the Creative Commons Attribution 4.0 License.
the Creative Commons Attribution 4.0 License.
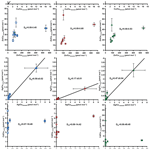
Heavy metal uptake of nearshore benthic foraminifera during multi-metal culturing experiments
Ed C. Hathorne
Joachim Schönfeld
Dieter Garbe-Schönberg
Heavy metal pollution originating from anthropogenic sources, e.g. mining, industry and extensive land use, is increasing in many parts of the world and influences coastal marine environments even after the source has ceased to pollute. The elevated input of heavy metals into the marine system potentially affects the biota because of their toxicity, persistence and bioaccumulation. An emerging tool for environmental applications is the heavy metal incorporation into foraminiferal calcite tests, which facilitates monitoring of anthropogenic footprints on recent and past environmental systems. The aim of this study was to investigate whether the incorporation of heavy metals into foraminifera is a direct function of their concentration in seawater. Culturing experiments with a mixture of dissolved chromium (Cr), manganese (Mn), nickel (Ni), copper (Cu), zinc (Zn), silver (Ag), cadmium (Cd), tin (Sn), mercury (Hg) and lead (Pb) in artificial seawater were carried out over a wide concentration range to assess the uptake of heavy metals by the nearshore foraminiferal species Ammonia aomoriensis, Ammonia batava and Elphidium excavatum. Seawater analyses revealed increasing concentrations for most metals between culturing phases and high metal concentrations in the beginning of the culturing phases due to sudden metal addition. Furthermore, a loss of metals during the culturing process was discovered by an offset between the added and the actual concentrations of the metals in seawater. Laser ablation ICP-MS (inductively coupled plasma mass spectrometry) analysis of the newly formed calcite revealed species-specific differences in the incorporation of heavy metals. The foraminiferal calcite of all three species exhibited Pb and Ag concentrations strongly correlated with concentrations in the seawater culturing medium (partition coefficients and standard deviation for Ag – Ammonia aomoriensis, 0.50 ± 0.02; Ammonia batava, 0.17 ± 0.01; Elphidium excavatum, 0.47 ± 0.04; for Pb – Ammonia aomoriensis, 0.39 ± 0.01; Ammonia batava, 0.52 ± 0.01; Elphidium excavatum, 0.91 ± 0.01). Ammonia aomoriensis further showed a correlation with Mn and Cu, A. batava with Mn and Hg, and E. excavatum with Cr and Ni and partially also with Hg. However, Zn, Sn and Cd showed no clear trend for the species studied, which in the case of Sn was maybe caused by the lack of variation in the seawater Sn concentration. The calibrations and the calculated partition coefficients render A. aomoriensis, A. batava and E. excavatum as natural archives that enable the determination of variations in some heavy metal concentrations in seawater in polluted and pristine environments.
- Article
(5834 KB) - Full-text XML
-
Supplement
(308 KB) - BibTeX
- EndNote
Particular heavy metals, for example, zinc (Zn), iron (Fe), molybdenum (Mo), cobalt (Co) and copper (Cu), serve as micronutrients (e.g. Hänsch and Mendel, 2009) for eukaryotic life and play an important role in the metabolism, growth, reproduction and enzymatic activity of organisms (e.g. Martín-González et al., 2005; Gallego et al., 2007). Other metals like mercury (Hg), on the other hand, are not known to have any positive effect on the body and are therefore believed to have higher toxic potential (Jan et al., 2015). All these metals occur naturally in the environment as geogenic traces in soils, water and rocks and, consequently, in plants and animals. However, at higher concentrations, most heavy metals become toxic and have hazardous effects on marine biota (Stankovic et al., 2014). Heavy metals are defined herein as elements with a density > 7 g cm−3 (Venugopal and Luckey, 1975) and an atomic number beyond calcium (Bjerrum, 1936; Thornton, 1995). Furthermore, they are highly persistent in the marine environment and are not easily excreted by organisms after the uptake of these metals into their system and cells (Flora et al., 2012; Kennish, 2019). Coastal environments act as natural catchments for anthropogenic pollutants because these areas are directly affected by industry, agriculture and urban runoff (e.g. Alloway, 2013; Julian, 2015; Tansel and Rafiuddin, 2016).
In marginal seas and coastal areas, benthic foraminifera are common, and the chemical composition of their calcite test can be used as proxies for changing environmental parameters like water temperature (; e.g. Nürnberg et al., 1995, 1996), salinity (; e.g. Wit et al., 2013; Bertlich et al., 2018) and redox conditions (; Groeneveld and Filipsson, 2013b; Koho et al., 2015, 2017; Kotthoff et al., 2017; Petersen et al., 2018; Guo et al., 2019). Foraminifera take up heavy metals and incorporate them into their calcium carbonate shells during calcification (e.g. Boyle, 1981; Rosenthal et al., 1997; Dissard et al., 2009, 2010a, b; Munsel et al., 2010; Nardelli et al., 2016; Frontalini et al., 2018a, b; Titelboim et al., 2018; Smith et al., 2020). Moreover, foraminifera have a short life cycle (< 1 year; e.g. Haake, 1967; Boltovskoy and Lena, 1969; Wefer, 1976; Murray, 1992) and thus react immediately to changing environmental conditions and contamination levels of the surrounding environment. Therefore, foraminifera archive environmental signals and fossil records from sediments can be used to determine parameters of interest throughout space and time.
Species of the foraminiferal genera Elphidium and Ammonia are among the most abundant foraminiferal taxa in intertidal and shelf environments worldwide. They are found from subtidal water depths to the outer continental shelves (Murray, 1991). Furthermore, their calcite tests are often well preserved in the fossil record (Poignant et al., 2000; McGann, 2008; Xiang et al., 2008) and therefore provide the opportunity to assess past environmental conditions. The combination of all these properties makes foraminifera, and especially Elphidium and Ammonia species, suitable indicators of anthropogenic pollution (e.g. Sen Gupta et al., 1996; Platon et al., 2005). As such, this group of organisms comprises excellent candidates for monitoring the spatial and temporal distribution of heavy metals in seawater to evaluate, for example, the effectiveness of contemporary measures of reducing emissions caused by anthropogenic inputs.
The majority of culturing studies on heavy metal incorporation into benthic foraminifera were designed to assess the influence and uptake of one particular metal, e.g. manganese (Mn) (Barras et al., 2018), copper (Cu) (de Nooijer et al., 2007), chromium (Cr) (Remmelzwaal et al., 2019), lead (Pb) (Frontalini et al., 2015), zinc (Zn) (e.g. Smith et al., 2020), mercury (Hg) (Frontalini et al., 2018a) or cadmium (Cd) (Linshy et al., 2013). This approach is adequate to detail the effects on shell chemistry, growth or physiology. Only two studies reported culturing experiments with elevated levels of Cu, Mn and Ni (Munsel et al., 2010) and elevated levels of Mn, Ni and Cd (Sagar et al., 2021b) in the same culturing medium. However, in reality there is rarely only one metal polluting an environment, but instead a combination of several pollutant metals is usually found (e.g. Mutwakil et al., 1997; Cang et al., 2004; Vlahogianni et al., 2007; Huang et al., 2011; Wokhe, 2015; Saha et al., 2017). How foraminifera incorporate and react to heavy metals when they are co-exposed to more than one metal at a time is less constrained to date. A mixture of different metals will lead to interactions which may result in more severe damage of tissue than exposure to each of them individually would (Tchounwou et al., 2012). For example, a co-exposure to arsenic and cadmium causes more damage of human kidneys than exposure to only one of these elements (Nordberg et al., 2005). Furthermore, a chronic low-dose exposure to multiple elements can cause similar synergistic effects (e.g. Wang et al., 2008). It is therefore reasonable to assume that other organisms are likewise harmed more when exposed to several potentially toxic elements simultaneously.
Here we present results from culturing studies with Ammonia aomoriensis, Elphidium excavatum and Ammonia batava assessing the relationship between heavy metal concentrations in seawater and foraminiferal tests. The partitioning factor between the concentration of an element in the ambient seawater and the calcium carbonate of the foraminifers is constrained by determining both the dissolved metal concentrations in water and the metal contents of individual chambers of the foraminiferal shell that have been precipitated in the culturing medium. In particular, foraminifera were grown while exposed to a combination of 10 different heavy metals, i.e. cadmium (Cd), copper (Cu), chromium (Cr), lead (Pb), manganese (Mn), mercury (Hg), nickel (Ni), silver (Ag), tin (Sn) and zinc (Zn), over a range of concentrations that prevail in polluted nearshore environments today. These metals are the most common representatives of marine heavy metal pollution (Alve, 1995; Martinez-Colon et al., 2009). Once the carbonate vs. seawater metal partitioning coefficients are known, investigations of the chemistry of benthic foraminiferal shells offer a reliable method to monitor short-term changes in the concentration of heavy metals in seawater.
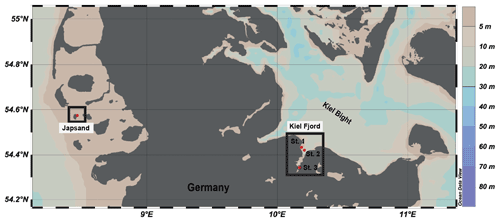
Figure 1Location of the sampling stations in the North Sea (Japsand area) and in the Baltic Sea (Kiel Fjord, St. 1 Strander Bucht, St. 2 Laboe, St. 3 Mönkeberg). The map was drawn with Ocean Data View (Schlitzer, 2016) on the basis of bathymetric data. Water depths in metres are indicated by the colour scale.
2.1 Field sampling
2.1.1 North Sea, Japsand
Living specimens of A. batava were collected at the barrier sand Japsand near Hallig Hooge in the German Wadden Sea in July 2019 at two stations (St. 1 – 54∘34.480′ N, 8∘27.919′ E; St. 2 – 54∘34.491′ N, 8∘27.895′ E) (Fig. 1). The sediment was a glacial till or Eemian clay at Station 1 and fine to medium sand at Station 2. Temperature and salinity of seep waters were measured with a WTW 3210 conductivity meter in excavated holes in the vicinity. The temperature at Station 1 was 21.1 ∘C, and at Station 2 it was 21.6 ∘C. Salinity was 34 PSU at Station 1 and 33.6 PSU at Station 2. The samples were recovered during low tide by scrapping off the uppermost centimetre of the surface sediment with a spoon made out of stainless steel. Natural seawater (NSW) with a salinity of 30.3 PSU was collected near the sites for further processing of the samples. Once back on the nearby island Hallig Hooge, the sediment was washed with NSW through stacked sieves with a mesh size of 2000 and 63 µm. The 2000 µm sieve was used to remove larger organisms and excess organic material (macroalgae, gastropods, lugworms, etc.) that could have induced anoxic conditions in the sediment during transport and storage. The residue was stored in Mucasol soap-washed and acid-cleaned Emsa CLIP & CLOSE® boxes, sparged with air, and some algae food was provided. Back in the laboratory at GEOMAR, the residue was stored at 8 ∘C in a fridge until culturing. These stock cultures were fed twice a week with green-coloured Nannochloropsis concentrate (BlueBioTech), and water was partly exchanged with NSW from the sampling site once a week.
2.1.2 Baltic Sea, Kiel Bight
Living specimens of A. aomoriensis and E. excavatum were collected from different stations in Kiel Fjord, the western Baltic Sea (St. 1, Strander Bucht – 54∘26.001′ N, 10∘11.1078′ E; St. 2, Laboe – 54∘25.254′ N, 10∘12.346′ E; St. 3, Mönkeberg – 54∘20.752′ N, 10∘10.150′ E; water depth – 12.5, 12.3 and 14.3 m, respectively), in September and October 2019 with FB Polarfuchs and FS Alkor, respectively (Fig. 1). A Rumohr corer (inner diameter 55 mm) was used on FB Polarfuchs, and nine cores were taken (two at St. 1 and seven at St. 3). The sediment from the cores was collected in Mucasol-treated and acid-cleaned plastic containers with NSW from the site.
On FS Alkor, a Reineck box corer was used (200 mm × 250 mm), and three replicates at each station were taken (St. 1–3). The first 1 to 2 cm of the sediment surface of the box core was scrapped off with a spoon made out of stainless steel, and the material was stored in a Mucasol-treated and acid-cleaned plastic box with NSW from the location.
Back in the laboratory at GEOMAR, the samples were treated the same way as Japsand samples from the North Sea. Artificial seawater (ASW, Tropic Marin) with a salinity of 30 PSU was used for washing and storage of the surface samples from Kiel Fjord. The use of artificial seawater ensured that no harmful microorganism could invade the cultures.
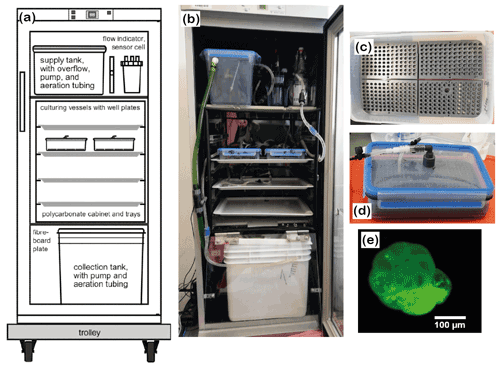
Figure 2Culturing setup. (a) Conceptual draft and (b) assembly of the system. Tubing and hoses were omitted from the draft for clarity. (c) A well plate with mounted specimens and sand; (d) closed culturing vessel with well plates and conduits. (e) Calcein-stained foraminifer under a fluorescence microscope. Please note that the last 2.5 chambers are labelled and fluorescing brightly. The specimen shown in the picture was dead, cleaned and dried, which ensured that the test itself and not the cytoplasm showed the fluorescence.
2.2 Culturing setup
2.2.1 Picking of the samples
The three foraminiferal species that were used in this study have been described in detail in the literature (e.g. Lutze, 1965; Nikulina et al., 2008; Schweizer et al., 2011; Francescangeli et al., 2021; Schmidt and Schönfeld, 2021). For extracting the foraminiferal specimens from the sediment, about 1 cm3 of the 63-to-2000 µm size fraction was transferred to a petri dish. All living specimens were picked with a paintbrush from this subsample and collected in a small petri dish with ASW. All plastic utensils were treated with Mucasol water and rinsed with 5 % HNO3 prior to use. The paintbrush was cleaned with ethanol to protect the culture from harmful microorganisms. Only specimens with a glossy, transparent and undamaged test were chosen. After picking, a drop of concentrated food (pure culture of Nannochloropsis, green-coloured algae) was added, and the foraminifera were left untouched for a night.
Specimens that met one or more of the following criteria were considered living and used for further procedures:
-
The cytoplasm of the specimens was present in more than two chambers that were connected, including the innermost chambers.
-
Specimens showed a structural infill of cytoplasm with a bright green colour, indicating that they took up the food overnight.
-
They developed a film or strings of pseudopodia that firmly stuck to sediment particles or food.
-
They had covered themselves or gathered a cyst of sediment or food particles.
Specimens were identified and sorted by species and stained with calcein (10 mg L−1; Bernhard et al., 2004) (bis[N,N-bis(carboxymethyl)aminomethyl]-fluorescein) (Sigma-Aldrich) directly before each culturing phase to ensure that freshly labelled foraminifera were inserted into the culturing system (Fig. 2e). Staining lasted for 14 d. Petri dishes were stored at 8 ∘C in a fridge; partial water exchanges and feeding of the foraminifera were performed twice a week. After the staining, the foraminifera were transferred to a petri dish with ASW and left for 1 to 2 d to remove excess calcein from seawater vacuoles in their cytoplasm prior to the introduction into the culturing system.
Table 1Heavy metal concentration in the multi-metal stock solution, target concentration of these metals in each phase and salt compounds used. All salts used were provided in pro analysi quality and were purchased from Carl Roth (CrCl3 ⋅ 6 H2O, SnCl2 ⋅ 2 H2O and PbCl2), Walter CMP (CdCl2) and Sigma-Aldrich (MnCl2 ⋅ 4 H2O, NiCl2 ⋅ 6 H2O, CuCl2 ⋅ 2 H2O, ZnCl2, AgNO3 and HgCl2).
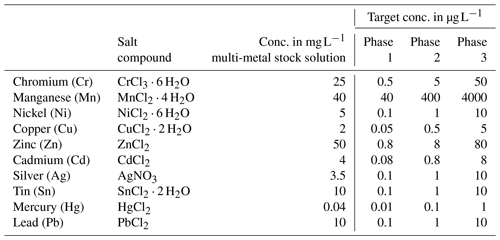
2.2.2 Culturing system
We used two closed-circulation incubation systems for foraminifera (Fig. 2a and b) provided by the Institute of General Microbiology, Kiel University (Woehle et al., 2018, their Fig. S4). The systems were further developed based on earlier closed-circulation systems for culturing foraminifera (Hintz et al., 2004; Haynert et al., 2011). They were slightly modified for the requirements of this study, but the basic operational principle is described by Woehle et al. (2018). In detail, the systems consisted of three levels with different functions. They were built into Bauknecht WLE 885 fridges for temperature control. Each system accommodated two culturing vessels, which were arranged pairwise on a tray in a polycarbonate cabinet (Fig. 2a and b). The water was pumped from the collection tank at the lowest level to the top level into the supply tank. From the supply tank, the water was directed to the culturing vessels, and the flow was regulated, ensuring that the same amount of water was provided to every culturing vessel. After passing the culturing vessels, the water was redirected to the collection tank. The systems were filled with 15 L of ASW with a salinity of 30.5 PSU. The water was aerated in the supply and the collection tank with filtered (0.2 µm) air from outside the system. Monitoring of temperature and salinity was performed with a WTW 3210 conductivity meter. Uncertainty in the conductivity measurements was ± 0.5 % and ± 0.1 ∘C for temperature according to the manufacturer's test certificate. pH was monitored using a pH electrode (GHL) for aquarium purposes with uncertainties of ± 0.06. All parts that were introduced into the system were sterilised before use by autoclaving, UV-lamp exposure or applying DanKlorix®.
2.2.3 Preparation for incubation
For the incubation of the foraminifera, well plates with cavities made from PVC were used (Fig. 2c). All well plates had been used in previous experiments for culturing foraminifera in seawater, which ensured that potentially toxic substances or additives were already released from the material (Woehle et al., 2018). Before the foraminifera were placed in the cavities, each cavity was filled with sterile quartz sand up to a 1.5 mm height. The cavities were subsequently filled with artificial seawater, and the specimens were inserted randomly. Prepared well plates were left untouched for one night to make sure that the foraminifera were able to spread their pseudopodial network before incubation. This ensures that they were stably anchored in the cavities and did not float when the culturing vessels were filled and mounted (Haynert et al., 2011). Four well plates were assembled in each airtight Emsa CLIP & CLOSE® box (Fig. 2d). Each culturing vessel had a lid with an inflow and an outflow conduit, for which cleaned food-grade Tygon® tubing was used. To guarantee that the foraminiferal specimens were not flushed away by the incoming water, the inflow conduit reached almost the bottom of the culturing vessel and was placed between two well plates. Once all well plates were arranged in the culturing vessel, the lid was equipped with an additional, elastic sealing and closed. Before the culturing vessels were placed in the culturing systems, each chamber was slowly filled with ASW. Thereafter, the culturing vessels were placed on the shelf in the culturing system and were connected to the supply hoses.
2.2.4 Culturing experiment
The culturing experiment had four different phases. The first, phase 0, was designated as the control phase, and no heavy metals were added. This phase allowed both systems to equilibrate in terms of physicochemical and biological processes and made it possible to determine the background values in terms of seawater constituents. This phase lasted 21 d. Afterwards, one system was used as the control system, where no heavy metals were added. In the other system, three phases with elevated heavy metal concentrations were performed. The phases lasted 21 d each. Tropic Marin Pro-Reef salt was mixed with deionised water for adjusting the salinity. This artificial salt contains all elements and nutrients in sufficient amounts required by marine organisms. A stock solution containing all metals of interest was mixed, and this solution is called the multi-metal stock solution hereafter. It was added to the supply tank of the system (see Fig. 2a) (phase 1, 1 mL; phase 2, 10 mL; phase 3, 150 mL) at the beginning of each phase to reach the target concentration (Table 1). Additionally, a smaller aliquot of the same multi-metal stock solution (phase 1, 0.1 mL; phase 2, 1 mL; phase 3, 10 mL) was introduced twice a week during the 3 weeks of a phase. This was to counteract the loss of metals during the culturing phase through, for example, uptake of metals by foraminifera or algae or by adsorption to surfaces of the culturing system. The target concentration of the elements at each phase were chosen after earlier culturing experiments with foraminifers (Mn, Cu, Ni – Munsel et al., 2010; Pb – Frontalini et al., 2015, 2018b; Zn – Nardelli et al., 2016; Cd – Linshy et al., 2013; Cu – de Nooijer et al., 2007; Le Cadre and Debenary et al., 2006; Cr – Remmelzwaal et al., 2019; Hg – Frontalini et al., 2018a) and to resemble conditions observed in threatened environments. Examples of such environments are San Francisco Bay, California (Thomas et al., 2002); the Black Sea, Turkey (Baltas et al., 2017); or the Gulf of Chabahar, the Oman Sea (Bazzi, 2014). Furthermore, the Adriatic Sea (Ag; Barriada et al., 2007), Jakarta Bay (Williams et al., 2000; Putri et al., 2012), and polluted US and European rivers (Byrd and Andreae, 1982; Kannan et al., 1998; Thomas et al., 2002) were considered. Table A4 summarises the heavy metal concentration in seawater in different areas around the world to compare them to the experimental values. Additionally, the maximum metal concentration as recommended by the EPA (Environmental Protection Agency, USA) is the lower boundary of the concentration range from this study (Prothro, 1993). This was taken into account to ensure that the foraminifera were not limited in their growth and were able to maintain normal physiological functions. A lower concentration than the EPA value is also covered by our study during the control phase or in the control system. The heavy metal concentrations in the culturing media obtained during each phase were monitored by frequent water sampling.
Over the entire culturing period, both systems were exposed to a natural day-and-night cycle, and the flow rate was adjusted to 1.02 mL min−1 (one drop per second) within the culturing vessels. The foraminifera were fed with Nannochloropsis concentrate twice a week (∼ 2000 µg). After 21 d (meaning after each culturing phase) one culturing vessel per system was exchanged. Vessels and specimens were left in the culturing system for the complete culturing phase (21 d), and no exchange took place during a culturing phase.
Temperature and salinity were kept stable at 15.0 ± 0.1 ∘C and 30.2 ± 0.3 PSU (heavy metals) and at 14.9 ± 0.2 ∘C and 30.4 ± 0.4 PSU (control) over the complete culturing period. As the system was mostly closed, evaporation had a minor effect. Demineralised water was added when necessary to keep the salinity stable. The exchanges of culturing vessels between phases inferred a partial water exchange of approximately 10 % (i.e. 1.5 L) every 3 weeks, which ensured a repetitive renewal of water with adequate quality.
2.3 Water samples
2.3.1 Collection of water samples
Water samples for determining the heavy metal concentrations were taken frequently from the supply tanks (see Fig. 2a) of both systems using acid-cleaned syringes (Norm-Ject® disposable syringe, 20 mL, sterile) and sample bottles (LLG narrow-neck bottles, 50 mL, LDPE – low-density polyethylene; Hg – GL 45 laboratory bottle 250 mL with blue cap and ring, boro 3.3). From the beginning of phase 1, sampling was performed once a week. Water samples to be analysed for mercury concentrations had to be treated differently due to analytical constraints as detailed below. The water was filtered through a 0.2 µm PES filter (CHROMAFIL Xtra disposable filters, membrane material – polyethersulfone pore) for heavy metal samples and through a 0.2 µm quartz filter for Hg samples (HPLC syringe filters, 30 mm glass fibre syringe filters/nylon). Filters were rinsed with the sample water before taking the sample. Every water sample was immediately acidified with concentrated ultrapure HCl to a pH of approximately 2 to avoid changes in the heavy metal concentrations due to adsorption to the sample bottle walls or the formation of precipitates.
2.3.2 Preparation of water samples before analysis
For Mn, Zn, Ni, Pb, Cu and Cd concentration analyses, the water samples were pre-concentrated offline using a seaFAST system (ESI, USA). To fill a sample loop, 12 mL of each sample was used, and it was pre-concentrated by a factor of 25 using the seaFAST column into 1.5 M HNO3. All samples were spiked with indium as an internal standard for monitoring and the pre-concentration procedure. Both MilliQ water and bottle blanks of acidified MilliQ water (pH ∼ 2) were stored in the same bottles until the samples were passed through the pre-concentration system. Additionally, procedural blanks were filtered as the samples were also pre-concentrated and measured. A variety of international (open ocean seawater NASS-6, river water SLRS-6, estuarine seawater SLEW-3, all distributed by NRC-CNRC Canada) and in-house (South Atlantic surface water, South Atlantic Gyre water) reference materials were pre-concentrated like the samples. All samples were subsequently analysed by ICP-MS (inductively coupled plasma mass spectrometry).
Other metals (Cr, Ag and Sn) were diluted and directly introduced into the ICP-MS instrument as they are not retained on the Nobias resin used by the seaFAST system. The dilution was performed with indium-spiked nitric acid (2 %), and to match the matrix of these samples, blanks and standards with added NaCl were prepared.
All heavy metals except mercury were measured using an Agilent 7500ce quadrupole ICP-MS instrument. Raw intensities were calibrated with mixed standards, which were made from single-element solutions covering a wide concentration range. Additionally, a dilution series (dilution factors – 1, , and ) of SLRS-6 of river water reference material (NRC Canada; Yeghicheyan et al., 2019) was measured for quality control. Mean values and relative standard deviations (RSDs) derived from the reference materials are summarised in the Appendix (Table A2).
Prior to the measurements of Hg concentrations, all samples were treated with BrCl solution at least 24 h before the analysis to guarantee the oxidation and release of mercury species that were possibly present in a different oxidation states or phases. The BrCl was removed again by adding hydroxylamine hydrochloride at least 1 h prior to analysis before the Hg was reduced to the volatile Hg0 species with acidic SnCl2 (20 % ) during the measuring process. All preparations of the water samples took place in a clean lab within a metal clean atmosphere, and all vials were acid cleaned prior to use. Mercury concentrations were determined using a Total Mercury Manual System (Brooks Rand Model III). The reduced volatile Hg0 was nitrogen-purged onto a gold-coated trap and released again by heating before it was measured via cold vapour atomic fluorescence (CVAFS) under a continuous argon carrier stream. Quality control of the Hg measurements was carried out by measuring mixed standards, made from single-element solutions and confirmed with replicate measurements throughout each analysis. The measurement uncertainty was smaller than 4.5% RSD for all analyses.
The calcium concentration of culture seawater was analysed using a VARIAN 720-ES ICP-OES (inductively coupled plasma optical emission spectrometer). Yttrium was added as an internal spike, and samples were diluted. IAPSO seawater standard (ORIL) was measured after every 15 samples for further quality control which revealed a measurement uncertainty < 0.35 (RSD in percent) for the elements analysed (mean Ca concentration, IAPSO, this study, 419.6 ± 0.15 mg L−1; reference Ca concentration, IAPSO, Batch 161, 423 mg L−1).
2.4 Foraminiferal samples
After every culturing phase, the culturing vessels were taken out of the culturing system, and foraminiferal specimens were collected from their cavities within 1 d. The individuals were cleaned with tap water and ethanol before they were mounted in cell slides to mechanically remove salt scale and organic coatings with a paintbrush. Dead specimens could be identified because they lost the colour of their cytoplasm and, furthermore, did not gather food and particles anymore and thus were lacking a detritus cyst by their aperture.
Table 2Number of inserted and recovered foraminifera from the different systems (C is control system; M is metal system) and phases (0–3). Numbers of living individuals after the experiment and individuals that formed chambers during their individual culturing phase are given in percent. Note that the percentage of living foraminifera is based on the number of foraminifera that could be recovered alive and not on the number of inserted individuals. The number of laser spots is indicated as well.
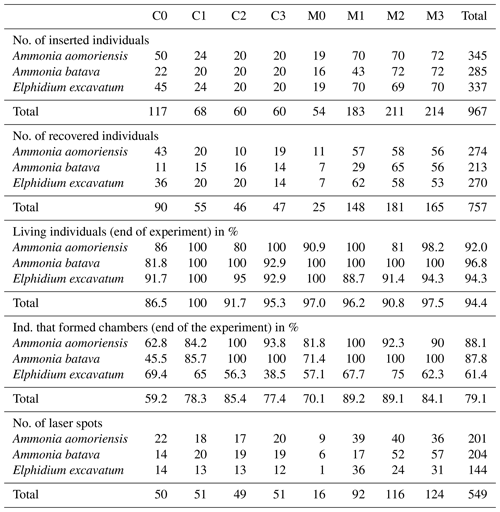
In order to check the growth of foraminifera during the culture experiment, the total number of chambers was counted before and after the experiment for every specimen (Table 2). This was performed to double check the growth in cases where calcein staining may have failed. As the foraminifera were stained with calcein before the experiment, it was possible to cross-check the growth with a fluorescent microscope (Zeiss Axio Imager 2) if new chambers without fluorescence were added and hence check whether the specimen had grown or not (Fig. 2e). Only individuals clearly showing new chambers were analysed by laser ablation ICP-MS.
Prior to the laser ablation analyses, the foraminifera were transferred into individual, acid-leached, 500 µL micro-centrifuge tubes and thoroughly cleaned, applying a procedure adapted from Martin and Lea (2002). The specimens were rinsed three times with MilliQ water and introduced into the ultrasonic bath for a few seconds at the lowest power setting after each rinse. Afterwards, clay and adhering particles were removed by twice rinsing the sample with ethanol, which was followed by three MilliQ rinses again with minimal ultrasonic treatment. Oxidative cleaning was applied using 250 µL of a 0.1 M NaOH and 0.3 % H2O2 mixture added to each sample and the vials were kept for 20 min in a 90 ∘C water bath. Afterwards, the samples were rinsed with MilliQ three times to remove the remaining chemicals. The reductive step of the cleaning procedure was not applied. This step is necessary to remove metal oxides, which of course could also influence the heavy metal concentration within the foraminiferal shell carbonate, but these are usually considered to be added during early deposition (e.g. Boyle, 1983) and therefore unlikely to occur during culture experiments. For laser ablation inductively coupled plasma mass spectroscopy (LA-ICP-MS) measurements, all cleaned specimens were fixed on a double-sided adhesive tape (Plano).
Micro-analytical analyses with LA-ICP-MS were performed at the Institute of Geosciences, Kiel University, using a 193 nm ArF excimer GeoLasPro HD system (Coherent) with a large-volume ablation cell (Zurich-type LDHCLAC; Fricker et al., 2011) and helium as the carrier gas with 14 mL min−1 H2 added prior to the ablation cell. For the foraminiferal samples, the pulse rate was adjusted to 4 to 5 Hz with a fluence between 2 and 3.5 J cm−2. The spot size was set to 44 or 60 µm depending on the size of the foraminiferal chamber. All chambers of a foraminifer that were built up in the culturing medium were analysed, starting from the earliest inner chamber adjacent to the calcein-stained chamber. The laser was manually stopped once it broke through the foraminiferal shell. The ablated material was analysed by a tandem ICP-MS–MS instrument (8900, Agilent Scientific Instruments) in no-gas mode. The NIST SRM 612 glass (Jochum et al., 2011) was used for calibration and monitoring of instrument drift, while NIST SRM 614 was measured for quality control. The glass was chosen because all elements of interest (except Hg) were reported in the literature, which was not the case for established carbonate reference materials. Glasses were ablated with a pulse rate of 10 pulses s−1, an energy density of 10 J cm−2 and a crater size of 60 µm. Dueñas-Bohórquez et al. (2009) demonstrated that different energy densities between the foraminiferal calcite and the glass standard do not affect the analyses. Carbonate matrix reference materials coral JCp-1, giant clam JCt-1, limestone ECRM 752-1 and synthetic spiked carbonate MACS-3 (Inoue et al., 2004; Jochum et al., 2019) in the form of nano-particle pellets (Garbe-Schönberg and Müller, 2014) were analysed for quality control. Carbonate reference material was ablated with a pulse rate of 5 pulses s−1, an energy density of 5 J cm−2 and a crater size of 60 µm. MACS-3 was used for calibrating the mercury content in the samples as Hg is not present in the NIST SRM glasses. All results for the reference materials are given in the Appendix (Table A3). Trace element-to-calcium ratios were quantified using the following isotopes: 26Mg, 27Al, 52Cr, 55Mn, 60Ni, 63Cu, 65Cu, 68Zn, 107Ag, 111Cd, 114Cd, 118Sn, 201Hg, 202Hg and 208Pb normalised to 43Ca. If more than one isotope was measured for an element, the average concentration of these was used after data processing. Analytical uncertainty (in % RSD) was better than 5 % for all trace element (TE) Ca ratios. The lowest RSD in percent based on the NIST SRM 612 glass was 2.1 % for , and the highest was 5.0 % for . Uncertainties in all used standards and reference materials are summarised in Table A3. Each acquisition interval lasted for 90 s and started and ended with measuring 20 s of gas blank, used as the background baseline to subtract from sample intensities during the data reduction process. Furthermore, the background monitoring ensured that the system was flushed properly after a sample. In cases when foraminiferal test walls were very fragile, causing the test to break very quickly, and, hence, the length of the sample data acquisition interval was less than 15 s, these profiles were excluded from further consideration.
Transient logs of raw intensities given in counts per second for all isotopes measured were processed with the software Iolite (Version 4; Paton et al., 2011), producing averages of every time-resolved laser profile. The determination of element Ca ratios was performed after the method of Rosenthal et al. (1999). High values of 25Mg, 27Al or 55Mn at the beginning of an ablation profile were related to contamination on the surface of the foraminiferal shell or remains of organic matter (e.g. Eggins et al., 2003), and these parts of the profiles were excluded from further data processing. The detection limit was defined by 3.3 ⋅ SD of the gas blank in counts per second for every element in the raw data. Only values above this limit were used for further analyses, and no data below the LOQ (limit of quantification, 10 ⋅ SD) were interpreted. After processing the data with Iolite, an outlier detection of the ratios of the samples was performed. If trace metal values from a spot deviated more than ± 2 SD from the average of the samples from the corresponding culturing phase, values were defined as outliers and discarded. The number of rejected points is indicated in the supplementary material (Table S1 in the Supplement).
All statistical tests of the values in the foraminiferal shell and the water were carried out using the statistical program PAST (Hammer, 2001). As the concentration of heavy metals in seawater varied during individual phases in the metal system (Table A1 and Fig. B1 in the Appendix), the mean concentration was calculated by applying an individual curve fit for every phase. The curve was linear, exponential or a power function depending on the trend the particular metal showed. If the type of trend was not clear, the curve type with the highest p and r2 values were chosen. Based on these curves, water values were calculated for every day, and the weighted average from all days was used for further calculations. This ensured that high concentrations at the beginning of each phase did not influence the mean value disproportionately. The partition coefficients of the different trace-metal-to-calcium ratios were calculated using the trace element (TE) and calcium ratios in calcite and seawater. The following equation was used:
When the correlation between the metal concentration in seawater and the metal concentration in the foraminiferal test was positive and significant (r2 > 0.4; p < 0.05), the DTE's are derived from the mean values of all phases and represent the slope of the calculated regression line. In cases where a significant positive correlation between phases could not be identified, the DTE values were calculated from the means of each phase separately and the ranges given. The regression line was forced through the origin, which is a common practice and is applied in many other studies (e.g. Lea and Spero, 1994; Munsel et al., 2010; Remmelzwaal et al., 2019; Sagar et al., 2021a). The reason for this approach is that foraminifers are expected not to incorporate any metals into their shell if the metal concentration is zero in the seawater. In cases where there was clearly a non-zero intercept (Mn of A. batava with phase 3 and Hg of E. excavatum without phase 3), obvious if the course of the regression line changed significantly or the r2 value decreased, then the trend line was not forced through the origin.
3.1 Survival rates, growth rates and reproduction
On average 74.5 % of the specimens inserted into the experiment could be recovered after their individual culturing phase of 21 d, and 94.4 % of these recovered specimens survived. Approximately 79.1 % of the surviving specimens also formed at least one new chamber. Fewer specimens of E. excavatum formed new chambers (61.4 %) than A. batava (87.8 %) or A. aomoriensis (88.1 %) (Table 2). On average, E. excavatum formed only one or rarely two new chambers, whereas both Ammonia species formed usually more than four new chambers. Reproduction happened very sporadically occurring in between two and six specimens per phase, on average 5 %, for the two Ammonia species but not for E. excavatum. No malformed chambers were observed in specimens that were recovered from the heavy-metal-contaminated system.
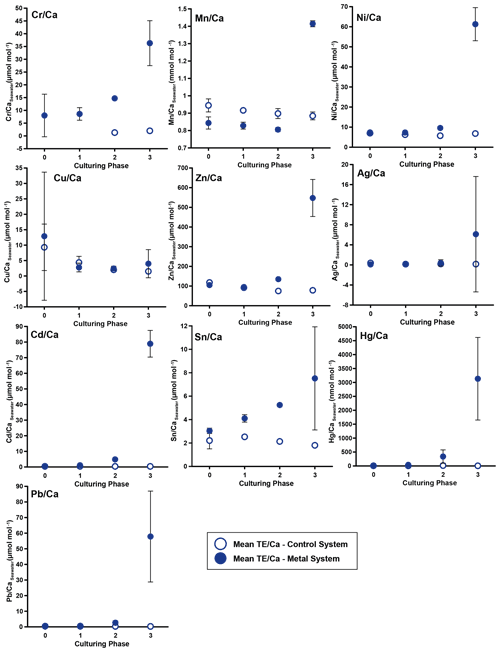
Figure 3Weighted mean values in the culturing medium in µmol mol−1. Error bars display the standard deviation of the mean. Open symbols represent the control system, where no extra metals were added during the complete culturing period (phase 0 to 3), and closed symbols represent the metal system. In this system, phase 0 is the control phase without any extra added metals, and for phase 1 to 3, the heavy metal concentration in the culturing medium was elevated. Note that the standard deviation is comparably high in phase 3 because the heavy metal concentration in this phase varied more strongly, which is shown in the Appendix (Table A1, Fig. B1). Therefore, this error is derived from the real values in the seawater and not from analytical uncertainties. Note that the values from the control system in phase 0 and 1 are not given as these values were below the detection limit.
3.2 Culturing media
In phases 1 and 0 the concentrations in both systems were nearly equal for most elements. Only Cr and Sn had slightly elevated concentrations in the metal system. Furthermore, Cu concentration was higher in the metal system in phase 0 and phase 3 (Fig. 3). In phase 2, all metals but Mn and Cu showed higher concentrations in the metal system than in the control system. Mn concentrations were higher in the control system during phase 0 to phase 2. In phase 3, the concentrations of all heavy metals were elevated in the metal system compared to the control system. The variation in the metal concentration was highest in phase 3, in both systems, for all elements but Cu, which showed the highest variation in phase 0 (Fig. 3). The control system generally displayed a smaller degree of variation than the metal system.
Table 3Weighted mean values in the culturing medium of the control and the metal system ± the standard deviation of the mean. Furthermore, the factors between the target concentrations (Table 1) and the measured concentrations as well as the factors between individual phases are given. Values given without a standard error originate from only one measurement. Averaged values of a phase were calculated based on single values measured on samples from different days during the culturing phase. These single values can be found in the Appendix (Table A1).
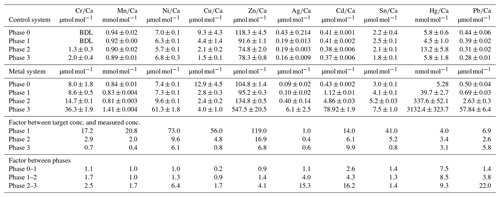
BDL denotes below detection limit.
Even though the aim was to maintain the target concentrations shown in Table 1 during the 21 d of each culturing period by the bi-weekly addition of an aliquot of the multi-metal stock solution, the target concentration of the metals was not obtained for most metals in phase 1 and 2; the only exception was Ag in phase 1 (Table 3). The difference factors between the target and measured concentration were highest (> 50) for Ni, Cu and Zn in phase 1 and decreased in phase 2 and 3. In phase 3, the metals Cr, Mn, Cu, Ag and Sn reached concentrations closer (factor 0.4–0.8) to the target concentration and Ni, Zn, Cd, Hg and Pb concentrations were higher (factor 3.1–9.9) than expected. Furthermore, the change in metal concentration was small for the transition from phase 0 to 1 (factor < 1.4) for all elements but Cd (factor 2.6) and Hg (factor 7.5).
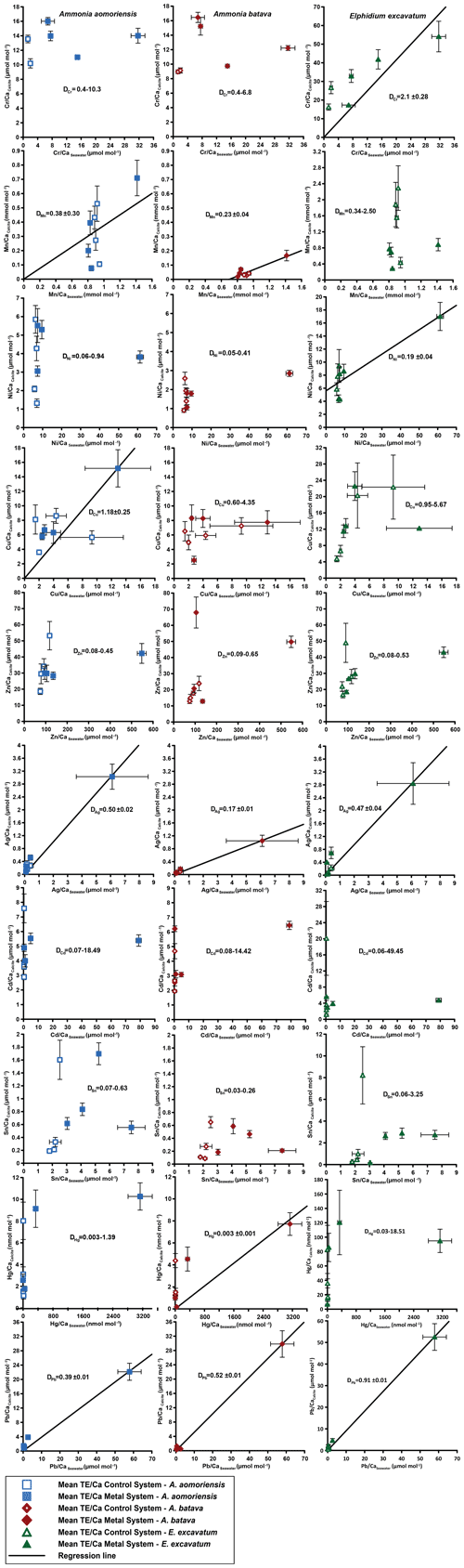
Figure 4Mean values in the foraminiferal calcite versus the mean values in the corresponding culturing medium based on phase 0 to 3. Each data point represents the mean value of all laser ablation ICP-MS measurements on single foraminiferal chambers built up during the individual culturing phase plotted against the mean metal concentrations in the seawater averaged over the culturing phase (Table 3). Because calculating p and r2 values of the regression lines and the DTE's with the mean per phase resulted in comparable values to when calculating with the overall dataset, we considered this approach adequate. Error bars symbolise the standard error of the mean. The linear regression line (± standard deviation) is displayed when elements showed a significant correlation between seawater and calcite. DTE's of E. excavatum were considered without values for phase 0 of the metal system as only data from one newly formed chamber were available. All values can be found in Table 4. An enlarged graph based on the calculations without phase 3 is provided in the Appendix (Fig. B2).
Table 4Mean heavy-metal-to-calcium values of A. aomoriensis, A. batava and E. excavatum in the control and the metal system. Errors are standard errors of the mean (standard deviation ). Values marked with an asterisk were derived from only one laser spot and thus are not considered for further discussion. Furthermore, the calculated DTE values, the slope of the linear regression line (OLS – ordinary least squares) of all means, Pearson's correlation coefficient (r2) and its significance (p) are given for the calculation with all phases and when removing phase 3 from the calculations. Cases where the regression lines were forced through the origin are indicated. In cases when a regression did not show significant correlation, the DTE range calculated separately from the individual phases is given. In cases when the regression was significant, the DTE values represent the slope of the regression line. Ph is phase; SD is standard deviation. Values in Table S1 are the basis of all calculations.
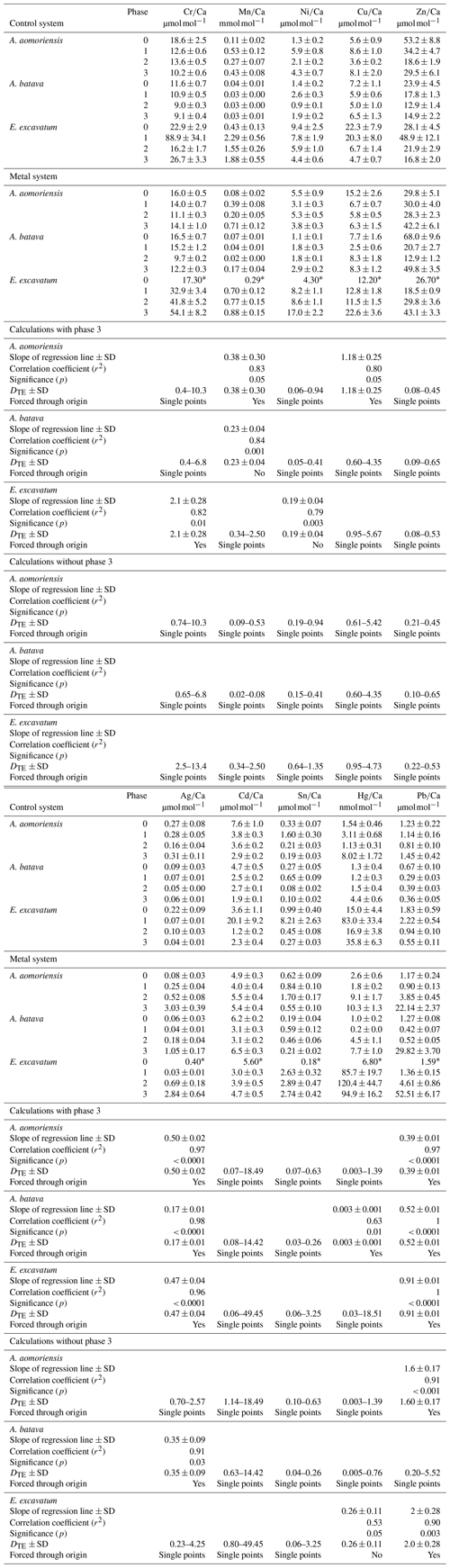
3.2.1 Incorporation of heavy metals into the foraminiferal shell
Measurable incorporation into the foraminiferal calcite was found for all the heavy metals analysed, but the degree of incorporation varied profoundly within and between species (Fig. 4 and Table 4). In both systems, the heavy metal concentration in E. excavatum was higher than in the other species (A. aomoriensis and A. batava) for Cr, Mn, Ni, Hg and Sn. This trend is also visible but less pronounced in the Cu values of the control system.
Cr, Ni, Cu, Zn, Cd, Pb and Ag values of A. aomoriensis displayed the highest standard error of the mean paired with the highest concentrations in the water in the metal system. Sn, Mn and Hg did not show any clear pattern. In the control system, all heavy metal concentrations had higher standard errors of the mean when the concentration of these metals in the culturing medium was higher. The trend was also shown in A. batava and E. excavatum for all heavy metals of the control and the metal system. Note that even though no extra metals were added to the culturing medium of the control system, differences in the heavy metal concentration occurred (Fig. 3 and Table 3).
Calculations were performed with and without phase 3 of the metal system (Figs. 4 and B2 and Table 4) to address a possible overload effect when it comes to higher metal concentrations in the seawater.
When phase 3 was included, a strong positive correlation (r2 > 0.9; p < 0.05) between Ag and Pb concentrations in the foraminiferal shell and the culturing medium was found for all three species. Furthermore, A. batava also displayed a positive correlation for Hg (r2 = 0.63; p < 0.01), as did A. aomoriensis for Cu (r2 = 0.80; p < 0.05) and E. excavatum for Cr (r2 = 0.82; p < 0.01) and Ni (r2 = 0.79; p < 0.003). Weaker but still significant positive correlations were recorded for Mn (r2 > 0.84; p < 0.05) for both Ammonia species. An indistinct correlation of the concentration in the seawater and in the foraminiferal test was recognised for Zn in all three species, whereas Cd and Sn showed no covariance (Fig. 4 and Table 4).
When phase 3 was excluded from the calculations, A. aomoriensis and E. excavatum showed a positive correlation for Pb (r2 > 0.9; p < 0.003), as did A. batava for Ag (r2 = 0.91; p = 0.03), and in E. excavatum Hg correlated more weakly positively (r2 > 0.53; p < 0.05). All other elements showed no significant correlation (Fig. 4 and Table 4).
3.2.2 Partition coefficient (DTE)
The majority of DTE values were lower than 1 in A. aomoriensis (with phase 3, 61 %; without phase 3, 57 %) and A. batava (with phase 3, 75 %; without phase 3, 73 %); i.e. uptake but no enrichment took place. DTE values derived from E. excavatum on the other hand showed a smaller proportion < 1 (with phase 3, 47 %; without phase 3, 42 %). For most elements (Cr, Mn, Ni, Cu, Cd, Sn, Pb and Hg) DTE values derived from E. excavatum were higher than DTE values from the two Ammonia species (Table 4, Fig. 4), which showed comparable DTE values for most elements. DZn formed the exception because all values were within a similar range (DZn ∼ 0.08–0.65) independent of the species. For A. aomoriensis DCu was > 1, and DCd and DPb were also > 1 when phase 3 was excluded from the calculations. Elphidium excavatum displayed DTE values > 1 for Cr and Cu for the calculations with phase 3 and also for Pb without phase 3. The highest variation between minimum and maximum DTE for all species was found for Cd and Hg.
4.1 Experimental uncertainties
Calcein was used for staining the foraminiferal tests before they were placed into the culturing system. It can be assumed that a period of 1 or 2 d for removing excess calcein was sufficient because the youngest chambers were not stained. Calcein binds to Ca and is incorporated into the mineralised calcium carbonate (Bernhard et al., 2004). It is conceivable that the heavy metal incorporation could also be affected by calcein. However, no evidence for such effects has been found so far in a variety of studies (e.g. Hintz et al., 2006; de Nooijer et al., 2007; Dissard et al., 2009). Furthermore, calcein was only used prior to the experiment to mark the last chamber that was grown outside the culturing system. Therefore, the incorporation of the metals measured in subsequent chambers was not affected by the calcein application.
The element concentrations within the culturing medium of each culturing phase were comparably stable for most elements in the control system. In the metal system, the variations were higher, which is due to the sudden input of the multi-metal stock solution for reaching the next phase concentration (Table A1, Fig. B1). This sudden addition of metals resulted in a high peak concentration in the beginning of the new phase, which equilibrated after a while. This trend was most pronounced in phase 3 as the added amount of the multi-metal stock solution was highest for this phase, which was also why the standard error of this phase was comparably high. Furthermore, the variations in the metal concentrations were in a comparable range to those presented in other culturing studies (e.g. Maréchal-Abram et al., 2004; de Nooijer et al., 2007; Munsel et al., 2010; Remmelzwaal et al., 2019). Generally, many other studies (e.g. Remmelzwaal et al., 2019; Sagar et al., 2021a; Titelboim et al., 2021) measured the heavy metal concentration in the seawater less frequently than done in this study. Therefore, the stability of metal concentrations during the culturing phases of those studies is often inferred. Furthermore, pollution events in nature are in most cases not persistent and stable but transient as was mirrored by the concentration changes in our experiments.
The measured metal concentrations in the culturing seawater were smaller than expected (Table 3). This in combination with the varying metal concentration within one phase suggested that several processes were affecting the concentration in such a complex culturing system. One possible mechanism was sorption of the metals onto surfaces (e.g. tubing, culturing vessels, plates, organic matter or the foraminiferal test itself), which could have lowered the metal concentration in the culturing medium. Therefore, sorption could have contributed to the overall budget of the metals. On the other hand, Cu appeared to have been released from components of the culturing system even though the system was cleaned before use and was operated with seawater for 14 d before the experiments began. For instance, the concentration of Cu was high in phase 0, where no metals were added, suggesting release from system parts. In phase 1, the Cu concentration decreased, meaning the contamination derived from the system was removed by a process similar to that observed for the other metals after additions were made. Similar effects have been reported by de Nooijer et al. (2007) for Cu and Havach et al. (2001) for Cd. Other processes like the uptake of the metals by the foraminifera themselves and the growth of algae could further have an influence on the metal concentration in the culturing medium. Germs of algae were introduced accidentally together with the living foraminifera and grew during the experiment. Such processes are difficult to predict and even more challenging to avoid but probably mirror real environments more realistically than sterile petri dish experiments (e.g. Havach et al., 2001; Hintz et al., 2004; Munsel et al., 2010).
Neither the survival rate nor the formation of new chambers was influenced by the elevated metal concentrations during the culturing period. These features were rather constant between the four different phases. Furthermore, no test morphology malformations were recognised. Elevated heavy metal concentrations are thought to induce a higher rate of malformations in benthic foraminifera (e.g. Sharifi et al., 1991; Yanko et al., 1998), whereas recent studies constrained them as a reaction to stressful environments, not necessarily created by high heavy metal concentrations (Frontalini and Coccioni, 2008; Polovodova and Schönfeld, 2008). The lack of malformations in our experiments suggested that the foraminifera were neither poisoned by elevated heavy metal concentrations nor stressed too much by strongly varying environmental parameters, maintaining a normal metabolism and growth. Reproduction was generally very rare, which may indicate that the conditions were not ideal. In field studies foraminiferal reproduction has been linked to short periods of elevated food supply (e.g. Lee et al., 1969; Gooday, 1988; Schönfeld and Numberger, 2007). The regular feeding of foraminifera in our experiment twice a week at constant rates therefore probably did not provide supply levels that trigger reproduction. Nevertheless, it can be assumed that a sufficient amount of food was provided because after the experiments, leftovers covering the sediment surfaces in the cavities were evident. These would have likely been consumed by the foraminifera if they had needed more. Furthermore, the foraminifera calcified, which would not have been the case if any malnourishment had occurred (e.g. Lee et al., 1991; Kurtarkar et al., 2019). Therefore, the nutritional status is unlikely to have influenced the metal uptake by the foraminifera.
The calibrations between the heavy metal concentration in seawater and the foraminiferal shell rely on the values from phase 3 because the difference in seawater concentration was highest compared to other phases. Nevertheless, data points from other phases do play a role, and forcing through the origin adds a further fixed point. High variability for DTE values like those observed here for Cd or Cu is difficult to explain. Such variability suggests there are factors affecting these metals we do not understand, and therefore it is also important to show the data for these elements. Furthermore, the experimental design, especially the mixture of metals, was chosen to best simulate metal conditions in real environments, which could naturally enhance the variability in DTE. This knowledge is indispensable for the application of heavy metal concentrations in foraminifera as a proxy for the heavy metal concentration in seawater.
4.2 Incorporation of heavy metals in the foraminiferal test
Many heavy metals have been demonstrated to be incorporated into the foraminiferal shell (e.g. Cr – Remmelzwaal et al., 2019; Mn – Koho et al., 2015, 2017; Barras et al., 2018; Cu – de Nooijer et al., 2007; Ni – Munsel et al., 2010; Hg – Frontalini et al., 2018a; Cd – Havach et al., 2001; Pb – Frontalini et al., 2018b; Titelboim et al., 2018; Sagar et al., 2021a, b; Zn – Marchitto et al., 2000; Van Dijk et al., 2017), and the incorporation of all of these metals has been measured here. Additionally, to the best of our knowledge, Sn and Ag were investigated here for the first time. The levels observed were well above control values, indicating an elevated incorporation of Ag and Sn into the foraminiferal test calcite with increasing metal concentrations in seawater.
Different factors can influence the incorporation of these metals into the foraminiferal test. First of all, the uptake depends on metabolic pathways during the calcification process. Fundamental biomineralisation processes of foraminifera are the subject of an ongoing discussion, and several (partly) competing models have been proposed (e.g. Elderfield and Erez, 1996; Erez, 2003; de Nooijer et al., 2009b, 2014; Nehrke et al., 2013). One model proposes that the foraminifera take up ions directly from the surrounding seawater by endocytosis or by building seawater vacuoles, which are transported to the site of calcification (SOC) (Elderfield and Erez, 1996; Erez 2003; de Nooijer et al., 2009b, a; Khalifa et al., 2016). The SOC is located outside the foraminiferal cell, and the formation of new calcite takes place in this zone (see de Nooijer et al., 2014, for a summary and illustration). There is evidence that this SOC is separated from the surrounding seawater (e.g. Spindler, 1978; Bé et al., 1979; de Nooijer et al., 2009b, 2014; Glas et al., 2012; Nehrke et al., 2013). The other competing model suggests that the uptake of ions and the transport to the SOC is performed directly from the seawater across the cell membrane by active trans-membrane-transports (TMTs) and/or passive transport via gaps in the pseudopodial network of the foraminifera (Nehrke et al., 2013; de Nooijer et al., 2014). The dependence of heavy metal concentrations in the foraminiferal test on their seawater concentration relies on the prevailing mechanism. Biomineralisation based on endocytosis would infer that the metals' concentration in the seawater is directly mirrored by their concentration in the foraminiferal shell, which is not generally supported by the results of our study except for Ag and Pb. Several metals showed partition coefficients > 1 or < 1 when the DTE's were calculated separately for each culturing phase. Only Pb and Cr in E. excavatum and Cu and Pb in A. aomoriensis consistently displayed mean DTE's > 1 paired with a positive correlation of the concentration in seawater and in the foraminiferal shell, which could indicate a non-selective uptake of these metals, meaning uptake not only driven by the chemical properties of the ion such as the size of the metal ion itself. If this were the case, DTE values > than 1 would be expected especially for metals ions that are smaller than Ca (Rimstidt et al., 1998). On the other hand, the DTE values of many elements (Ni, Zn, Cd, Hg, Pb) dramatically decreased with increasing concentration in the seawater in the highest metal treatment in all species (Fig. 4). This kind of overload effect has also been noted by Nardelli et al. (2016) for Zn, by Barras et al. (2018) for Mn, by Mewes et al. (2015) for Mg and by Munsel et al. (2010) for Ni. Nardelli et al. (2016) suggested that some biological mechanism expels or blocks these metals if the concentration is too high and imminent intoxication is probable, which may be managed by controlling the ion uptake via TMT. Therefore, it may well be possible that the highest concentration of the metals in our study was close to the tipping point of the biological mechanism taking over and protecting the organism.
Besides biologically controlled factors, physicochemical properties also play an important role when it comes to the uptake of ions. One chemical factor is the aqueous speciation and solubility of the metals. Metals with a free ion form with a charge of 2+ are more similar to Ca2+, which makes incorporation more likely (Railsback, 1999). Nearly all metals in this study were added as dissolved chlorides and therefore had a charge of 2+. The only exceptions were Ag, which was added as AgNO3 with a charge of 1+, and Cr, which was added as CrCl3 ⋅ 6 H2O. The charge of the cation as such does not seem to make a major difference as Ag was incorporated into all three species and Cr into E. excavatum with a significant positive correlation with concentrations in the culturing medium. Furthermore, it is possible that the oxidative state of the elements changed due to their pH dependency, which will be discussed for every element separately. Furthermore, other ions with a charge of 1+ are also known to be incorporated into calcite. Examples are Li+ (e.g. Delaney et al., 1985; Hall et al., 2004) and Na+ (e.g. Wit et al., 2013; Bertlich et al., 2018), which are believed to occupy interstitial positions in calcite where the calcite lattice has defects (Ishikawa and Ichikuni, 1984; Okumura and Kitano, 1986). In addition, rare earth elements with a charge of 3+ are also detected in the foraminiferal calcite (e.g. Haley et al., 2005; Roberts et al., 2012).
The aqueous speciation of many metals is strongly influenced by the pH (e.g. Förstner, 1993; Pagnanelli et al., 2003; Spurgeon et al., 2006; Powell et al., 2015; Huang et al., 2017). As the pH during the experiment was stable at around 8.0 ± 0.1 (measured twice a week), speciation changes between phases due to varying pH values can be excluded. However, it is possible that some metals were not available in a form that could be readily incorporated into the calcite such as the free ion or carbonate species. Cr was not available in an optimal speciation to substitute Ca as a pH of 8 would favour Cr3+ or Cr4+ as well as oxides and hydroxides (Elderfield, 1970; Geisler and Schmidt, 1991). Furthermore, the used Cr salt may not have dissolved completely, even though the multi-metal stock solution was heated and stirred during the process. Both factors in combination may lead to the small variation in the seawater concentrations between the different phases. Interferences that could possibly have influenced the Cr measurements in the water samples are chlorine oxides or hydroxides (e.g. Tan and Horlick, 1986; McLaren et al., 1987; Reed et al., 1994; Laborda et al., 1994). Measurements of reference materials revealed slightly elevated Cr concentrations compared to those presented in the literature (Table A2), which indicates that interferences could be responsible for some of the observed variability for Cr. Similar pH-dependent processes could also have affected Cu. Nevertheless, Cu and Cr were taken up by all species, and therefore, this factor cannot be decisive when it comes to incorporation of these metals into the foraminiferal shell.
If the incorporation of metals were straightforward and only depended on the speciation of the metal and other physicochemical factors, the behaviour of the metals would mostly be influenced by the ionic radius in combination with the charge of the metal ions as described for carbonate minerals by Rimstidt et al. (1998). The endocytotic pathway of seawater into the foraminifer should produce a behaviour of ion incorporation comparable to inorganic calcite precipitation. It was found that cations are incorporated into inorganic calcite by substitution of Ca2+ (e.g. Reeder et al., 1999), especially when the effective ionic radius of these ions is comparable to the one of calcium (i.e. 1.0 Å).
Some metals like Mn, Zn and Cu are known to be fundamentally necessary as micronutrients in maintaining the biological and physiological function of a cell (e.g. Mertz, 1981; Tchounwou et al., 2012; Martinez-Colon et al., 2009; Maret, 2016). Therefore, these elements should preferentially be taken up into the foraminiferal cell, where they are used for further processes. This in turn could lead to the consumption of these metals before they can be incorporated into the foraminiferal tests. The artificial sea salt used in this study ensured that these elements were present in a sufficient quantity of micronutrients. All of these ions have a similar ionic radius (Cu, 0.73 Å; Mn, 0.67 Å; Zn, 0.74 Å) in 6-fold coordination (Rimstidt et al., 1998), which would also suggest that their behaviour is comparable. The ionic radii are much smaller than that of Ca but are rather similar to that of Mg (0.72 Å; Rimstidt et al., 1998).
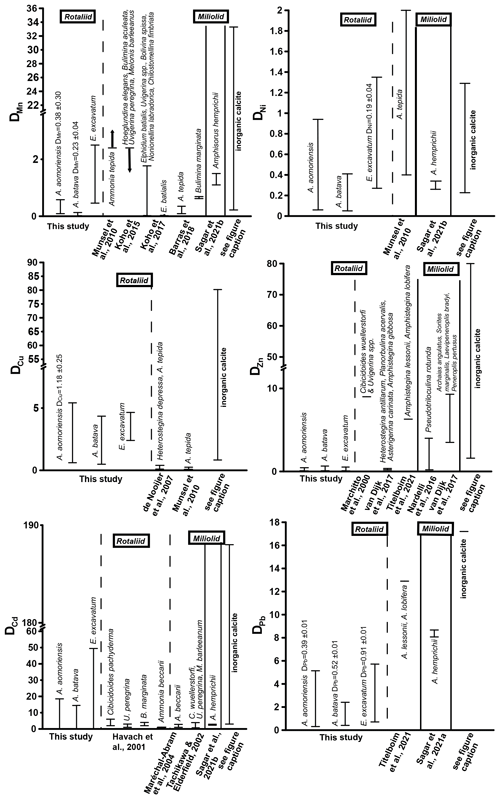
Figure 5Comparison of DTE values of this study with DTE values from the literature of different rotaliid and miliolid foraminiferal species. The range of DTE values based on the different culturing phases is given, and if a correlation between the heavy metal concentration in seawater and the foraminiferal shell was detected, the mean DTE value ± SD (i.e. slope of the regression line) is also indicated. Note that the x axis is clipped for some elements. (Literature for inorganic calcite DTE values: Ni – Rimstidt et al., 1998; Alvarez et al., 2021; Mn – Lorens, 1981; Dromgoole and Walter, 1990; Wang et al., 2021; Cu – Kitano et al., 1973, 1980; Wang et al., 2021; Zn – Kitano et al., 1973, 1980; Rimstidt et al., 1998; Wang et al., 2021; Cd – Rimstidt et al., 1998; Day and Henderson, 2013; Pb – Rimstidt et al., 1998.)
Mn showed a positive correlation between its concentration in seawater and the foraminiferal test in the two Ammonia species when the calculations included phase 3. This indicates that this element serves as a well-behaved proxy influenced mainly by its concentration in seawater. However, E. excavatum did not show this positive correlation. DMn values of this study were comparable with rotaliid and miliolid species and partly with DMn values from inorganic precipitation (Fig. 5). Species-specific partition coefficients of elements like Mg or Na are already reported in the literature (e.g. Toyofuku et al., 2011; Barras et al., 2018; Wit et al., 2013) and could also explain the different DTE values of E. excavatum in this study (see below). Furthermore, it is known that the presence of toxic metals such as Cd, Ni or Hg can inhibit the uptake of essential metals like Mn into the cell if these metals are present in low concentrations (e.g. Sunda and Huntsman, 1998a, b). It is possible that this mechanism is more pronounced in E. excavatum than in the Ammonia species. Zn was clearly incorporated above control levels into all three species, but its behaviour was influenced by more factors than the concentration of Zn in the culturing medium (Fig. 4, Table 4). DZn values of this study are in good agreement with those calculated by Van Dijk et al. (2017) for four hyaline species and Nardelli et al. (2016) for the miliolid Pseudotriloculina rotunda (Fig. 5). Other studies reported higher values. It is again possible that the mixture of metals inhibited the uptake of essential metals like Zn similarly to Mn. Cu showed a simple well-behaved proxy behaviour with a significant positive correlation in A. aomoriensis but not in the other two species. The DCu values presented in the literature for rotaliid species are lower than DCu values from this study. Inorganic values were mostly higher (Fig. 5). These differences could arise from the lower concentration of Cu in this study or from the mixture of metals. It is also reported that the exposure to more than one metal can cause an increased uptake of another metal into the cell (Archibald and Duong, 1984; Martinez-Finley et al., 2012; Bruins et al., 2000; Shafiq et al., 2020). If more Cu is taken up into the cell after the usage of Cu as a micronutrient, more Cu is left over and could possibly be deposited into the calcite. It is therefore conceivable that one particular metal in our study was effecting a co-uptake of Cu, which led to an elevated incorporation into the calcite as compared to other studies.
The non-essential elements Hg, Cd and Pb are not used in physiological processes and are therefore believed to have higher toxic potential (Barbier et al., 2005; Raikwar et al., 2008; Ali and Khan, 2019). This could first of all make the foraminifera prevent the uptake of these metals into their cell. But if the uptake of heavy metals into the cells cannot be prevented, the foraminifera may remove the metals to their shells instead of keeping them in their cells. This is a common mechanism for avoiding intoxication reported for various organisms (benthic foraminifera – Bresler and Yanko, 1995; yeast – Adle et al., 2007; bacteria – Shaw and Dussan, 2015; microalgae – Duque et al., 2019). Furthermore, this would mean that the incorporation of these metals into the foraminiferal calcite increases. The ionic radii of Pb2+ in calcite coordination is 1.19 Å, which is remarkably higher than those of Hg2+ (1.02 Å) and Cd2+ (0.95 Å), which are comparable to Ca. This similarity should also favour the incorporation of Cd and Hg into calcite, which holds only partly true, as Cd showed no trends with complex behaviour, but Hg was linearly incorporated into A. batava and into E. excavatum if the high concentrations of phase 3 were excluded. Pb emerged as a well-behaved proxy under these experimental conditions with all three species incorporating Pb linearly (Fig. 4, Table 4). When comparing DPb values in the literature, our DPb values are slightly lower (Fig. 5). For Hg, no partition coefficients were published so far. DCd values from different studies (Havach et al., 2001; Tachikawa and Elderfield, 2002; Maréchal-Abram et al., 2004; Sagar et al., 2021b) have overall a smaller range of DCd values than found here (Fig. 5). The greater variability in the DCd values of our study makes a comparison difficult.
The importance of other metals like Sn, Cr, Ag and Ni is not fully understood yet, but some of them are believed to have certain biological functions in the cells of animals or plants (Horovitz, 1988; Mertz, 1993; Lukaski, 1999; Pilon-Smits et al., 2009; Hänsch and Mendel, 2009; Chen et al., 2009). For example, Ni is important for plants and bacteria (Poonkothai and Vijayavathi, 2012; Maret, 2016). The ionic radii of these metals in calcite coordination is rather different (Sn, 1.18 Å; Ag, 1.15 Å; Cr, 0.62 Å; Ni, 0.69 Å) and deviate from the ionic radius of Ca2+ too.
Ni was incorporated with a positive trend in E. excavatum but with no clear trend in the Ammonia species (Fig. 4, Table 4). DNi values from rotaliid and miliolid foraminifera and from inorganic calcite are in good agreement with our results (Fig. 5). Ag exhibited a strong positive correlation between seawater and foraminiferal shell in all three foraminiferal species. Partition coefficients for Ag (A. aomoriensis DAg, 0.50 ± 0.02; A. batava DAg, 0.17 ± 0.01; E. excavatum DAg, 0.47 ± 0.04) cannot be compared to other studies as no literature data are available.
Cr and Sn, on the other hand, were not incorporated in a higher amount when the concentration of these metals in the culturing medium was raised, except for Cr in E. excavatum, which showed a positive correlation. The DCr values presented in Remmelzwaal et al. (2019) (DCr > 107), based on culturing experiments with the tropical, symbiont-bearing foraminifera Amphistegina spp., are at least 1 order of magnitude higher than DCr values in this study (A. aomoriensis DCr, 0.74–10.3; A. batava DCr, 0.4–6.8; E. excavatum DCr, 2.1 ± 0.28). One possible reason for the dynamics of Cr is the comparably low concentrations in the culturing medium, and furthermore, the differences between the phases were also very low (Figs. 3 and B1, Table 3). It may be that the concentration of Cr needs to be further elevated and the concentration range needs to be extended before the foraminifera are able to incorporate Cr with significant differences between concentrations. For Sn, no comparative studies are available, so we may speculate that the same could apply for Sn. Nevertheless, we recognised a correlation between the concentration of Cr in the culturing medium and in the foraminiferal calcite of E. excavatum but not for both Ammonia species.
4.3 Interspecies variability
The three different species cultured in this study clearly incorporated the same metal in different ways, which is most visible in the overall higher values of E. excavatum compared to species from the genus Ammonia (Figs. 4 and 5, Table 4). Koho et al. (2017) suggested that these differences in the incorporation result from different microhabitats used by different foraminiferal species. This might be true in nature. In our experiments, however, the sediment in the cavities was only a few millimetres thick and no redox horizon was recognised when recovering the foraminifera after the experiment. Therefore, all foraminifera were living in the same microhabitat. Leftover food may have created a microhabitat, but this effect would have been the same in all cavities and therefore cannot account for the differences between the species. In our experiment, dead Nannochloropsis were fed, which is certainly not the preferred food source for E. excavatum (Pillet et al., 2011). This could lead to a slower growth, and E. excavatum built on average only one chamber during the individual culturing period of 21 d, while Ammonia species built more than four chambers. Furthermore, E. excavatum did not reproduce, even though the culturing period is close to the generation time of this species (Haake, 1962). When growth is slower, it could be possible that a higher amount of a metal is incorporated into the shell, which would lead to higher values in this species. It is possible that a preferred food source would have stimulated enhanced growth and influenced the incorporation of heavy metal into the shells of E. excavatum. For instance, the closely related species E. clavatum prefers bacillariophycean diatoms (Schönfeld and Numberger, 2007). It may also be possible that E. excavatum is simply a more slowly growing species than Ammonia, which seems not to be necessarily connected to a specific food source (e.g. Haynert et al., 2020). One could assume that slower growth would provide more time to remove potentially toxic metals from the cell to the foraminiferal shell, which could explain why E. excavatum incorporated a higher metal concentration than A. aomoriensis and A. batava.
Another possibility for the higher metal concentration found in E. excavatum is the timing of chamber formation. As E. excavatum formed on average one new chamber, it is possible that this chamber was formed during the high peak in the metal concentration during the beginning of the culturing phases (Fig. B1, Table A1). This could in turn lead to a higher uptake of the metals and apparently higher DTE values. Both Ammonia species, on the other hand, formed more chambers, which makes it most likely that the first high concentrations did not particularly influence the overall DTE value. Unfortunately, it is not possible to constrain exactly when the specimens formed their new chambers. It was checked whether the evolution of the metal concentration in seawater of phase 3 was reflected in the intra-test (chamber-to-chamber) data for the two Ammonia species. Particularly, the initial high concentration of certain heavy metals was found in the first chambers of very few individuals after the staining (i.e. the first chamber built in culture). This is most likely due to the individual timing of calcification. Furthermore, it could also be possible that the foraminifera did not calcify during the first high peak due to initial intoxication. Therefore, a mean value over the whole culturing phase was considered the most representative.
Comparing Ammonia and Elphidium species showed that the DTE of the Ammonia species of this study are partly comparable to literature data (Fig. 5).
DTE values are known to be generally higher in tropical high-Mg calcite taxa like Amphistegina (e.g. Titelboim et al., 2021), and also high-Mg miliolid taxa like Amphisorus (e.g. Sagar et al., 2021a) incorporate a higher quantity of metals compared to rotaliid low-Mg taxa like Ammonia or Elphidium. Comparing our data with high-Mg species, it is visible that this trend can be partly confirmed (Fig. 5). For Mn, both Ammonia species of this study show lower values than miliolid species, but the DMn of E. excavatum is comparable. DNi values of Amphisorus hemprichii determined by Sagar et al. (2021b) display the same range as the values for low-Mg species here, and furthermore DZn values of the miliolid P. rotunda (Nardelli et al., 2017) overlap with our findings. On the other hand, DZn values from miliolids in van Dijk et al. (2017) and high-Mg rotaliids from Titelboim et al. (2021) are much higher. The same trend is observed for DPb (Titelboim et al., 2021; Sagar et al., 2021a). When comparing the concentration in the foraminiferal shell directly to values from Titelboim et al. (2018), who analysed the Cu, Zn and Pb concentration in rotaliid and miliolid species from a field site, our values show similarities with both groups. in the foraminiferal calcite of our study was a maximum of ∼ 68 µmol mol−1, which is slightly lower than reported in Titelboim et al. (2018) for the low-Mg species Pararotalia calcariformata (195 µmol mol−1) but much lower than reported for the high-Mg species Lachlanella (2540 µmol mol−1). Differences between the low-Mg species may be due to different concentrations in the seawater that the foraminifera grew in. As the seawater metal concentration is not given in Titelboim et al. (2018), this cannot be evaluated. It may also be possible that high-Mg species have more defects in their tests, which would result in more interstitial space, leading to more space for ions other than Ca. Maximum values of our study are ∼ 23 µmol mol−1 in E. excavatum, which fits the findings of Titelboim et al. (2018) for rotaliid species (P. calcariformata ∼ 21 µmol mol−1) and is lower than in high-Mg species (Lachlanella ∼ 186 µmol mol−1). of ∼ 12 µmol mol−1 in P. calcariformata described by Titelboim et al. (2018) is lower than found here (max in E. excavatum of this study ∼ 53 µmol mol−1), whereas our findings are more comparable to Lachlanella ( ∼ 125 µmol mol−1).
Culturing experiments with different foraminiferal species (A. aomoriensis, A. batava and E. excavatum) that were exposed to a mixture of 10 different metals (Cr, Mn, Ni, Cu, Zn, Ag, Cd, Sn, Hg and Pb) at varying concentrations (Table 3, Figs. 3 and B1) were carried out, and laser ablation ICP-MS analysis of the newly formed calcite revealed the following.
-
All metals used in this study were incorporated into the foraminiferal calcite of all three species (Fig. 4, Table 4).
-
Species-specific differences in the incorporation of heavy metals occurred.
-
The following metals showed a positive correlation between the metal concentration in seawater and the foraminiferal calcite, implying that the uptake of these metals mainly depends on their concentration in seawater:
- a.
Ammonia aomoriensis – DMn, 0.38 ± 0.3; DCu, 1.18 ± 0.25; DAg, 0.50 ± 0.02; DPb, 0.39 ± 0.01;
- b.
Ammonia batava – DMn, 0.23 ± 0.04; DAg, 0.17 ± 0.01; DHg, 0.003 ± 0.001; DPb, 0.52 ± 0.01;
- c.
Elphidium excavatum – DCr, 2.1 ± 0.28; DNi, 0.19 ± 0.04; DAg, 0.47 ± 0.04; DPb, 0.91 ± 0.01.
- a.
-
Other metals like Zn, Sn and Cd showed no clear correlation between seawater and calcite, which may be linked to the mixture of metals leading to synergetic effects.
-
DTE values of Ni, Zn, Cd, Hg and Pb decreased with increasing heavy metal concentration in the seawater, which may be evidence for an early protective mechanism, prior to damage, reduced growth or death of the organism.
The results of this study facilitate the determination of variations in the heavy metal concentration in seawater for elements showing a correlation between ratios in calcite and seawater (A. aomoriensis – Mn, Cu, Ag, Pb; A. batava – Mn, Ag, Hg, Pb; E. excavatum – Cr, Ni, Ag, Pb). Such estimates can be based on foraminiferal samples from the fossil sediment record and recent surface sediments. This facilitates monitoring of anthropogenic footprints on the environment today and in the past. Foraminifera offer the opportunity for long- and short-term monitoring of heavy metal concentration because they store environmental signals over a period of time and not only at one point in time.
Table A1 values from single weeks during the culturing period of the metal system. Measurements were carried out with ICP-MS. These values are the basis for the calculations of the mean values in Table 3 and for Fig. B1.
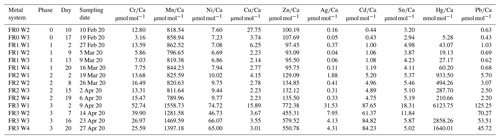
Table A2Average concentration; RSD (1σ in %); literature values; accuracy in comparison to literature values; and number of measurements of the reference materials SLRS-6, SLEW-3, in-house reference materials (South Atlantic surface water and South Atlantic Gyre water) and NASS-6 measured with ICP-MS. Average concentration, RSD and accuracy values displayed here are averaged from single measuring days. Cr values are analysed after dilution of the samples, and all other elements are from analyses after pre-concentration with a seaFAST system.
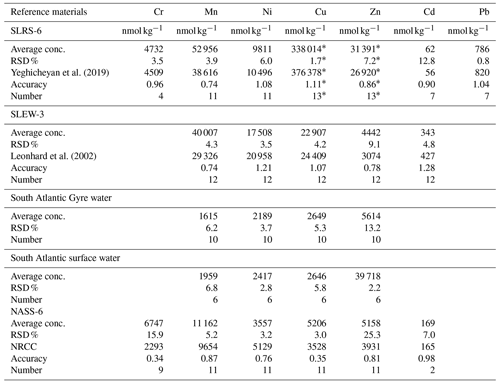
NRCC denotes National Research Council Canada. ∗ Values originated from 1:10 dilution of SLRS-6.
Table A3Average concentration; RSD (1σ in %); literature values; accuracy in comparison to literature values; and number of measurements of the reference materials NIST SRM 614, JCt-1, JCp-1, MACS-3 and ECRM 752-1 measured with LA-ICP-MS. JCt-1NP and JCp-1NP indicate JCt-1 and JCp-1 analysed in the form of nano-particle (NP) pellets. Please note that for ECRM 752-1 no reported values for the elements of interest are available, which is also the case for some elements in other reference materials. It is important to note that the values in the NIST glasses are not reliable as Hg is volatile and most likely volatilised during the glass formation. Average concentration, RSD and accuracy values displayed here are averaged from single measuring days.
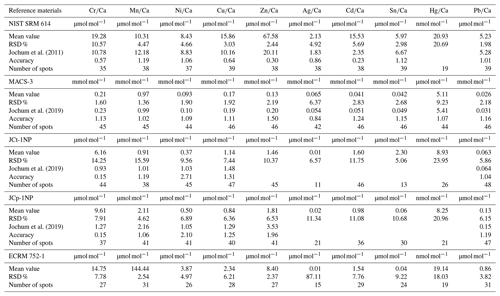
Table A4Comparison of the heavy metal concentrations in seawater of different regions of the world to values used for the culturing experiments in this study. It is indicated whether the values of this study are comparable to environmental values or whether values from this study are higher or lower. EPA denotes Environmental Protection Agency, USA; FI denotes field injection; SF-ICP-MS denotes sector field inductively coupled plasma mass spectrometry; GF denotes graphite atomic; (F)AAS denotes (flame) graphite atomic absorption spectrometry; APDC-MIBK denotes ammonium pyrrolidine dithiocarbamate-methyl isobutyl ketone; ASV denotes anodic stripping voltammetry; AES denotes atomic emission spectrometry; CVAFS denotes cold vapour atomic fluorescence spectrometry; FPD denotes flame photometric detector.
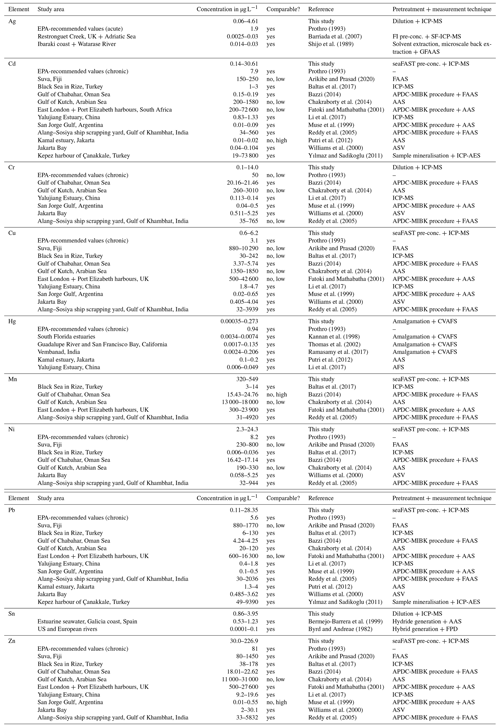
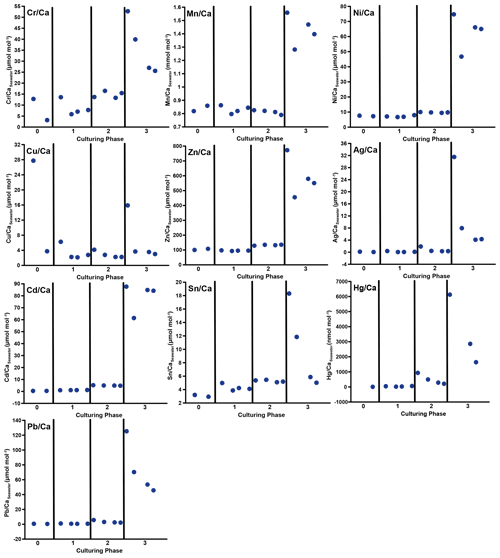
Figure B1 values in the culturing medium of the metal system in µmol mol−1 or nmol mol−1 divided by individual culturing phases. In this system, phase 0 is the control phase without any extra added metals, and for phase 1 to 3, the heavy metal concentration in the culturing medium was elevated. The data the figure is based on can be found in Table A1.
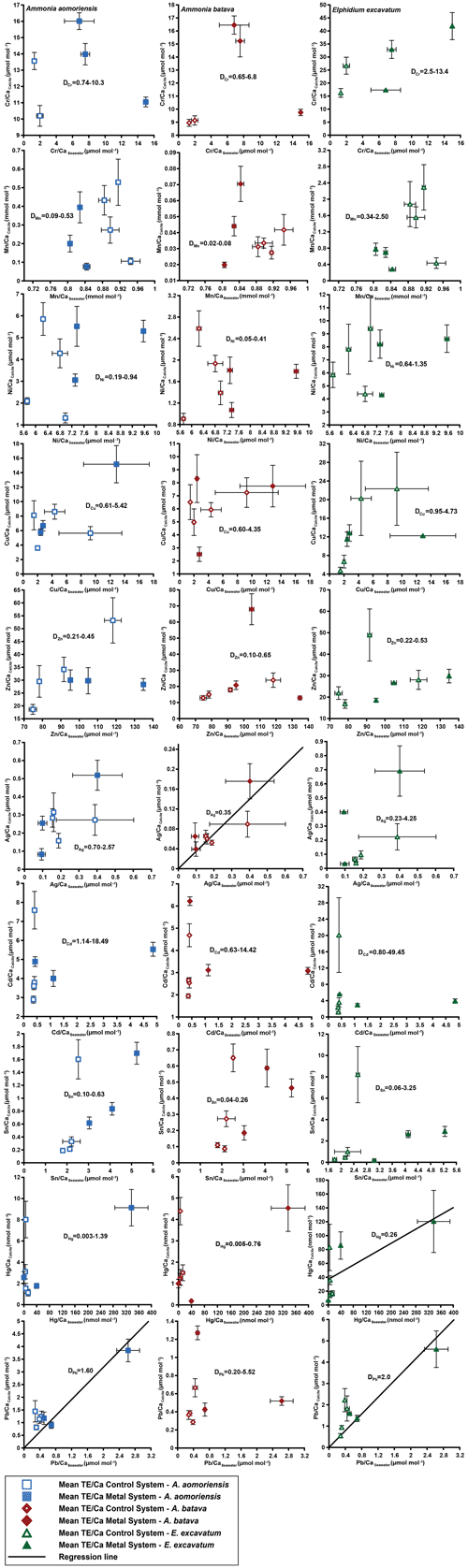
Figure B2Mean values in the foraminiferal calcite versus the mean values in the corresponding culturing medium without phase 3. Each data point represents the mean value of all laser ablation ICP-MS measurements on single foraminiferal chambers built up during the individual culturing phase plotted against the mean metal concentrations in the seawater averaged over the culturing phase (Table 3). Error bars symbolise the standard error of the mean. The linear regression line is based on the calculations excluding phase 3 and is only displayed when elements showed a significant correlation between seawater and calcite. DTE's of E. excavatum are considered without values for phase 0 as only data from one newly formed chamber are available. All values can be found in Table 4.
All data generated or analysed during this study are included in this published article and its supplementary information files.
Tables S1–S3 show TE CaCalcite values from Ammonia aomoriensis (Table S1), Ammonia batava (Table S2) and Elphidium excavatum (Table S3). Values represent single laser ablation spots on foraminiferal chambers that were formed during the individual culturing period in the control and the metal system. Only values above the detection limits of the individual element are presented. Furthermore, outliers are also excluded. These values are the basis for the calculation of the mean TE Ca values in Table 4 and Fig. 4. The sample ID indicates the species (AA is A. aomoriensis; AB is A. batava; E is E. excavatum); the culturing phase; the system (R is metal system; L is control system); the individual; and the chamber that was ablated, starting from the innermost chamber going to the youngest one. The supplement related to this article is available online at: https://doi.org/10.5194/bg-19-629-2022-supplement.
This study was initiated by JS and EH. SaS collected the samples; cultured the foraminifera; processed the samples in the laboratory; and acquired, analysed and interpreted the water and foraminiferal data. JS helped with the sampling logistics, design and implementation of the culturing experiments. EH advised and helped with the processing and analysis of the water samples, and EH and DGS advised and helped with the measurements of the foraminiferal samples. SaS wrote the manuscript with all the authors contributing to the discussion and data interpretation and editing of the work.
The contact author has declared that neither they nor their co-authors have any competing interests.
Publisher's note: Copernicus Publications remains neutral with regard to jurisdictional claims in published maps and institutional affiliations.
We are indebted to Tal Dagan and Alexandra-Sophie Roy, Kiel University, for providing the basic compounds of the culturing setup and for helping us with setting up the systems. Claas Hiebenthal, KIMOCC, had previously helped with the system design. Furthermore, Regina Surberg carried out the ICP-OES measurements, Kathleen Gosnell performed the Hg measurements in the water samples, and Ulrike Westernströer set up and helped with the laser ablation measurements, which were vitally important for this paper. The fieldwork was supported by Schutzstation Wattenmeer on Hallig Hooge, in particular by a guided tour to the Japsand and by providing laboratory facilities at their station. Leif Boyens is thanked for his flexibility and his accommodation space on Hooge. The help of Danny Arndt during fieldwork is gratefully acknowledged. The paper benefited from the suggestions of the two anonymous reviewers and the associate editor Hiroshi Kitazato.
The article processing charges for this open-access publication were covered by the GEOMAR Helmholtz Centre for Ocean Research Kiel.
This paper was edited by Hiroshi Kitazato and reviewed by two anonymous referees.
Adle, D. J., Sinani, D., Kim, H., and Lee, J.: A cadmium-transporting P1B-type ATPase in yeast Saccharomyces cerevisiae, J. Biol. Chem., 282, 947–955, https://doi.org/10.1074/jbc.M609535200, 2007.
Ali, H. and Khan, E.: Trophic transfer, bioaccumulation, and biomagnification of non-essential hazardous heavy metals and metalloids in food chains/webs – Concepts and implications for wildlife and human health, Human Ecol. Risk Assess., 25, 1353–1376, https://doi.org/10.1080/10807039.2018.1469398, 2019.
Alloway, B. J.: Sources of heavy metals and metalloids in soils, in: Heavy Metals in Soils, Environmental Pollution series, vol. 22, edited by: Alloway, B., Springer, Dordrecht, 11–50, https://doi.org/10.1007/978-94-007-4470-7_2, 2013.
Alvarez, C. C., Quitté, G., Schott, J., and Oelkers, E. H.: Nickel isotope fractionation as a function of carbonate growth rate during Ni coprecipitation with calcite, Geochim. Cosmochim. Ac., 299, 184–198, https://doi.org/10.1016/j.gca.2021.02.019, 2021.
Alve, E.: Benthic foraminiferal responses to estuarine pollution: A review, J. Foramin. Res., 25, 190–203, https://doi.org/10.2113/gsjfr.25.3.190, 1995.
Archibald, F. S. and Duong, M.-N.: Manganese acquisition by Lactobacillus plantarum, J. Bacteriol., 158, 1–8, https://doi.org/10.1128/jb.158.1.1-8.1984, 1984.
Arikibe, J. E. and Prasad, S.: Determination and comparison of selected heavy metal concentrations in seawater and sediment samples in the coastal area of Suva, Fiji, Mar. Pollut. Bull., 157, 111157, https://doi.org/10.1016/j.marpolbul.2020.111157, 2020.
Baltas, H., Kiris, E., and Sirin, M.: Determination of radioactivity levels and heavy metal concentrations in seawater, sediment and anchovy (Engraulis encrasicolus) from the Black Sea in Rize, Turkey, Mar. Pollut. Bull., 116, 528–533, https://doi.org/10.1016/j.marpolbul.2017.01.016, 2017.
Barbier, O., Jacquillet, G., Tauc, M., Cougnon, M., and Poujeol, P.: Effect of heavy metals on, and handling by, the kidney, Nephron Physiol., 99, p105-p110, https://doi.org/10.1159/000083981, 2005.
Barras, C., Mouret, A., Nardelli, M. P., Metzger, E., Petersen, J., La, C., Filipsson, H. L., and Jorissen, F.: Experimental calibration of manganese incorporation in foraminiferal calcite, Geochim. Cosmochim. Ac., 237, 49–64, https://doi.org/10.1016/j.gca.2018.06.009, 2018.
Barriada, J. L., Tappin, A. D., Evans, E. H., and Achterberg, E. P.: Dissolved silver measurements in seawater, TrAC-Trend. Anal. Chem., 26, 809–817, https://doi.org/10.1016/j.trac.2007.06.004, 2007.
Bazzi, A. O.: Heavy metals in seawater, sediments and marine organisms in the Gulf of Chabahar, Oman Sea, Journal of Oceanography and Marine Science, 5, 20–29, https://doi.org/10.5897/JOMS2014.0110, 2014.
Bé, A. W. H., Hemleben, C., Anderson, O. R., and Spindler, M.: Chamber formation in planktonic foraminifera, Micropaleontology, 25, 294–307, https://doi.org/10.2307/1485304, 1979.
Bermejo-Barrera, P., Ferrón-Novais, M., González-Campos, G., and Bermejo-Barrera, A.: Tin determination in seawater by flow injection hydride generation atomic absorption spectroscopy, Atom. Spectrosc., 120, 120–125, https://doi.org/10.46770/AS.1999.03.007, 1999.
Bernhard, J. M., Blanks, J. K., Hintz, C. J., and Chandler, G. T.: Use of the fluorescent calcite marker calcein to label foraminiferal tests, J. Foramin. Res., 34, 96–101, https://doi.org/10.2113/0340096, 2004.
Bertlich, J., Nürnberg, D., Hathorne, E. C., de Nooijer, L. J., Mezger, E. M., Kienast, M., Nordhausen, S., Reichart, G.-J., Schönfeld, J., and Bijma, J.: Salinity control on Na incorporation into calcite tests of the planktonic foraminifera Trilobatus sacculifer – evidence from culture experiments and surface sediments, Biogeosciences, 15, 5991–6018, https://doi.org/10.5194/bg-15-5991-2018, 2018.
Bjerrum, N.: Bjerrum's Inorganic Chemistry, 3rd Danish edn., Heinemann, London, 1936.
Boltovskoy, E. and Lena, H.: Seasonal occurrences, standing crop and production in benthic Foraminifera of Puerto Deseado, Contributions from the Cushman Foundation for Foraminiferal Research, 20, 87–95, 1969.
Boyle, E. A.: Cadmium, zinc, copper, and barium in foraminifera tests, Earth Planet. Sc. Lett., 53, 11–35, https://doi.org/10.1016/0012-821X(81)90022-4, 1981.
Boyle, E. A.: Chemical accumulation variations under the Peru Current during the past 130,000 years, J. Geophys. Res.-Oceans, 88, 7667–7680, https://doi.org/10.1029/JC088iC12p07667, 1983.
Bresler, V. and Yanko, V.: Chemical ecology; a new approach to the study of living benthic epiphytic Foraminifera, J. Foramin. Res., 25, 267–279, https://doi.org/10.2113/gsjfr.25.3.267, 1995.
Bruins, M. R., Kapil, S., and Oehme, F. W.: Microbial resistance to metals in the environment, Ecotox. Environ. Safe., 45, 198–207, https://doi.org/10.1006/eesa.1999.1860, 2000.
Byrd, J. T. and Andreae, M. O.: Tin and methyltin species in seawater: Concentrations and fluxes, Science, 218, 565–569, https://doi.org/10.1126/science.218.4572.565, 1982.
Cang, L., Wang, Y.-J., Zhou, D.-M., and Dong, Y.-H.: Heavy metals pollution in poultry and livestock feeds and manures under intensive farming in Jiangsu Province, China, J. Environ. Sci., 16, 371–374, 2004.
Chakraborty, S., Bhattacharya, T., Singh, G., and Maity, J. P.: Benthic macroalgae as biological indicators of heavy metal pollution in the marine environments: A biomonitoring approach for pollution assessment, Ecotox. Environ. Safe., 100, 61–68, https://doi.org/10.1016/j.ecoenv.2013.12.003, 2014.
Chen, C., Huang, D., and Liu, J.: Functions and toxicity of nickel in plants: Recent advances and future prospects, Clean-Soil Air Water, 37, 304–313, https://doi.org/10.1002/clen.200800199, 2009.
Day, C. C. and Henderson, G. M.: Controls on trace-element partitioning in cave-analogue calcite. Geochim. Cosmochim. Ac., 120, 612–627, https://doi.org/10.1016/j.gca.2013.05.044, 2013.
de Nooijer, L. J., Reichart, G. J., Dueñas-Bohórquez, A., Wolthers, M., Ernst, S. R., Mason, P. R. D., and van der Zwaan, G. J.: Copper incorporation in foraminiferal calcite: results from culturing experiments, Biogeosciences, 4, 493–504, https://doi.org/10.5194/bg-4-493-2007, 2007.
de Nooijer, L. J., Langer, G., Nehrke, G., and Bijma, J.: Physiological controls on seawater uptake and calcification in the benthic foraminifer Ammonia tepida, Biogeosciences, 6, 2669–2675, https://doi.org/10.5194/bg-6-2669-2009, 2009a.
de Nooijer, L. J., Toyofuku, T., and Kitazato, H.: Foraminifera promote calcification by elevating their intracellular pH, P. Natl. Acad. Sci. USA, 106, 15374–15378, https://doi.org/10.1073/pnas.0904306106, 2009b.
de Nooijer, L. J., Spero, H. J., Erez, J., Bijma, J., and Reichart, G.-J.: Biomineralization in perforate foraminifera, Earth-Sci. Rev., 135, 48–58, https://doi.org/10.1016/j.earscirev.2014.03.013, 2014.
Delaney, M. L., Bé, A. W. H., and Boyle, E. A.: Li, Sr, Mg, and Na in foraminiferal calcite shells from laboratory culture, sediment traps, and sediment cores, Geochim. Cosmochim. Ac., 49, 1327–1341, https://doi.org/10.1016/0016-7037(85)90284-4, 1985.
Dissard, D., Nehrke, G., Reichart, G. J., Nouet, J., and Bijma, J.: Effect of the fluorescent indicator calcein on Mg and Sr incorporation into foraminiferal calcite, Geochem. Geophy. Geosy., 10, ISSN: 1525-2027, https://doi.org/10.1029/2009GC002417, 2009.
Dissard, D., Nehrke, G., Reichart, G. J., and Bijma, J.: The impact of salinity on the and ratio in the benthic foraminifera Ammonia tepida: Results from culture experiments, Geochim. Cosmochim. Ac., 74, 928–940, https://doi.org/10.1016/j.gca.2009.10.040, 2010a.
Dissard, D., Nehrke, G., Reichart, G. J., and Bijma, J.: Impact of seawater pCO2 on calcification and and ratios in benthic foraminifera calcite: results from culturing experiments with Ammonia tepida, Biogeosciences, 7, 81–93, https://doi.org/10.5194/bg-7-81-2010, 2010b.
Dromgoole, E. L., and Walter, L. M.: Iron and manganese incorporation into calcite: Effects of growth kinetics, temperature and solution chemistry, Chem. Geol., 81, 311–336, https://doi.org/10.1016/0009-2541(90)90053-A, 1990.
Dueñas-Bohórquez, A., da Rocha, R. E., Kuroyanagi, A., Bijma, J., and Reichart, G. J.: Effect of salinity and seawater calcite saturation state on Mg and Sr incorporation in cultured planktonic foraminifera, Mar. Micropaleontol., 73, 178–189, https://doi.org/10.1016/j.marmicro.2009.09.002, 2009.
Duque, D., Montoya, C., and Botero, L. R.: Cadmium (Cd) tolerance evaluation of three strains of microalgae of the genus Ankistrodesmus, Chlorella and Scenedesmus, Rev. Fac. Ing.-Univ. Ant., 88–95, https://doi.org/10.17533/udea.redin.20190523, 2019.
Eggins, S., de Deckker, P., and Marshall, J.: variation in planktonic foraminifera tests: Implications for reconstructing palaeo-seawater temperature and habitat migration, Earth Planet. Sc. Lett., 212, 291–306, https://doi.org/10.1016/S0012-821X(03)00283-8, 2003.
Elderfield, H.: Chromium speciation in sea water, Earth Planet. Sc. Lett., 9, 10–16, https://doi.org/10.1016/0012-821X(70)90017-8, 1970.
Elderfield, H., Bertram, C. J., and Erez, J.: A biomineralization model for the incorporation of trace elements into foraminiferal calcium carbonate, Earth Planet. Sc. Lett., 142, 409–423, https://doi.org/10.1016/0012-821X(96)00105-7, 1996.
Erez, J.: The source of ions for biomineralization in foraminifera and their implications for paleoceanographic proxies, Rev. Mineral. Geochem., 54, 115–149, https://doi.org/10.2113/0540115, 2003.
Fatoki, O. S. and Mathabatha, S.: An assessment of heavy metal pollution in the East London and Port Elizabeth harbours, Water SA, 27, 233–240, https://doi.org/10.4314/wsa.v27i2.4997, 2001.
Flora, G., Gupta, D., and Tiwari, A.: Toxicity of lead: A review with recent updates, Interdisciplinary Toxicology, 5, 47–58, https://doi.org/10.2478/v10102-012-0009-2, 2012.
Förstner, U.: Metal speciation-general concepts and applications, Int. J. Environ. An. Ch., 51, 5–23, https://doi.org/10.1080/03067319308027608, 1993.
Francescangeli, F., Milker, Y., Bunzel, D., Thomas, H., Norbisrath, M., Schönfeld, J., and Schmiedl, G.: Recent benthic foraminiferal distribution in the Elbe Estuary (North Sea, Germany): A response to environmental stressors, Estuar. Coast. Shelf S., 251, 107198, https://doi.org/10.1016/j.ecss.2021.107198, 2021.
Fricker, M. B., Kutscher, D., Aeschlimann, B., Frommer, J., Dietiker, R., Bettmer, J., and Günther, D.: High spatial resolution trace element analysis by LA-ICP-MS using a novel ablation cell for multiple or large samples, Int. J. Mass Spectrom., 307, 39–45, https://doi.org/10.1016/j.ijms.2011.01.008, 2011.
Frontalini, F. and Coccioni, R.: Benthic foraminifera for heavy metal pollution monitoring: A case study from the central Adriatic Sea coast of Italy, Estuar. Coast. Shelf S., 76, 404–417, https://doi.org/10.1016/j.ecss.2007.07.024, 2008.
Frontalini, F., Curzi, D., Giordano, F. M., Bernhard, J. M., Falcieri, E., and Coccioni, R.: Effects of lead pollution on Ammonia parkinsoniana (foraminifera): Ultrastructural and microanalytical approaches, Eur. J. Histochem., 59, 2460, https://doi.org/10.4081/ejh.2015.2460, 2015.
Frontalini, F., Greco, M., Di Bella, L., Lejzerowicz, F., Reo, E., Caruso, A., Cosentino, C., Maccotta, A., Scopelliti, G., and Nardelli, M. P.: Assessing the effect of mercury pollution on cultured benthic foraminifera community using morphological and eDNA metabarcoding approaches, Mar. Pollut. Bull., 129, 512–524, https://doi.org/10.1016/j.marpolbul.2017.10.022, 2018a.
Frontalini, F., Nardelli, M. P., Curzi, D., Martín-González, A., Sabbatini, A., Negri, A., Losada, M. T., Gobbi, P., Coccioni, R., and Bernhard, J. M.: Benthic foraminiferal ultrastructural alteration induced by heavy metals, Mar. Micropaleontol., 138, 83–89, https://doi.org/10.1016/j.marmicro.2017.10.009, 2018b.
Gallego, A., Martín-González, A., Ortega, R., and Gutiérrez, J. C.: Flow cytometry assessment of cytotoxicity and reactive oxygen species generation by single and binary mixtures of cadmium, zinc and copper on populations of the ciliated protozoan Tetrahymena thermophila, Chemosphere, 68, 647–661, https://doi.org/10.1016/j.chemosphere.2007.02.031, 2007.
Garbe-Schönberg, D. and Müller, S.: Nano-particulate pressed powder tablets for LA-ICP-MS, J. Anal. Atom. Spectrom., 29, 990–1000, https://doi.org/10.1039/C4JA00007B, 2014.
Geisler, C.-D. and Schmidt, D.: An overview of chromium in the marine environment, Deutsche Hydrografische Zeitschrift, 44, 185–196, https://doi.org/10.15835/BUASVMCN-VM:63:1-2:2516, 1991.
Glas, M. S., Langer, G., and Keul, N.: Calcification acidifies the microenvironment of a benthic foraminifer (Ammonia sp.), J. Exp. Mar. Biol. Ecol., 424, 53–58, https://doi.org/10.1016/j.jembe.2012.05.006, 2012.
Gooday, A. J.: A response by benthic foraminifera to the deposition of phytodetritus in the deep sea, Nature, 332, 70–73, https://doi.org/10.1038/332070a0, 1988.
Groeneveld, J. and Filipsson, H. L.: and ratios in benthic foraminifera: the potential to reconstruct past variations in temperature and hypoxia in shelf regions, Biogeosciences, 10, 5125–5138, https://doi.org/10.5194/bg-10-5125-2013, 2013.
Guo, X., Xu, B., Burnett, W. C., Yu, Z., Yang, S., Huang, X., Wang, F., Nan, H., Yao, P., and Sun, F.: A potential proxy for seasonal hypoxia: LA-ICP-MS ratios in benthic foraminifera from the Yangtze River Estuary. Geochim. Cosmochim. Ac., 245, 290–303, https://doi.org/10.1016/j.gca.2018.11.007, 2019.
Haake, F. W.: Zum Jahresgang von Populationen einer Foraminiferen-Art in der westlichen Ostsee, Meyniana, 17, 13–27, 1967.
Haake, F.-W.: Untersuchungen an der Foraminiferen-Fauna im Wattgebiet zwischen Langeoog und dem Festland, Meyniana, 12, 25–64, 1962.
Hänsch, R. and Mendel, R. R.: Physiological functions of mineral micronutrients (Cu, Zn, Mn, Fe, Ni, Mo, B, Cl), Curr. Opin. Plant Biol., 12, 259–266, https://doi.org/10.1016/j.pbi.2009.05.006, 2009.
Haley, B. A., Klinkhammer, G. P., and Mix, A. C.: Revisiting the rare earth elements in foraminiferal tests, Earth Planet. Sc. Lett., 239, 79–97, https://doi.org/10.1016/j.epsl.2005.08.014, 2005.
Hall, J. M. and Chan, L.-H.: Li/Ca in multiple species of benthic and planktonic foraminifera: Thermocline, latitudinal, and glacial–interglacial variation, Geochim. Cosmochim. Ac., 68, 529–545, https://doi.org/10.1016/S0016-7037(03)00451-4, 2004.
Hammer, Ø., Harper, D. A. T., and Ryan, P. D.: PAST: Paleontological statistics software package for education and data analysis, available at: http://palaeo-electronica.org/2001_1/past/issue1_01.htm Palaeontol. Electron., 4, 9, 2001.
Havach, S. M., Chandler, G. T., Wilson-Finelli, A., and Shaw, T. J.: Experimental determination of trace element partition coefficients in cultured benthic foraminifera, Geochim. Cosmochim. Ac., 65, 1277–1283, https://doi.org/10.1016/S0016-7037(00)00563-9, 2001.
Haynert, K., Schönfeld, J., Riebesell, U., and Polovodova, I.: Biometry and dissolution features of the benthic foraminifer Ammonia aomoriensis at high pCO2, Mar. Ecol. Prog. Ser., 432, 53–67, https://doi.org/10.3354/meps09138, 2011.
Haynert, K., Gluderer, F., Pollierer, M. M., Scheu, S., and Wehrmann, A.: Food spectrum and habitat-specific diets of benthic Foraminifera from the Wadden Sea–A fatty acid biomarker approach, Frontiers in Marine Science, 7, 815, https://doi.org/10.3389/fmars.2020.510288, 2020.
Hintz, C. J., Chandler, G. T., Bernhard, J. M., McCorkle, D. C., Havach, S. M., Blanks, J. K., and Shaw, T. J.: A physicochemically constrained seawater culturing system for production of benthic foraminifera, Limnol. Oceanogr.-Meth., 2, 160–170, https://doi.org/10.4319/lom.2004.2.160, 2004.
Hintz, C. J., Shaw, T. J., Bernhard, J. M., Chandler, G. T., McCorkle, D. C., and Blanks, J. K.: Trace/minor element: Calcium ratios in cultured benthic foraminifera. Part II: Ontogenetic variation, Geochim. Cosmochim. Ac., 70, 1964–1976, https://doi.org/10.1016/j.gca.2005.12.019, 2006.
Horovitz, C. T.: Is the major part of the periodic system really inessential for life?, J. Trace Elem. Elect. H., 2, 135–144, 1988.
Huang, H., Yuan, X., Zeng, G., Zhu, H., Li, H., Liu, Z., Jiang, H., Leng, L., and Bi, W.: Quantitative evaluation of heavy metals' pollution hazards in liquefaction residues of sewage sludge, Bioresource Technol., 102, 10346–10351, https://doi.org/10.1016/j.biortech.2011.08.117, 2011.
Huang, J., Yuan, F., Zeng, G., Li, X., Gu, Y., Shi, L., Liu, W., and Shi, Y.: Influence of pH on heavy metal speciation and removal from wastewater using micellar-enhanced ultrafiltration, Chemosphere, 173, 199–206, https://doi.org/10.1016/j.chemosphere.2016.12.137, 2017.
Inoue, M., Nohara, M., Okai, T., Suzuki, A., and Kawahata, H.: Concentrations of trace elements in carbonate reference materials coral JCp-1 and giant clam JCt-1 by inductively coupled plasma-mass spectrometry, Geostand. Geoanal. Res., 28, 411–416, https://doi.org/10.1111/j.1751-908X.2004.tb00759.x, 2004.
Ishikawa, M. and Ichikuni, M.: Uptake of sodium and potassium by calcite, Chem. Geol., 42, 137–146, https://doi.org/10.1016/0009-2541(84)90010-X, 1984.
Jan, A. T., Azam, M., Siddiqui, K., Ali, A., Choi, I., and Haq, Q. M.: Heavy metals and human health: Mechanistic insight into toxicity and counter defense system of antioxidants, Int. J. Mol. Sci., 16, 29592–29630, https://doi.org/10.3390/ijms161226183, 2015.
Jochum, K. P., Weis, U., Stoll, B., Kuzmin, D., Yang, Q., Raczek, I., Jacob, D. E., Stracke, A., Birbaum, K., and Frick, D. A.: Determination of reference values for NIST SRM 610–617 glasses following ISO guidelines, Geostand. Geoanal. Res., 35, 397–429, https://doi.org/10.1111/j.1751-908X.2011.00120.x, 2011.
Jochum, K. P., Garbe-Schönberg, D., Veter, M., Stoll, B., Weis, U., Weber, M., Lugli, F., Jentzen, A., Schiebel, R., and Wassenburg, J. A.: Nano-powdered calcium carbonate reference materials: Significant progress for microanalysis?, Geostand. Geoanal. Res., 43, 595–609, https://doi.org/10.1111/ggr.12292, 2019.
Julian, P.: South Florida Coastal Sediment Ecological Risk Assessment, B. Environ. Contam. Tox., 95, 188–193, https://doi.org/10.1007/s00128-015-1583-8, 2015.
Kannan, K., Smith Jr, R. G., Lee, R. F., Windom, H. L., Heitmuller, P. T., Macauley, J. M., and Summers, J. K.: Distribution of total mercury and methyl mercury in water, sediment, and fish from south Florida estuaries, Arch. Environ. Con. Tox., 34, 109–118, https://doi.org/10.1007/s002449900294, 1998.
Kennish, M. J.: Ecology of estuaries: Anthropogenic effects, Vol. 1, CRC Press, Boca Raton, https://doi.org/10.2307/1351438, 2019.
Khalifa, G. M., Kirchenbuechler, D., Koifman, N., Kleinerman, O., Talmon, Y., Elbaum, M., Addadi, L., Weiner, S., and Erez, J.: Biomineralization pathways in a foraminifer revealed using a novel correlative cryo-fluorescence–SEM–EDS technique, J. Struct. Biol., 196, 155–163, https://doi.org/10.1016/j.jsb.2016.01.015, 2016.
Kitano, Y. and Okumura, M.: Coprecipitation of fluoride with calcium carbonate, Geochem. J., 7, 37–49, https://doi.org/10.2343/geochemj.7.37, 1973.
Kitano, Y., Okumura, M., and Idogaki, M.: Abnormal behaviors of copper (II) and zinc ions in parent solution at the early stage of calcite formation, Geochem. J., 14, 167–175, https://doi.org/10.2343/geochemj.14.167, 1980.
Koho, K. A., de Nooijer, L. J., and Reichart, G. J.: Combining benthic foraminiferal ecology and shell to deconvolve past bottom water oxygenation and paleoproductivity, Geochim. Cosmochim. Ac., 165, 294–306, https://doi.org/10.1016/j.gca.2015.06.003, 2015.
Koho, K. A., de Nooijer, L. J., Fontanier, C., Toyofuku, T., Oguri, K., Kitazato, H., and Reichart, G.-J.: Benthic foraminiferal ratios reflect microhabitat preferences, Biogeosciences, 14, 3067–3082, https://doi.org/10.5194/bg-14-3067-2017, 2017.
Kotthoff, U., Groeneveld, J., Ash, J. L., Fanget, A.-S., Krupinski, N. Q., Peyron, O., Stepanova, A., Warnock, J., Van Helmond, N. A. G. M., Passey, B. H., Clausen, O. R., Bennike, O., Andrén, E., Granoszewski, W., Andrén, T., Filipsson, H. L., Seidenkrantz, M.-S., Slomp, C. P., and Bauersachs, T.: Reconstructing Holocene temperature and salinity variations in the western Baltic Sea region: a multi-proxy comparison from the Little Belt (IODP Expedition 347, Site M0059), Biogeosciences, 14, 5607–5632, https://doi.org/10.5194/bg-14-5607-2017, 2017.
Kurtarkar, R. S., Saraswat, R., Kaithwar, A., and Nigam, R.: How will benthic foraminifera respond to warming and changes in productivity?: A Laboratory Culture Study on Cymbaloporetta plana, Acta Geol. Sin.-Engl., 93, 175–182, https://doi.org/10.1111/1755-6724.13776, 2019.
Laborda, F., Baxter, M. J., Crews, H. M., and Dennis, J.: Reduction of polyatomic interferences in inductively coupled plasma mass spectrometry by selection of instrumental parameters and using an argon–nitrogen plasma: Effect on multi-element analyses, J. Anal. Atom. Spec., 9, 727–736, https://doi.org/10.1039/JA9940900727, 1994.
Le Cadre, V. and Debenay, J.-P.: Morphological and cytological responses of Ammonia (foraminifera) to copper contamination: Implication for the use of foraminifera as bioindicators of pollution, Environ. Pollut., 143, 304–317, https://doi.org/10.1016/j.envpol.2005.11.033, 2006.
Lea, D. W., and Spero, H. J.: Assessing the reliability of paleochemical tracers: Barium uptake in the shells of planktonic foraminifera, Paleoceanography, 9, 445–452, https://doi.org/10.1029/94PA00151, 1994.
Lee, J. J., Muller, W. A., Stone, R. J., McEnery, M. E., and Zucker, W.: Standing crop of foraminifera in sublittoral epiphytic communities of a Long Island salt marsh, Mar. Biol., 4, 44–61, https://doi.org/10.1007/BF00372165, 1969.
Lee, J. J., Sang, K., Ter Kuile, B., Strauss, E., Lee, P. J., and Faber, W. W.: Nutritional and related experiments on laboratory maintenance of three species of symbiont-bearing, large foraminifera, Mar. Biol., 109, 417–425, https://doi.org/10.1007/BF01313507, 1991.
Leonhard, P., Pepelnik, R., Prange, A., Yamada, N., and Yamada, T.: Analysis of diluted sea-water at the ng L−1 level using an ICP-MS with an octopole reaction cell, J. Anal. Atom. Spectrom., 17, 189–196, https://doi.org/10.1039/B110180N, 2002.
Li, H., Lin, L., Ye, S., Li, H., and Fan, J.: Assessment of nutrient and heavy metal contamination in the seawater and sediment of Yalujiang Estuary, Mar. Pollut. Bull., 117, 499–506, https://doi.org/10.1016/j.marpolbul.2017.01.069, 2017.
Linshy, V. N., Saraswat, R., Kurtarkar, S. R., and Nigam, R.: Experiment to decipher the effect of heavy metal cadmium on coastal benthic foraminifer Pararotalia nipponica (Asano), J. Palaeontol. Soc. Ind., 58, 205–211, 2013.
Lorens, R. B.: Strontium, cadmium, manganese, and cobalt distribution in calcite as a function of calcite precipitation rate, Geochim. Cosmochim. Ac., 45, 553–561, 1981.
Lukaski, H. C.: Chromium as a supplement, Annual Review of Nutrition, 19, 279–302, https://doi.org/10.1146/annurev.nutr.19.1.279, 1999.
Lutze, G.: Zur Foraminiferen-Fauna der Ostsee, Meyniana, 15, 75–142, 1965.
McLaren, J. W., Beauchemin, D., and Berman, S. S.: Application of isotope dilution inductively coupled plasma mass spectrometry to the analysis of marine sediments, Anal. Chem., 59, 610–613, https://doi.org/10.1021/ac00131a015, 1987.
Marchitto Jr, T. M., Curry, W. B., and Oppo, D. W.: Zinc concentrations in benthic foraminifera reflect seawater chemistry, Paleoceanography, 15, 299–306, https://doi.org/10.1029/1999PA000420, 2000.
Maréchal-Abram, N., Debenay, J.-P., Kitazato, H., and Wada, H.: Cadmium partition coefficients of cultured benthic foraminifera Ammonia beccarii, Geochem. J., 38, 271–283, https://doi.org/10.2343/geochemj.38.271, 2004.
Maret, W.: The metals in the biological periodic system of the elements: Concepts and conjectures, Int. J. Mol. Sci., 17, 66, https://doi.org/10.3390/ijms17010066, 2016.
Martin, P. A. and Lea, D. W.: A simple evaluation of cleaning procedures on fossil benthic foraminiferal , Geochem. Geophy. Geosy., 3, 1–8, https://doi.org/10.1029/2001GC000280, 2002.
Martinez-Colon, M., Hallock, P., and Green-Ruíz, C.: Strategies for using shallow-water benthic foraminifers as bioindicators of potentially toxic elements: A review, J. Foramin. Res., 39, 278–299, https://doi.org/10.2113/gsjfr.39.4.278, 2009.
Martinez-Finley, E. J., Chakraborty, S., Fretham, S. J. B., and Aschner, M.: Cellular transport and homeostasis of essential and nonessential metals, Metallomics, 4, 593–605, https://doi.org/10.1039/c2mt00185c, 2012.
Martín-González, A., Borniquel, S., Díaz, S., Ortega, R., and Gutiérrez, J. C.: Ultrastructural alterations in ciliated protozoa under heavy metal exposure, Cell Biol. Int., 29, 119–126, https://doi.org/10.1016/j.cellbi.2004.09.010, 2005.
McGann, M.: High-resolution foraminiferal, isotopic, and trace element records from Holocene estuarine deposits of San Francisco Bay, California, J. Coastal Res., 24, 1092–1109, https://doi.org/10.2112/08A-0003.1, 2008.
Mertz, W.: The essential trace elements, Science, 213, 1332–1338, https://doi.org/10.1126/science.7022654, 1981.
Mertz, W.: Chromium in human nutrition: A review, J. Nutr., 123, 626–633, https://doi.org/10.1093/jn/123.4.626, 1993.
Mewes, A., Langer, G., Thoms, S., Nehrke, G., Reichart, G.-J., de Nooijer, L. J., and Bijma, J.: Impact of seawater [Ca2+] on the calcification and calcite of Amphistegina lessonii, Biogeosciences, 12, 2153–2162, https://doi.org/10.5194/bg-12-2153-2015, 2015.
Munsel, D., Kramar, U., Dissard, D., Nehrke, G., Berner, Z., Bijma, J., Reichart, G.-J., and Neumann, T.: Heavy metal incorporation in foraminiferal calcite: results from multi-element enrichment culture experiments with Ammonia tepida, Biogeosciences, 7, 2339–2350, https://doi.org/10.5194/bg-7-2339-2010, 2010.
Murray, J. W.: Ecology and palaeoecology of benthic foraminifera, Longman Scientific and Technical, Harlow, Essex, UK, https://doi.org/10.4324/9781315846101, 1991.
Murray, J. W.: Distribution and population dynamics of benthic foraminifera from the southern North Sea, J. Foramin. Res., 22, 114–128, https://doi.org/10.2113/gsjfr.22.2.114, 1992.
Muse, J. O., Stripeikis, J. D., Fernandez, F. M., d'Huicque, L., Tudino, M. B., Carducci, C. N., and Troccoli, O. E.: Seaweeds in the assessment of heavy metal pollution in the Gulf San Jorge, Argentina, Environ. Pollut., 104, 315–322, https://doi.org/10.1016/S0269-7491(98)00096-7, 1999.
Mutwakil, M., Reader, J. P., Holdich, D. M., Smithurst, P. R., Candido, E. P. M., Jones, D., Stringham, E. G., and de Pomerai, D. I.: Use of stress-inducible transgenic nematodes as biomarkers of heavy metal pollution in water samples from an English river system, Arch. Environ. Con. Tox., 32, 146–153, https://doi.org/10.1007/s002449900167, 1997.
Nardelli, M. P., Malferrari, D., Ferretti, A., Bartolini, A., Sabbatini, A., and Negri, A.: Zinc incorporation in the miliolid foraminifer Pseudotriloculina rotunda under laboratory conditions, Mar. Micropaleontol., 126, 42–49, https://doi.org/10.1016/j.marmicro.2016.06.001, 2016.
Nehrke, G., Keul, N., Langer, G., de Nooijer, L. J., Bijma, J., and Meibom, A.: A new model for biomineralization and trace-element signatures of Foraminifera tests, Biogeosciences, 10, 6759–6767, https://doi.org/10.5194/bg-10-6759-2013, 2013.
Nikulina, A., Polovodova, I., and Schönfeld, J.: Environmental response of living benthic foraminifera in Kiel Fjord, SW Baltic Sea, eEarth, 3, 37–49, https://doi.org/10.5194/ee-3-37-2008, 2008.
Nordberg, G. F., Jin, T., Hong, F., Zhang, A., Buchet, J.-P., and Bernard, A.: Biomarkers of cadmium and arsenic interactions, Toxicol. Appl. Pharm., 206, 191–197, https://doi.org/10.1016/j.taap.2004.11.028, 2005.
Nürnberg, D.: Magnesium in tests of Neogloboquadrina pachyderma sinistral from high northern and southern latitudes, J. Foramin. Res., 25, 350–368, https://doi.org/10.2113/gsjfr.25.4.350, 1995.
Nürnberg, D., Bijma, J., and Hemleben, C.: Assessing the reliability of magnesium in foraminiferal calcite as a proxy for water mass temperatures, Geochim. Cosmochim. Ac., 60, 803–814, https://doi.org/10.1016/0016-7037(95)00446-7, 1996.
Okumura, M. and Kitano, Y.: Coprecipitation of alkali metal ions with calcium carbonate, Geochim. Cosmochim. Ac., 50, 49–58, https://doi.org/10.1016/0016-7037(86)90047-5, 1986.
Pagnanelli, F., Esposito, A., Toro, L., and Veglio, F.: Metal speciation and pH effect on Pb, Cu, Zn and Cd biosorption onto Sphaerotilus natans: Langmuir-type empirical model, Water Res., 37, 627–633, https://doi.org/10.1016/S0043-1354(02)00358-5, 2003.
Paton, C., Hellstrom, J., Paul, B., Woodhead, J., and Hergt, J.: Iolite: Freeware for the visualisation and processing of mass spectrometric data, J. Anal. Atom. Spectrom., 26, 2508–2518, https://doi.org/10.1039/C1JA10172B, 2011.
Petersen, J., Barras, C., Bézos, A., La, C., de Nooijer, L. J., Meysman, F. J. R., Mouret, A., Slomp, C. P., and Jorissen, F. J.: Mn/Ca intra- and inter-test variability in the benthic foraminifer Ammonia tepida, Biogeosciences, 15, 331–348, https://doi.org/10.5194/bg-15-331-2018, 2018.
Pillet, L., de Vargas, C., and Pawlowski, J.: Molecular identification of sequestered diatom chloroplasts and kleptoplastidy in foraminifera, Protist, 162, 394–404, https://doi.org/10.1016/j.protis.2010.10.001, 2011.
Pilon-Smits, E. A. H., Quinn, C. F., Tapken, W., Malagoli, M., and Schiavon, M.: Physiological functions of beneficial elements, Curr. Opin. Plant Biol., 12, 267–274, https://doi.org/10.1016/j.pbi.2009.04.009, 2009.
Platon, E., Gupta, B. K. S., Rabalais, N. N., and Turner, R. E.: Effect of seasonal hypoxia on the benthic foraminiferal community of the Louisiana inner continental shelf: The 20th century record, Mar. Micropaleontol., 54, 263–283, https://doi.org/10.1016/j.marmicro.2004.12.004, 2005.
Poignant, A., Mathieu, R., Levy, A., and Cahuzac, B.: Haynesina germanica (Ehrenberg), Elphidium excavatum (Terquem) ls et Porosononion granosum (d'Orbigny), espèces margino-littorales de foraminiféres d'Aquitaine centrale (SO France) au Miocène Moyen (Langhien). Le problème d'Elphidium lidoense Cushman, Revue de Micropaléontologie, 43, 393–405, https://doi.org/10.1016/S0035-1598(00)90200-9, 2000.
Polovodova, I. and Schönfeld, J.: Foraminiferal test abnormalities in the western Baltic Sea, J. Foramin. Res., 38, 318–336, https://doi.org/10.2113/gsjfr.38.4.318, 2008.
Poonkothai, M. and Vijayavathi, B. S.: Nickel as an essential element and a toxicant, International J. Environ. Sci., 1, 285–288, 2012.
Powell, K. J., Brown, P. L., Byrne, R. H., Gajda, T., Hefter, G., Leuz, A.-K., Sjöberg, S., and Wanner, H.: Chemical Speciation of Environmentally Significant Metals: An IUPAC contribution to reliable and rigorous computer modelling, Chemistry International, 37, 15–19, https://doi.org/10.1515/ci-2015-0105, 2015.
Prothro, M. G.: Office of Water policy and technical guidance on interpretation and implementation of aquatic life metals criteria, United States Environmental Protection Agency, Washington, D.C., 1993.
Putri, L. S. E., Prasetyo, A. D., and Arifin, Z.: Green mussel (Perna viridis L.) as bioindicator of heavy metals pollution at Kamal estuary, Jakarta Bay, Indonesia, Journal of Environmental Research and Development, 6, 389–396, 2012.
Raikwar, M. K., Kumar, P., Singh, M., and Singh, A.: Toxic effect of heavy metals in livestock health, Veterinary World, 1, 28, https://doi.org/10.5455/vetworld.2008.28-30, 2008.
Railsback, L. B.: Patterns in the compositions, properties, and geochemistry of carbonate minerals, Carbonate Evaporite., 14, 1–20, https://doi.org/10.1007/BF03176144, 1999.
Ramasamy, E. V., Jayasooryan, K. K., Chandran, M. S., and Mohan, M.: Total and methyl mercury in the water, sediment, and fishes of Vembanad, a tropical backwater system in India, Environ. Monit. Assess., 189, 130, https://doi.org/10.1007/s10661-017-5845-2, 2017.
Reddy, M. S., Basha, S., Joshi, H. V., and Ramachandraiah, G.: Seasonal distribution and contamination levels of total PHCs, PAHs and heavy metals in coastal waters of the Alang–Sosiya ship scrapping yard, Gulf of Cambay, India, Chemosphere, 61, 1587–1593, https://doi.org/10.1016/j.chemosphere.2005.04.093, 2005.
Reed, N. M., Cairns, R. O., Hutton, R. C., and Takaku, Y.: Characterization of polyatomic ion interferences in inductively coupled plasma mass spectrometry using a high resolution mass spectrometer, J. Anal. Atom. Spec., 9, 881–896, https://doi.org/10.1039/JA9940900881, 1994.
Reeder, R. J., Lamble, G. M., and Northrup, P. A.: XAFS study of the coordination and local relaxation around Co2+, Zn2+, Pb2+, and Ba2+ trace elements in calcite, Am. Mineral., 84, 1049–1060, https://doi.org/10.2138/am-1999-7-807, 1999.
Remmelzwaal, S. R. C., Sadekov, A. Y., Parkinson, I. J., Schmidt, D. N., Titelboim, D., Abramovich, S., Roepert, A., Kienhuis, M., Polerecky, L., and Goring-Harford, H.: Post-depositional overprinting of chromium in foraminifera, Earth Planet. Sc. Lett., 515, 100–111, https://doi.org/10.1016/j.epsl.2019.03.001, 2019.
Rimstidt, J. D., Balog, A., and Webb, J.: Distribution of trace elements between carbonate minerals and aqueous solutions, Geochim. Cosmochim. Ac., 62, 1851–1863, https://doi.org/10.1016/S0016-7037(98)00125-2, 1998.
Roberts, N. L., Piotrowski, A. M., Elderfield, H., Eglinton, T. I., and Lomas, M. W.: Rare earth element association with foraminifera, Geochim. Cosmochim. Ac., 94, 57–71, https://doi.org/10.1016/j.gca.2012.07.009, 2012.
Rosenthal, Y., Boyle, E. A., and Slowey, N.: Temperature control on the incorporation of magnesium, strontium, fluorine, and cadmium into benthic foraminiferal shells from Little Bahama Bank: Prospects for thermocline paleoceanography, Geochim. Cosmochim. Ac., 61, 3633–3643, https://doi.org/10.1016/S0016-7037(97)00181-6, 1997.
Rosenthal, Y., Field, M. P., and Sherrell, R. M.: Precise determination of element/calcium ratios in calcareous samples using sector field inductively coupled plasma mass spectrometry, Anal. Chem., 71, 3248–3253, https://doi.org/10.1021/ac981410x, 1999.
Sagar, N., Sadekov, A., Scott, P., Jenner, T., Vadiveloo, A., Moheimani, N. R., and McCulloch, M.: Geochemistry of large benthic foraminifera Amphisorus hemprichii as a high-resolution proxy for lead pollution in coastal environments, Mar. Pollut. Bull., 162, 111918, https://doi.org/10.1016/j.marpolbul.2020.111918, 2021a.
Sagar, N., Sadekov, A., Jenner, T., Chapuis, L., Scott, P., Choudhary, M., and McCulloch, M.: Heavy metal incorporation in foraminiferal calcite under variable environmental and acute level seawater pollution: multi-element culture experiments for Amphisorus hemprichii, Environ. Sci. Pollut. R., 1–14, https://doi.org/10.1007/s11356-021-15913-z, 2021b.
Saha, N., Rahman, M. S., Ahmed, M. B., Zhou, J. L., Ngo, H. H., and Guo, W.: Industrial metal pollution in water and probabilistic assessment of human health risk, J. Environ. Manage., 185, 70–78, https://doi.org/10.1016/j.jenvman.2016.10.023, 2017.
Schlitzer, R.: Ocean Data View, available at: http://odv.awi.de (last access: 21 October 2021), 2016.
Schmidt, S. and Schönfeld, J.: Living and dead foraminiferal assemblage from the supratidal sand Japsand, North Frisian Wadden Sea: distributional patterns and controlling factors, Helgoland Mar. Res., 75, 1–22, https://doi.org/10.1186/s10152-021-00551-2, 2021.
Schönfeld, J. and Numberger, L.: The benthic foraminiferal response to the 2004 spring bloom in the western Baltic Sea, Mar. Micropaleontol., 65, 78–95, https://doi.org/10.1016/j.marmicro.2007.06.003, 2007.
Schweizer, M., Polovodova, I., Nikulina, A., and Schönfeld, J.: Molecular identification of Ammonia and Elphidium species (foraminifera, Rotaliida) from the Kiel Fjord (SW Baltic Sea) with rDNA sequences, Helgoland Mar. Res., 65, 1–10, https://doi.org/10.1007/s10152-010-0194-3, 2011.
Sen Gupta, B. K., Eugene Turner, R., and Rabalais, N. N.: Seasonal oxygen depletion in continental-shelf waters of Louisiana: Historical record of benthic foraminifers, Geology, 24, 227–230, https://doi.org/10.1130/0091-7613(1996)024<0227:SODICS>2.3.CO;2, 1996.
Shafiq, S., Ali, A., Sajjad, Y., Zeb, Q., Shahzad, M., Khan, A. R., Nazir, R., and Widemann, E.: The Interplay between Toxic and Essential Metals for Their Uptake and Translocation Is Likely Governed by DNA Methylation and Histone Deacetylation in Maize, Int. J. Molec. Sci., 21, 6959, https://doi.org/10.3390/ijms21186959, 2020.
Sharifi, A. R., Croudace, I. W., and Austin, R. L.: Benthic foraminiferids as pollution indicators in Southampton Water, southern England, UK, J. Micropalaeontol., 10, 109–113, https://doi.org/10.1144/jm.10.1.109, 1991.
Shaw, D. R. and Dussan, J.: Mathematical modelling of toxic metal uptake and efflux pump in metal-resistant bacterium Bacillus cereus isolated from heavy crude oil, Water Air Soil Poll., 226, 1–14, https://doi.org/10.1007/s11270-015-2385-7, 2015.
Shijo, Y., Shimizu, T., and Tsunoda, T.: Determination of silver in seawater by graphite furnace atomic absorption spectrometry after solvent extraction and microscale back-extraction, Anal. Sci., 5, 65–68, https://doi.org/10.2116/analsci.5.65, 1989.
Smith, C. W., Fehrenbacher, J. S., and Goldstein, S. T.: Incorporation of heavy metals in experimentally grown foraminifera from SAPELO island, Georgia and little duck key, Florida, USA, Mar. Micropaleontol., 101854, https://doi.org/10.1016/j.marmicro.2020.101854, 2020.
Spindler, M.: The development of the organic lining in Heterostegina depressa (Nummulitidae; Foraminifera), J. Foramin. Res., 8, 258–261, https://doi.org/10.2113/gsjfr.8.3.258, 1978.
Spurgeon, D. J., Lofts, S., Hankard, P. K., Toal, M., McLellan, D., Fishwick, S., and Svendsen, C.: Effect of pH on metal speciation and resulting metal uptake and toxicity for earthworms, Environ. Toxicol. Chem., 25, 788–796, https://doi.org/10.1897/05-045R1.1, 2006.
Stankovic, S., Kalaba, P., and Stankovic, A. R.: Biota as toxic metal indicators, Environ. Chem. Lett., 12, 63–84, https://doi.org/10.1007/s10311-013-0430-6, 2014.
Sunda, W. G. and Huntsman, S. A.: Interactions among Cu2+, Zn2+, and Mn2+ in controlling cellular Mn, Zn, and growth rate in the coastal alga Chlamydomonas, Limnol. Oceanogr., 43, 1055–1064, https://doi.org/10.4319/lo.1998.43.6.1055, 1998a.
Sunda, W. G. and Huntsman, S. A.: Processes regulating cellular metal accumulation and physiological effects: Phytoplankton as model systems, Sci. Total Environ., 219, 165–181, https://doi.org/10.1016/S0048-9697(98)00226-5, 1998b.
Tachikawa, K. and Elderfield, H.: Microhabitat effects on and δ13C of benthic foraminifera, Earth Planet. Sc. Lett., 202, 607–624, https://doi.org/10.1016/S0012-821X(02)00796-3, 2002.
Tan, S. H. and Horlick, G.: Background spectral features in inductively coupled plasma/mass spectrometry, Appl. Spectros., 40, 445–460, https://doi.org/10.1366/0003702864508944, 1986.
Tansel, B. and Rafiuddin, S.: Heavy metal content in relation to particle size and organic content of surficial sediments in Miami River and transport potential, Int. J. Sediment Res., 31, 324–329, https://doi.org/10.1016/j.ijsrc.2016.05.004, 2016.
Tchounwou, P. B., Yedjou, C. G., Patlolla, A. K., and Sutton, D. J.: Heavy metal toxicity and the environment, Molecular, clinical and environmental toxicology, 133–164, https://doi.org/10.1007/978-3-7643-8340-4_6, 2012.
Thomas, M. A., Conaway, C. H., Steding, D. J., Marvin-DiPasquale, M., Abu-Saba, K. E., and Flegal, A. R.: Mercury contamination from historic mining in water and sediment, Guadalupe River and San Francisco Bay, California, Geochemistry: Exploration, Environment, Analysis, 2, 211–217, https://doi.org/10.1144/1467-787302-024, 2002.
Thornton, I.: Metals in the global environment: facts and misconceptions, International Council on Metals and the Environment, Ottawa, Canada, 1995.
Titelboim, D., Sadekov, A., Hyams-Kaphzan, O., Almogi-Labin, A., Herut, B., Kucera, M., and Abramovich, S.: Foraminiferal single chamber analyses of heavy metals as a tool for monitoring permanent and short term anthropogenic footprints, Mar. Pollut. Bull., 128, 65–71, https://doi.org/10.1016/j.marpolbul.2018.01.002, 2018.
Titelboim, D., Sadekov, A., Blumenfeld, M., Almogi-Labin, A., Herut, B., Halicz, L., Benaltabet, T., Torfstein, A., Kucera, M., and Abramovich, S.: Monitoring of heavy metals in seawater using single chamber foraminiferal sclerochronology, Ecol. Indic., 120, 106931, https://doi.org/10.1016/j.ecolind.2020.106931, 2021.
Toyofuku, T., Suzuki, M., Suga, H., Sakai, S., Suzuki, A., Ishikawa, T., de Nooijer, L. J., Schiebel, R., Kawahata, H., and Kitazato, H.: and δ18O in the brackish shallow-water benthic foraminifer Ammonia 'beccarii', Mar. Micropaleontol., 78, 113–120, https://doi.org/10.1016/j.marmicro.2010.11.003, 2011.
van Dijk, I., de Nooijer, L. J., and Reichart, G.-J.: Trends in element incorporation in hyaline and porcelaneous foraminifera as a function of pCO2, Biogeosciences, 14, 497–510, https://doi.org/10.5194/bg-14-497-2017, 2017.
Venugopal, B. and Luckey, T. D.: “Toxicology of nonradio-active heavy metals and their salts”, in: Heavy Metal Toxicity, Safety and Hormology, edited by: Luckey, T. D., Venugopal, B., and Hutcheson, D., George Thieme, Stuttgart, 1975.
Vlahogianni, T., Dassenakis, M., Scoullos, M. J., and Valavanidis, A.: Integrated use of biomarkers (superoxide dismutase, catalase and lipid peroxidation) in mussels Mytilus galloprovincialis for assessing heavy metals' pollution in coastal areas from the Saronikos Gulf of Greece, Mar. Pollut. Bull., 54, 1361–1371, https://doi.org/10.1016/j.marpolbul.2007.05.018, 2007.
Wang, G. and Fowler, B. A.: Roles of biomarkers in evaluating interactions among mixtures of lead, cadmium and arsenic, Toxicol. Appl. Pharm., 233, 92–99, https://doi.org/10.1016/j.taap.2008.01.017, 2008.
Wang, Z., Chen, J., Cai, H., Yuan, W., and Yuan, S.: Coprecipitation of metal ions into calcite: an estimation of partition coefficients based on field investigation, Acta Geochimica, 40, 67–77, https://doi.org/10.1007/s11631-020-00443-1, 2021.
Wefer, G.: Umwelt, Produktion und Sedimentation benthischer Foraminiferen in der westlichen Ostsee, Reports/Sonderforschungsbereich 95 Wechselwirkung Meer–Meeresboden, 14, 1–103, Ph. D. dissertation, Kiel University, Germany, 1976.
Williams, T. M., Rees, J. G., and Setiapermana, D.: Metals and trace organic compounds in sediments and waters of Jakarta Bay and the Pulau Seribu Complex, Indonesia, Mar. Pollut. Bull., 40, 277–285, https://doi.org/10.1016/S0025-326X(99)00226-X, 2000.
Wit, J. C., de Nooijer, L. J., Wolthers, M., and Reichart, G. J.: A novel salinity proxy based on Na incorporation into foraminiferal calcite, Biogeosciences, 10, 6375–6387, https://doi.org/10.5194/bg-10-6375-2013, 2013.
Woehle, C., Roy, A.-S., Glock, N., Wein, T., Weissenbach, J., Rosenstiel, P., Hiebenthal, C., Michels, J., Schönfeld, J., and Dagan, T.: A novel eukaryotic denitrification pathway in foraminifera, Curr. Biol., 28, 2536–2543, https://doi.org/10.1016/j.cub.2018.06.027, 2018.
Wokhe, T. B.: Heavy metals pollution of water and sediment in Mada River, Nigeria, Journal of Scientific Research and Reports, 6, 157–164, https://doi.org/10.9734/JSRR/2015/14803, 2015.
Xiang, R., Yang, Z., Saito, Y., Fan, D., Chen, M., Guo, Z., and Chen, Z.: Paleoenvironmental changes during the last 8400 years in the southern Yellow Sea: Benthic foraminiferal and stable isotopic evidence, Mar. Micropaleontol., 67, 104–119, https://doi.org/10.1016/j.marmicro.2007.11.002, 2008.
Yanko, V., Ahmad, M., and Kaminski, M.: Morphological deformities of benthic foraminiferal tests in response to pollution by heavy metals; implications for pollution monitoring, J. Foramin. Res., 28, 177–200, 1998.
Yeghicheyan, D., Aubert, D., Bouhnik-le Coz, M., Chmeleff, J., Delpoux, S., Djouraev, I., Granier, G., Lacan, F., Piro, J.-L., and Rousseau, T.: A New Interlaboratory Characterisation of Silicon, Rare Earth Elements and Twenty-Two Other Trace Element Concentrations in the Natural River Water Certified Reference Material SLRS-6 (NRC-CNRC), Geostand. Geoanal. Res., 43, 475–496, https://doi.org/10.1111/ggr.12268, 2019.
Yılmaz, S. and Sadikoglu, M.: Study of heavy metal pollution in seawater of Kepez harbor of Canakkale (Turkey), Environ. Monit. Assess., 173, 899–904, https://doi.org/10.1007/s10661-010-1432-5, 2011.