the Creative Commons Attribution 4.0 License.
the Creative Commons Attribution 4.0 License.
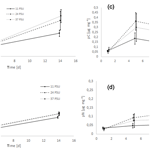
Salinity-dependent algae uptake and subsequent carbon and nitrogen metabolisms of two intertidal foraminifera (Ammonia tepida and Haynesina germanica)
Michael Lintner
Bianca Biedrawa
Julia Wukovits
Wolfgang Wanek
Petra Heinz
Benthic foraminifera are abundant marine protists which play an important role in the transfer of energy in the form of organic matter and nutrients to higher trophic levels. Due to their aquatic lifestyle, factors such as water temperature, salinity and pH are key drivers controlling biomass turnover through foraminifera. In this study the influence of salinity on the feeding activity of foraminifera was tested. Two species, Ammonia tepida and Haynesina germanica, were collected from a mudflat in northern Germany (Friedrichskoog) and cultured in the laboratory at 20 ∘C and a light–dark cycle of 16:08 h. A lyophilized algal powder from Dunaliella tertiolecta, which was isotopically enriched with 13C and 15N, was used as a food source. The feeding experiments were carried out at salinity levels of 11, 24 and 37 practical salinity units (PSU) and were terminated after 1, 5 and 14 d. The quantification of isotope incorporation was carried out by isotope ratio mass spectrometry. Ammonia tepida exhibited a 10-fold higher food uptake compared to H. germanica. Furthermore, in A. tepida the food uptake increased with increasing salinity but not in H. germanica. Over time (from 1–5 to 14 d) food C retention increased relative to food N in A. tepida while the opposite was observed for H. germanica. This shows that if the salinity in the German Wadden Sea increases, A. tepida is predicted to exhibit a higher C and N uptake and turnover than H. germanica, with accompanying changes in C and N cycling through the foraminiferal community. The results of this study show how complex and differently food C and N processing of foraminiferal species respond to time and to environmental conditions such as salinity.
- Article
(309 KB) - Full-text XML
-
Supplement
(145 KB) - BibTeX
- EndNote
The intertidal zone is one of the most extreme habitats on earth. This ecotone, also known as the foreshore or seashore, is determined by tidal activity. It is an important habitat for various living organisms like starfishes, sea urchins, corals and foraminifera (Allen, 2000). Due to the alternating presence–absence of water, organisms living here must adapt to the specific environmental conditions. Important factors shaping the intertidal environment are the fluctuating water temperature and salinity, pH, available food sources, sediment organic matter content, and fresh water supply. These environmental factors significantly influence the activity of foraminifera (e.g., Schafer et al., 1996; Caldeira and Wickett, 2005; Keul et al., 2013; Wukovits et al., 2017).
Foraminifera are unicellular organisms, which live predominantly in marine environments. A recent field study showed that benthic foraminifera can account for up to 84 % of total protozoan biomass in mudflats (Lei et al., 2014). Many foraminifera feed on phytoplankton (algae, diatoms) and thus play an important role in passing on energy in the form of organic matter to higher trophic levels (Azam et al., 1983; Beringer et al., 1991). Due to the large quantity of foraminifera in the deep and shallow ocean waters and their large contribution to the uptake of primary produced organic material, foraminifera significantly contribute to the global marine carbon and nitrogen cycles (Altenbach, 1992; Graf, 1992; Gooday et al., 1992; Nomaki et al., 2008; Glock et al., 2013).
Foraminifera can even change between active feeding and passive ingestion diets depending on how much food is available (Sliter, 1965). Some foraminifera can retain organelles (chloroplasts) from certain food sources and integrate them into their own metabolic cycle. This process is commonly referred to as kleptoplastidy. Currently nine benthic foraminiferal genera are known to follow this lifestyle: Bulimina, Elphidium, Haynesina, Nonion, Nonionella, Nonionellina, Reophax, Stainforthia und Virgulinella (Lopez, 1979; Lee et al., 1988; Cedhagen, 1991; Bernhard and Bowser, 1999; Correia and Lee, 2000; Grzymski et al., 2002; Goldstein et al., 2004; Pillet et al., 2011; Lechliter, 2014; Tsuchiya et al., 2015). In the temperate Wadden Sea, a part of the North Sea, two foraminifera species occur most frequently, Ammonia tepida and Haynesina germanica, and have been relatively well studied in terms of trophic ecology. While Ammonia does not seem to be capable of kleptoplastidy (Jauffrais et al., 2016), H. germanica possesses chloroplasts which are absorbed from food (microalgae) and are retained as organelles (Lopez, 1979). Cesborn et al. (2017) demonstrated that the plastids in H. germanica are photosynthetically active, based on changes in O2 consumption rates during dark–light transitions. Haynesina germanica therefore follows a mixotrophic lifestyle, with autotrophic and heterotrophic nutrition (Cesborn et al., 2017). While Ammonia can rapidly ingest organic carbon (Moodley et al., 2000) and A. tepida has a higher potential to convert algal organic matter into cellular biomass in a short time frame compared to H. germanica (Wukovits et al., 2018), the latter species (H. germanica) can eventually reduce its dependency on external food due to the presence of kleptoplasts.
The uptake of food by foraminifera depends on several factors such as food quality and quantity, temperature, and salinity (Lee et al., 1966; Dissard et al., 2009; Wukovits et al., 2017). Past experiments with A. tepida and H. germanica showed that increasing temperature had a negative effect on food uptake of foraminifera (Wukovits et al., 2017). The highest food uptake rates were recorded at 20 ∘C in comparison with the other tested temperatures 25 and 30 ∘C. As the temperature increased foraminifera of both species consumed less food (Wukovits et al., 2017). Today not only increasing temperature but also salinity changes play an important role in the oceans, mainly because of anthropogenic influence; however effects of salinity on food uptake and digestion by foraminifera have not yet been studied. Based on the strong variability and fluctuations in salinity levels in the intertidal systems, we studied food uptake of A. tepida and H. germanica at different salinity levels to provide a better understanding of the turnover of phytoplankton by foraminifera with changing physical conditions (salinity).
2.1 Sampling
The sample material was collected in May 2018 during low tide at Friedrichskoog-Spitze (German Wadden Sea, at 54∘02′ N, 8∘50′ E). At that time the seawater had a salinity of 24.2 PSU and a temperature of 13 ∘C, and the air temperature was 11 ∘C. The collected sediment was directly wet-sieved with seawater from the location at the site through a 125 and a 63 µm sieve to remove larger meiofauna and smaller organic particles. In the laboratory, the sediments (size class 63–125 µm) containing living benthic foraminifera were fed regularly with Dunaliella tertiolecta (green algae) until the start of the experiment and were kept at a temperature of 21 ∘C and a salinity of 24 PSU. A value of 1 PSU (1 practical salinity unit) corresponds approximately to 1 g salt per kilogram seawater.
2.2 Preparation of 13C15N-labeled phytodetritus
A f∕2 medium (Guillard and Ryther, 1962; Guillard, 1975), enriched with 13C (1.5 mmol L−1 NaH13CO3) and 15N (0.44 mmol L−1 Na15NO3) was used as a nutrient solution for the cultivation and production of isotopically labeled D. tertiolecta, a common food source in laboratory experiments with benthic foraminifera (e.g., Heinz et al., 2002; Wukovits et al., 2017). It should be noted that H. germanica prefers to eat diatoms (Austin et al., 2005); however significant uptake of D. tertiolecta was also previously reported (Wukovits et al., 2017). The algal culture was kept in an incubator at 20 ∘C with a light–dark cycle of 16:08 h. Once the algae had grown to high density in the medium, they were collected by centrifugation at 800 rpm for 10 min. The algal pellet was washed three times with artificial seawater (Enge et al., 2011). After each washing step the culture was centrifuged and the supernatant decanted. For the storage of the labeled algae, the pellet was shock frozen in liquid nitrogen and then lyophilized for 4 d at 0.180 mbar. The labeled algal powder was isotopically enriched at about 3.3 at. % 13C and 32.3 at. % 15N (C:N ratio is about 5.58).
3.1 Sample preparation
The experiment was run in triplicates. For each salinity level (11, 24 and 37 PSU) and each time point of harvest (1, 5 and 14 d) three glass crystallization dishes were set up for A. tepida and for H. germanica. The selected salinities correspond to a brackish milieu (11 PSU), to the natural conditions in the North Sea (24 PSU) and to a highly saline basin (37 PSU). For A. tepida 55 individuals and for H. germanica 60 individuals were prepared per replicate to obtain a dry mass of cytoplasm between 1 and 2 mg. The crystallization dishes were filled with 280 mL of filtered (pore size: 0.45 µm) natural seawater from the sampling site. The salinity was previously adjusted to the desired PSU value by adding NaCl or distilled water. The foraminifera were then placed in the dishes (without sediment) and acclimated at 20 ∘C and a light–dark cycle of 16:08 h for 3 d in an incubator. The crystallization dishes were sealed tightly with Parafilm.
For our experiments, we only used foraminifera with densely filled cytoplasm. In addition, we only used individuals with an intense yellowish color of the cytoplasm. The incubation time of foraminifera in the crystallization dishes before feeding was used for the “crawling test”. Foraminifera were placed in the center of the crystallization dish immediately after removal from the cultures. After 24 h individuals could be identified that have moved away from the center. Accordingly, these individuals have active pseudopodia and are alive. In all experiments it was very rare (below 4 %) that single individuals did not show colored cytoplasm and they were therefore counted as “survive”. Completely empty tests, which would clearly stand for dead individuals, were not found.
In order not to disturb the experiments, no water change was carried out. O2 and pH were also not measured, since we did not expect a significant change due to the small amount of added food.
Following the acclimation period, 5 mg of lyophilized labeled algal powder was added as the only food source to each replicate and left in the incubator for the desired incubation time. The algae powder settled down on the bottom of the dish and was available for foraminifera. In addition, untreated (not fed) foraminifera were taken to obtain the natural abundance of 13C and 15N as a reference. At the end of the experiments a precipitate of the algal powder was still visible in the crystallization dishes, which confirms the continuous availability of food during the experiments. The salinity was checked daily and corrected when necessary.
3.2 Sample preparation and processing
Before the start of the experiments all glassware was cleaned by combusting at 500 ∘C for 5 h in a muffle furnace. The “picking tools” and tin capsules were cleaned by rinsing with a 1:1 (v:v) mixture of dichloromethane (CH2Cl2) and methanol (CH3OH). After the incubation period, foraminifera were removed from the crystallization dishes, cleaned and washed three times with distilled water. Then they were transferred into the tin capsules (Sn 99.9 %, IVA Analysentechnik GmbH & Co. KG) and excess water was removed. The samples were air-dried for 3 d (Enge et al., 2018) and then decarbonated with 4 % HCl (3×5 µL for A. tepida and 2×5 µL for H. germanica). During the decarbonization of foraminiferal tests, the samples were kept at 60 ∘C for 24 h. Finally, the samples were dried for 3 d at 60 ∘C, before being weighed to the nearest hundredth of a milligram.
3.3 Analyses
The measurements of C and N contents as well as the isotope ratios of the samples were carried out in the Stable Isotope Laboratory for Environmental Research (SILVER) of the University of Vienna. The ratios of 13C∕12C and 15N∕14N were measured by an isotope ratio mass spectrometer (IRMS, DeltaPLUS, coupled by a ConFlo III interface to an elemental analyzer EA 1110, Thermo Finnigan). In the following calculations, X stands for the heavy isotopes of C and N, i.e., 13C and 15N, respectively. The atomic percentage of heavy isotopes (at. % 13C and at. % 15N) was calculated using the measured δ13C and δ15N values and the international standards for C (Vienna PeeDee Belemnite RVPDB=0.0112372) and N isotopes (atmospheric nitrogen RatmN=0.0036765) according to the following equations:
where R depicts the ratio of heavy isotope to light isotope i.e., 13C:12C or 15N:14N, in samples and international standards, respectively.
Subsequently, the values needed to be corrected for the at. % X present in the natural environment, i.e., in unlabeled foraminifera. The so-called isotope excess (E) was calculated according to Middelburg et al. (2000):
In the next step, the isotope incorporation was determined according to the following equation:
Depending on the biomass units used, Iiso results in the unit micrograms per milligram (based on dry matter of the cytoplasm) or micrograms per individual (based on the number of individuals).
Finally, the uptake of phytodetrital C (pC) and phytodetrital N (pN) was calculated for the cytoplasm of foraminifera:
where at. %phyto represents the isotopic enrichment in 13C and 15N of the labeled D. tertiolecta food. All results were additionally converted to time-based food uptake rates (µg mg−1 h−1).
3.4 Statistics
Regression analysis was applied to statistically test for time effects on food uptake, and linear and curvilinear models were tested. The best models were selected based on the highest coefficient of determination (R2). Three-way analysis of variance (ANOVA) was applied to test for the main effects of species, salinity and time, and two-way ANOVA was applied for salinity and time effects on pC and pN within species, followed by Fisher's least significant distance (LSD) post hoc tests. All statistical tests were performed using R (R development Core Team, 2008).
4.1 Carbon uptake
The isotope measurements showed that the offered labeled food source was utilized by both A. tepida and H. germanica. Three-way ANOVA showed a significant effect of species (A. tepida > H. germanica, p<0.001), time (p<0.001) and salinity (p<0.001) on pC. Moreover, two-way ANOVA highlighted a significant effect of time (p<0.001) and salinity (p<0.001) on pC in A. tepida and of time (p<0.001) but not salinity (p=0.0739) on pC in H. germanica. Salinity had a major impact on food uptake (pC) only in A. tepida.
As shown in Fig. 1a, A. tepida had the highest pC value at a salinity level of 37 PSU for the most dates, followed by 24 PSU. At lowest salinity (11 PSU) pC further decreased. It should be noted that from day 1 to day 5 the uptake of C at 24 PSU and 37 PSU decreased considerably before it increased again towards day 14. This intermediate minimum was not recognizable at 11 PSU. At 11 PSU pC increased linearly with time (f(d) = 0.05163 × d + 0.06530, R2=0.9985, based on mean values of pC).
Time kinetics were different for H. germanica. After one feeding day the measured pC values did not differ between salinity levels and were lowest. Food C uptake peaked after 5 d and thereafter declined. However, salinity did not affect pC in this species.
In addition to pC values, C uptake rates for the 1 d sample were also determined. Ammonia tepida showed the highest uptake rates at 24 (0.029 µg mg−1 h−1) and 37 PSU (0.036 µg mg−1 h−1) after 1 d of food supply. For 11 PSU, C uptake rates were much lower (0.004 µg mg−1 h−1). For H. germanica, C uptake rates at salinities of 11 (0.002 µg mg−1 h−1), 24 (0.003 µg (mg−1 h−1) and 37 (0.002 µg mg−1 h−1) PSU are in the same area.
4.2 Nitrogen uptake
Two-way ANOVA showed a significant effect of salinity (p<0.001) and time (p<0.001) on nitrogen uptake (pN) for A. tepida. For H. germanica, as with pC, pN was only affected by time (p=0.0027) but not by salinity (p=0.0690).
Nitrogen uptake of A. tepida showed a highly comparable pattern to C uptake (Fig. 1). Minimum N uptake was always recorded at the lowest salinity level. However, the uptake of N after 5 d was approximately the same at 24 and 37 PSU and reached a minimum at both salinities here. The development of pN at 11 PSU could be described by a straight line (f(d) = 0.02354 × d + 0.02011) with a very high coefficient of determination (R2=0.9978).
Haynesina germanica exhibited lower values of pN compared to A. tepida (Fig. 1d). The highest N uptake after 5 and 14 d was at the moderate salinity level (24 PSU), though this was not significant. Again, food N uptake increased linearly with time (f(d) = 0.00185 × d + 0.03522, R2=0.9317) at the lowest salinity level but showed a saturating behavior at 24 and 37 PSU.
Food N uptake rates are also calculated. For A. tepida the N uptake rates are similar to the C uptake rates, and C uptake rates were approximately twice as high as N uptake rates. For H. germanica the average N uptake rates were very close at all three salinity levels, suggesting similar N uptake rates independent of salinity in H. germanica.
4.3 Relations between food C and N incorporation
All data of C and N uptake obtained in this study were plotted as pC-to-pN relationships in Fig. 2.
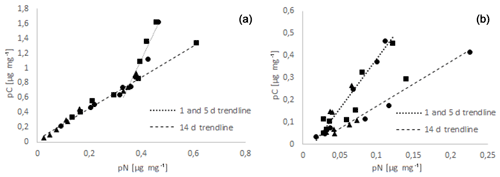
Figure 2Relationship between food N uptake (pN) and food C uptake (pC) for A. tepida (a) and H. germanica (b) for the time windows day 1 to 5, and day 14. Regressions were run separately for these time windows. The triangles correspond to the values at 11 PSU, the circles to those at 24 PSU, and the squares to the values at 37 PSU.
Ammonia tepida showed a continuous increase in pC with pN (Fig. 2). The 1 and 5 d samples were all plotting on a straight line, with the lowest-salinity samples having the lowest pC and pN values. At later stages (day 14) the slope and therefore the pC:pN ratios increased markedly (from 2.1 to 7.4). Haynesina germanica also showed a general increase in pC with pN. However, the slope between both of them decreased over time in contrast to A. tepida, indicating a decrease in pC:pN (from 4.5 at day 1 and 5 to 2.0 at day 14) and thereby an increased relative retention of food N compared to food C over time.
5.1 Influence of salinity on food uptake
Both examined foraminifera species showed different responses to salinity variations in terms of food uptake and food uptake rates. The time course of pC and pN in A. tepida showed a noticeable minimum after 5 d. This partial decrease in pC and pN was already reported in experiments testing the effects of temperature on food uptake in the same species (Wukovits et al., 2017). In Wukovits et al. (2017) food uptake was highest on day one and then decreased sharply (5 d) and remained nearly constant thereafter (14 d). These data suggest that A. tepida was “starved” due to the 3 d acclimatization period and immediately responded with rapid food uptake when food was added. The pseudopodia of A. tepida are particularly stimulated by the green algae Dunaliella (Lee et al., 1961). Excessive food uptake in the short time (1 d) can lead to longer-lasting saturation, which explains the significantly lower uptake rates at the intermediate time points.
There are some points which could lead to the low (0.1 %) uptake of food in comparison to the biomass of the foraminifera. An important aspect to consider is the method used when processing the samples. Foraminiferal tests are dissolved with hydrochloric acid and thus carbonate is lost, but new mineral phases are also formed which influence the total weight of the sample. This step is needed to remove the 13C, which may be bound in the test. Comparing with other studies like Wukovits et al. (2017), it can be seen that the uptake values of our experiments are of the same order of magnitude. In comparison, A. tepida showed an average amount of C between 0.8 and 1 µg mg−1 and N of about 0.3 µg mg−1 in our experiments. Wukovits et al. (2017) recorded about 2 µg mg−1 for C and 0.5 µg mg−1 for N in their experiments (at 20 ∘C) with A. tepida. The amount of C absorbed by H. germanica is in both our experiments and Wukovits et al. (2017) (at 20 ∘C) at about 0.3 µg mg−1 and N amounts to 0.05–1 µg mg−1.
Another aspect is that foraminifera are stressed during experimental conditions and therefore may have a lower turnover. It should be noted that the food uptake is determined by the isotope content in the cytoplasm, which can also vary over time.
Since these experiments were all carried out under laboratory conditions, we would not consider pC and pN as absolute values, due to seasonal and environmental fluctuations.
The time course of food uptake at the lowest salinity level was different in A. tepida, starting slow, but then pC and pN increased continuously over time. This might be caused by lowest salinity levels being suboptimal in the short term and that therefore metabolic activation takes longer, causing the linear increase in pC and pN. This explanation supported by the observation that after 5 d food uptake was similar across all three salinity levels. Haynesina germanica showed a different pattern than A. tepida in terms of time dependency of pC and pN. In the former species the presence of kleptoplasts may have attenuated the “starvation effect”, with the result that only a small amount of ingested C and N can be measured after 1 d. This low initial C and N uptake can be related to the results of Cesborn (2017), which show that kleptoplasts are potential C or N sources for foraminifera in starvation periods. However, it should be considered that H. germanica less readily absorbed the offered food compared to A. tepida. Although there was a greater increase in pN between days 1 and 5 than between days 5 and 14, the C and N uptake rates were much lower than those of A. tepida.
It must be noted that while food C and N uptake is related through the C:N of the food source, internal foraminiferal metabolism and release processes can cause a decoupling of C and N metabolism and of isotope labeling patterns. Carbon is incorporated into organic molecules as well as into the calcareous shells or simply released during cellular respiration (e.g., Hannah et al., 1994). The latter leads to a release of carbon into the environment, whereby the measured values of C isotope incorporation are influenced. Nitrogen is also utilized for the production of organic molecules such as DNA or proteins (DeLaca, 1982; Nomaki et al., 2014). Again the release of nitrogen-rich excretion products into the environment has an impact on the nitrogen isotope incorporation patterns.
It should be mentioned that the processing method could result in loss of the cytoplasm. After the experiment, foraminifera were washed with distilled water to remove any salts around their tests, which could influence the mass. This washing process should be carried out carefully, because the tests could burst. In general, all samples were always treated the same way, which means they were all washed with the same volume of distilled water. This way any impact that may have arisen from using the distilled water has the same effect on all samples.
According to Stouff et al. (1999) A. tepida shows hardly any shell deformations at normal marine conditions of 37 PSU. This observation is consistent with the results of this study, as A. tepida had a higher uptake and turnover of organic matter at higher salinities (24–37 PSU), and therefore its optimal living conditions are at higher salinity levels. Yet, in the hypersaline environment (50 PSU) this species generates a high number of deformed juvenile individuals (Stouff et al., 1999). The German Wadden Sea is subject to seasonal salinity fluctuations and has a mean salinity of 30.7–32.5 PSU (Postma, 1983). Depending on the supply of fresh water and evaporation rates, the water in this region can drop to salinities of 25 and reach up to 37 PSU (Maywald, 1991). Our experiments showed that the change in salinity from 24 to 33 PSU had a smaller impact on food uptake than that between 11 and 24 PSU. This shows once again that the two commonly occurring species, A. tepida and H. germanica, have adapted very well to these fluctuations. The lowest salinity (11 PSU) in our experiments represents the transition from brackish to marine milieu. It turned out that at this salinity level the food uptake tended to be the lowest for both species. From the literature it is known that such brackish marshes are mainly inhabited by agglutinated foraminifera (Sen Gupta, 1999). Considering the uptake of C and N by A. tepida and H. germanica in our experiments (Fig. 1), it can be seen that the low salinities do not correspond to the optimum conditions of these foraminifera.
5.2 Effect of salinity on cytoplasmic C:N ratios and δ13C values
Foraminiferal C:N ratios and δ13C signatures in the cytoplasm have been applied as a salinity proxy for marine systems for some time (e.g., Scott and Medioli, 1986; Chmura and Aharon, 1995; Mackie et al., 2005). According to Mackie et al. (2005) δ13C values in the range of −16 ‰ to −22 ‰ represent organic matter and organisms of marine origin. Brackish and freshwater organisms have lighter δ13C values (−22 ‰ to −25 ‰ and −25 ‰ to −30 ‰, respectively) (Mackie et al., 2005). The foraminiferal species studied here showed background δ13C values of −13.9 ‰ (H. germanica) and −15.9 ‰ (A. tepida). These values clearly point towards marine isotope signatures, concordant with a salinity of 24.2 PSU measured during the sampling of the foraminifera.
A change in cytoplasmic C:N ratio of foraminifera in intertidal habitats is fundamentally influenced by two factors: on the one hand by the composition of the local fauna and flora (food) (Stelzer and Lamberti, 2001; Bowman et al., 2005; LeKieffre, 2018) and on the other hand by changes in the physiological processes in the organisms themselves (Frost and Elser, 2002; Cross et al., 2005). Both benthic foraminifera species showed divergent changes in C versus N metabolism of ingested food over time. Ammonia tepida showed an increase in pC:pN with feeding time, resulting from a combination of altered N metabolism (storage of N in form of proteins or DNA versus N excretions) and/or changes in C metabolism (investment of C into cellular components versus losses by cellular respiration). The observed increase in pC:pN may therefore represent either an increase in C incorporation relative to N incorporation due to lower stress (less cellular respiration) or a decrease in N retention (increased N excretion) in the foraminifera after a prolonged feeding time. Haynesina germanica also showed a general increase in pC with pN. However, the slope between pC and pN decreased over time, indicating a decrease in pC:pN and thereby an increased relative retention of food N compared to food C. In our experiments the change in salinity did not affect the pC:pN ratios. In other words the salinity did not cause a change in relative C versus N metabolism in both species. Investigating the behavior of other nutrients such as P or Mg alongside C and N might provide further interesting insights into the intake and metabolism of food and its biochemical constituents. Phosphorus serves as an important building block in nucleic acids and phospholipids and might be an indicator for cellular energy status because it is used for the formation of energy storage molecules such as ATP. The behavior of P at changing environmental conditions may therefore indirectly indicate the stress behavior of foraminifera. Magnesium is an important component of chlorophyll. Based on the Mg content of foraminifera it is possible to reconstruct the amount of chlorophyll and therefore the presence of chloroplasts. It is also possible to quantify chlorophyll directly, for example via spectroscopy. However, this is only possible if the pure cytoplasm is examined without the residues of the shells.
An important point is the different affinity of foraminifera to food. As H. germanica possesses kleptoplasts, which are absent in A. tepida, the two species have different metabolisms and food dependencies. Ammonia tepida showed an approximately 10-fold higher food uptake than H. germanica, partially explained by the preference of A. tepida for the green algae Dunaliella sp. (Lee et al., 1961) which served as the food source here while H. germanica prefers to eat diatoms due to kleptoplastidy.
Furthermore, the alteration and aging of food sources can play an important role affecting feeding and food metabolism, as indicated by the preference for “fresh” or “younger” phytodetritus (Lee et al., 1966). In the experiments here food from the same lyophilized algal batch was always used to avoid this effect. Moreover, selective food uptake of different species of foraminifera needs to be considered, and this was clearly demonstrated in a study where a total of 28 different diatom and chlorophyte species were fed to three littoral benthic foraminifera species but only four to five of these food sources were consumed at significant rates (Lee and Müller, 1973). Ultimately one needs to be aware that contamination by bacteria or other microbes cannot be ruled out, particularly in longer-term experiments, as these organisms also use the food offered as a C or N source (Murray et al., 1986; Dobbs et al., 1989; Middelburg et al., 2000; Gihring et al., 2009).
5.3 Effects of salinity on the foraminiferal community
The foraminifera of the mudflats of Friedrichskoog have been investigated for their responses to environmental parameters such as temperature and organic matter flux (Llobret-Brossa et al., 1998; Brasse et al., 1999; Tillmann et al., 2000). In this study we could show that A. tepida and H. germanica reacts with a lower food uptake compared to a decreasing salinity. At low tide the benthic organisms are strongly exposed to the ambient weather conditions such as wind, rain or sunlight. Due to the geographic location the growth of organisms is strongly linked to the spring and summer months. Past data from Tillmann et al. (2000) showed that growth of phytoplankton in winter is limited or almost zero. During spring local phytoplankton blooms may occur with a daily water column particulate gross production up to 2200 mg C m−2 d−1 (Tillmann et al., 2000). Over this period food availability is not a limiting factor for foraminifera and this situation corresponds to the conditions in our experiments.
The composition of the foraminiferal community in the German Wadden Sea changes within small areas (subzones) (Müller-Navarra et al., 2016). The specific microhabitats are formed by natural parameters such as sediment grain size, pH or food source availability but also by anthropogenic influences such as diking, ditching or sheep grazing (Müller-Navarra et al., 2016). This leads to changes in the hydrological situation, and in combination with natural factors such as precipitation or seepage of ground water, the salinity in mudflats varies significantly in relation to the open ocean (De Rijk, 1995). It seems that the assemblage of foraminifera in such human-influenced salt marshes is controlled mainly by changes in salinity (De Rijk, 1995). De Rijk (1995) showed that in areas with widely varying salinity only a few different types of foraminifera occur. Moreover, it was shown that in years with high precipitation the salinity in areas such as the Wadden Sea or in salt marshes is reduced, causing the density of foraminifera to decrease sharply (Murray, 1968). So the tidal habitats in the region around Friedrichskoog are characterized by multiple environmental factors. This leads to the formation of subzones, where particularly physical influences such as pH, salinity, temperature or tides play an important role. This area is also of particular interest for the future as the anthropogenic impact on fluctuating ecosystems can be monitored very well here. Changes in salinity therefore are a major factor shaping the composition and activity of foraminiferal communities. In this study we could show that the two tested foraminiferal species, A. tepida and H. germanica, responded very differently to salinity in terms of food intake and C and N metabolism. Moreover, a former study demonstrated that the temperature response and temperature optima also differ between these two most abundant foraminifera species of the German Wadden Sea (Wukovits et al., 2017). Therefore environmental and climate change can strongly affect the composition of the foraminiferal community, thereby causing changes in the feeding rates and in the C–N metabolism of the foraminiferal community, and ultimately altering the C–N cycling of these intertidal ecosystems.
The data are available in the Supplement.
The supplement related to this article is available online at: https://doi.org/10.5194/bg-17-3723-2020-supplement.
The study was planned by ML, BB and PH. ML and BB sampled the material in the field and performed the lab work. WW performed the isotopical analysis and revised the manuscript critically.
The authors declare that they have no conflict of interest.
This paper was edited by Clare Woulds and reviewed by two anonymous referees.
Allen, J.: Morphodynamics of Holocene salt marshes: a review sketch from the Atlantic and southern North Sea coast of Europe, Quat. Sci. Rev., 19, 1155–1231, 2000.
Altenbach, A.: Short-term processes and patterns in the foraminiferal response to organic flux rates, Mar. Micropaleontol., 19, 119–129, 1992.
Austin, H., Austin, W., and Paterson, D.: Extracellular cracking and content removal of benthic diatom Pleurosigma angulatum (Quekett) by the benthic foraminifera Haynesina germanica (Ehrenberg), Mar. Micropaleontol., 57, 68–73, 2005.
Azam, F., Fenchel, T., Field, J., Gray, J., Meyer-Reil, L., and Thingstad, F.: The ecological role of water-column microbes in the sea, Mar. Ecol. Prog. Ser., 10, 257–263, 1983.
Beringer, U., Caron, D., Sanders, R., and Finaly, B.: Heterotrophic flagellates of planktonic communities. their characteristics and methods of study, in: The Biology of Free-Living Heterotrophic Flagellates, edited by: Patterson, D. J. and Larsen, J., Clarendon Press. Oxford, 45, 39–56, 1991.
Bernhard, J. and Bowser, S.: Benthic foraminifera of dysoxic sediments: chloroplast sequestration and functional morphology, Earth-Sci. Rev., 46, 149–165, 1999.
Caldeira, K. and Wickett, M.: Ocean model predictions of chemistry changes from carbon dioxide emissions to the atmosphere and ocean, J. Geophys. Res., 110, C09S04, https://doi.org/10.1029/2004JC002671, 2005.
Cedhagen, T.: Retention of chloroplasts and bathymetric distribution in the Sublittoral Foraminiferan Nonionellina labradorica, Ophelia, 33, 17–30, 1991.
Cesborn, F., Geslin, E., Kieffre, E., Jauffrais, T., Nardelli, M., Langlet, D., Mabilleau, G., Jorissen, F., Jezequel, D., and Metzger, E.: Sequestered Chloroplasts in the benthic Foraminifer Haynesina germanica: Cellular organization, oxygen fluxes and potential ecological implications, J. Foramin. Res., 47, 268–278, 2017.
Chmura, G. and Ahron, P.: Stable carbon isotope signatures of sedimentary carbon in coastal wetlands as indicators of salinity regime, J. Coast. Res., 11, 124–135, 1995.
Cross, W., Benstead, J., Frost, P., and Thomas, S.: Ecological stoichiometry in freshwater benthic systems: recent progress and perspectives, Freshwater Biol., 50, 1895–1912, 2005.
Correia, M. and Lee, J.: Chloroplast retention by Elphidium excavatum (Terquem), Is it a selective process?, Symbiosis., 29, 343–355, 2000.
DeLaca, T.: Use of dissolved amino acids by the foraminifer Notodendrodes antarcticos, Am. Zool., 22, 683–690, 1982.
De Rijk, S.: Salinity control on the distribution of salt marsh foraminifers (Great Marshes. Massachusetts), J. Foramin. Res., 25, 156–166, 1995.
Dissard, D., Nehrke, G., Reichart, G., and Bijma, J.: The impact of salinity on the Mg/Ca and Sr/Ca ratio in the benthic foraminifera Ammonia tepida: Results from culture experiments, Geochi. Cosmochim. Ac., 74, 928–940, 2009.
Dobbs, F., Guckert, B., and Carmin, K.: Comparison of three techniques for administering radiolabeled substrates to sediments for trophic studies: Incorporation by microbes, Microbiol. Ecol., 17, 237–250, 1989.
Enge, A., Nomaki, N., Ogawa, N., Witte, U., Moeseneder, M., Lavik, G., Ohkouchi, N., Kitazato, H., Kucera, M., and Heinz, P.: Response of the benthic foraminiferal community to a simulated short-term phytodetritus pulse in the abyssal North Pacific, Mar. Ecol. Prog. Ser., 438, 129–142, 2011.
Enge, A., Wanek, W., and Heinz, P.: Preservation effects on isotopic signatures in benthic foraminiferal biomass, Mar. Micropaleontol., 144, 50–59, 2018.
Frost, P. and Elser, J.: Effect of light and nutrients on the net accumulation and element composition of epilithon in boreal lakes, Freshwater Biol., 47, 173–183, 2002.
Glock, N., Schönfeld, J., Eisenhauer, A., Hensen, C., Mallon, J., and Sommer, S.: The role of benthic foraminifera in the benthic nitrogen cycle of the Peruvian oxygen minimum zone, Biogeosciences, 10, 4767–4783, https://doi.org/10.5194/bg-10-4767-2013, 2013.
Goldstein, S., Bernhard, J., and Richardson, E.: Chloroplast Sequestration in the Foraminifer Haynesina germanica: Application of High Pressure Freezing and Freeze Substitution, Microsc. Microanal., 10, 1458–1459, 2004.
Gooday, A.: The role of benthic foraminifera in deep-sea food webs and carbon cycling, in: Deep-sea food chains and the global carbon cycle, edited by: Rowe, G. T. and Pariente, V., Kluwer Academic Publishers, Dordrecht, 63–91, 1992.
Graf, G.: Benthic-pelagic coupling: a benthic review, Oceanogr. Mar. Biol. Annu. Rev., 30, 149–190, 1992.
Grzymski, J., Schönfield, O., Falkowski, P., and Bernhard, J.: The function of plastids in the deep-sea benthic foraminifer, Nonionella stella, Limnol. Oceanogr., 47, 1569–1580, 2002.
Gihring, T., Humphrys, M., Mills, H., Huet, M., and Kostka, J.: Identification of phytodetritus-degrading microbial communities in sublittoral Gulf of Mexico sands, Limnol. Oceanogr., 54, 1073–1083, 2009.
Guillard, R.: Culture of phytoplankton for feeding marina Invertebrates, in: Culture of marine invertebrates animals, Springer, 29–60, 1975.
Guillard, R. and Ryther, J.: Studies of marina planktonic diatoms: I Cyclotella nana Hustedt. and Detonula confervacea (CLEVE) Gran. Can. J. Microbiol., 8, 229–239, 1962.
Hannah, F., Rogerson, R., and Laybourn-Parry, J.: Respiration rates and biovolumes of common benthic Foraminifera (Protozoa), Cambridge University Press., 72, 301–312, 1994.
Heinz, P., Hemleben, C., and Kitazato, H.: Time-response of cultured deep-sea benthic foraminifera to different algal diets, Deep-Sea Res. Pt. I, 49, 517–537, 2002.
Jauffrais, T., Jesus, B., Metzger, E., Mouget, J.-L., Jorissen, F., and Geslin, E.: Effect of light on photosynthetic efficiency of sequestered chloroplasts in intertidal benthic foraminifera (Haynesina germanica and Ammonia tepida), Biogeosciences, 13, 2715–2726, https://doi.org/10.5194/bg-13-2715-2016, 2016.
Keul, N., Langer, G., de Nooijer, L. J., and Bijma, J.: Effect of ocean acidification on the benthic foraminifera Ammonia sp. is caused by a decrease in carbonate ion concentration, Biogeosciences, 10, 6185–6198, https://doi.org/10.5194/bg-10-6185-2013, 2013.
Lechliter, S.: Preliminary study of kleptoplastidy in foraminifera of South Carolina, Bridges, A Journal of Student Research, 8, 44–54, 2014.
Lee, J., Price, S., Tentchoff, M., and McLaughin, J.: Growth and Physiology of Foraminifera in the Laboratory: Part 1: Collection and Maintenance – Micropaleontology, 7, 461–466, 1961.
Lee, J., McEnery, M., Pierce, S., Freudenthal, H., and Müller, W.: Tracer experiments in feeding littoral foraminifera, J. Protozool., 13, 657–670, 1966.
Lee, J., Lanners, E., and Kuile, B.: The retention of chloroplasts by the foraminifer Elphidium crispum, Symbiosis, 5, 45–59, 1988.
Lee, J. and Müller, W.: Trophic dynamics and niches of salt marsh foraminifera, Am. Zool., 13, 215–223, 1973.
Lei, Y., Stumm, K., Wickham, S., and Beringer, U.: Distributions and Biomass of Benthic Ciliates, Foraminifera and Amoeboid Protists in Marine, Brackish. and Freshwater Sediments, J. Eukaryot. Microbiol., 61, 493–508, 2014.
LeKieffre, C., Jauffrais, T., Geslin, E., Jesus, B., Bernhard, J. M., Giovani M., and Meibom, A.: Inorganic carbon and nitrogen assimilation in cellular compartments of a benthic kleptoplastic foraminifer, Sci. Rep., 8, 10140, https://doi.org/10.1038/s41598-018-28455-1, 2018.
Lobet-Brossa, E., Rossello Mora, R., and Aman, A.: Microbial Community Composition of Wadden Sea Sediments as Revealed by Fluorescence in Situ Hybridization, Environ. Microbiol., 64, 2691–2696, 1998.
Lopez, E.: Algal chloroplasts in the protoplasm of three species of benthic foraminifera: taxonomic affinity, Viability and persistence, Mar. Biol., 53, 201–211, 1979.
Mackie, E., Leng, M., Lloyd, J., and Arrowsmith, C.: Bulk organic δ13C. C∕N ratios as paleosalinity indicators within a Scottish isolation basin, J. Quat. Sci., 20, 303–312, 2005.
Maywald, A.: Das Watt, 1. Aufl. Ravensburg, Otto Maier, Ravensburg, ISBN 10:3473461709, 1991.
Middelburg, J., Barranguet, C., Boschker, H., Herman, P., Moens, T., and Heip, C.: The fate of intertidal microphytobenthos carbon: An in situ 13C-labeling study, Limnol. Oceanogr., 45, 1224–1234, 2000.
Moodley, L., Boschker, H., Middelburg, J., Pel, R., Herman, P., and De Deckere, E.: Ecological significance of benthic foraminifera 13C labelling experiments, Mar. Ecol. Prog. Ser., 202, 289–295, 2000.
Müller-Navara, K., Milker, Y., and Schmiedl, G.: Natural and anthropogenic influence on the distribution of salt marsh foraminifera in the bay of Tümlau, German North Sea, J. Foramin. Res., 46, 61–74, 2016.
Murray, J.: The living Foraminifera of Christchurch Harbour, England, Micropal., 14, 83–96, 1968.
Murray, R., Cooksley, K., and Priscu, J.: Stimulation of bacterial DNA synthesis by algal exudates in attached algal-bacterial consortia, Appl. Environ. Microbiol., 52, 1177–1182, 1986.
Nomaki, H., Ogawa, N., Ohkouchi, N., Suga, H., Toyofuku, T., Shimanaga, M., Nakatsuka, T., and Kitazato, H.: Benthic foraminifera as trophic links between phytodetritus and benthic metazoans: carbon and nitrogen isotopic evidence, Mar. Ecol. Prog. Ser., 357, 153–164, 2008.
Nomaki, H., Chikaraishi, Y., Tsuchiya, M., Ohkouchi, N., Uematsu, K., Tame, A., and Kitazato, H.: Nitrate uptake by foraminifera and use in conjunction with endobionts under anoxic conditions, Limnol. Oceanogr., 59, 1879–1888, 2014.
Pillet, L., Vargas, C., and Pawlowski, J.: Molecular identification of sequestered diatom chloroplasts and kleptoplastidy in foraminifera, Protist, 162, 394–404, 2011.
Postma, H.: Hydrography of the Wadden Sea: Movements and properties of water and particulate matter, in: Ecology of the Wadden Sea, edited by: Wolff, W. J. and Balkema, A. A., Rotterdam, 1–75, 1983.
Sen Gupta, B.: Foraminifera in marginal marine environments, Modern Foraminifera, 141–159, 1999.
Schafer, C., Cole, F., Frobel, D., Rice, N., and Buzas, M.: An in situ experiment on temperature sensitivity of nearshore temperate benthic foraminifera, Oceanographic Literature Review, J. Foramin. Res., 9, 53–63, 1996.
Scott, F. and Medioli, D.: Foraminifera as sea-level indicators, Sea Level Res., 435–456, 1986.
Sliter, W.: Laboratory Experiments on the Life Cycle and Ecological Controls of Rosalina globularis d'Orbigny, J. Eukaryotic Microb., 12, 210–215, 1965.
Stelzer, R. and Lamberti, G.: Effect of N:P ratio and total nutrient concentration on stream periphyton community structure, Biomass and element composition, Limnol. Oceanogr., 46, 356–367, 2001.
Stouff, V., Geslin, E., Debenaj, J., and Lesourd, M.: Origin of morphological abnormalities in Ammonia (Foraminifera): studies in laboratory and natural environments, J. Foramin. Res., 29, 152–170, 1999.
Tillmann, U., Hesse, K., and Colijn, F.: Planktonic primary production in the German Wadden Sea, J. Plankton Res., 22, 1253–1276, 2000.
Tsuchiya, M., Toyofuko, T., Uematsu, K., Bruchert, V., Collen, J., Yamamoto, H., and Kitazato, H.: Cytologic and genetic characteristics of endobiotic bacteria and kleptoplasts of Virgulinella fragilis (Foraminifera), J. Eukaryotic Microb., 62, 454–469, 2015.
Wukovits, J., Enge, A. J., Wanek, W., Watzka, M., and Heinz, P.: Increased temperature causes different carbon and nitrogen processing patterns in two common intertidal foraminifera (Ammonia tepida and Haynesina germanica), Biogeosciences, 14, 2815–2829, https://doi.org/10.5194/bg-14-2815-2017, 2017.
Wukovits, J., Oberrauch, M., Enge, A. J., and Heinz, P.: The distinct roles of two intertidal foraminiferal species in phytodetrital carbon and nitrogen fluxes – results from laboratory feeding experiments, Biogeosciences, 15, 6185–6198, https://doi.org/10.5194/bg-15-6185-2018, 2018.