lett., 294, 101–110, 2010.
Marx, D. H.: The influence of ectotrophic ectomycorrhizal fungi on the resistance of pine roots to pathogen infections I, Antagonism of mycorrhizal fungi to root pathogenic fungi and soil bacteria, Phytopathology, 59, 153–163, 1969.
Naples, B. K. and Fisk, M. C.: Belowground insights into nutrient limitation in northern hardwood forests, Biogeochemistry, 97, 109–121, 2010.
Neaman, A., Chorover, J., and Brantley, S. L.: Efects of organic ligands on granite dissolution in batch experiments at pH 6, Am. J. Sci., 306, 451–473, 2006.
Norby, R. J., Wullschleger, S. D., Gunderson, C. A., Johnson, D. W., and Ceulemans, R.: Tree responses to rising CO2 in field experiments: implications for the future forest, Plant Cell Environ., 22, 683–714, 1999.
Norby, R. J., DeLucia, E. H., and Gielen, B.: Forest response to elevated CO2 is conserved across a broad range of productivity, P. Natl. Acad. Sci. USA, 102, 18052–18056, 2005.
Ochs, M., Brunner, I., Stumm, M., and Cosovic, B.: Effects of root exudates and humic substances on weathering kinetics, Water Air Soil Pollut., 68, 213–229, 1993.
Paris, F., Botton, B., and Lapeyrie, F.: In vitro weathering of phlogopite by ectomycorrhizal fungi, 2. Effect of K+ and Mg2+ deficiency and N sources on accumulation of oxalate and H+, Plant Soil, 179, 141–50, 1996.
Parrent, J. L. and Vilgalys, R.: Biomass and compositional responses of ectomycorrhizal fungal hyphae to elevated CO2 and nitrogen fertilization, New Phytol., 176, 164–174, 2007.
Pawlik, L., Phillips, J. D., and Šamonil, P.: Roots, rock, and regolith: Biomechanical and biochemical weathering by trees and its impact on hillslopes – A critical literature review, Earth-Sci. Rev., 159, 142–159, 2016.
Pinkard, E. A., Beadle, C. L., Mendham, D. S., Carter, J., and Glen, M.: Determining photosynthetic responses of forest species to elevated [CO2]: Alternatives to FACE, Forest Ecol. Manag., 260, 1251–1261, 2010.
Pokrovsky, O. S., Shirokova, L. S., Benezeth, P., Schott, J., and Golubev, S. V.: Effect of organic ligands and heterotrophic bacteria on wollastonite dissolution kinetics, Am. J. Sci., 309, 731–772, 2009.
R Core Team: R: A language and environment for statistical computing, R Foundation for Statistical Computing, Vienna, Austria, available at: http://www.R-project.org/ (last access: 23 September 2019), 2015.
Ray, P. and Adholeya, A.: Correlation between organic acid exudation and metal uptake by ectomycorrhizal fungi grown on pond ash in vitro, Biometals, 22, 275–281, 2009.
Reichard, P. U., Kretzschmar, R., and Kraemer, S. M.: Rate laws of steady-state and non-steady-state ligand-controlled dissolution of goethite, Colloid. Surfaces A, 306, 22–28, 2007.
Rosenstock, N. P.: Can ectomycorrhizal weathering activity respond to host nutrient demands?, Fungal Biol. Rev., 23, 107–114, 2009.
Rosenstock, N. P., Berner, C., Smits, M. M., Kram, P., and Wallander, H.: The role of phosphorus, magnesium and potassium availability in soil fungal exploration of mineral nutrient sources in Norway spruce forests, New Phytol., 211, 542–553, 2016.
Rosling, A.: Trees, mycorrhiza and minerals – field relevance of in vitro experiments, Geomicrobiol. J., 26, 389–401, 2009.
Rosling, A., Lindahl, B. D., Taylor, A. F. S., and Finlay, R. D.: Mycelial growth and substrate acidification of ectomycorrhizal fungi in response to different minerals, FEMS Microbiol. Ecol., 47, 31–37, 2004.
Schmalenberger, A., Duran, A. L., Bray, A. W., Bridge, J., Bonneville, S., Benning, L. G., Romero-Gonzalez, M. E., Leake, J. R., and Banwart, S. A.: Oxalate secretion by ectomycorrhizal Paxillus involutus is mineral-specific and controls calcium weathering from minerals, Sci. Rep., 5, 12187, https://doi.org/10.1038/srep12187, 2015.
Smith, S. E. and Read, D. J.: Mycorrhizal Symbiosis, 3rd Edn., Academic Press, Amsterdam, London, 800 pp., 2008.
Stillings, L. L., Drever, J. I., Brantley, S. L., Sun, Y., and Oxburgh, R.: Rates of feldspar dissolution at pH 3–7 with 0–8 mM oxalic acid, Chem. Geol., 132, 79–89, 1996.
Strobel, B. W.: Influence of vegetation on low-molecular-weight carboxylic acids in soil solution – a review, Geoderma, 99, 169–198, 2001.
Uroz, S., Calvaruso, C., Turpault, M. P., Pierrat, J. C., Mustin, C., and Frey-Klett, P.: Effect of the Mycorrhizosphere on the Genotypic and Metabolic Diversity of the Bacterial Communities Involved in Mineral Weathering in a Forest Soil, Appl. Environ. Microb., 73, 3019–3027, 2007.
Uroz, S., Calvaruso, C., Turpault, M. P., Sarniguet, A., de Boer, W., Leveau, J. H. J., and Frey-Klett, P.: Efficient mineral weathering is a distinctive functional trait of the bacterial genus Collimonas, Soil Biol. Biochem., 41, 2178–2186, 2009.
van Grinsven, J. J. M. and van Riemsdijk, W. H.: Evaluation of batch and column techniques to measure weathering rates in soils, Geoderma, 52, 41–57, 1992.
van Hees, P. A. W., Lundström, U. S., and Mörth, C. M.: Dissolution of microcline and labradorite in a forest O horizon extract: the effect of naturally occurring organic acids, Chem. Geol., 189, 199–211, 2002.
van Hees, P. A. W., Jones, D. L., Jentschke, G., and Godbold, D. L.: Mobilization of aluminum, iron and silicon by Picea abies and ectomycorrhizas in a forest soil, Eur. J. Soil Sci., 55, 101–111, 2004.
van Hees, P. A. W., Jones, D. L., Finlay, R., Godbold, D. L., and Lundström, U. S.: The carbon we do not see – the impact of low molecular weight compounds on carbon dynamics and respiration in forest soils: a review, Soil Biol. Biochem., 37, 1–13, 2005.
van Hees, P. A. W., Rosling, A., and Finlay, R. D.: The impact of trees, ectomycorrhiza and potassium availability on simple organic compounds and dissolved organic carbon in soil, Soil Biol. Biochem., 38, 1912–1923, 2006.
van Schöll, L., Smits, M. M., and Hoffland, E.: Ectomycorrhizal weathering of the soil minerals muscovite and hornblende, New Phytol., 171, 805–814, 2006.
Wallander, H.: Uptake of P from apatite by Pinus sylvestris seedlings colonized by different ectomycorrhizal fungi, Plant Soil, 218, 249–256, 2000.
Watteau, F. and Berthelin, J.: Microbial dissolution of iron and aluminum from soil minerals – efficiency and specificity of hydroxamate siderophores compared to aliphatic-acids, Eur. J. Soil Biol., 30, 1–9, 1994.
Welch, S. A. and Ullman, W. J.: The effect of organic acids on plagioclase dissolution rates and stoichiometry, Geochim. Cosmochim. Ac., 57, 2725–2736, 1993.
Zarcinas, B. A., Cartwright, B., and Spouncer, L. R.: Nitric-acid digestion and multielement analysis of plant material by inductively coupled plasma spectrometry, Commun. Soil Sci. Plant Anal., 18, 131–146, 1987.