the Creative Commons Attribution 4.0 License.
the Creative Commons Attribution 4.0 License.
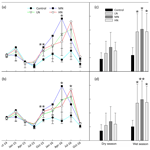
The simulated N deposition accelerates net N mineralization and nitrification in a tropical forest soil
Yanxia Nie
Xiaoge Han
Jie Chen
Mengcen Wang
Elevated nitrogen (N) deposition affects soil N transformations in the N-rich soil of tropical forests. However, the change in soil functional microorganisms responsible for soil N cycling remains largely unknown. Here, we investigated the variation in soil inorganic N content, net N mineralization (Rm), net nitrification (Rn), inorganic N leaching (Rl), N2O efflux and N-related functional gene abundance in a tropical forest soil over a 2-year period with four levels of N addition. The responses of soil net N transformations (in situ Rm and Rn) and Rl to N additions were negligible during the first year of N inputs. The Rm, Rn, and Rl increased with the medium nitrogen (MN) and high nitrogen (HN) treatments relative to the control treatments in the second year of N additions. Furthermore, the Rm, Rn, and Rl were higher in the wet season than in the dry season. The Rm and Rn were mainly associated with the N addition-induced lower C:N ratio in the dry season but with higher microbial biomass in the wet season. Throughout the study period, high N additions increased the annual N2O emissions by 78 %. Overall, N additions significantly facilitated Rm, Rn, Rl and N2O emission. In addition, the MN and HN treatments increased the ammonia-oxidizing archaea (AOA) abundance by 17.3 % and 7.5 %, respectively. Meanwhile, the HN addition significantly increased the abundance of nirK denitrifiers but significantly decreased the abundance of ammonia-oxidizing bacteria (AOB) and nosZ-containing N2O reducers. To some extent, the variation in functional gene abundance was related to the corresponding N-transformation processes. Partial least squares path modelling (PLS-PM) indicated that inorganic N contents had significantly negative direct effects on the abundances of N-related functional genes in the wet season, implying that chronic N deposition would have a negative effect on the N-cycling-related microbes and the function of N transformation. Our results provide evidence that elevated N deposition may impose consistent stimulatory effects on soil N-transformation rates but differentiated impacts on related microbial functional genes. Long-term experimentation or observations are needed to decipher the interrelations between the rate of soil N-transformation processes and the abundance or expression of related functional genes.
- Article
(2245 KB) - Full-text XML
-
Supplement
(327 KB) - BibTeX
- EndNote
Due to anthropogenic activity in recent decades, the increased atmospheric reactive nitrogen (N) deposition in terrestrial ecosystems has altered the N status and dynamics (Galloway et al., 2008). Excessive N inputs to forest ecosystems will certainly influence soil N cycling and ecosystem function. In the last 3 decades, several studies have focused on the impacts of N deposition on soil N cycling in northern and temperate forests (Aber et al., 1989, 1998; Gundersen et al., 1998; Nave et al., 2009; Tian et al., 2018). However, in recent years, tropical forests have received the most dramatic increases in N deposition and are considered as N-rich areas (Hietz et al., 2011; Liu et al., 2013). In southern China, forest ecosystems, such as the hotspots of N deposition receiving 13.8–113 kg N ha−1 yr−1 through precipitation, have reached N-saturation status (Fang et al., 2008; Chen et al., 2016; Yu et al., 2018). Little is known about the hazards of constant N inputs on N-saturated forest ecosystem functioning. More attention should be focused on examining the effects of N addition on soil N transformations in N-rich tropical forests.
Soil N availability and turnover are quantified by the N-transformation rates in the forest soil (Gao et al., 2016; Patel and Fernandez, 2018). Few previous studies reported the alteration of N-transformation rates after N additions have received inconsistent results for tropical forest ecosystems. For example, in Hawaiian Islands, N addition to a phosphorous (P)-limited tropical forest (4.1-million-year-old) did not change the rate of net N mineralization (Rm) but significantly increased the net nitrification rate (Rn) and nitrogen oxide (i.e. NO and N2O) emission (Lohse and Matson, 2005). In contrast, N addition to a younger N-limited forest (300-year-old) significantly increased soil Rm, Rn and nitrate leaching (Hall and Matson, 1999, 2003), and the differentiated responses between the two forests were mainly determined by soil age and nutrient status (Hall and Matson, 1999, 2003; Lohse and Matson, 2005). The N addition effects on gross N mineralization and nitrification rates were apparent after chronic (9-year) N additions in a lowland tropical forest but were obvious with short-term (1-year) N additions in a montane tropical forest in Panama; the difference was mainly due to the different soil types and whether there existed an organic layer (Koehler et al., 2009; Corre et al., 2010). In southern China, a 6-year N addition significantly increased N2O emission and nitrate leaching but decreased Rm and Rn in a tropical broadleaf forest, possibly due to the alteration of the soil microbial community composition and reduction of enzyme activity with N addition (Chen et al., 2016). In contrast, significant increases in Rm, Rn and Rl were observed with a 3-year N addition in an adjacent broadleaf forest (W. Zhang et al., 2008; Fang et al., 2009b, 2011). These previous studies suggest that the responses of soil N transformations to N addition in the tropical forests may vary with soil type, nutrient status (e.g. N limited, N saturated, or P limited), duration of N addition, and the alteration of soil microbial communities. Until now, only a small number of studies have directly quantified soil N-transformation rates in tropical forests, and the mechanisms of their conflicting responses to N additions are still unclear (Cheng et al., 2019).
A global meta-analysis showed that N deposition had a negative effect on soil microbial growth, diversity, composition and function (T. A. Zhang et al., 2018; Wang et al., 2018a), but soil N mineralization was mainly driven by soil microorganisms (Ollivier et al., 2011; Z. L. Li et al., 2019). Ammonia oxidation, the first and rate-limiting step of autotrophic nitrification, is performed by ammonia-oxidizing archaea (AOA) and ammonia-oxidizing bacteria (AOB) harbouring AOA amoA and AOB amoA genes, respectively, which are valuable indices for predicting soil potential nitrification rates (Petersen et al., 2012). AOA play a dominant role in ammonia oxidation in acidic forest soil and have a positive correlation with gross nitrification rates (Isobe et al., 2012). In addition, elevated N deposition enhances N loss by nitrate leaching and denitrification in tropical forest soil (Chen et al., 2016). The second step in denitrification of reducing to nitric oxide is catalysed by copper-containing reductase (encoded by the nirK gene) or cytochrome cd1-containing reductase (encoded by the nirS gene) (Braker et al., 2000). Previous studies have shown that nirK denitrifiers are more sensitive to environmental changes than the nirS denitrifiers (Chen et al., 2010; Y. Li et al., 2019). Furthermore, the abundance of the nirK gene is positively related to potential denitrification rates in an acidic forest soil (M. Y. Zhang et al., 2018). The reduction of N2O to N2 catalysed by nitrous oxide reductase (encoded by the nosZ gene) plays a vital role in mitigating N2O emissions (Liu et al., 2014; Nie et al., 2016). Therefore, the combination of soil N-transformation processes and functional gene abundances is essential to better explain the response mechanism of the soil N cycle to N additions, to explore the relationships between the abundances of soil N-related functional genes and N-transformation rates, and to assess the effects of N addition on soil N-related functional microbes.
Soil net N-transformation rates are thought to be primarily controlled by environmental factors, including temperature, precipitation, carbon to nitrogen (C : N) ratio, soil organic matter (SOM) content, soil texture and pH (Templer et al., 2005; Chen et al., 2017; Song et al., 2018). Importantly, the contents of soil organic carbon (SOC) and carbon to nitrogen (C : N) ratio are the key factors that determine soil net N dynamics in terrestrial ecosystems (Li et al., 2014; Liu et al., 2017). On the other hand, N inputs to forests could alter soil properties. For instance, elevated N deposition can result in soil acidification (Lu et al., 2014; Mao et al., 2017), a relatively lower soil C : N ratio and lower available P in the forest soil (Shi et al., 2018). The tropical forest soil itself is P limited and acidic; thus, it is also essential to assess the complex interactions between soil physiochemical characteristics and net N-transformation rates under N deposition.
Here, we investigated the effects of N addition on Rm, Rn, Rl, N2O emission and N-related functional gene abundance within 2 years using the in situ intact soil core incubation method in an acidic tropical forest. The main aims were to investigate (1) the effects of elevated N deposition on field-measured net N-transformation rates; (2) the effects of N deposition on the abundance of microbial functional genes associated with the N-transformation processes and (3) the relationships among functional gene abundance, N-transformation rates and environmental factors under elevated N deposition.
2.1 Study sites
The study was carried out in the Dinghushan Biosphere Reserve (DHSBR) (23∘10′ N, 112∘10′ E) in Guangdong Province of southern China. An experiment using a gradient of nitrogen addition was used to simulate N deposition in an evergreen broad-leaved forest with the age of about 110 years. The climate of this forest is considered a humid monsoon with an annual average temperature of 21 ∘C and a mean annual precipitation of 1927 mm (Mo et al., 2006; Zhao et al., 2011). The minimum monthly mean temperature in this study area is 12.6 ∘C in January, and the maximum monthly mean temperature is 28.0 ∘C in July (Mo et al., 2006). The elevation of this site ranges from 300 to 355 m above sea level. The major tree species of the study site are Castanopsis chinensis, Schima superba, Cryptocarya chinensis, and Randia canthioides. In this site, the wet season is concentrated from April to September (approximately 80 % of the annual rainfalls), and the dry season extends from October to March (approximately 20 % of the annual rainfalls). Wet N deposition measured as dissolved inorganic N in bulk precipitation was 34.6 and 31.6 kg N ha−1 yr−1 in 2004 and 2005, respectively (Fang et al., 2008). In addition, the soil type in this region is classified as strongly acidic lateritic red earth formed from sandstone with a pH below 4.0 (Mo et al., 2006; W. Zhang et al., 2008).
2.2 Experimental design
Four concentrations of NH4NO3 were applied: control (0), low N (LN, 35 kg N ha−1 yr−1), medium N (MN, 70 kg N ha−1 yr−1) and high N (HN, 105 kg N ha−1 yr−1), which were 1, 2, and 3 times the ambient wet N deposition rate, respectively. Twelve (four treatments × three replicates) experimental plots (15 m × 15 m per plot) were randomly scattered in the study area and established in October 2013; the plots were surrounded by buffer strips (>10 m wide) to avoid the disturbance of surface runoff and flow diffusion between adjacent plots. The corresponding dose of N (NH4NO3) solution (30 L) and an equal amount of water (without NH4NO3) were evenly sprayed over the N-treated and control plots, respectively, below the canopy using a knapsack sprayer (i.e. a low rate of 0.1 L m−2 was applied to avoid liquid effects) at the end of each month starting in September 2014.
2.3 Soil N transformations
Soil net mineralization, net nitrification and inorganic N leaching rates were determined nine times from September 2014 to October 2016 using the in situ resin-core incubation method (Reichmann et al., 2013; Chen et al., 2017). The installation of the incubation PVC tubes were done a couple of days before 24th of the month when N addition was applied. The nine incubations were scattered across 2 years from September 2014 to September 2016, with an interval of three months. The incubation period lasted for 30 d.
In each plot, six soil incubation sites were evenly distributed in uphill and downhill areas. At each incubation site, a pair of PVC tubes (5 cm in diameter and 17 cm in length) were inserted into the soil surface layer (10 cm depth) after the surface litter was removed. A resin bag containing 30 g ion exchange resin (cation exchange resin: anion resin = 1:2) was placed in the bottom of one PVC tube (accounting for approximately 2 cm of the PVC tube) under a 10 cm soil layer. The resin cores in the PVC tubes were incubated in situ for 30 d in the field prior to the collection of the soil samples and resin bags to measure the concentrations of soil -N and -N. The other PVC tube with a 10 cm soil core was taken immediately, and then the soils in the PVC tubes were mixed thoroughly (six total soil cores in each plot) into a composite soil sample for further analysis. Soil samples were divided into two parts. One part was passed through a 2 mm sieve and used to analyse the initial concentration of soil -N and -N, and a small part of the fresh soil was kept at −80 ∘C to extract soil DNA for quantifying the functional microorganisms. The other part was air-dried at room temperature, and then it was passed through a 100-mesh sieve to estimate the basic soil physicochemical properties. Soil net mineralization (Rm), net nitrification (Rn) and inorganic N leaching (Rl) rates were calculated according to the following formulas:
where ti and ti+1 are the beginning and end dates of each incubation period, respectively; and are the contents of soil -N before and after incubation, respectively, and and are the concentrations of soil -N before and after incubation, respectively (Li et al., 2018). and are the contents of -N and -N in the resin after 30 d of incubation, respectively.
In addition, the concentrations of -N and -N in the resin were used to calculate the ammonium and nitrate leaching rates, respectively. Soil N2O emissions were monitored using the closed chamber method, and N2O gas samples were taken twice in the middle and the end of each month across October 2014 to September 2016. The N2O concentrations was analysed with a gas chromatograph (Agilent 7890A, Agilent Technologies, USA) as previously described (Chen et al., 2017). The N2O efflux rate was calculated using the following equation:
where F represents the N2O flux (µg N m−2 h−1); ρ the density of N2O under standard conditions (mg L−1), V gas volume in the chamber (m3), A chamber coverage area (m2), P atmosphere pressure at the sampling time (Pa), P0 standard atmosphere pressure (Pa), T absolute temperature (K) at the sampling time, absolute temperature (K) under standard conditions and dC1∕dt the liner slope of gas concentration changes within the sampling time period. The annual rates of N2O emission (kg N ha−1 yr−1) after N addition were calculated by linear interpolation between sampling dates in the two observation years: October 2014 to September 2015 and October 2015 to September 2016.
2.4 Soil physiochemical properties
The soil organic carbon (SOC) was estimated using the external heating method with potassium dichromate (K2Cr2O7). To obtain the total nitrogen (TN) and total phosphorus (TP), semi-micro Kjeldahl digestion and molybdenum antimony colorimetric approaches were performed, respectively. The contents of soil -N and -N were detected with 1 M KCl extraction by indophenol-blue colorimetry and double wavelength (220 and 275 nm), respectively, using a spectrophotometer (UV-6000, China). Soil pH was measured by a pH meter with a glass electrode (Horiba F-71S, Japan) (soil : water ratio, 1 : 2.5 dry wt : v). Soil microbial carbon (MBC) and soil microbial nitrogen (MBN) were determined on a TOC analyser (Shimadzu TOC-VCSH Analyser) by the fumigation–extraction method (Vance et al., 1987) and calculated using the conversion factors of 0.45 and 0.54, respectively (Brookes et al., 1985; Joergensen et al., 2011).
2.5 Quantification of the abundances of soil functional genes
Soil DNA was extracted using a PowerSoil® DNA Isolation Kit (MOBIO Laboratories, Carlsbad, CA, USA). DNA concentrations were quantified on a Qubit 2.0 fluorometer (Life Technologies, Carlsbad, CA, USA). Subsequently, quantitative PCR was performed on an ABI 7500 CFX96 optical real-time detection system (Bio-Rad Laboratories, Inc., Hercules, CA, USA) to quantify the abundances of N-cycling functional genes, including AOA amoA and AOB amoA genes in nitrification and nirK and nosZ genes in denitrification. The pair primers of these functional genes are shown in Table S1 in the Supplement. The total volume (20 µL) of the reaction systems contained 10 µL SYBR® Premix Ex Taq™ (TaKaRa Biotech, Japan), 0.4 µL forward and 0.4 µL reverse primer, 0.4 µL Rox Reference Dye II (TaKaRa Biotech, Japan), 1 µL amplification template (genomic DNA) and 7.8 µL sterile ddH2O. The preparation of standard curves and the details of the amplification conditions were conducted as described in Table S2. The amplification efficiencies of qPCR ranged from 95.3 % to 103.0 %, and the R2 values of the calibration curves were ≥0.98.
2.6 Statistics
One-way analysis of variance (ANOVA) was used to compare the differences in inorganic N concentrations, soil N transformations, and soil functional gene abundances between control and N-treated plots at each sampling time. The least significant difference (LSD) test for multiple comparisons and two-way repeated measures ANOVA were performed to examine the effects of N additions on these variables over time using the SPSS Statistics software package (SPSS 18.0, SPSS Inc., Chicago, USA). Redundancy analysis (RDA) was conducted to determine the comprehensive relationships among soil physiochemical properties, functional gene abundance and N transformations using Canoco 5.0 (Wageningen University and Research, the Netherlands). The correlation coefficients of soil properties, soil N transformations and functional genes were calculated using PAST (version 2.16). The partial least squares path modelling (PLS-PM) was carried out to test the effects of inorganic N, soil conditions, microbial biomass and functional gene abundance on soil N-transformation rates (Rm, Rn, Rl and N2O emission) using the “plspm” package in R (version 3.3.3).
3.1 Soil properties and inorganic N contents
The soil C:N ratio in this study site ranged from 11.3 to 18.5, and the pH was between 3.7 and 3.9 (Table S3). The HN addition decreased the SOC, C:N ratio and pH by 14.1 %, 9.3 % and 1.4 %, respectively. The soil TN showed no significant difference between the control and N-treated plots after N addition (Table S3). The concentrations of MBC and MBN decreased obviously by 15.1 % and 14.5 %, respectively, in the HN treatment plots in the dry season (Table S3). The contents of soil -N and -N significantly increased with N addition (P<0.05, Fig. 1). Our results showed that the amounts of soil -N and -N in the MN and HN plots were significantly higher than those in the control plots. The mean value of -N accounted for 25.1 % of the mean total inorganic N, and the -N ∕ -N ratio ranged from 0.05 to 0.97. Over the entire study period, the mean soil -N contents in the LN, MN and HN treatment plots increased by 27.5 %, 38.3 % and 38.6 %, respectively. Similarly, the mean concentrations of -N in these three plots increased by 0.4 %, 29.3 % and 37.2 %, respectively.
3.2 Soil net N mineralization and nitrification rates
The results showed that in situ Rm and Rn significantly increased after one year of N addition in the MN and HN plots (P<0.05, Fig. 2a and b). However, there were no significant differences in both N-transformation rates between the control and N-treated plots during the first N-treated year (P>0.05). The range of in situ Rm (from 4.9 to 44.9 mg N kg−1 month−1) over the first year of N addition was obviously lower by approximately 50 % than the range (from 10.0 to 108.6 mg N kg−1 month−1) over the second year of N addition. In addition, the responses of Rm and Rn to N addition exhibited different seasonal patterns. The mean values of Rm in the LN, MN and HN plots in the wet season were 60.3 %, 18.5 %, and 50.2 % higher than those in the dry season over the second year of N addition, respectively. Similarly, the mean value of Rn in the wet season in these three N-treated plots was 1.5-, 1.2-, and 1.3-fold higher than those in the dry season within the same period of N addition. A repeated measures ANOVA indicated that N additions had significant effects on Rm and Rn, which also exhibited the significant time effects. However, there were no significant interaction effects between N and time in these two N processes (Table S4).
3.3 Inorganic N leaching and N2O emission
The HN addition significantly increased the ammonium-leaching rates (Fig. 3a), but the ammonium leaching rates accounted for only a small proportion (less than 20 %) in the total of Rl and were found to range from 0.08 to 10 mg N kg−1 month−1. After a 1-year period of N additions, the nitrate leaching rates significantly increased in the MN and HN treatment plots (P<0.05, Fig. 3b). The Rl was significantly correlated with the nitrate leaching rate (Fig. 3d, R=0.939, P<0.001), indicating that inorganic N leaching was predominantly determined by nitrate leaching. The mean values of Rl in the LN, MN, and HN treated plots in the wet season were 1.22, 0.56, and 1.11 times greater than those in the dry season, respectively (Fig. 3e). The addition of N significantly increased the annual N2O emission (Fig. 3f, P<0.05), showing increases of 18.3 %, 18.4 % and 77.7 % in the LN, MN and HN, respectively, in comparison to the control plots. In addition, a strong positive correlation was observed between the soil -N concentration and nitrate leaching rate in the wet season (R=0.63, P<0.001) (Table 1b). This finding suggested that the accumulation of -N content with N addition might accelerate N loss from the acidic forest soil. Repeated measures ANOVA showed that N additions had significant effects on N2O emission. However, the interaction effects between N and time were indistinctive (Table S4).
Table 1The linear correlation R and P values among soil properties, N-related functional genes and N-transformation processes in the dry (a) and wet (b) seasons. The R values are indicated in bold, and the P values are indicated in normal font.
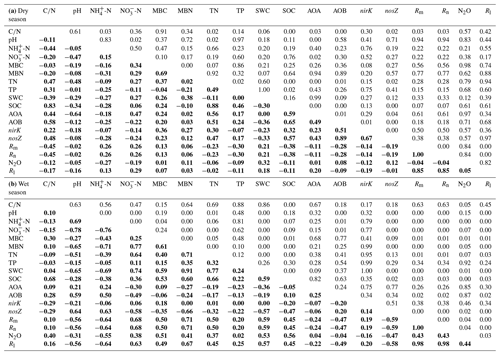
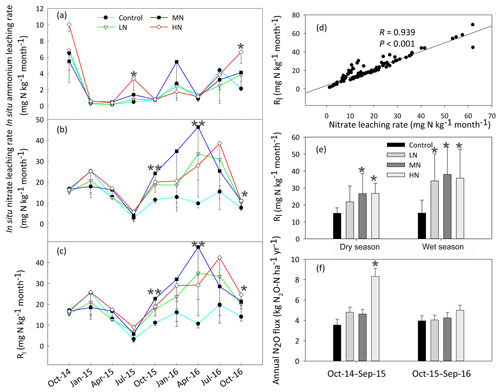
Figure 3Dynamics of the in situ -N (a), -N (b) and total inorganic N leaching rates (Rl) (c) in the soils at different samplings. (d) The correlation between the rates of nitrate leaching and inorganic nitrogen leaching (Rl). (e) The variation in Rl in the dry and wet season. (f) The rates of annual N2O emission with N addition. Bars represent standard errors of the mean (n=3). Significance levels are indicated by * P<0.05, P<0.01.
3.4 Soil microbial functional genes
As shown in Fig. 4a, the copy numbers of the archaeal AOA amoA gene ranged from 1.7×108 to 5.2×108 g−1 dry soil. Although AOA abundance showed no significant difference in all treatments, its mean value increased by 17.3 % and 7.5 % in the MN and HN plots, respectively, compared with the value in the control plots. AOA abundance showed a significantly negative correlation with soil pH (, P<0.01) and a positive correlation with -N content (R=0.47, P<0.05) in the dry season (Table 1a). However, the MN and HN additions significantly decreased the copy numbers of the AOB amoA gene (P<0.05 and P<0.01, respectively, Fig. 4b). In addition, AOA were more abundant than AOB in the acidic forest soils. The ratio of AOA : AOB abundance ranged from 9.5 to 191.2. However, the abundance of nirK genes significantly increased in the second year of HN addition (P<0.01, Fig. 4c). Initially, the abundance of nosZ genes decreased in the HN-treated plots compared with that in the control plots in January 2015 and January 2016 (P=0.057, Fig. 4d). However, the differences between both were weakened with the duration of N addition. Repeated measures ANOVA also indicated the interaction effects of N and time on soil functional gene abundance were not apparent except for nirK genes (Table S5).
3.5 Interactions among N-transformation rates, soil physicochemical properties and functional gene abundance
Redundancy analysis (RDA) was carried out to separately determine the relationship between the soil biotic and abiotic factors and the N-transformation rates for the dry seasons and wet seasons. RDA in the dry season was confirmed as unreliable because the P value of the RDA was >0.05 (data not shown). Linear correlation analysis showed that the C:N ratio had significant negative correlations with both the Rm and Rn (, P<0.05, Table 1a) but had positive relationships with the abundance of AOA amoA, AOB amoA and nosZ genes (R=0.44, P<0.05; R=0.58, P<0.01; R=0.48, P<0.05, respectively). In addition, no significant correlations were found between N-transformation rates and biotic factors in the dry season (Table 1a). In the wet season, the first two axes of the RDA explained 65.3 % of the total variance in all determined biotic and abiotic parameters and N-transformation rates of the soil samples (Fig. 5). The Rm, Rn and Rl had significantly positive correlations with the soil -N contents, MBN, MBC, soil water content (SWC), SOC and TN. In contrast, the above N-transformation rates had significantly negative relationships with soil pH and -N contents. Similarly, N2O emission was significantly positively correlated with the MBN, MBC, SWC, SOC and TN but significantly negatively correlated with the soil -N content. According to the above analysis, we found more complex relationships among the biotic and abiotic factors and N transformations in the wet season than in the dry season.
The PLS-PM was constructed to integrate the complex interrelationships among environmental factors, microbial biomass and soil N transformations in the wet season (Fig. 6). The results showed that inorganic N had positive direct effects on soil conditions (path coefficient = 0.78, P<0.001), microbial biomass (path coefficient = 0.11, P>0.05) and N transformations (path coefficient = 0.18, P>0.05). However, inorganic N had a negative direct effect on N-related functional gene abundance (path coefficient = −0.7, P<0.01). Soil conditions had a positive direct effect on microbial biomass (path coefficient = 0.75, P<0.001). The positive direct contributors to N transformations were inorganic N (path coefficient = 0.18, P>0.05) and microbial biomass (path coefficient = 0.44, P>0.05). In contrast, the negative direct effects on N transformations were soil conditions (path coefficient = −0.07, P>0.05) and N-related functional gene abundance (path coefficient = −0.37, P=0.09).
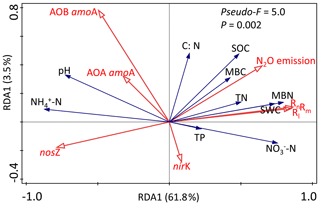
Figure 5Redundancy analysis (RDA) among environmental variables (-N, -N, pH, SOC, TN, C∕N ratio and SWC), functional genes (AOA amoA, AOB amoA, nirK and nosZ) and soil N-transformation rates (Rm, Rn, Rl and N2O emission) in the wet season. Values on the axes indicate the percentages of total variation explained by each axis.
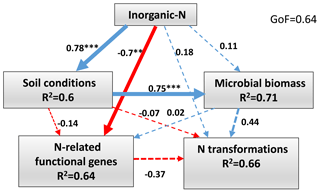
Figure 6Directed graph of the partial least squares path model (PLS-PM) of the inorganic N (-N and -N), soil conditions (pH, SOC, TN, C∕N ratio and SWC), microbial biomass (MBC and MBN) and the abundance of functional genes (AOA amoA, AOB amoA, nirK and nosZ) effects on soil N-transformation rates (Rm, Rn, Rl and N2O emission) in the wet season. Path coefficients and explained variability (R2) reflected in the width of the arrow were calculated after 1000 bootstraps. Blue and red arrows represent positive and negative effects, respectively. Solid arrows indicate P<0.05 and dashed arrows indicate P>0.05. The model was assessed using the goodness of fit (GoF). Significance levels are indicated by * P<0.05, P<0.01 and P<0.001.
4.1 Effects of N addition on N-transformation rates
In contrast to N-limited temperate forests, the N-rich tropical broadleaved forest soil in the DHSBR was considered to be N saturated (Fang et al., 2008). In our study, no significant differences of Rm, Rn and Rl were found in the control and N-treated plots during the first year of N addition, which could possibly be ascribed to plant uptake of mineral N and soil N retention (Fang et al., 2011; Gurmesa et al., 2016). However, Rm, Rn and Rl significantly increased in the MN and HN treatment plots in the second year of N addition (Figs. 2a, b and 3c). This result is in agreement with the hypothesis proposed earlier whereby once N input exceeds the total biotic demands, it will form a status of N saturation and subsequently promote N mineralization, nitrification, N loss through nitrate (-N) leaching and N2O emissions in boreal and temperate forest ecosystems (Aber et al., 1989, 1998). The strong increments of Rm, Rn and Rl under N additions lasted from October 2015 to July 2016 (Figs. 2a, b and 3c). These data provide evidence of the stimulating effects of N inputs on net N-transformation processes (i.e. in situ Rm and Rn) in tropical forests. In a recent laboratory incubation study using the 15N dilution method with the soil samples collected from the same experimental plots, Han et al. (2018) found that the N additions stimulated the gross N mineralization rate but decreased the gross N immobilization rate in the second-year soil samples. Therefore, the increased net N mineralization rate observed in the second year of this study might be due to the simulative N effect on gross N mineralization and the suppressive effect on gross immobilization rate. However, the filed-measured net N mineralization rate in this study cannot be directly and quantitatively linked to the laboratory-measured gross N mineralization and immobilization rates in the study of Han et al. (2018), since they are inherently different measures of N-transformation rates (Cheng et al., 2019). Further studies combining field-measured net N-transformation rates, laboratory-incubation-measured net N-transformation rates (as in Lovett et al., 2004) and 15N dilution-measured gross N-transformation rates may provide a more mechanistic understanding to the impacts of N addition on soil N-transformation processes.
The significant increases in Rm and Rn in the second year of N addition are consistent with the result of the previous study showing significant increases of the two N-transformation processes after a 3-year N addition (Fang et al., 2011). However, significant decreases in Rm and Rn after a 6-year N addition were previously demonstrated in the adjacent tropical forest (Chen et al., 2016). The different effects of short-term and long-term N addition on Rm and Rn are possibly caused by the reasons below. First, long-term N additions could lead to high amounts of -N accumulation relative to short-term N additions, which may form high osmotic potential and ion toxicity and directly affect soil microorganisms (Wang et al., 2018a). Second, long-term N addition results in a lower soil pH (Lu et al., 2014) and an increase in Al3+ content which is toxic to soil microorganisms (He et al., 2012). Third, long-term N deposition has negative impacts on protein depolymerization (Chen et al., 2018), which is considered a rate-limiting step of organic N mineralization (Jan et al., 2009; Mooshammer et al., 2014).
Although the rates of nitrate leaching measured under the 10 cm soil layer might overestimate the N loss attributed to plant uptake below this layer, the result is in agreement with the previous studies of substantial nitrate leaching under N deposition (Fang et al., 2009a; Chen et al., 2016). The inorganic N leaching (Rl) mainly resulted from nitrate leaching (Fig. 3a, b, c and d), because the negatively charged -N is easier to lose from the soil, while -N tends to be taken by plants in acidic forest soils (Fang et al., 2011; Chen et al., 2017). Hall and Matson (1999) found that N2O emissions were higher in the P-limited tropical forest than in the N-limited forest. In this study, the mean rates of soil N2O emissions in the control plots were 40.4±6.5 and 45.1±5.7 µg N2O-N m−2 h−1 in the first 2 years after N additions, respectively (Table S6), which were obviously higher than the results of 29.3±1.6 µg N2O-N m−2 h−1 reported by W. Zhang et al. (2008), indicating that N2O emission rates had increased over the past 10 years in the forests of DHSBR. In addition, the rates of N2O emissions (95.0±9.0 µg N2O-N m−2 h−1) in the HN treatment plots were significantly higher than those in the LN, MN and control plots, indicating that the soil N2O emission flux was dependent on the N-addition gradients (W. Zhang et al., 2008; Fang et al., 2011; Chen et al., 2016).
4.2 Response of microbial functional gene abundances to N additions
AOA play a more important role than do AOB in ammonia oxidation of acidic soils (Zhang et al., 2012; Tang et al., 2016). Similar to the results of a previous study performed in the old-growth broadleaf forest at DHSBR (Isobe et al., 2012), AOA were also more abundant than AOB in our younger broadleaf forest. The two ammonia oxidizers showed different response patterns to N additions; the abundance of AOA did not differ statistically significantly among the four N treatments but showed an increasing trend as N-addition level increased (Fig. 4a); contrastingly the abundance of AOB was significantly decreased by the MN and HN treatments (Fig. 4b). These results are similar to those found in a previous study in which a 6-year N input increased AOA abundance but decreased AOB abundance in an acidic subtropical forest soil (Shi et al., 2018). The reason for the increased AOA but decreased AOB abundance under N addition might be ascribed to the decreased soil pH and the lowered ammonia (NH3) availability. Previous studies have shown that the AOA : AOB ratio increases with decreasing soil pH (He et al., 2007; Shen et al., 2008; Yao et al., 2011), mainly because AOA are well adapted to strongly acidic soil conditions, but AOB tend to be more abundant in neutral or slightly alkaline soils over all terrestrial ecosystems (Nicol et al., 2008; Hu et al., 2013; Wang et al., 2019). Furthermore, AOA are often more sensitive to N enrichment (Ning et al., 2015) and more competitive for the direct substrate (NH3) than AOB (He et al., 2012; Shi et al., 2018). The abundances of nirK denitrifiers have been found positively related to potential denitrification (M. Y. Zhang et al., 2018; Tang et al., 2019). Here, we found that HN additions initially decreased nirK gene abundance in the first year of N addition but significantly increased nirK gene abundance in the second year of N addition (Fig. 4b), which is possibly ascribed to the accumulation of soil -N and the subsequent acceleration of denitrification. The reduction of N2O to N2 was reported to be regulated by the abundance of nosZ-harbouring denitrifiers (Levy-Booth et al., 2014). In our study, the HN addition decreased nosZ gene abundance in the earlier stage of N addition, but a decrease in the difference was also observed with the duration of N addition. The decrease in the difference in nosZ gene abundance between the control and N-treated plots with time was possibly attributed to the tendency of microbial adaption to N addition. Furthermore, N2O emissions had significantly negative correlation with nosZ gene abundance in the wet season (Table 1b). It supports the previous findings that decreased nosZ gene abundance with N addition is a major factor causing low N2O consumption and high N2O emissions in the acidic forest soil (W. Zhang et al., 2008). These variations in nirK and nosZ gene abundances and the greater abundance of the nirK than the nosZ gene (Fig. 4c and d) could explain the significant increase in N2O emissions with N additions in the tropical forest soil (Han et al., 2018). In contrast, an opposite pattern with a higher nosZ gene abundance, but a lower total nirK and nirS gene abundance was found in a Masson pine forest soil with low N2O emissions with a mean annual temperature of 17 ∘C and a mean annual precipitation of 1200–1400 mm (Y. Li et al., 2019), suggesting that nirS, nirK and nosZ denitrifiers are critical in regulating N2O emission in forest ecosystems. Therefore, the nirS gene abundance is also very important in mediating N2O emission (Chen et al., 2019). In addition, soil water contents exhibited a significantly negative relationship with the abundance of the nosZ gene in the wet season (Table 1b), which explains the higher N2O emission in the wet season than in the dry season (Fu et al., 2015).
4.3 Seasonal variations in N transformations under N additions
Seasonal patterns were more obvious for the N transformations in the second year of N additions. The Rm, Rn and Rl were apparently higher in the wet season than in the dry season (Figs. 2c, d and 3e), suggesting that soil temperature and moisture were the critical environmental factors affecting N transformations (Chen et al., 2017; Li et al., 2018). Similar seasonal patterns have been documented in previous studies (X. L. Zhang et al., 2008; Contosta et al., 2011; Li et al., 2014). In the dry season, the HN addition decreased the MBC and MBN by 15.1 % and 14.5 % respectively (Table S3), and the low temperature and precipitation suppressed the microbial biomass and activity and then depressed the N mineralization (Contosta et al., 2011; Chen et al., 2017). Our results indicate that the lower soil C:N ratio with N enrichment was the dominant factor that increased Rm and Rn, and subsequently led to higher N losses (N2O emission and nitrate leaching) in the dry season (Table 1a). In contrast, the factors controlling the processes of N transformation in the wet season were more complicated, with microbial biomass and SWC being the most important ones (Fig. 5) over soil pH, inorganic N content, TN, SOC and N-related functional gene abundance (Table 1b). The higher soil microbial biomass and SWC in the wet season could facilitate N mineralization and nitrification, and then cause larger nitrate leaching.
4.4 The interactions between soil N transformations and abiotic and biotic conditions
In the dry season, the variations in Rm, Rn and Rl exhibited significant negative correlations with the soil C:N ratio (Table 1a), suggesting that the C:N ratio was a dominant factor determining soil N dynamics (Fang et al., 2011; Liu et al., 2017). In this study, the HN addition decreased the soil C:N ratio, which was consistent with the results of a previous study in an acidic forest soil (Shi et al., 2018). Significant positive correlations between the C:N ratios and the abundances of AOA, AOB and nosZ-N2O reducers were also observed (Table 1a), indicating that a low C:N ratio had a negative effect on N-related functional microbes. AOA abundance was positively correlated with soil -N concentration, which was in accordance with the previous studies (Hu et al., 2013; Tang et al., 2016). In addition, AOA abundance was negatively correlated with soil pH (Y. Li et al., 2019), indicating that AOA could adapt to the strong acidic tropical forest soil.
In the wet season, AOB amoA and nosZ gene abundances were positively related to soil -N contents and pH but negatively related to soil -N contents (Fig. 5 and Table 1b), indicating that the lower pH and accumulation of soil -N with N addition might result in decreases in the AOB amoA and nosZ gene abundances. However, we found no significant relationship between AOA abundance and Rn during the 2-year study period (Table 1a and b), which is inconsistent with the results of Isobe et al. (2012), who found a significant correlation between AOA amoA abundance and gross nitrification rate in an adjacent tropical forest soil. This possibly because of the different N-transformation rates measured in the two studies: net N nitrification rate measured using the in situ incubation method in our study and gross N nitrification rate measured using the 15N dilution method in the study of Isobe et al. (2012). In addition, the findings also indicate that Rm, Rn and Rl were significantly and negatively correlated with soil -N content (Fig. 5), and a possible explanation is that -N could have a negative feedback on soil N mineralization (Geisseler et al., 2010; M. Y. Zhang et al., 2018). Interestingly, Rm, Rn and Rl were significantly and negatively correlated with soil pH, which contrasted with the results of previous studies (Fu et al., 1987; Kemmitt et al., 2006). The most reasonable explanation is that soil pH has a negative correlation with soil N transformation in strongly acidic soils (pH < 4.0), which is likely due to the highest nitrification rates existing in the soils with lower pH (Booth et al., 2005).
The PLS-PM showed that the inorganic N had significantly negative direct effects on the N-related functional gene abundance (Fig. 6), suggesting that the functional microorganisms were more sensitive to N addition, and ongoing N deposition had significant negative effects on soil functional microbes (T. A. Zhang et al., 2018). However, these negative effects of N addition on microbial gene abundance did not cause significant negative effects on net N transformations in the study period, which is possibly explained by the microbial function redundancy or buffer capacity of the acidic forest soil. In addition, we found that the microbial biomass was the dominant factor driving net N transformations in the wet season (Fig. 6), suggesting that microbes played a critical role in driving the processes of N transformation (Z. L. Li et al., 2019). However, it was previously found that a 13-year N addition significantly decreased the MBC and MBN in adjacent forest soil (Wang et al., 2018b), implying that chronic N deposition would have a negative effect on soil N transformations.
The addition of N increased the in situ net mineralization, net nitrification, inorganic N leaching rate and N2O emission during the short term, which supported the traditional N-saturation hypothesis. To some extent, the alterations of functional gene abundance with N additions were related to the corresponding processes of N transformation. The variations in Rm, Rn and Rl exhibited different seasonal patterns. They were higher in the wet season than in the dry season. The C:N ratio was the dominant driving factor of N transformations in the dry season, while the biotic factors (microbial biomass) played an important role in accelerating N transformations in the wet season. According to the PLS-PM analysis, N additions had negative effects on the abundance of N-related functional genes in the dry season, which implies that chronic N deposition poses a potential risk to forest ecosystem functions. More attention should be focused on the combination of soil nitrogen cycling processes and functional microorganisms to long-term continuous N inputs in forest ecosystems and the strategies to alleviate the negative effects of elevated N deposition on soil microbial function.
All the relevant data are presented in the paper and Supplement.
The supplement related to this article is available online at: https://doi.org/10.5194/bg-16-4277-2019-supplement.
WS designed the study, planned the field experiments and obtained research funding. YN carried out the experiment and analysed the data. YN, WS and MW wrote the paper. XH provided the N2O observations and guidance on their interpretation. JC helped in the field experiments of N transformation (in situ Rm, Rn and Rl) and provided part of the data. All the authors provided feedback and gave constructive suggestions on the paper.
The authors declare that they have no conflict of interest.
We would like to thank for Wei Zhang, Feng Huang and Xiangping Tan for their assistance in soil sampling and data collection. We thank Suping Liu, Zhuang Ni, Wenjuan Wang and Shaoyun Lv for their helpful assistance in the laboratory analysis. This study was financially supported by the National Natural Science Foundation of China (31600382, 31425005, 31290222 and 31600430), the National Ten Thousand Talents Program, the Guangdong Province Baiqianwan Talents Program, the open project of Guangdong Provincial Key Laboratory of Plant Resources (2017B030314023) and the National Key Research and Development Program of China (2017YFD0202100).
This research has been supported by the National Natural Science Foundation of China (grant nos. 31600382, 31425005, 31290222 and 31600430).
This paper was edited by Zhongjun Jia and reviewed by two anonymous referees.
Aber, J., McDowell, W., Nadelhoffer, K., Magill, A., Berntson, G., Kamakea, M., McNulty, S., Currie, W., Rustad, L., and Fernandez, I.: Nitrogen saturation in temperate forest ecosystems – hypotheses revisited, Bioscience, 48, 921–934, https://doi.org/10.2307/1313296, 1998.
Aber, J. D., Nadelhoffer, K. J., Steudler, P., and Melillo, J. M.: Nitrogen saturation in northern forest ecosystems, Bioscience, 39, 378–386, https://doi.org/10.2307/1311067, 1989.
Booth, M. S., Stark, J. M., and Rastetter, E.: Controls on nitrogen cycling in terrestrial ecosystems: a synthetic analysis of literature data, Ecol. Monogr., 75, 139–157, https://doi.org/10.1890/04-0988, 2005.
Braker, G., Zhou, J. Z., Wu, L. Y., Devol, A. H., and Tiedje, J. M.: Nitrite reductase genes (nirK and nirS) as functional markers to investigate diversity of denitrifying bacteria in Pacific northwest marine sediment communities, Appl. Environ. Microb., 66, 2096–2104, https://doi.org/10.1128/AEM.66.5.2096-2104.2000, 2000.
Brookes, P. C., Landman, A., Pruden, G., and Jenkinson, D. S.: Chloroform fumigation and the release of soil nitrogen: a rapid direct extraction method to measure microbial biomass nitrogen in soil, Soil Biol. Biochem., 17, 837–842, https://doi.org/10.1016/0038-0717(85)90144-0, 1985.
Chen, H., Gurmesa, G. A., Zhang, W., Zhu, X. M., Zheng, M. H., Mao, Q. G., Zhang, T., and Mo, J. M.: Nitrogen saturation in humid tropical forests after 6 years of nitrogen and phosphorus addition: hypothesis testing, Funct. Ecol., 30, 305–313, https://doi.org/10.1111/1365-2435.12475, 2016.
Chen, H., Li, D. J., Zhao, J., Xiao, K. C., and Wang, K. L.: Effects of nitrogen addition on activities of soil nitrogen acquisition enzymes: a meta-analysis, Agr. Ecosyst. Environ., 252, 126–131, https://doi.org/10.1016/j.agee.2017.09.032, 2018.
Chen, J., Xiao, G., Kuzyakov, Y., Jenerette, G. D., Ma, Y., Liu, W., Wang, Z., and Shen, W.: Soil nitrogen transformation responses to seasonal precipitation changes are regulated by changes in functional microbial abundance in a subtropical forest, Biogeosciences, 14, 2513–2525, https://doi.org/10.5194/bg-14-2513-2017, 2017.
Chen, J., Kuzyakov, Y., Jenerette, G. D., Xiao, G., Liu, W., Wang, Z., and Shen, W.: Intensified precipitation seasonality reduces soil inorganic N content in a subtropical forest: greater contribution of leaching loss than N2O emissions, J. Geophys. Res.-Biogeo., 124, 494–508, https://doi.org/10.1029/2018jg004821, 2019.
Chen, Z., Luo, X. Q., Hu, R. G., Wu, M. N., Wu, J. S., and Wei, W. X.: Impact of long-term fertilization on the composition of denitrifier communities based on nitrite reductase analyses in a paddy soil, Microbiol. Ecol., 60, 850–861, https://doi.org/10.1007/s00248-010-9700-z, 2010.
Cheng, Y., Wang, J., Chang, S. X., Cai, Z. C., Müller, C., and Zhang, J. B.: Nitrogen deposition affects both net and gross soil nitrogen transformations in forest ecosystems: a review, Environ. Pollut., 244, 608–616, https://doi.org/10.1016/j.envpol.2018.10.054, 2019.
Contosta, A. R., Frey, S. D., and Cooper, A. B.: Seasonal dynamics of soil respiration and N mineralization in chronically warmed and fertilized soils, Ecosphere, 2, 21, https://doi.org/10.1890/es10-00133.1, 2011.
Corre, M. D., Veldkamp, E., Arnold, J., and Wright, S. J.: Impact of elevated N input on soil N cycling and losses in old-growth lowland and montane forests in Panama, Ecology, 91, 1715–1729, https://doi.org/10.1890/09-0274.1, 2010.
Fang, H. J., Yu, G. R., Cheng, S. L., Zhu, T. H., Zheng, J. J., Mo, J. M., Yan, J. H., and Luo, Y. Q.: Nitrogen-15 signals of leaf-litter-soil continuum as a possible indicator of ecosystem nitrogen saturation by forest succession and N loads, Biogeochemistry, 102, 251–263, https://doi.org/10.1007/s10533-010-9438-1, 2011.
Fang, Y. T., Gundersen, P., Mo, J. M., and Zhu, W. X.: Input and output of dissolved organic and inorganic nitrogen in subtropical forests of South China under high air pollution, Biogeosciences, 5, 339–352, https://doi.org/10.5194/bg-5-339-2008, 2008.
Fang, Y. T., Gundersen, P., Mo, J. M., and Zhu, W. X.: Nitrogen leaching in response to increased nitrogen inputs in subtropical monsoon forests in southern China, Forest Ecol. Manag., 257, 332–342, https://doi.org/10.1016/j.foreco.2008.09.004, 2009a.
Fang, Y. T., Yoh, M., Mo, J. M., Gundersen, P., and Zhou, G. Y.: Response of nitrogen leaching to nitrogen deposition in disturbed and mature forests of southern China, Pedosphere, 19, 111–120, https://doi.org/10.1016/s1002-0160(08)60090-9, 2009b.
Fu, M. H., Xu, X. C., and Tabatabai, M. A.: Effect of pH on nitrogen mineralization in crop-residue-treated soils, Biol. Fert. Soils, 5, 115–119, 1987.
Fu, X., Liu, X., Li, Y., Shen, J., Wang, Y., Zou, G., Li, H., Song, L., and Wu, J.: Wet-season spatial variability in N2O emissions from a tea field in subtropical central China, Biogeosciences, 12, 3899–3911, https://doi.org/10.5194/bg-12-3899-2015, 2015.
Galloway, J. N., Townsend, A. R., Erisman, J. W., Bekunda, M., Cai, Z. C., Freney, J. R., Martinelli, L. A., Seitzinger, S. P., and Sutton, M. A.: Transformation of the nitrogen cycle: recent trends, questions, and potential solutions, Science, 320, 889–892, https://doi.org/10.1126/science.1136674, 2008.
Gao, W. L., Kou, L., Yang, H., Zhang, J. B., Müller, C., and Li, S. G.: Are nitrate production and retention processes in subtropical acidic forest soils responsive to ammonium deposition?, Soil Biol. Biochem., 100, 102–109, https://doi.org/10.1016/j.soilbio.2016.06.002, 2016.
Geisseler, D., Horwath, W. R., Joergensen, R. G., and Ludwig, B.: Pathways of nitrogen utilization by soil microorganisms – a review, Soil Biol. Biochem., 42, 2058–2067, https://doi.org/10.1016/j.soilbio.2010.08.021, 2010.
Gundersen, P., Emmett, B. A., Kjonaas, O. J., Koopmans, C. J., and Tietema, A.: Impact of nitrogen deposition on nitrogen cycling in forests: a synthesis of NITREX data, Forest Ecol. Manage., 101, 37–55, https://doi.org/10.1016/s0378-1127(97)00124-2, 1998.
Gurmesa, G. A., Lu, X. K., Gundersen, P., Mao, Q. G., Zhou, K. J., Fang, Y. T., and Mo, J. M.: High retention of 15N-labeled nitrogen deposition in a nitrogen saturated old-growth tropical forest, Glob. Change Biol., 22, 3608–3620, https://doi.org/10.1111/gcb.13327, 2016.
Hall, S. J. and Matson, P. A.: Nitrogen oxide emissions after nitrogen additions in tropical forests, Nature, 400, 152–155, https://doi.org/10.1038/22094, 1999.
Hall, S. J. and Matson, P. A.: Nutrient status of tropical rain forests influences soil N dynamics after N additions, Ecol. Monogr., 73, 107–129, https://doi.org/10.1890/0012-9615(2003)073[0107:NSOTRF]2.0.CO;2, 2003.
Han, X. G., Shen, W. J., Zhang, J. B., and Müller, C.: Microbial adaptation to long-term N supply prevents large responses in N dynamics and N losses of a subtropical forest, Sci. Total Environ., 626, 1175–1187, https://doi.org/10.1016/j.scitotenv.2018.01.132, 2018.
He, J. Z., Shen, J. P., Zhang, L. M., Zhu, Y. G., Zheng, Y. M., Xu, M. G., and Di, H. J.: Quantitative analyses of the abundance and composition of ammonia-oxidizing bacteria and ammonia-oxidizing archaea of a Chinese upland red soil under long-term fertilization practices, Environ. Microbiol., 9, 2364–2374, https://doi.org/10.1111/j.1462-2920.2007.01358.x, 2007.
He, J. Z., Hu, H. W., and Zhang, L. M.: Current insights into the autotrophic thaumarchaeal ammonia oxidation in acidic soils, Soil Biol. Biochem., 55, 146–154, https://doi.org/10.1016/j.soilbio.2012.06.006, 2012.
Hietz, P., Turner, B. L., Wanek, W., Richter, A., Nock, C. A., and Wright, S. J.: Long-term change in the nitrogen cycle of tropical forests, Science, 334, 664–666, https://doi.org/10.1126/science.1211979, 2011.
Hu, H. W., Zhang, L. M., Dai, Y., Di, H. J., and He, J. Z.: pH-dependent distribution of soil ammonia oxidizers across a large geographical scale as revealed by high-throughput pyrosequencing, J. Soils Sediments, 13, 1439–1449, https://doi.org/10.1007/s11368-013-0726-y, 2013.
Isobe, K., Koba, K., Suwa, Y., Ikutani, J., Fang, Y. T., Yoh, M., Mo, J. M., Otsuka, S., and Senoo, K.: High abundance of ammonia-oxidizing archaea in acidified subtropical forest soils in southern China after long-term N deposition, FEMS Microbiol. Ecol., 80, 193–203, https://doi.org/10.1111/j.1574-6941.2011.01294.x, 2012.
Jan, M. T., Roberts, P., Tonheim, S. K., and Jones, D. L.: Protein breakdown represents a major bottleneck in nitrogen cycling in grassland soils, Soil Biol. Biochem., 41, 2272–2282, https://doi.org/10.1016/j.soilbio.2009.08.013, 2009.
Joergensen, R. G., Wu, J., and Brookes, P. C.: Measuring soil microbial biomass using an automated procedure, Soil Biol. Biochem., 43, 873–876, https://doi.org/10.1016/j.soilbio.2010.09.024, 2011.
Kemmitt, S. J., Wright, D., Goulding, K. W. T., and Jones, D. L.: pH regulation of carbon and nitrogen dynamics in two agricultural soils, Soil Biol. Biochem., 38, 898–911, https://doi.org/10.1016/j.soilbio.2005.08.006, 2006.
Koehler, B., Corre, M. D., Veldkamp, E., Wullaert, H., and Wright, S. J.: Immediate and long-term nitrogen oxide emissions from tropical forest soils exposed to elevated nitrogen input, Glob. Change Biol., 15, 2049–2066, https://doi.org/10.1111/j.1365-2486.2008.01826.x, 2009.
Levy-Booth, D. J., Prescott, C. E., and Grayston, S. J.: Microbial functional genes involved in nitrogen fixation, nitrification and denitrification in forest ecosystems, Soil Biol. Biochem., 75, 11–25, https://doi.org/10.1016/j.soilbio.2014.03.021, 2014.
Li, M., Zhou, X. H., Zhang, Q. F., and Cheng, X. L.: Consequences of afforestation for soil nitrogen dynamics in central China, Agr. Ecosyst. Environ., 183, 40–46, https://doi.org/10.1016/j.agee.2013.10.018, 2014.
Li, X. J., Yang, H. T., Shi, W. L., Li, Y. F., and Guo, Q.: Afforestation with xerophytic shrubs accelerates soil net nitrogen nitrification and mineralization in the Tengger Desert, Northern China, Catena, 169, 11–20, https://doi.org/10.1016/j.catena.2018.05.026, 2018.
Li, Y., Chen, Z., He, J. Z., Wang, Q., Shen, C. C., and Ge, Y.: Ectomycorrhizal fungi inoculation alleviates simulated acid rain effects on soil ammonia oxidizers and denitrifiers in Masson pine forest, Environ. Microbiol., 21, 299–313, https://doi.org/10.1111/1462-2920.14457, 2019.
Li, Z. L., Tian, D. S., Wang, B. X., Wang, J. S., Wang, S., Chen, H. Y. H., Xu, X. F., Wang, C. H., He, N. P., and Niu, S. L.: Microbes drive global soil nitrogen mineralization and availability, Glob. Change Biol., 25, 1078–1088, https://doi.org/10.1111/gcb.14557, 2019.
Liu, B. B., Frostegard, A., and Bakken, L. R.: Impaired reduction of N2O to N2 in acid soils is due to a posttranscriptional interference with the expression of nosZ, mBio, 5, 10, https://doi.org/10.1128/mBio.01383-14, 2014.
Liu, X. J., Zhang, Y., Han, W. X., Tang, A. H., Shen, J. L., Cui, Z. L., Vitousek, P., Erisman, J. W., Goulding, K., Christie, P., Fangmeier, A., and Zhang, F. S.: Enhanced nitrogen deposition over China, Nature, 494, 459–462, https://doi.org/10.1038/nature11917, 2013.
Liu, Y., Wang, C. H., He, N. P., Wen, X. F., Gao, Y., Li, S. G., Niu, S. L., Butterbach-Bahl, K., Luo, Y. Q., and Yu, G. R.: A global synthesis of the rate and temperature sensitivity of soil nitrogen mineralization: latitudinal patterns and mechanisms, Glob. Change Biol., 23, 455–464, https://doi.org/10.1111/gcb.13372, 2017.
Lohse, K. A. and Matson, P.: Consequences of nitrogen additions for soil losses from wet tropical forests, Ecol. Appl., 15, 1629–1648, https://doi.org/10.1890/03-5421, 2005.
Lovett, G. M., Weathers, K. C., Arthur, M. A., and Schultz, J. C.: Nitrogen cycling in a northern hardwood forest: do species matter?, Biogeochemistry, 67, 289–308, https://doi.org/10.1023/B:BIOG.0000015786.65466.f5, 2004.
Lu, X. K., Mao, Q. G., Gilliam, F. S., Luo, Y. Q., and Mo, J. M.: Nitrogen deposition contributes to soil acidification in tropical ecosystems, Glob. Change Biol., 20, 3790–3801, https://doi.org/10.1111/gcb.12665, 2014.
Mao, Q. G., Lu, X. K., Zhou, K. J., Chen, H., Zhu, X. M., Mori, T. K., and Mo, J. M.: Effects of long-term nitrogen and phosphorus additions on soil acidification in an N-rich tropical forest, Geoderma, 285, 57–63, https://doi.org/10.1016/j.geoderma.2016.09.017, 2017.
Mo, J. M., Brown, S., Xue, J. H., Fang, Y. T., and Li, Z. A.: Response of litter decomposition to simulated N deposition in disturbed, rehabilitated and mature forests in subtropical China, Plant Soil, 282, 135–151, https://doi.org/10.1007/s11104-005-5446-7, 2006.
Mooshammer, M., Wanek, W., Hammerle, I., Fuchslueger, L., Hofhansl, F., Knoltsch, A., Schnecker, J., Takriti, M., Watzka, M., Wild, B., Keiblinger, K. M., Zechmeister-Boltenstern, S., and Richter, A.: Adjustment of microbial nitrogen use efficiency to carbon: nitrogen imbalances regulates soil nitrogen cycling, Nat. Commun., 5, 7, https://doi.org/10.1038/ncomms4694, 2014.
Nave, L. E., Vance, E. D., Swanston, C. W., and Curtis, P. S.: Impacts of elevated N inputs on north temperate forest soil C storage, C/N, and net N-mineralization, Geoderma, 153, 231–240, https://doi.org/10.1016/j.geoderma.2009.08.012, 2009.
Nicol, G. W., Leininger, S., Schleper, C., and Prosser, J. I.: The influence of soil pH on the diversity, abundance and transcriptional activity of ammonia oxidizing archaea and bacteria, Environ. Microbiol., 10, 2966–2978, https://doi.org/10.1111/j.1462-2920.2008.01701.x, 2008.
Nie, Y. X., Li, L., Isoda, R., Wang, M. C., Hatano, R., and Hashidoko, Y.: Physiological and genotypic characteristics of nitrous oxide (N2O)-emitting Pseudomonas species isolated from dent corn Andisol farmland in Hokkaido, Japan, Microbes Environ., 31, 93–103, https://doi.org/10.1264/jsme2.ME15155, 2016.
Ning, Q. S., Gu, Q., Shen, J. P., Lv, X. T., Yang, J. J., Zhang, X. M., He, J. Z., Huang, J. H., Wang, H., Xu, Z. H., and Han, X. G.: Effects of nitrogen deposition rates and frequencies on the abundance of soil nitrogen-related functional genes in temperate grassland of northern China, J. Soils Sediments, 15, 694–704, https://doi.org/10.1007/s11368-015-1061-2, 2015.
Ollivier, J., Töwe, S., Bannert, A., Hai, B., Kastl, E.-M., Meyer, A., Su, M. X., Kleineidam, K., and Schloter, M.: Nitrogen turnover in soil and global change, FEMS Microbiol. Ecol., 78, 3–16, https://doi.org/10.1111/j.1574-6941.2011.01165.x, 2011.
Patel, K. F. and Fernandez, I. J.: Nitrogen mineralization in O horizon soils during 27 years of nitrogen enrichment at the Bear Brook Watershed in Maine, USA, Environ. Monit. Assess., 190, 563, https://doi.org/10.1007/s10661-018-6945-3, 2018.
Petersen, D. G., Blazewicz, S. J., Firestone, M., Herman, D. J., Turetsky, M., and Waldrop, M.: Abundance of microbial genes associated with nitrogen cycling as indices of biogeochemical process rates across a vegetation gradient in Alaska, Environ. Microbiol., 14, 993–1008, https://doi.org/10.1111/j.1462-2920.2011.02679.x, 2012.
Reichmann, L. G., Sala, O. E., and Peters, D. P. C.: Water controls on nitrogen transformations and stocks in an arid ecosystem, Ecosphere, 4, 1–17, https://doi.org/10.1890/es12-00263.1, 2013.
Shen, J. P., Zhang, L. M., Zhu, Y. G., Zhang, J. B., and He, J. Z.: Abundance and composition of ammonia-oxidizing bacteria and ammonia-oxidizing archaea communities of an alkaline sandy loam, Environ. Microbiol., 10, 1601–1611, https://doi.org/10.1111/j.1462-2920.2008.01578.x, 2008.
Shi, X. Z., Hu, H. W., Wang, J. Q., He, J. Z., Zheng, C. Y., Wan, X. H., and Huang, Z. Q.: Niche separation of comammox Nitrospira and canonical ammonia oxidizers in an acidic subtropical forest soil under long-term nitrogen deposition, Soil Biol. Biochem., 126, 114–122, https://doi.org/10.1016/j.soilbio.2018.09.004, 2018.
Song, Y. Y., Song, C. C., Hou, A. X., Ren, J. S., Wang, X. W., Cui, Q., and Wang, M. Q.: Effects of temperature and root additions on soil carbon and nitrogen mineralization in a predominantly permafrost peatland, Catena, 165, 381–389, https://doi.org/10.1016/j.catena.2018.02.026, 2018.
Tang, Y. C., Zhang, X. Y., Li, D. D., Wang, H. M., Chen, F. S., Fu, X. L., Fang, X. M., Sun, X. M., and Yu, G. R.: Impacts of nitrogen and phosphorus additions on the abundance and community structure of ammonia oxidizers and denitrifying bacteria in Chinese fir plantations, Soil Biol. Biochem., 103, 284–293, https://doi.org/10.1016/j.soilbio.2016.09.001, 2016.
Tang, Y. G., Yu, G. R., Zhang, X. Y., Wang, Q. F., Tian, D. S., Tian, J., Niu, S. L., and Ge, J. P.: Environmental variables better explain changes in potential nitrification and denitrification activities than microbial properties in fertilized forest soils, Sci. Total Environ., 647, 653–662, https://doi.org/10.1016/j.scitotenv.2018.07.437, 2019.
Templer, P. H., Groffman, P. M., Flecker, A. S., and Power, A. G.: Land use change and soil nutrient transformations in the Los Haitises region of the Dominican Republic, Soil Biol. Biochem., 37, 215–225, https://doi.org/10.1016/j.soilbio.2004.07.031, 2005.
Tian, P., Zhang, J. B., Muller, C., Cai, Z. C., and Jin, G. Z.: Effects of six years of simulated N deposition on gross soil N transformation rates in an old-growth temperate forest, J. Forest Res., 29, 647–656, https://doi.org/10.1007/s11676-017-0484-6, 2018.
Vance, E. D., Brookes, P. C., and Jenkinson, D. S.: An extraction method measuring soil microbial biomass-C, Soil Biol. Biochem., 19, 703–707, https://doi.org/10.1016/0038-0717(87)90052-6, 1987.
Wang, C., Liu, D. W., and Bai, E.: Decreasing soil microbial diversity is associated with decreasing microbial biomass under nitrogen addition, Soil Biol. Biochem., 120, 126–133, https://doi.org/10.1016/j.soilbio.2018.02.003, 2018a.
Wang, C., Lu, X. K., Mori, T., Mao, Q. G., Zhou, K. J., Zhou, G. Y., Nie, Y. X., and Mo, J. M.: Responses of soil microbial community to continuous experimental nitrogen additions for 13 years in a nitrogen-rich tropical forest, Soil Biol. Biochem., 121, 103–112, https://doi.org/10.1016/j.soilbio.2018.03.009, 2018b.
Wang, Z. H., Meng, Y., Zhu-Barker, X., He, X. H., Horwath, W. R., Luo, H. Y., Zhao, Y. P., and Jiang, X. J.: Responses of nitrification and ammonia oxidizers to a range of background and adjusted pH in purple soils, Geoderma, 334, 9–14, https://doi.org/10.1016/j.geoderma.2018.07.038, 2019.
Yao, H. Y., Gao, Y. M., Nicol, G. W., Campbell, C. D., Prosser, J. I., Zhang, L. M., Han, W. Y., and Singh, B. K.: Links between ammonia oxidizer community structure, abundance, and nitrification potential in acidic soils, Appl. Environ. Microb., 77, 4618–4625, https://doi.org/10.1128/aem.00136-11, 2011.
Yu, Q., Duan, L., Yu, L. F., Chen, X., Si, G. Y., Ke, P. P., Ye, Z. X., and Mulder, J.: Threshold and multiple indicators for nitrogen saturation in subtropical forests, Environ. Pollut., 241, 664–673, https://doi.org/10.1016/j.envpol.2018.06.001, 2018.
Zhang, L. M., Hu, H. W., Shen, J. P., and He, J. Z.: Ammonia-oxidizing archaea have more important role than ammonia-oxidizing bacteria in ammonia oxidation of strongly acidic soils, Isme J., 6, 1032–1045, https://doi.org/10.1038/ismej.2011.168, 2012.
Zhang, M. Y., Wang, W. J., Wang, D. J., Heenan, M., and Xu, Z. H.: Short-term responses of soil nitrogen mineralization, nitrification and denitrification to prescribed burning in a suburban forest ecosystem of subtropical Australia, Sci. Total Environ., 642, 879–886, https://doi.org/10.1016/j.scitotenv.2018.06.144, 2018.
Zhang, T. A., Chen, H. Y. H., and Ruan, H. H.: Global negative effects of nitrogen deposition on soil microbes, Isme J., 12, 1817–1825, https://doi.org/10.1038/s41396-018-0096-y, 2018.
Zhang, W., Mo, J. M., Yu, G. R., Fang, Y. T., Li, D. J., Lu, X. K., and Wang, H.: Emissions of nitrous oxide from three tropical forests in Southern China in response to simulated nitrogen deposition, Plant Soil, 306, 221–236, https://doi.org/10.1007/s11104-008-9575-7, 2008.
Zhang, X. L., Wang, Q. B., Li, L. H., and Han, X. G.: Seasonal variations in nitrogen mineralization under three land use types in a grassland landscape, Acta Oecol.-Int. J. Ecol., 34, 322–330, https://doi.org/10.1016/j.actao.2008.06.004, 2008.
Zhao, H. B., Peng, S. L., Chen, Z. Q, Wu, Z. M., Zhou, G. Y., Wang, X., and Qiu, Z. J.: Abscisic acid in soil facilitates community succession in three forests in China, J. Chem. Ecol., 37, 785–793, https://doi.org/10.1007/s10886-011-9970-z, 2011.