the Creative Commons Attribution 4.0 License.
the Creative Commons Attribution 4.0 License.
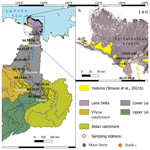
Particulate organic matter in the Lena River and its delta: from the permafrost catchment to the Arctic Ocean
Gesine Mollenhauer
Bennet Juhls
Tina Sanders
Juri Palmtag
Matthias Fuchs
Hendrik Grotheer
Paul J. Mann
Rapid Arctic warming accelerates permafrost thaw, causing an additional release of terrestrial organic matter (OM) into rivers and, ultimately, after transport via deltas and estuaries, to the Arctic Ocean nearshore. The majority of our understanding of nearshore OM dynamics and fate has been developed from freshwater rivers despite the likely impact of highly dynamic estuarine and deltaic environments on the transformation, storage, and age of OM delivered to coastal waters. Here, we studied particulate organic carbon (POC) dynamics in the Lena River delta and compared them with POC dynamics in the Lena River main stem along a ∼ 1600 km long transect from Yakutsk, downstream to the delta. We measured POC, total suspended matter (TSM), and carbon isotopes (δ13C and Δ14C) in POC to compare riverine and deltaic OM composition and changes in OM source and fate during transport offshore. We found that TSM and POC concentrations decreased by 70 % during transit from the main stem to the delta and Arctic Ocean. We found deltaic POC to be strongly depleted in 13C relative to fluvial POC. Dual-carbon (Δ14C and δ13C) isotope mixing model analyses indicated a significant phytoplankton contribution to deltaic POC (∼ 68 ± 6 %) and suggested an additional input of permafrost-derived OM into deltaic waters (∼ 18 ± 4 % of deltaic POC originates from Pleistocene deposits vs. ∼ 5 ± 4 % in the river main stem). Despite the lower concentration of POC in the delta than in the main stem (0.41 ± 0.10 vs. 0.79 ± 0.30 mg L−1, respectively), the amount of POC derived from Yedoma deposits in deltaic waters was almost twice as large as the amount of POC of Yedoma origin in the main stem (0.07 ± 0.02 and 0.04 ± 0.02 mg L−1, respectively). We assert that estuarine and deltaic processes require consideration in order to correctly understand OM dynamics throughout Arctic nearshore coastal zones and how these processes may evolve under future climate-driven change.
- Article
(2142 KB) - Full-text XML
-
Supplement
(653 KB) - BibTeX
- EndNote
Arctic rivers contribute substantial quantities of freshwater and organic matter (OM) to the Arctic Ocean, thereby influencing coastal patterns of stratification, ocean chemistry, and biogeochemistry. These freshwater and OM loads originate from an extensive accumulated pan-Arctic watershed area larger than the Arctic Ocean itself (Terhaar et al., 2021). Arctic rivers, therefore, provide an integrated signature of processes and changes that occur across Arctic watersheds. In these Arctic watersheds, soils and permafrost contain between 1460–1600 Pg of organic carbon (C – within the upper 25 m depth; Hugelius et al., 2014; Strauss et al., 2021a), corresponding to about 2.5 times as much C as that in the current atmosphere and more than half of the organic C stored in soils globally (Köchy et al., 2015). Annual Arctic air temperatures have risen by more than 4 times the magnitude of the global mean air temperature rise (Rantanen et al., 2022), with warming in winter being 4 times greater than in summer (Ballinger et al., 2020). Precipitation is increasing as well and is projected to be > 50 % higher by 2100 (Overland et al., 2014). In response to warming air temperatures, permafrost is thawing (Biskaborn et al., 2019; Fox-Kemper et al., 2021).
Permafrost can modify fluvial processes and their functioning, for example via the occurrence of surface runoff from hillslopes and sediment erosion in river valleys and channels (Tananaev and Lotsari, 2022). Permafrost thaw has consequences for river discharge in several ways. Deepening of the seasonal active layer and the release of waters from melting ground ice result in intensified summer river runoff and increased groundwater storage (Walvoord and Striegl, 2007). In addition, the delayed active-layer freeze-up increases winter river runoff (Walvoord and Kurylyk, 2016; Lamontagne-Hallé et al., 2018; Wang et al., 2021a). Enhanced terrestrial permafrost thaw and intensification of hydrological cycles have the potential to enrich Arctic rivers with remobilised OM and nutrients, modifying food web dynamics and changing the connectivity between terrestrial landscapes and nearshore ecosystems (Brown et al., 2019; Terhaar et al., 2021; Mann et al., 2022). Thus, together with rising temperatures, precipitation, and changes in evapotranspiration, permafrost degradation alters the biogeochemical cycle of the rivers and the freshwater cycle of the Arctic and ultimately modifies river discharge (Carmack et al., 2016; Lique et al., 2016; Vihma et al., 2016; Oliva and Fritz, 2018; Brown et al., 2019; Yang et al., 2021). Permafrost degradation and associated active-layer thickening accelerates riverine carbonate, nitrogen, and phosphorus exports (Zhang et al., 2021) and provides additional C of permafrost origin, especially in summer, fall, and winter (Wild et al., 2019). The permafrost mobilised from catchments that enter a river can subsequently undergo a variety of processes. Once mobilised, OM from permafrost is susceptible to transformation and modification during transport (Vonk et al., 2019). Upon discharge into, and offshore transport within, the Arctic Ocean (Bröder et al., 2018), it is re-buried in marine sedimentary depo-centres, where it is either removed from the active C cycle or re-mineralised further (Grotheer et al., 2020).
The nearshore coastal zone of the Arctic Ocean (including deltas, estuaries, and coasts) is of great importance as a location of terrestrial organic matter burial (Lisitzin, 1994) but also as a biogeochemically active area where major transformation processes of terrestrial material are expected to take place (Tanski et al., 2019; Jong et al., 2020; Sanders et al., 2022). Despite the importance of Arctic estuarine and deltaic environments in OM biogeochemistry, their functioning is still poorly understood; coastal ocean dynamics are inferred from freshwater endmembers based purely on riverine OM properties. Since 2003, the hydrology and biogeochemistry of the greatest (largest) Arctic rivers (Ob, Yenisei, Lena, Kolyma, Yukon, and Mackenzie) have been measured as part of the Arctic Great Rivers Observatory (ArcticGRO; https://arcticgreatrivers.org/, last access: 1 June 2022). Historical records, together with ArcticGRO data, have demonstrated that the long-term increasing freshwater discharge trend has been most pronounced for rivers across the Eurasian Arctic, constituting the strongest evidence of Arctic freshwater cycle intensification (Feng et al., 2021; Shiklomanov et al., 2021a).
Within the framework of the ArcticGRO, depending on the year, between four and six samples were collected per year by the ArcticGRO consortium (Holmes et al., 2021). Samples characterising the OM from the largest Arctic rivers are taken directly from the rivers' main stems rather than from their deltas and estuaries. For example, sampling from the Lena River, the river which transports the largest amount of particulate organic C (POC) of all Arctic rivers (McClelland et al., 2016) to the Arctic Ocean and which has one of the world's biggest deltas (Fedorova et al., 2015), took place ∼ 800 km upstream from the Lena River delta at the town of Zhigansk. This long distance from the sampling site to the areas where the river enters the Arctic Ocean, along with the deficit of information about the delta and the potential biogeochemical processes taking place there (OM transformation, sedimentation, and enrichment), may lead to a distortion or a lack of information about the final state of OM reaching the Arctic Ocean.
In this study, we aim to bridge this gap by characterising POC along the Lena River over a transect from the upper reaches of the Lena River near Yakutsk (approximately 1640 km from the coast) north of the Lena delta in order to decipher the distribution, main sources, and transformation of particulate organic matter (POM) on its way from the permafrost catchment to the Arctic Ocean. Our findings show that the concentration and composition of the POC pool are highly dynamic during transport and that the transformation and storage of riverine OM need to be accounted for when examining and projecting contemporary and future changes, respectively, in coastal processes.
2.1 Study area
The Lena River is one of the greatest Arctic rivers. It discharges approximately 543 km3 yr−1 of water into the Laptev Sea (mean annual discharge in the period 1936–2019; Wang et al., 2021b), which is the second-largest amount of water discharged into the Arctic Ocean out of all the Arctic rivers (Gordeev, 2006; Holmes et al., 2019). The Laptev Sea is a marginal sea of the Arctic Ocean and is a key region controlling the sea-ice formation and drift patterns (Krumpen et al., 2019). Together with the East Siberian Sea and the Russian part of the Chukchi Sea, it constitutes the largest shelf system in the Arctic: the East Siberian Arctic Shelf.
The Lena River is 4400 km long from its origin at 53∘ N, north of Lake Baikal, to 71∘ N, where it reaches the Laptev Sea. It drains an area of ∼ 2.61 × 106 km2, of which more than 94 % is assumed to be underlain by permafrost (mainly continuous – 70.5 %; Obu et al., 2019; Juhls et al., 2020) and is covered by taiga forest (72 % coverage) and tundra ecosystems (12 % coverage; Amon et al., 2012). Running from the south to the north of east Siberia, the Lena River receives OM from various sources within its basin, such as Holocene and Pleistocene deposits (Yedoma), which are widespread across the region and cover approximately 3.5 % of the Lena watershed area (Strauss et al., 2013, 2021b). The Lena River watershed was subdivided into the Aldan and Vilyuy catchments and the upper and the lower Lena, which contribute differently to the TSM and water discharge into the Lena River and are characterised by distinct morphologies (Fig. 1a). Here, we define the Aldan and Vilyuy catchments and the upper and lower Lena according to the area of subcatchments of the Lena River using the HydroSHEDS database (Lehner and Grill, 2013), and we follow the terminology for the subcatchments of Kutscher et al. (2017) and Liu et al. (2005). The separation between the upper and lower Lena was defined approximately 150 km downstream from Yakutsk (Fig. 1a). The upper Lena includes the southern limits of the river and the catchment upstream of the Aldan junction. Its watershed covers an extensive area between Lake Baikal and Yakutsk and includes dozens of tributaries, including creeks and small rivers. The lower Lena consists of the catchment area downstream of the Aldan junction, excluding the catchments of Aldan and Vilyuy. It flows from downstream of Yakutsk into the Laptev Sea and receives waters from catchments including the Verkhoyansk Range (Fig. 1a). The delta of the Lena River occupies an area of 28.5 × 103 km2; it is the largest delta in the Arctic on the Eurasian continent and one of the biggest deltas in the world (Semiletov et al., 2011). The Lena main stem reaches Stolb Island at the apex of the Lena delta (Fig. 1a); there, it divides into numerous branches and more than 800 transverse channels with a total length of 6500 km, forming the delta (Fedorova et al., 2015). About 80 %–90 % of Lena-River-derived water and 85 % of sediments enter the eastern Laptev Sea along two major branches: the Sardakhskaya–Trofimovskaya system (accounting for 60 %–75 % of Lena River water and up to 70 % of sediment discharge, with the Sardakhskaya branch itself transporting 23 %–33 %) and the Bykovskaya branch (accounting for 20 %–25 % of water and up to 15 % of sediment discharge; Ivanov and Piskun, 1999; Charkin et al., 2011). The other two main branches are the Olenekskaya and Tumanskaya (together transporting 5 %–10 % of water and 10 % of sediment discharge), which flow towards the western and central Laptev Sea (Charkin et al., 2011).
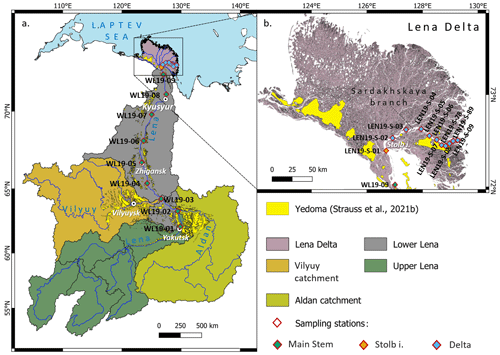
Figure 1The Lena River catchment and its delta, with Yedoma distribution (Strauss et al., 2021b) in the catchment area. (a) Sampling locations along the Lena River main stem (n=9) and the Lena River catchment area; (b) sampling locations at Stolb Island (n=3) and in the Lena delta along the Sardakhskaya branch (n=20).
Nowadays, constant patterns of shifting temperature and precipitation regimes are observed in the Lena watershed (Pohl et al., 2020), which is characterised by permafrost and is particularly sensitive to climate change. Thus, over the past 84 years, the discharge of the Lena River has increased by 22 % (Wang et al., 2021b) and has reached as much as 626 km3 yr−1 in the years 2016–2018 compared to an average of 557 km3 yr−1 in the years 1981–2010 (Holmes et al., 2019). Nevertheless, characteristics of OM discharged from the Lena River are measured by ArcticGRO, which collects water samples near Zhigansk, located ∼ 800 km upstream from the Lena delta. It is also known that a significant fraction of suspended matter carried by the Lena River is deposited before the Lena reaches Kyusyur along a narrow part of the Lena main stem referred to as the Lena pipe (Semiletov et al., 2011; Fedorova et al., 2015). Since the ArcticGRO sampling site is far from where the Lena runoff enters the Arctic Ocean, biogeochemical processes taking place downstream from Zhigansk and particularly in the delta are not reflected in the ArcticGRO data. Thus, the properties of water and suspended materials sampled at Zhigansk may in fact not be entirely representative of the discharge to the ocean.
2.2 Sampling
In this study, we sampled along the Lena main stem from Yakutsk to Stolb Island and then onward through the Sardakhskaya branch. Samples from the Lena main stem and its delta were taken separately during two independent expeditions in 2019. The first sampling campaign took place between 20 and 30 June 2019 and covered a transect along the Lena main stem. Surface water was taken along a 1300 km long transect from Yakutsk to the Lena delta (Fig. 1a) on board the vessel Merzlotoved. Sampling took place at nine sites approximately every 150 km on the way to the Lena delta. This transect intersects the location of the perennial ArcticGRO observation programme and includes a sampling station downstream of the town of Zhigansk (station WL19-05). Water samples were taken from the surface (∼ 1 m water depth) using a pre-cleaned plastic bucket. The water samples were stored in 500 mL high-density polyethylene (HDPE) bottles (pre-cleaned with 10 % HCl for 3 d) and immediately frozen at −10 ∘C for preservation. The second sampling campaign started on 7 August 2019. The aim of the expedition was to conduct a detailed investigation along the Sardakhskaya branch, from Stolb Island to the eastern Laptev Sea (Fuchs et al., 2021). The distance between the sampling locations varied between ∼ 20 and ∼ 5 km, with coarser sampling at the beginning of the transect near Stolb Island and increasing spatial resolution towards the Laptev Sea (Fig. 1b).
We used a 5 L water sampler (UWITEC, Austria) to collect water from one to three depths per station (depending on the bottom depth at the sampling location). We took surface water (0–1 m) at each sampling site. If the river depth at a location was 3–7 m, two depths were sampled: surface water (0–1 m) and above the bottom depth (3–7 m). When water depth exceeded 7 m, water was sampled at three depths: (1) surface (0–1 m), (2) mid-depth in the water column (3–7 m), and (3) just above the river bottom (8–18 m). The water samples were collected in 20 L plastic canisters, which were pre-cleaned with 10 % HCL and kept cool until return to the laboratory on Samoylov Island, where the samples were further analysed.
Additionally, samples from previous Lena delta expeditions were used for this study. Those samples were taken near Stolb Island in 2016 (8 and 15 August) and in 2017 (11 and 25 July). This collection of samples and further analyses were conducted in the same way as for the samples from the riverine deltaic transects obtained in 2019.
2.3 Laboratory analyses
2.3.1 Total suspended-matter concentration
Directly after sampling (delta transect) or after 40 d of freezing (Yakutsk–delta transect), samples were delivered to the Samoylov Island research station laboratory, where they were processed for further analyses. Water was filtered through pre-combusted (4.5 h, 450 ∘C) and pre-weighed glass fibre filters (GF/F Whatman, 0.75 µm membrane, ∅ 2.5 cm) for total suspended-matter (TSM) content, POC concentration, and C isotope analysis. Filters were stored frozen in pre-combusted glass petri dishes. After filtration, the filters were dried for 24 h at 40 ∘C and then were weighed. We used the difference in weights between dried filters with TSM and pre-weighed empty filters to calculate TSM concentration per unit volume of water (mg L−1).
2.3.2 Particulate organic carbon concentration, carbon isotope analyses (Δ14C, δ13C), and relative organic carbon content in total suspended matter
After calculation of TSM, selected filters (different replicates from the same water sample) were further processed (1) to determine total POC concentration (mg L−1) together with stable C isotopes and (2) to determine Δ14C of POC. For this purpose, in both cases, filters were acidified with 10 % HCl to remove inorganic C (sufficient HCl to wet the filter surface, including its sediment). Then they were dried again for 24 h at 40 ∘C and packed or rolled into small tin boats ( mm; Mollenhauer et al., 2021). For filters with TSM concentrations above 20 mg L−1, it was expected that the C contents of the filter exceeded 100 µg. For those samples, only a subsample of the filter was used.
POC content on the filter and its δ13C signature were measured on a Sercon 20–20 isotope ratio mass spectrometer (IRMS) coupled to an automated nitrogen carbon analyser (ANCA). Stable C isotope values were expressed as δ13C in per mil (‰) and normalised against the Peedee belemnite (PDB) standard.
Precision and accuracy of the isotope ratios and C masses were assessed by repeated analysis of in-house standards (isoleucine, National Institute of Standards and Technology, RM 8573, USGS40) with known isotopic composition (−26.39 ± 0.09 ‰). The precision of δ13C measurements was better than ± 0.2 ‰, the mean uncertainty for POC was ± 3.34 µg, and the concentrations were determined by dividing the POC content per filter by the volume of water filtered through that filter.
The relative organic carbon (OC) content of the TSM (OCTSM, wt %) was calculated by dividing the sample POC content by the TSM content (Eq. 1).
Radiocarbon dating by means of an accelerator mass spectrometer (AMS) was achieved on a MIni CArbon Dating System (MICADAS) following Mollenhauer et al. (2021). We report radiocarbon data as Δ14C values in ‰, which expresses the relative difference in 14C activity between the absolute international standard (reference year 1950) and the sample activity corrected for sampling time and normalised to δ13C = 25 ‰ (Stuiver and Polach, 1977). A blank sample was determined by means of five empty combusted blank filters (GF/F, 2.5 cm ∅) treated identically to the samples (Mollenhauer et al., 2021).
Since radiocarbon analysis is commonly used as a method for determining OM age (Stuiver and Polach, 1977), for discussion of the results, we referred to more 14C-depleted samples as ancient C from ancient OM sources (less than −900 ‰ or ∼ 18 500 Δ14C years), and we referred to more 14C -enriched samples (in the range between −50 ‰ and −900 ‰ or ∼ 400–18 500 Δ14C years) as old and to the samples with Δ14C above −50 ‰ as young.
2.3.3 Data representation and calculations
The data used in this study were subdivided into three groups according to the sampling locations and the differences in the hydrological regimes and studied parameters: (1) samples from the Lena River main channel along the sampling transect from Yakutsk to the Lena delta, (2) samples from near Stolb Island at the apex of the Lena River main stem and the Lena delta, and (3) samples from the Sardakhskaya branch in the Lena delta itself. We discuss our data in the context of a compilation of summer data obtained by the ArcticGRO initiative (group 4). This dataset includes parameters measured from 2004 to 2019 (Holmes et al., 2021) in the summer between 15 June and 31 August. This subset of ArcticGRO samples was chosen to allow for a direct comparison of the published results with data presented here and to avoid extreme events of the hydrologic system like the spring–early summer ice breakup (maximum water and TSM discharge) and winter ice cover (minimum water and TSM discharge; Magritsky et al., 2018).
The discharge data are provided by the Russian Federal Service for Hydrometeorology and Environmental Monitoring (Roshydromet, published by Shiklomanov et al., 2021b) for the Lena River at Kyusyur (70.68∘ N, 127.39∘ E; see Fig. 1a).
The groups of data defined for this study are described in Figs. 2–5 and Table 2 as “Main stem”, “Delta”, “Stolb”, and “ArcticGRO”.
Endmember modelling analysis was performed to derive quantitative estimates of the relative input of a potential C source endmember into the POC pool of every water sample; this is described in detail in Sect. 4.2.3.
3.1 Depth distribution
We sampled water in the delta from different depths to investigate the distribution of OM through the water profile in the deltaic ecosystem. We did not observe systematic and significant differences between TSM, POC concentration, OC content, or carbon isotopes of POC for different water depths. Previous measurements using a conductivity, temperature, and depth (CTD) probe during the sampling campaign showed no temperature or conductivity stratification of the water profile (Fuchs et al., 2022). Thus, to characterise other studied parameters in the Lena delta, we considered all the data measured in the delta from all water depths to be one dataset without further subdividing the values into depth groups.
3.2 Total suspended matter; particulate organic carbon and its content in total suspended matter
3.2.1 Total suspended matter
TSM concentration varied strongly between the river main stem, which exhibits high spatial variability, and the Lena River delta, where TSM was distributed homogeneously (Fig. 2a). The concentration of TSM from the main stem decreased from the upstream catchment to the delta, from 34.5 to 15.0 mg L−1, with an average of 22.7 ± 6.3 mg L−1 (mean ± SD). We measured the highest TSM concentration at a station 150 km downstream from Yakutsk (WL19-02), where the largest Lena tributary (Aldan) flows into the main stem (Figs. 1a, 2a). From there on, TSM concentrations consistently decreased, reaching a minimum at the two stations closest to the delta near Stolb Island (WL19-08 and WL19-09).
Compared to the Lena River main-stem transect, the TSM concentrations in the delta were lower and rather homogeneous, displaying no obvious trends in spatial distribution. The highest TSM concentrations in the delta were found for several samples along the entire transect: 20.0 mg L−1 40 km downstream from Stolb Island (LEN19-S-03), 16.4 mg L−1 in the middle of the Sardakhskaya branch (LEN19-S-05), and 19.4 and 18.8 mg L−1 closer to the delta outlet (LEN19-S-08 and LEN19-S-89). The mean concentration of TSM for the delta was 9.3 ± 5.2 mg L−1, which is lower than in the river main stem. The mean TSM concentration at Stolb Island (transition zone between the Lena main stem and the delta) was 8.6 ± 3.7 mg L−1, with a maximum of up to 13.8 mg L−1 in the middle of the profile, which is within the range of the lowest values measured in the main-stem transect and the average deltaic values (Fig. 1a).
TSM reported in the ArcticGRO dataset varied within a greater range than our main-stem-sample result (7.6 and 51.0 mg L−1); nevertheless, the average TSM (27.8 ± 11.3 mg L−1) was similar to that of our main-stem-sample result.
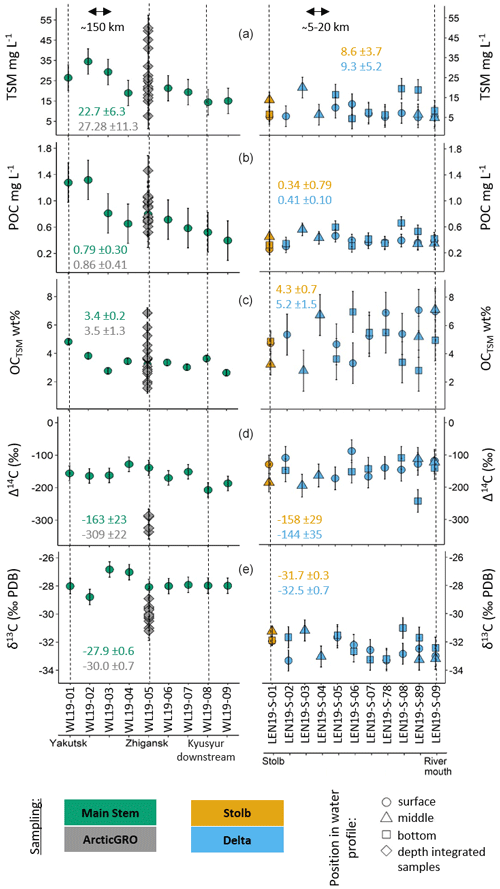
Figure 2Distribution of studied parameters along the transect in the Lena main stem and the Lena delta and for the ArcticGRO dataset (mean ± SD); discrete values displayed on each panel represent the average (± SD) for every sampling group: (a) TSM mg L−1; (b) POC, mg L−1; (c) OCTSM, wt %; (d) Δ14C of POC, ‰; (e) δ13C of POC, ‰.
3.2.2 Particulate organic carbon and its content in total suspended matter
The distribution pattern of POC concentrations along the Lena River main stem was similar to that of TSM concentrations (Fig. 2b). POC decreased by ∼ 70 % downstream, from 1.32 mg L−1 at Yakutsk (WL19-01) to 0.40 mg L−1 at the last station (WL19-09). The mean POC concentration in the Lena River was 0.79 ± 0.30 mg L−1. The highest POC concentration was measured at the two southernmost sites, WL19-01 and WL19-02 (1.32 and 1.28 mg L−1, respectively).
Deltaic POC concentration was, on average, lower than riverine POC, with a mean of 0.41 ± 0.10 mg L−1 (range 0.26–0.66 mg L−1). The samples with high TSM had high POC concentrations as well. These values were similar to the range of POC concentrations found for the second half of the river main-channel transect.
Like TSM, POC concentrations provided by the ArcticGRO database were very similar to the values we obtained in this study. The average POC concentration from ArcticGRO is 0.86 ± 0.41 mg L−1, within the range of 0.52–1.46 mg L−1.
The POC content in TSM (OCTSM, wt %) from main-stem samples was relatively constant along the complete river transect, with a mean OCTSM of 3.4 ± 0.2 wt %. The southernmost station (WL19-01, Yakutsk) was an exception, being enriched in suspended matter and especially in POC (TSM: 26.5; POC: 1.28 mg L−1); in addition, OCTSM was slightly higher than the transect average (4.8 wt %; Fig. 2c). In the deltaic waters, OCTSM was significantly higher than in main-stem samples, and the values within the delta increased from Stolb Island (4.3 ± 0.7 wt %; LEN19-S-01) toward the river mouth (up to 7.1 wt% for LEN19-S-09, sampled at 5 m depth). The average OCTSM in the delta was 5.2 ± 1.5 wt %, with a minimum as low as 2.8 w % for stations with the highest TSM and POC concentrations (40 km downstream from Stolb Island, LEN19-S-03).
OCTSM at Zhigansk from ArcticGRO was within a similar range as the values we found in the main Lena River channel. The average value was 3.5 ± 1.3 wt %. The two lowest values of 1.9 wt % were measured for samples with very high TSM and POC concentrations. Two high values stood out from the other values and were measured in the sample with the lowest POC and TSM concentrations. The mean OCTSM within ArcticGRO was not significantly different from the mean POC content in the river transect data, with values of 3.6 % and 3.4 %, respectively.
3.3 Isotopic composition of particulate organic carbon
3.3.1 Δ14C of particulate organic carbon
Radiocarbon levels of POC varied within a wide range between −243 ‰ and −88 ‰ (between 2236 and 740 Δ14C years mean age, respectively) along the entire transect (Fig. 2d) and did not differ substantially between river main-stem and delta samples. The mean radiocarbon value for the main-stem part of the transect was −163 ± 23 ‰ (range from −207 ‰ to −128 ‰). The Δ14C of POC at the first three upstream main-stem stations (WL19-01, -02, and -03) were similar at −160 ± 3 ‰. Further downstream, Δ14C increased to −128 ‰ at the station ∼ 150 km upstream from Zhigansk (WL19-04) and decreased to −207 ‰ at station WL19-08 on the way to Stolb Island (LEN19-S-01), where it was −158 ‰. In the delta, the mean Δ14C ‰ was −144 ± 35 ‰, with a minimum of −243 ‰ at station LEN19-S-89 before the delta discharges into the sea and a maximum of −88 ‰ at LEN19-S-06 in the middle of the delta transect. Thus, Lena delta POC in 2019 was within the same age as or was younger (higher Δ14C concentration) than POC in the Lena River main stem.
ArcticGRO reported significantly lower Δ14C values than what we found. The average Δ14C value was −309 ‰, while our value for Zhigansk in 2019 was −164 ‰ (WL19-05), which was similar to the average for the entire transect in 2019 (−163 ± 23 ‰).
3.3.2 δ13C of particulate organic carbon
A strong difference was noted in the δ13C of POC. In the main stem, δ13C values were significantly higher than in the delta and at Stolb, with means of −27.9 ± 0.6 ‰ in the main stem and −31.7 ‰ ± 0.3 ‰ and −32.5 ± 0.7 ‰ at Stolb and in the delta, respectively (Fig. 2e). Values within the main-stem part of the transect ranged between −28.8 ‰ and −26.8 ‰, with higher values for stations WL19-03 (−26.8 ‰) and WL19-04 (−27.0 ‰) after the Aldan and Vilyuy Lena tributaries exit the Lena main stem. The δ13C values of POC within the delta and at Stolb displayed slightly more spatial variation, with values between −33.1 ‰ and −31.0 ‰ and no specific distribution trend; thus, the lowest value of −33.3 ‰ was measured at several deltaic stations (LEN19-S-02, LEN19-S-07, LEN19-S-78, and LEN19-S-89; Fig. 2e). The δ13C of POC reported by ArcticGRO was −30.0 ± 0.7 ‰, which was in between the values measured by us in 2019 at the Lena main stem and delta.
4.1 Organic carbon load in the Lena main stem and the Lena delta
The TSM and POC concentrations measured in 2019 displayed generally higher and more variable values along the Lena River main stem than in the delta, while the OC content of TSM was higher in the Lena delta (Fig. 2c). This pattern could be explained by (a) the local flow regime and its hydrological changes, such as flow velocity, water and sources distribution, and mean catchment slope along the Lena main stem and its delta; (b) the time of sampling relative to the annual spring–summer flooding and the discharge fluctuations; or (c) a combination of both factors. In the following, we discuss these factors in detail.
4.1.1 Discharge and sampling time
The Lena River is characterised by a nival hydrograph regime, with a distinct flood event in the beginning of summer during the snowmelt and ice breakup period (May–June) and a very low water flow in winter (Yang et al., 2002). Discharge has a strong effect on the amount of solids and OM released by a river (Magritsky et al., 2018). The peak of annual POC concentrations (> 3.6 mg L−1; McClelland et al., 2016) and TSM concentrations (> 150 mg L−1; ArcticGRO) in the Lena River occurs right after the flooding following ice breakup in late May–early June.
The peak water yield in 2019 took place on 2 June, reaching 83 000 m3 s−1, then it decreased and varied in the range of 49 200–45 999 m3 s−1 during the time interval when the main-stem transect was sampled (Shiklomanov et al., 2021b). During the sampling in the delta (7–9 August 2019), the discharge was 19 600–19 000 m3 s−1, which was less than half the discharge during main-stem sampling.
We assessed all ArcticGRO data on TSM and POC for the Lena River to demonstrate that TSM and POC concentrations were correlated with discharge (Fig. S1). However, when considering a discharge ranging between 15 000 and 50 000 m3 s−1 (the typical range of discharge values in summer (including 2019) and in September), there is no significant relationship between discharge and TSM and POC concentrations (Fig. S2). Thus, the strong relationship between discharge and TSM and POC appears to be driven by the large difference between the maxima and minima in all parameters (observed during the spring flood and during low flow in winter, respectively).
Average surface water TSM and POC concentrations in the Lena River in 2019 agree with average TSM and POC concentrations observed by ArcticGRO during periods with discharge within the mentioned range (TSM and POC of 21.29 and 0.77 mg L−1 in this study as compared with 22.66 and 0.79 mg L−1 for ArcticGRO, respectively). TSM and POC concentrations in the Lena delta in summer 2019 were 2 and 1.5 times lower, respectively, than values reported by ArcticGRO for a comparable time of year and under similar discharge conditions (TSM: 9.3 ± 5.2; POC: 0.41 ± 0.10 mg L−1; Fig. 2a and b). On the other hand, our deltaic POC concentrations are similar to previously published POC data for the Lena delta (Winterfeld et al., 2015; Fig. 3). This shows that the difference we observe between river and delta is a persistent feature that is not biased by sampling time or depth but is mostly caused by other factors such as, e.g. flow and velocity.
4.1.2 Hydrology of the Lena River
The first station of our main-stem transect was situated at the upper Lena, and the second station marks the transition from the upper to the lower Lena. According to Kutscher et al. (2017), the upper and lower areas of the Lena account for the following fractions of the total watershed area and POC discharge: the upper Lena accounts for 37 % of the entire Lena River watershed and 2.9 Tg C yr−1 of TSM; Aldan, the watershed of the Lena River's main tributary, accounts for 29 % and 1.8 Tg C yr−1; the lower Lena accounts for 15 % and 1.1 Tg C yr−1; and Vilyuy, the second big tributary of the Lena, accounts for 19 % and 0.6 Tg C yr−1.
Surface river water concentrations of TSM and POC in 2019 display a decreasing trend from Yakutsk to Kyusyur downstream along the Lena River (Fig. 2a and b). The highest TSM and POC concentrations (34.5 and 1.32 mg L−1, respectively) were found at the location close to where the Aldan tributary flows into the Lena River (WL19-02); downstream from where the Vilyuy tributary feeds into the Lena (WL19-03), TSM and POC concentrations steadily decrease. Several factors may account for this observation.
OM concentrations in the lower Lena were lower compared to in the upper Lena. It has been suggested that this is related to the difference in the geological setting in the northern part of the catchment compared to that in the southern part (Kutscher et al., 2017) and to the mean slope of the upper Lena subcatchment, which is reflected by OM concentrations in the river (Mulholland, 1997). The upper Lena is sourced from regions with higher precipitation (Chevychelov et al., 2010), a smaller extent of continuous permafrost (Obu et al., 2019), and more productive forests (Stone and Schlesinger, 2003). In contrast, the lower Lena receives a considerable part of the water flowing from the steeply sloped mountainous areas of the Verkhoyansk Range. The water catchment area of the lower Lena is covered by shallow, OM-poor soils: gleyzems, which are Al–Fe humic soils, and shallow, weakly developed soils that develop in mountainous areas (Stolbovoi, 2002). OM from the upper Lena catchment may reach the lower Lena, as we measured at the beginning of the riverine transect. TSM and POC concentrations decreased in the lower Lena regime because local tributaries carry less OM, with possibly relatively more mineral compounds, which, for example, is reflected in the decrease in OCTSM from the south to the north of the main-stem transect (Fig. 2c).
The lower TSM and POC downstream from the Vilyuy could also result from the hydrological change, which takes place inside the lower Lena itself. Kääb et al. (2013) reported that the velocity of the Lena River decreases downstream from south to north along the 620 km long Lena River transect between 67.00 and 71.58∘ N (corresponding to the river stretch between our stations WL19-05 (Zhigansk) and WL19-09 (the nearest to the delta)), reaching the lowest values approximately 40 km south of Kyusyur. This is a result of the topography of the region, where the base slope elevation of the river flattens near 150 km south of Kyusyur. It is also known that the majority of TSM brought by the Lena River from the water catchment is deposited before the Lena reaches the stream north of Kyusyur, which is known as the Lenskaya truba (which means Lena pipe; Antonov, 1960). This is the narrowest (sometimes less than 2 km) and deepest (more than 20 m) part of the river (Fedorova et al., 2015) where the TSM sedimentation takes place. The preferential sedimentation of mineral particles may also affect the isotopic composition of transported OM. As was shown by Vonk et al. (2014), contemporary terrestrial OM is dispersed mainly by horizontal transport, while mineral-associated (i.e. heavier), older OC from topsoil and Yedoma is affected mainly by vertical transport and seems to settle rapidly. Thus, decreasing velocity in the lower Lena (from 2.5 to 0.8 m s−1 in May, Kääb et al., 2013) and in the Lena delta itself (from 1.3 to 0.9 m s−1 in August; Nigamatzyanova et al., 2015) allows for further sedimentation of TSM and old C, resulting in a decrease of its concentration.
The Lena delta was characterised by the lowest TSM and POC values in 2019, as previously described. Several factors may account for these findings. It is known that the sediment transport from the Lena delta to the Laptev Sea is controlled by the distribution of water and sediment discharge along the different deltaic branches (Rachold et al., 1996). According to Rachold et al. (1996), the Trofimovskaya–Sardakhskaya branch system receives 61 % of the annual water volume registered at Stolb station. These channels have complex structures and contain several different regional sub-delta systems. As a result, only 7 % to 8 % of the initial water volume reaches the mouth of the Trofimovskaya channel (Rachold et al., 1996). The water, loaded with suspended matter, is distributed into the numerous channels; this affects the amount of TSM detected in the big channels. Due to the extensive branching of the channels in the delta, velocity decreases, which allow for settling and sedimentation of TSM, occur mostly on flood plains (Sanders et al., 2022) following the high-water flooding season.
The OMTSM is much higher in the delta compared to in the Lena main stem, which may be a result of preferential settling of denser mineral-rich particles between the main stem and the delta. Further input of OM originates from the islands, supplied by bank erosion and degradation of ice-rich permafrost deposits such as Yedoma; both processes take place in the delta (Stettner et al., 2018; Fuchs et al., 2020; Haugk et al., 2022). Based on these observed patterns, we suggest that the Lena delta should be distinguished as the third hydrographically and sedimentologically distinct part of the Lena River system.
4.2 Potential sources of particulate organic carbon
4.2.1 Stable carbon isotope sources
The δ13C values of POC in the Lena main-stem surface water ranged between −28.8 ‰ and −26.8 ‰, with a mean of −27.9 ± 0.6 ‰, comparable to a typical δ13C of terrestrial C3 plants (about −27 ‰; Finlay and Kendall, 2008). C3 plants are dominant in the Lena catchment area and account for most of the soil OM (SOM; from −28.4 ‰ to −27.0 ‰), including that of Yedoma deposits (−27.5 ‰) that have been described for the Lena watershed (Vonk et al., 2017; Schirrmeister et al., 2011) and for the terrestrial primary production (−27.7 ‰) such as organic and litter layers (Wild et al., 2019). The observed δ13C values also overlap with the range of δ13C values determined for soils from the first terrace of Lena delta soils (from −27.0 ‰ to −25.1 ‰; Winterfeld et al., 2015).
In contrast to main-stem POC, deltaic POC was more depleted in 13C (mean −32.5 ± 0.7 ‰; Figs. 2e, 3a). Similarly low values were found in the delta in 2016 and 2017 and were previously reported in several publications for the Lena delta (e.g. Winterfeld et al., 2015), the Lena River (Kutscher et al., 2017; Fig. 3), and the Kolyma River (Bröder et al., 2020). Those authors related riverine POC depleted in 13C to the influence of aquatic primary production and suggested a phytoplankton contribution to explain the isotopic composition of POM. However, in these studies, reported POC δ13C values below −30 ‰ were the exception rather than the rule. This is in contrast to our set of deltaic samples from 2019, where POC is consistently strongly depleted in 13C, showing δ13C values between −33.3 ‰ and −31 ‰.
Unfortunately, neither of the cited publications reports the phytoplankton biomass and/or chlorophyll a data for the studied water samples nor were these measurements part of our sampling campaign. This lack of quantitative observational data requires a theoretical estimation of phytoplankton input into POC.
According to the data from Finlay and Kendal (2008), δ13C from phytoplankton in river POC ranges from −42 ‰ to −25 ‰. However, these authors stress that the range of isotopic values is smaller within each single river. Regardless, low δ13C of POC, as observed in our deltaic samples, is consistent with substantial contributions of POC from aquatic production during phytoplankton bloom.
In the Arctic, 2019 was a warm and dry year when mean annual surface air temperature over the Arctic land mass was the second highest in the observational period (1900–present; Richter-Menge et al., 2019). Our Lena delta sampling period was between 20 July and 10 August, which is the season when the highest water temperatures were observed by Liu et al. (2005). Higher water temperatures induce higher metabolic rates and thus increased aquatic primary production and heterotrophic activity (Paczkowska et al., 2019; Bosco-Galazzo et al., 2018; Allen et al., 2005). The average water temperature in the delta in 2019 according to the CTD measurements was 15.2 ∘C (Fuchs et al., 2022). Liu et al. (2005) reported a decrease of water temperatures from the upper Lena to the north. Average Lena water temperatures at Kyusyur (the northernmost station of that study) were 3–5 ∘C lower in mid-open-water season (end of June–end of August) compared to at the Aldan and upper Lena basins and barely reached 15 ∘C (Liu et al., 2005). Likewise, ArcticGRO reports a water temperature of 15.0 ∘C on 2 August 2019. Thus, the water temperatures in the Lena delta during our sampling campaign (7–8 August) were higher than in Zhigansk (∼ 800 km to the south of the delta) despite the latitudinal gradient in water temperatures observed by Liu et al. (2005). A heat wave in 2019 may have had further consequences in the Lena delta, such as the formation of isolated small deltaic channels, which were separated due to extremely low water conditions. These channels may represent favourable habitats for the development of high numbers of algae despite the absence of an additional nutrient flux, as temperature and velocity appear to exert a stronger positive influence on phytoplankton growth, outbalancing the lack of nutrients (Li et al., 2013). From this, it follows that the delta provided more favourable thermal conditions for phytoplankton than the main stem did; this was particularly true in the very warm year of 2019. We suggest that sampling during potential algal blooms took place in earlier years as well. For example, in 2017, we observed a δ13C decrease from −27.9 ‰ to −32.3 ‰ within only 2 weeks (11 July–25 July 2017), suggesting that an algal bloom developed between the two sampling dates (Fig. 3a).
Additional support for the increase of aquatic production in August 2019 is provided by gradients in nitrate (1.5 to 0.25 µmol L−1) and silicate (50 to 18 µmol L−1) observed by Sanders et al. (2022) on the way along the sampling transect from Stolb Island further into the delta (LEN19-S-01, LEN19-S-02, and LEN19-S-03). Sanders et al. (2022) suggest that phytoplankton such as diatoms may be responsible for this uptake, as described in Hawkings et al. (2017).
The C isotopic fractionation during photosynthesis is not constant and may depend on the environmental conditions, but isotopic composition of phytoplankton generally strongly correlates with the isotopic composition of dissolved inorganic C (DIC; Rounick and James, 1984). In the Lena delta, the δ13C of DIC has not been measured, but the low δ13C of POC suggests a 13C-depleted DIC pool. Low δ13C in DIC can be caused by several processes, as shown for riverine DIC in the rivers of Patagonia: degradation of dissolved OC (DOC) containing soil organic C will result in low δ13C of DIC, and δ13C of DIC was found to be negatively correlated with DOC concentration (Brunet et al., 2005). It is conceivable that DOC remineralisation leads to similarly reduced δ13C of DIC in the Lena delta, which is in agreement with observations of rapid remineralisation of DOC released from thawing permafrost, as reported for the Kolyma catchment (Mann et al., 2015) and for thaw creeks on an island in the Lena delta (Stolpmann et al., 2022).
The fact that the lowest δ13C values tend to be in samples with the lowest POC concentrations offers another interesting perspective (Fig. 3a). As discussed above, TSM content (and POC from terrestrial sources) appears to be related to flow velocity. It is known that velocity exerts a strong negative effect on chlorophyll a concentration (Li et al., 2013). Together with other hydrological properties such as increasing suspended matter and turbidity, velocity could be one of the critical forcing factors regulating phytoplankton biomass; a high velocity dilutes phytoplankton cells, reduces light availability, and changes the entire dynamic of aquatic production (Salmaso and Braioni, 2008). In turn, low flow velocities in the deltaic channels, as suggested by low POC values, could provide more favourable conditions for aquatic production while, at the same time, larger or denser mineral-bearing particles might be settling.
The combined factors of low flow velocity in the shallow delta channels, where sunlight penetrates much of the water column which contains only small amounts of suspended particles, and the extremely warm summer conditions during our sampling campaign might have resulted in high primary production, providing larger relative amounts of POC than in the main stem and during previous years.
δ13C from ArcticGRO was lower than the δ13C of POC we measured in the main stem, which may indicate more phytoplankton contribution to ArcticGRO samples. This may have resulted from a potentially higher C3 plant contribution to POC in the main stem in 2019 instead of contributions from phytoplankton, which were very likely to be higher for ArcticGRO samples due to the sampling time (9 of 12 δ13C ArcticGRO records were measured in samples taken in August and at the end of July) and/or less DOC recycling in June (main-stem sampling 2019) than at the end of July–August (ArcticGRO).
4.2.2 Δ14C in particulate organic matter
Δ14C of POC sampled in 2019 was homogeneous along the studied transect, both along the Lena River main stem and within the Lena delta (Fig. 2d). In contrast to this, Δ14C values reported by ArcticGRO were strongly depleted in 14C compared to our 2019 transect data, although the values found in this study for the Lena delta and nearby Stolb Island fit within the range of other previously published data from the Lena delta (Figs. 3b, 4). Values published by Winterfeld et al. (2015) for multiple summer seasons in the Lena delta vary between −145 ‰ and −194 ‰; Karlsson et al. (2016) reported values in the range of −433 ‰ to −97 ‰ (Fig. 4). This comparison reveals that the 2019 deltaic transect data are not atypical, as they are similar to the values for August 2009 and are very close to those for July–August 2010 and June–July 2011.
The Δ14C of POC from Stolb in 2016 and 2017 were relatively similar to ArcticGRO values or were sometimes lower (Figs. 3b, 4). It is important to highlight that Δ14C in POC may change strongly within a relatively short period. For example, sampling in the summers of 2016 and 2017 was done twice, with only 1 (2016) or 2 (2017) weeks in between. Δ14C in 2017 increased over the course of 2 weeks (11–25 July) by almost 170 ‰, causing values to jump from −288 ‰ to −122 ‰ (Fig. 3b). The data from 2016 also showed a distinct but less pronounced increase in Δ14C within 1 week (8–15 August) from −387 ‰ up to −306 ‰. Thus, Δ14C of POC is a parameter that exhibits large variability along the Lena main stem and inside its delta. This variability makes this parameter hard to interpret and suggests that local and short-term effects exert strong control (e.g. collapse of a bluff along the channel bank or rain events).
The difference between ArcticGRO and the 2019 data may also be explained by the rather small number of ArcticGRO observations used for this comparison. The mean Δ14C value of the entire Lena dataset from ArcticGRO is −275 ‰ and ranges from −398 ‰ to −164 ‰, which includes our Δ14C values observed in 2019. We did not consider the entire ArcticGRO dataset for Lena River samples, as we expect that, during the discharge peak following the ice breakup in spring, POC might be of distinctly different origin than during the summer. We thus selected only values obtained for samples collected in the summer season after 15 June until 31 August. As a result, the reduced ArcticGRO dataset consists of only four samples, with a mean Δ14C value of −309 ‰ (min max −336 ‰ −286 ‰) sampled in August of 2004 and 2005, similar to the time of the year when our deltaic campaign took place.
Table 1Possible sources of C used for the endmember modelling, along with their isotopic composition (after Winterfeld et al., 2015; Wild et al., 2019; Galimov et al., 2006).

Table 2Relative OM source contribution to water sample POC in 2019 (mean ± SD).

* For this model, samples from Stolb Island were included with the deltaic samples and contributed to the final mean ± SD values for potential POC sources.
Another explanation for the difference in Δ14C of POC between ArcticGRO and for our riverine transect may be the fact that ArcticGRO samples are depth integrated, since, potentially, river water masses may be stratified (e.g. Mackenzie: Hilton et al., 2015). We did not collect samples from different water depths along the river transect from Yakutsk to Stolb Island but instead were only able to sample surface waters and from discrete water depths for the samples from the Lena delta. The Lena River itself is deep along its main stem (up to 20 m downstream from Yakutsk); thus, pronounced and systematic differences between water mass properties like temperature and light penetration could prevail, in turn influencing the composition of POC. We speculate that surface water POM might be biased towards more phytoplankton contribution in the sunlit surface waters and/or towards the contribution of vascular plants floating in the surface waters of the stream, resulting in apparently younger POM than at depth. This might explain why our 2019 river samples are systematically younger than the ArcticGRO values.
Samples with lower POC concentrations tend to display higher Δ14C values and vice versa (Fig. 3b). We suggests that conditions with low suspended particle load promote phytoplankton growth, while highly turbid waters contain substantial amounts of POC derived from riverbank erosion or cliff failure.
4.2.3 Estimation of organic matter sources based on endmember modelling
Combined radiocarbon and stable C isotope compositions are used to investigate the origin of OM. The difference in OM origin for POC measured along the transects in 2019 was illustrated by stable C isotope variations. δ13C values in the delta suggest an aquatic origin of POC, and those samples also display comparatively high Δ14C values. On the other hand, Δ14C of POC in the delta and in the main stem are similar but differ in terms of δ13C values from the river samples, which are less depleted in 13C. If the delta samples contain substantial amounts of aquatically produced OM, isotope mass balance considerations require that some of the POC collected from delta waters still derive from terrestrial sources which are older than the sources supplying POC to the main stem. To examine this hypothesis, we applied an endmember model.
Riverine POM is regarded as originating, to variable degrees, from autochthonous production of phytoplankton and from allochthonous sources such as vegetation and soils (Ittekkot and Laane, 1991). Terrestrial allochthonous sources for the Lena River catchment may be divided into two pools with different ages and origins: Holocene permafrost soils and organic-rich Pleistocene deposits. To illustrate possible sources of OM, we used a dual-carbon-isotope (Δ14C, δ13C) three-endmember mixing model. Endmembers for the OM sources in the Lena main stem and its Delta were defined as phytoplankton (I), Holocene soils (II), and Pleistocene deposits (III), and the respective endmember isotope values were taken from previously published studies (Table 1). For Holocene soils, endmember values were taken from Winterfeld et al. (2015). This study focused on the Lena delta, and Holocene soil endmembers were combined from data measured directly for deltaic soils by this group of authors in 2009, 2010, and 2011 and from a literature review. δ13C values which we chose to use as endmember values were measured for Holocene soils which resemble soils in Lena River ecosystems, for example from the Yenisey watershed, and Δ14C values were measured mostly within the Lena delta in different years (Winterfeld et al., 2015).
Endmember values for ancient permafrost Yedoma deposits have also been measured. We use endmember values from Wild et al. (2019). In this study, Δ14C and δ13C values of Pleistocene deposits were constrained using observations from Yedoma deposits from multiple researchers, which were combined together for, in total, 329 observations for Δ14C and 374 for δ13C.
The determination of phytoplankton endmembers required further consideration. The δ13C endmember values for phytoplankton assumed in previous studies (e.g. Mann et al., 2015; Vonk et al., 2010; Wild et al., 2019; Winterfeld et al., 2015) are higher than the range of δ13C measured by us in the 2019 samples. The δ13C values reported by Galimov et al. (2006) for phytoplankton, however, are in the same range as ours from the Lena delta and Stolb Island (−32.4 ‰). Those authors determined δ13C values of phytoplankton in the Yenisey estuary ranging from −27.8 ‰ to −37.0 ‰, where phytoplankton is dominated by species of the phylum Bacillariophyta, which is the dominant phylum in the Lena delta as well (42.3 % of all the species; Gabyshev et al., 2019). Therefore, we use the endmember value of −33.3 ± 2.3 ‰ for δ13C of phytoplankton.
We determined the Δ14C endmember value for phytoplankton based on the assumption that recent terrestrial and aquatic vegetation contains mostly modern C from the atmosphere, potentially even carrying elevated levels of 14C affected by nuclear-weapons testing during the 1960s and 1970s; thus, values from organic litter from Russia, Scandinavia, and Alaska were included (Wild et al., 2019).
Proposing phytoplankton as one of three main sources of POC does not exclude the input of contemporary vegetation into the riverine and deltaic OM. Δ14C signals from both modern vegetation and phytoplankton sources may, in many cases, be identical and similar to the atmosphere (Winterfeld et al., 2015; Wild et al. 2019), while their δ13C values are likely to be different. Moreover, modern plant material may display variable Δ14C values between leaves, stems, and roots. Therefore, phytoplankton was proposed as a modern OM source based on evidence from δ13C values of POC, corresponding to algal input (see Sect. 4.2.1) and suggesting sufficiently lower input of plant debits into POC. Modern plants likely contributed as well, but due to their rather constant δ13C signature paired with variable Δ14C values (plant debris vs. roots and litter), they cannot be distinguished from Holocene soils and must be regarded as a contributor to this endmember.
Isotope mass balance endmember modelling was based on the following equations:
where fphytoplankton, fholocene soils, and fpleistocene deposit are the fractions contributed to the samples by phytoplankton, Holocene soils, and Pleistocene deposits, respectively. We applied the random-sampling computer simulation (Monte Carlo simulation), which is based on the assumption that the endmember values are represented by a normal distribution, where the mean and the standard deviation were taken from previously published studies (Galimov et al., 2006; Wild et al., 2019; Winterfeld et al., 2015). Calculations were conducted using random sampling from this distribution while simultaneously applying the random sample to mass balance equations (Eqs. 2, 3, 4) with repeated random sampling (5 000 000 times). Modelling was carried out in RStudio using the code published by Andersson (2011) in a modified version (Grotheer et al., 2020).
Based on this endmember model, the phytoplankton contribution is highest in deltaic samples, with a mean of 68 ± 6 % (Tables 2, S1 and Figs. 4, 5), whereas main-stem POC consists of approximately 39 ± 8 % aquatically produced material. In contrast, Holocene soils account for 13 ± 10 % in the delta versus 56 ± 12 % in riverine POC. Moreover, dual-carbon-isotope (Δ14C, δ13C) three-endmember mixing model results are consistent with input from an additional old permafrost source into deltaic POC. Thus, the Pleistocene contribution to the Lena delta POC was estimated as 18 ± 4 %, which is almost 4 times higher than the main-stem POC, which held less than 5 % of ancient permafrost C in 2019 (Tables 2, S1 and Fig. 5). This demonstrates an additional source of permafrost-derived C, particularly in deltaic waters.
Our estimation of permafrost input into POC in the Lena main stem differs from that of Wild et al. (2019), who estimated pre-aged material input to the Lena River in summer to be 62 ± 0.5 %. Such a large variation is explained by the different approaches applied. Wild et al. (2019) divided endmember sources into (1) recent terrestrial primary production (vegetation, phytoplankton),and (2) pre-aged OM, which included all terrestrial OM, specifically the active layer, Holocene peat, and thermokarst Pleistocene deposits. Phytoplankton was not included as a potential source, but it is likely included in the recent primary-production category. In our study, we did not consider Holocene soils to be ancient C but emphasised Pleistocene deposits (Yedoma) as the contribution of ancient C in riverine and deltaic POC. The combined contribution to main-stem POC from both Holocene soils and Pleistocene deposits was estimated by us to be ∼ 61 %, which is similar to the contribution from a pre-aged OM source measured by Wild et al. (2019).
The estimated contributions of different OM sources to the actual average POC concentration measured for the Lena main stem and delta (Fig. 5) showed that POC derived from Holocene soils decreased from 0.44 to 0.05 mg L−1 due to sedimentation, which takes place in the lower Lena, particularly downstream from Kyusyur station, and in the delta itself, as explained in Sect. 4.1.2. POC derived from Pleistocene deposits almost doubles from 0.04 ± 0.02 (main stem) to 0.07 ± 0.02 mg L−1 (Lena delta). This is the case despite the lower concentration of POC in the delta than in the main stem and the lower discharge during the sampling time in the delta. This suggests that the Lena delta receives POC from an additional ancient permafrost deposit source, which is specific to the Lena delta. These observations support a finding from Karlsson et al. (2016), who estimated the contribution of carbon derived from Pleistocene deposits to surface coastal surface sediments for the East Siberian Arctic Shelf to be ∼ 53 %, with the surface soil contribution estimated to be only ∼ 0.23 %. Thus, the type of material that reaches the sea floor in the coastal zone reflects Yedoma deltaic origin.
We found a significant difference between the two investigated parts of the Lena River system, the main stem and the delta. TSM and POC in the main stem were significantly higher than in the delta. At the same time, TSM and POC concentrations along the main stem remained within the range of values registered by ArcticGRO for the years 2009–2019, while deltaic values of TSM and POC hardly reached the lowest values measured for Zhigansk. Conversely, the OMTSM is higher in the delta than in the river, likely resulting from a different composition of the suspended matter.
The distribution pattern of TSM along the main stem decreases downstream to Stolb Island, which is the apex of the Lena delta. This suggests that the major enrichment in TSM (mass wise) of the Lena main stem takes place along the main channel in the upper Lena catchment. This TSM is rich in mineral compounds, which tend to settle on the way to the ocean, where river velocity decreases. Settling is even more pronounced in the delta, where flow velocity is lower, because in the delta the main stem divides into multiple branches.
Modern OM, possibly from phytoplankton primary production, dominates in the delta combined with a higher input of ancient permafrost, while riverine OM is predominantly derived from soil OM. The actual concentration of ancient-permafrost-derived POC in the delta exceeds the concentration in the main stem (∼ 0.07 ± 0.02 and 0.04 ± 0.02 mg L−1, respectively) despite the lower concentration of POC (0.79 ± 0.30 mg L−1 in the river main stem and 0.41 ± 0.10 mg L−1 in the delta).
Our findings suggest that, if an estimation of Lena OM discharge to the coastal zone is based only on the data from the Lena main stem, it may overestimate the load of TSM and underestimate its sedimentation, which takes place in the lower Lena and its delta. In order to predict the effects on coastal waters of changes in permafrost due to climate change, additional and concurrent sampling from the river delta and the river main stem is needed. Particles that are mobilised from thawing permafrost in the Lena catchment may be deposited and decomposed on the way to the ocean. Therefore, investigating permafrost fingerprints only in samples from the main stem may lead to incorrect conclusions and a biased perspective of permafrost carbon release to the coastal zone and the Arctic Ocean. The Lena delta provides an additional source of permafrost carbon; Yedoma-derived OM, as part of the total permafrost carbon, could then be discharged into the Arctic coastal waters. The Lena delta, as the interface between the Lena River and the Arctic Ocean, plays a crucial role in determining the qualitative and quantitative composition of OM discharged into the Arctic Ocean.
The data presented in this study are freely available in the PANGAEA data repository: https://doi.org/10.1594/PANGAEA.950668 (Ogneva et al., 2022).
The supplement related to this article is available online at: https://doi.org/10.5194/bg-20-1423-2023-supplement.
OO, GM, and JS designed the concept of the study. OO drafted a first version of the paper and carried out laboratory analyses of TSM and POC, including radio C analyses together with HG. BJ designed the maps. HG provided R code for endmember analysis. TS, MF, JP, JS, and OO carried out the field work and collected the samples. All the co-authors contributed to the writing and editing processes.
The contact author has declared that none of the authors has any competing interests.
Publisher's note: Copernicus Publications remains neutral with regard to jurisdictional claims in published maps and institutional affiliations.
We are grateful to everyone who helped with and supported the CACOON project and the joint Russian-German Lena 2019 expeditions, particularly Volkmar Aßmann (AWI) for the logistics during the summer expedition and the Samoylov Research Station for the hospitality. We would particularly like to thank Waldemar Schneider (AWI) for the invaluable help with the sampling along the Lena River main stem.
This research is part of the “Changing Arctic Carbon cycle in the COastal
Ocean Near-shore (CACOON)” project and has been supported by the Bundesministerium für Bildung und Forschung (grant no. 03F0806A) and the Natural Environment Research Council (grant no. NE/R012806/1). Bennet Juhls was funded by the European
Space Agency (ESA) as part of the Climate Change Initiative (CCI) fellowship
(ESA ESRIN/Contract no. 4000l3376l/2l/I-NB).
The article processing charges for this open-access publication were covered by the Alfred Wegener Institute, Helmholtz Centre for Polar and Marine Research (AWI).
This paper was edited by Ny Riavo G. Voarintsoa and reviewed by three anonymous referees.
Allen, A. P., Gillooly, J. F., and Brown, J. H.: Linking the global carbon cycle to individual metabolism, Funct. Ecol., 19, 202–213, https://doi.org/10.1111/j.1365-2435.2005.00952.x, 2005.
Amon, R. M. W., Rinehart, A. J., Duan, S., Louchouarn, P., Prokushkin, A., Guggenberger, G., Bauch, D., Stedmon, C., Raymond, P. A., Holmes R. M., McClelland, J. W., Peterson, B. J., Walker S. A., and Zhulidov, A. V.: Dissolved organic matter sources in large Arctic rivers, Geochim. Cosmochim. Ac., 94, 217–237, https://doi.org/10.1016/j.gca.2012.07.015, 2012.
Andersson, A.: A systematic examination of a random sampling strategy for source apportionment calculations, Sci. Total Environ., 412–413, 232–238, https://doi.org/10.1016/j.scitotenv.2011.10.031, 2011.
Antonov, V. S.: The Lena River Delta – Works of oceanographic committee of the Acad, Sci. USSR, Vol. VI, 25–34, 1960 (in Russian).
Ballinger, T. J., Overland, J. E., Wang, M., Bhatt, U. S., Hanna, E., Hanssen-Bauer, I., Kim, S.-J., Thoman, R. L., and Walsh, J. E.: Arctic Report Card 2020: Surface Air Temperature, in: NOAA Arctic Report Card 2020, 21–27, edited by: Thomas, R. L., Richter-Menge, J., and Drukenmiller, M. L., 141 pp., https://doi.org/10.25923/gcw8-2z06, 2020.
Biskaborn, B. K., Smith, S. L., Noetzli, J., Matthes, H., Vieira, G., Streletskiy, D. A., Schoeneich, P., Romanovsky, V. E., Lewkow, A. G., Abramov, A., Allard, M., Boike, J., Cable, W. L., Christiansen, H. H., Delaloye, R., Diekmann, B., Drozdov, D., Etzelmüller, B., Grosse, G., Guglielmin, M., Thomas Ingeman-Nielsen, T., Ketil Isaksen, K., Ishikawa, M., Johansson, M., Johannsson, H., Joo, A., Kaverin, D., Kholodov, A., Konstantinov, P., Kröger, T., Lambiel, C., Lanckman, J.-P., Luo, D., Malkova, G., Meiklejohn, I., Moskalenko, N., Oliva, M., Phillips, M., Ramos, M., Sannel, A. B. K., Sergeev, D., Seybold, C., Skryabin, P., Vasiliev, A., Wu, Q.,Yoshikawa, K., Zheleznyak, M., and Lantuit, H.: Permafrost is warming at a global scale, Nat. Commun., 10, 264, https://doi.org/10.1038/s41467-018-08240-4, 2019.
Boscolo-Galazzo, F., Crichton, K. A., Barker, S., and Pearson, P. N.: Temperature dependency of metabolic rates in the upper ocean: A positive feedback to global climate change?, Glob. Planet. Change, 170, 201–212, https://doi.org/10.1016/j.gloplacha.2018.08.017, 2018.
Bröder, L., Tesi, T., Andersson, A., Semiletov, I., and Gustafsson, Ö.: Bounding cross-shelf transport time and degradation in Siberian-Arctic land-ocean carbon transfer, Nat. Commun., 9, 806, https://doi.org/10.1038/s41467-018-03192-1, 2018.
Bröder, L., Davydova, A., Davydov, S., Zimov, N., and Haghipour, N.: Particulate Organic Matter Dynamics in a Permafrost Headwater Stream and the Kolyma River Mainstem, J. Geophys. Res.-Biogeo., 125, 1–16, https://doi.org/10.1029/2019JG005511, 2020.
Brown, N. J., Nilsson, J., and Pemberton, P.: Arctic Ocean freshwater dynamics: Transient response to increasing river runoff and precipitation, J. Geophys. Res.-Ocean., 124, 5205–5219, https://doi.org/10.1029/2018JC014923, 2019.
Brunet, F., Gaiero, D., Probst, J. L., Depetris, P. J., Lafaye, F. G., and Stille, P.: δ13C tracing of dissolved inorganic carbon sources in patagonian rivers (Argentina), Hydrol. Process., 19, 3321–3344, https://doi.org/10.1002/hyp.5973, 2005.
Carmack, E. C., Yamamoto-Kawai, M., Haine, T. W. N., Bacon, S., Bluhm, B. A., Lique, C., Melling, H., Polyakov, I. V., Straneo, F., Timmermans, M.-L., and Williams, W. J.: Freshwater and its role in the Arctic Marine System: Sources, disposition, storage, export, and physical and biogeochemical consequences in the Arctic and global oceans, J. Geophys. Res.-Biogeos., 121, 675–717, https://doi.org/10.1002/2015JG003140, 2016.
Charkin, A. N., Dudarev, O. V., Semiletov, I. P., Kruhmalev, A. V., Vonk, J. E., Sánchez-García, L., Karlsson, E., and Gustafsson, Ö.: Seasonal and interannual variability of sedimentation and organic matter distribution in the Buor-Khaya Gulf: the primary recipient of input from Lena River and coastal erosion in the southeast Laptev Sea, Biogeosciences, 8, 2581–2594, https://doi.org/10.5194/bg-8-2581-2011, 2011.
Chevychelov, A. P. and Bosikov, N. P.: Natural conditions, in: The Far North. Plant and Vegetation, edited by: Troeva, E. I., Isaev, A. P., Cherosov, M. M., and Karpov, N. S., Springer, the Netherlands, 1–23, https://doi.org/10.1007/978-90-481-3774-9_1, 2010.
Fedorova, I., Chetverova, A., Bolshiyanov, D., Makarov, A., Boike, J., Heim, B., Morgenstern, A., Overduin, P. P., Wegner, C., Kashina, V., Eulenburg, A., Dobrotina, E., and Sidorina, I.: Lena Delta hydrology and geochemistry: long-term hydrological data and recent field observations, Biogeosciences, 12, 345–363, https://doi.org/10.5194/bg-12-345-2015, 2015.
Feng, D., Gleason, C. J., Lin, P., Yang, X., Pan, M., and Ishitsuka, Y.: Recent changes to Arctic river discharge, Nat. Commun., 12, 1–9, https://doi.org/10.1038/s41467-021-27228-1, 2021.
Finlay, J. C. and Kendall, C.: Stable Isotope Tracing of Temporal and Spatial Variability in Organic Matter Sources to Freshwater Ecosystems, in: Stable Isotopes in Ecology and Environmental Science, edited by: Michener, R. and Lajtha, K., Blackwell Publishing Ltd., 283–333, https://doi.org/10.1002/9780470691854.ch10, 2008.
Fox-Kemper, B., Hewitt, H. T., Xiao, C., Aðalgeirsdóttir, G., Drijfhout, S. S., Edwards, T. L., Golledge, N. R., Hemer, M., Kopp, R. E., Krinner, G., Mix, A., Notz, D., Nowicki, S., Nurhati, I. S., Ruiz, L., Sallée, J.-B., Slangen, A. B. A., and Yu, Y.: Ocean, Cryosphere and Sea Level Change, in: Climate Change 2021: The Physical Science Basis, Contribution of Working Group I to the Sixth Assessment Report of the Intergovernmental Panel on Climate Change, edited by: Masson-Delmotte, V., Zhai, P., Pirani, A., Connors, S. L., Péan, C., Berger, S., Caud, N., Chen, Y., Goldfarb, L., Gomis, M. I., Huang, M., Leitzell K., Lonnoy E., Matthews, J. B. R., Maycock, T. K., Waterfield, T., Yelekçi, O., Yu, R., and Zhou, B., Cambridge University Press, Cambridge, United Kingdom and New York, NY, USA, 1211–1362, https://www.ipcc.ch/report/ar6/wg1/downloads/report/IPCC_AR6_WGI_Chapter09.pdf (last access: 31 August 2022), 2021.
Fuchs, M., Nitze, I., Strauss, J., Günther, F., Wetterich, S., Kizyakov, A., Fritz, M., Opel, T., Grigoriev, M. N., Maksimov, G. T., and Grosse, G.: Rapid Fluvio-Thermal Erosion of a Yedoma Permafrost Cliff in the Lena River Delta, Front. Earth Sci., 8, 336, https://doi.org/10.3389/feart.2020.00336, 2020.
Fuchs, M., Ogneva, O., Sanders, T., Schneider, W., Polyakov, V., Becker, O. O., Bolshiyanov, D., Mollenhauer, G., and Strauss, J.: CACOON Sea – water sampling along the Sardakhskaya channel and near shore of the Laptev Sea, in: Reports on Polar and Marine Research, Russian-German Cooperation: Expeditions to Siberia in 2019, edited by: Fuchs, M., Bolshiyanov, D., Grigoriev, M. N., Morgenstern, A., and Dill, A., Bremerhaven, Alfred Wegener Institute, chap. 3.26, 141–149, ISBN: 1866-3192, https://doi.org/10.48433/BzPM_0749_2021, 2021.
Fuchs, M., Palmtag, J., Juhls, B., Overduin, P. P., Grosse, G., Abdelwahab, A., Bedington, M., Sanders, T., Ogneva, O., Fedorova, I. V., Zimov, N. S., Mann, P. J., and Strauss, J.: High-resolution bathymetry models for the Lena Delta and Kolyma Gulf coastal zones, Earth Syst. Sci. Data, 14, 2279–2301, https://doi.org/10.5194/essd-14-2279-2022, 2022.
Gabyshev, V. A., Tsarenko, P. M., and Ivanova, A. P.: Diversity and Features of the Spatial Structure of Algal Communities of Water Bodies and Watercourses in the Lena River Estuary, Inland Water Biol., 12, 1–9, https://doi.org/10.1134/S1995082919050067, 2019.
Galimov, E. M., Kodina, L. A., Stepanets, O. V., and Korobenik, G. S. Biogeochemistry of the Russian Arctic. Kara Sea: Research results under the SIRRO project, 1995–2003, Geochem. Int., 44, 1053–1104, https://doi.org/10.1134/S0016702906110012, 2006.
Gordeev, V. V.: Fluvial sediment flux to the Arctic Ocean, Geomorphology, 80, 94–104, https://doi.org/10.1016/j.geomorph.2005.09.008, 2006.
Grotheer, H., Meyer, V., Riedel, T., Pfalz, G., Mathieu, L., Hefter, J., Gentz, T., Mollenhauer, G., and Fritz, M.: Burial and origin of permafrost-derived carbon in the nearshore zone of the southern Canadian Beaufort Sea, Geophys. Res. Lett., 47, e2019GL085897, https://doi.org/10.1029/2019GL085897, 2020.
Haugk, C., Jongejans, L. L., Mangelsdorf, K., Fuchs, M., Ogneva, O., Palmtag, J., Mollenhauer, G., Mann, P. J., Overduin, P. P., Grosse, G., Sanders, T., Tuerena, R. E., Schirrmeister, L., Wetterich, S., Kizyakov, A., Karger, C., and Strauss, J.: Organic matter characteristics of a rapidly eroding permafrost cliff in NE Siberia (Lena Delta, Laptev Sea region), Biogeosciences, 19, 2079–2094, https://doi.org/10.5194/bg-19-2079-2022, 2022.
Hawkings, J., Wadham, J., Benning, L. Hendry, K. R., Tranter, M., Tedstone, A., Nienow, P., and Raiswell, R.: Ice sheets as a missing source of silica to the polar oceans, Nat. Commun., 8, 14198, https://doi.org/10.1038/ncomms14198, 2017.
Hilton, R., Galy, V., Gaillardet, J., Dellinger, M., Bryant, C., O'Regan, M., Gröcke, D. R., Coxall, H., Bouchez, J., and Calmels, D.: Erosion of organic carbon in the Arctic as a geological carbon dioxide sink, Nature, 524, 84–87, https://doi.org/10.1038/nature14653, 2015.
Holmes, R. M., Shiklomanov, A. I., Suslova, A., Tretiakov, M., McClelland, J. W., Spencer, R. G. M., and Tank., S. E.: River Discharge, in: State of the Climate in 2018, Bull. Am. Meteorol. Soc., 100, 161–163, https://https://doi.org/10.1175/2019BAMSStateoftheClimate.1, 2019.
Holmes, R. M., McClelland, J. W., Tank, S. E., Spencer, R. G. M., and Shiklomanov, A. I.: Arctic Great Rivers Observatory, Water Quality Dataset, Version 20210319, https://www.arcticgreatrivers.org/data (last access: 1 June 2022), 2021.
Hugelius, G., Strauss, J., Zubrzycki, S., Harden, J. W., Schuur, E. A. G., Ping, C.-L., Schirrmeister, L., Grosse, G., Michaelson, G. J., Koven, C. D., O'Donnell, J. A., Elberling, B., Mishra, U., Camill, P., Yu, Z., Palmtag, J., and Kuhry, P.: Estimated stocks of circumpolar permafrost carbon with quantified uncertainty ranges and identified data gaps, Biogeosciences, 11, 6573–6593, https://doi.org/10.5194/bg-11-6573-2014, 2014.
Ittekkot, V. and Laane, R. W. P. M.: Fate of riverine particulate organic matter, in: Biogeochemistry of Major World Rivers, edited by: Degens, E. T., Kempe, S., and Richey, J. E., Wiley, New York, 233–242, https://doi.org/10.1002/aqc.3270010209, 1991.
Ivanov, V. V. and Piskun, A. A.: Distribution of River Water and Suspended Sediment Loads in the Deltas of Rivers in the Basins of The Laptev and East-Siberian Seas, in: Land-Ocean Systems in the Siberian Arctic: Dynamics and History, edited by: Kassens, H., Bauch, H. A., Dmitrenko, I., Eicken, H., Hubberten, H.-W., Melles, Ž. M., Thiede, J., and Timokhov, L., Springer-Verlag, New York, 239–250, https://doi.org/10.1007/978-3-642-60134-7_22, 1999.
Jong, D., Bröder, L., Tanski, G., Fritz, M., Lantuit, H., Tesi, T., Haghipour, N., Eglinton, T. I., and Vonk, J. E.: Nearshore Zone Dynamics Determine Pathway of Organic Carbon From Eroding Permafrost Coasts, Geophys. Res. Lett., 47, e2020GL088561, https://doi.org/10.1029/2020GL088561, 2020.
Juhls, B., Stedmon, C. A., Morgenstern, A., Meyer, H., Hölemann, J., Heim, B., Povazhnyi, V., and Overduin P. P.: Identifying Drivers of Seasonality in Lena River Biogeochemistry and Dissolved Organic Matter Fluxes, Front. Environ. Sci., 8, 53, https://https://doi.org/10.3389/fenvs.2020.00053, 2020.
Kääb, A., Lamare, M., and Abrams, M.: River ice flux and water velocities along a 600 km-long reach of Lena River, Siberia, from satellite stereo, Hydrol. Earth Syst. Sci., 17, 4671–4683, https://doi.org/10.5194/hess-17-4671-2013, 2013.
Karlsson, E., Gelting, J., Tesi, T., van Dongen, B., Andersson, A., Semiletov, I., Charkin, A., Dudarev, O., and Gustafsson, Ö.: Different sources and degradation state of dissolved, particulate, and sedimentary organic matter along the Eurasian Arctic coastal margin, Global Biogeochem. Cy., 30, 898–919, https://https://doi.org/10.1002/2015GB005307, 2016.
Köchy, M., Hiederer, R., and Freibauer, A.: Global distribution of soil organic carbon – Part 1: Masses and frequency distributions of SOC stocks for the tropics, permafrost regions, wetlands, and the world, SOIL, 1, 351–365, https://doi.org/10.5194/soil-1-351-2015, 2015.
Krumpen, T., Belter, J., Boetius, A., Damm, E., Haas, C., Hendricks, S., Nicolaus, M., Nöthig, E. M., Paul, S., Peeken, I., Ricker, R., and Stein, R.: Arctic warming interrupts the Transpolar Drift and affects long range transport of sea ice and ice-rafted matter, Sci. Rep., 9, 5459, https://doi.org/10.1038/s41598-019-41456-y, 2019.
Kutscher, L., Mörth, C.-M., Porcelli, D., Hirst, C., Maximov, T. C., Petrov, R. E., and Andersson, P. S.: Spatial variation in concentration and sources of organic carbon in the Lena River, Siberia, J. Geophys. Res.-Biogeo., 122, 1999–2016, https://https://doi.org/10.1002/2017JG003858, 2017.
Lamontagne-Hallé, P., McKenzie, J. M., Kurylyk, B. L., and Zipper, S. C.: Changing groundwater discharge dynamics in permafrost regions, Environ. Res. Lett., 13, 084017, https://doi.org/10.1088/1748-9326/aad404, 2018.
Lehner, B. and Grill, G.: Global river hydrography and network routing: baseline data and new approaches to study the world's large river systems, Hydrol. Process., 27, 2171–2186, https://doi.org/10.1002/hyp.9740, 2013.
Li, F., Zhang, H., Zhu, Y., Xiao, Y., and Chen, L.: Effect of flow velocity on phytoplankton biomass and composition in a freshwater lake, Sci. Total Environ., 447, 64–71, https://doi.org/10.1016/j.scitotenv.2012.12.066, 2013.
Lique, C., Holland, M. M., Dibike, Y. B., Lawrence, D. M., and Screen, J. A.: Modeling the Arctic freshwater system and its integration in the global system: Lessons learned and future challenges, J. Geophys. Res.-Biogeo., 121, 540–566, https://doi.org/10.1002/2015JG003120, 2016.
Lisitzin, A. P.: A mariginal Filter of the Ocean, Okeanologiya, 34, 735–747, 1994 (in Russian).
Liu, B., Yang, D., Ye, B., and Berezovskaya, S.: Long-term open-water season stream temperature variations and changes over Lena River Basin in Siberia, Glob. Planet. Change, 48, 96–111, https://doi.org/10.1016/j.gloplacha.2004.12.007, 2005.
Magritsky, D., Alexeevsky, N., Aybulatov, D., Fofonova, V., and Gorelkin, A.: Features and Evaluations of Spatial and Temporal Changes of Water Runoff, Sediment Yield and Heat Flux in the Lena River Delta, Polarforschung, 87, 89–110, https://doi.org/10.2312/polarforschung.87.2.89, 2018.
Mann, P. J., Eglinton, T. I., McIntyre, C. P., Zimov, N., Davydova, A., Vonk, J. E., Holmes, R. M., and Spencer, R. G. M.: Utilization of old permafrost carbon in headwaters of Arctic fluvial networks, Nat. Commun., 6, 7856, https://https://doi.org/10.1038/ncomms8856, 2015.
Mann, P. J., Strauss, J., Palmtag, J., Dowdy, K., Ogneva, O., Fuchs, M., Bedington, M., Torres, R., Polimene, L., Overduin, P., Mollenhauer, G., Grosse, G., Rachold, V., Sobczak, W. V., Spencer, R. G. M., and Juhls, B.: Degrading permafrost river catchments and their impact on Arctic Ocean nearshore processes, Ambio, 51, 439–455, https://doi.org/10.1007/s13280-021-01666-z, 2022.
McClelland, J. W., Holmes, R. M., Peterson, B. J., Raymond, P. A., Striegl, R. G., Zhulidov, A. V., Zimov, S. A., Zimov N., Tank, S. E., Spencer, R. G. M., Staples, R., Gurtovaya, T. Y., and Griffin, C. G.: Particulate organic carbon and nitrogen export from major Arctic rivers, Global Biogeochem. Cy., 30, 629–643, https://https://doi.org/10.1002/2015GB005351, 2016.
Mollenhauer, G., Grotheer, H., Gentz, T., Bonk, E., and Hefter, J.: Standard operation procedures and performance of the MICADAS radiocarbon laboratory at Alfred Wegener Institute (AWI), Germany, Nucl. Instrum. Meth. B, 496, 45–51, https://doi.org/10.1016/j.nimb.2021.03.016, 2021.
Mulholland, P. J.: Dissolved organic matter concentration and flux in streams, J. North Am. Benthol. Soc., 16, 131–141, https://doi.org/10.2307/1468246, 1997.
Nigamatzyanova, G. R., Frolova, L. A., Chetverova, A. A., and Fedorova, I. V.: Hydrobiological investigation of channels in the mouth region of the Lena River, Uchenye Zapiski Kazanskogo Universiteta, Seriya Estestvennye Nauki, 157, 96–108, 2015, (in Russian).
Obu, J., Westermann, S., Bartsch, A., Berdnikov, N, Christiansen, H. H., Dashtseren, A., Delaloye, R., Elberling, B., Etzelmüller, B., Kholodov, A., Khomutov, A., Kääb, A., Leibman, M.O., Lewkowicz, A.G., Panda, S.K., Romanovsky, V., Way, R.G., Westergaard-Nielsen, A., Wu, T., Yamkhin, J., and Zou, D.: Northern Hemisphere permafrost map based on TTOP modelling for 2000–2016 at 1 km2 scale, Earth-Sci. Rev., 193, 299–316, https://doi.org/10.1016/j.earscirev.2019.04.023, 2019.
Ogneva, O., Mollenhauer, G., Juhls, B., Sanders, T., Palmtag, J., Fuchs, M., Grotheer, H., Mann, P. J., and Strauss, J.: Total suspended matter, particulate organic carbon and its isotopic composition in the Lena River and its Delta, PANGAEA [data set], https://doi.org/10.1594/PANGAEA.950668, 2022.
Oliva, M. and Fritz, M.: Permafrost degradation on a warmer Earth: Challenges and perspectives, Curr. Opin. Environ. Sci. Heal., 5, 14–18, https://doi.org/10.1016/j.coesh.2018.03.007, 2018.
Overland, J. E., Wang, M., Walsh, J. E., and Stroeve, J. C.: Future Arctic climate changes: Adaptation and mitigation time scales, Earth's Future, 2, 68–74, https://doi.org/10.1002/2013ef000162, 2014.
Paczkowska, J., Rowe, O. F., Figueroa, D., and Andersson, A.: Drivers of phytoplankton production and community structure in nutrient-poor estuaries receiving terrestrial organic inflow, Mar. Environ. Res., 151, 104778, https://doi.org/10.1016/j.marenvres.2019.104778, 2019.
Pohl, E., Grenier, C., Vrac, M., and Kageyama, M.: Emerging climate signals in the Lena River catchment: a non-parametric statistical approach, Hydrol. Earth Syst. Sci., 24, 2817–2839, https://doi.org/10.5194/hess-24-2817-2020, 2020.
Rachold, V., Alabyan, A., Hubberten, H.-W., Korotaev, V. N., and Zaitsev, A. A.: Sediment transport to the Laptev Sea–hydrology and geochemistry of the Lena River, Polar Res., 15, 183–196, https://doi.org/10.1111/j.1751-8369.1996.tb00468.x, 1996.
Rantanen, M., Karpechko, A. Y., Lipponen, A., Nordling, K., Hyvärinen, O., Ruosteenoja, K., Vihma, T., and Laaksonen, A.: The Arctic has warmed nearly four times faster than the globe since 1979, Commun. Earth Environ., 3, 168, https://doi.org/10.1038/s43247-022-00498-3, 2022.
Richter-Menge, J., Druckenmiller, M. L., and Jeffries, M. (Eds.): Arctic Report Card 2019, https://www.arctic.noaa.gov/Report-Card (last access: 1 June 2022), 2019.
Rounick, J. S. and James, M. R.: Geothermal and cold springs faunas: Inorganic carbon sources affect isotope values, Limnol. Oceanogr., 29, 386–389, https://doi.org/10.4319/lo.1984.29.2.0386, 1984.
Salmaso, N. and Braioni, M. G.: Factors controlling the seasonal development and distribution of the phytoplankton community in the lowland course of a large river in Northern Italy (River Adige), Aquat. Ecol., 42, 533–545, https://doi.org/10.1007/s10452-007-9135-x, 2008.
Sanders, T., Fiencke, C., Fuchs, M., Haugk, C., Juhls, B., Mollenhauer, G., Ogneva, O., Overduin, P., Palmtag, J., Povazhniy, V., and Strauss, J.: Seasonal nitrogen fluxes of the Lena River Delta, Ambio, 51, 423–438, https://doi.org/10.1007/s13280-021-01665-0, 2022.
Schirrmeister, L., Grosse, G., Wetterich, S., Overduin, P. P., Strauss, J., Schuur, E. A. G., and Hubberten, H.-W.: Fossil organic matter characteristics in permafrost deposits of the northeast Siberian Arctic, J. Geophys. Res.-Biogeo., 116, G00M02, https://https://doi.org/10.1029/2011jg001647, 2011.
Semiletov, I. P., Pipko, I. I., Shakhova, N. E., Dudarev, O. V., Pugach, S. P., Charkin, A. N., McRoy, C. P., Kosmach, D., and Gustafsson, Ö.: Carbon transport by the Lena River from its headwaters to the Arctic Ocean, with emphasis on fluvial input of terrestrial particulate organic carbon vs. carbon transport by coastal erosion, Biogeosciences, 8, 2407–2426, https://doi.org/10.5194/bg-8-2407-2011, 2011.
Shiklomanov, A., Déry, S., Tretiakov, M., Yang, D., Magritsky, D., Georgiadi A., and Tang W.: River Freshwater Flux to the Arctic Ocean, in: Arctic Hydrology, Permafrost and Ecosystems, edited by: Yang, D., and Kane, D. L., Springer, Cham, 703–738, https://doi.org/10.1007/978-3-030-50930-9_24, 2021a.
Shiklomanov, A. I., Holmes, R. M., McClelland, J. W., Tank, S. E., and Spencer, R. G. M.: Arctic Great Rivers Observatory Discharge Dataset, Version 20220630, Arct. Gt. Rivers Obs. Discharge, https://arcticgreatrivers.org/data/ (last access: 1 August 2022), 2021b.
Stettner, S., Beamish, A. L., Bartsch, A., Heim, B., Grosse, G., Roth, A., and Lantuit, H.: Monitoring Inter- and Intra-Seasonal Dynamics of Rapidly Degrading Ice-Rich Permafrost Riverbanks in the Lena Delta with TerraSAR-X Time Series, Remote Sens., 10, 51, https://doi.org/10.3390/rs10010051, 2018.
Stolbovoi, V.: Carbon in Russian soils, Climatic Change, 55, 131–156, https://doi.org/10.1023/A:1020289403835, 2002.
Stolpmann, L., Mollenhauer, G., Morgenstern, A., Hammes, J. S., Boike, J., Overduin, P. P., and Grosse, G.: Origin and Pathways of Dissolved Organic Carbon in a Small Catchment in the Lena River Delta, Front. Earth Sci., 9, 1303, https://doi.org/10.3389/feart.2021.759085, 2022.
Stone, T. A. and Schlesinger P.: RLC Forest Cover Map of the Former Soviet Union, 1990, ORNL DAAC: Oak Ridge, TN, USA, https://doi.org/10.3334/ORNLDAAC/691, 2003.
Strauss, J., Schirrmeister, L., Grosse, G., Wetterich, S., Ulrich, M., Herzschuh, U., and Hubberten, H.-W.: The deep permafrost carbon pool of the Yedoma region in Siberia and Alaska, Geophys. Res. Lett., 40, 6165–6170, https://https://doi.org/10.1002/2013GL058088, 2013.
Strauss, J., Abbott, B., Hugelius, G., Schuur, E. A. G., Treat, C., Fuchs, M., Schädel, C., Ulrich, M., Turetsky, M. R., Keuschnig, M., Biasi, C., Yang, Y., and Grosse, G.: Permafrost, in: Recarbonizing global soils – A technical manual of recommended management practices, edited by: Food and Agriculture Organization of the United Nations (FAO), FAO, Rome, Italy, 127–147, ISBN: 978-92-5-134838-3, https://doi.org/10.4060/cb6386en, 2021a.
Strauss, J., Laboor, S., Schirrmeister, L., Fedorov, A. N., Fortier, D., Froese, D., Fuchs, M., Günther, F., Grigoriev, M., Harden, J., Hugelius, G., Jongejans, L. L., Kanevskiy, M., Kholodov, A., Kunitsky, V., Kraev, G., Lozhkin., A., Rivkina, E., Shur, Y., Siegert, C., Spektor, V., Streletskaya, I., Ulrich, M., Vartanyan, S., Veremeeva, A., Walter, A. K., Wetterich, S., Zimov, N., and Grosse, G.: Circum-Arctic Map of the Yedoma Permafrost Domain, Front. Earth Sci., 9, 758360, https://https://doi.org/10.3389/feart.2021.758360, 2021b.
Stuiver, M. and Polach, H. A.: Discussion Reporting of 14C Data, Radiocarbon, 19, 355–363, https://doi.org/10.1017/S0033822200003672, 1977.
Tananaev, N. and Lotsari, E.: Defrosting northern catchments: Fluvial effects of permafrost degradation, Earth-Sci. Rev., 228, 103996, https://doi.org/10.1016/j.earscirev.2022.103996, 2022.
Tanski, G., Wagner, D., Knoblauch, C., Fritz, M., Sachs, T., and Lantuit, H.: Rapid CO2 release from eroding permafrost in seawater, Geophys. Res. Lett., 46, 11244–11252, https://doi.org/10.1029/2019GL084303, 2019.
Terhaar, J., Lauerwald, R., Regnier, P., Gruber, N., and Bopp, L.: Around one third of current Arctic Ocean primary production sustained by rivers and coastal erosion, Nat. Commun., 12, 1–10, https://doi.org/10.1038/s41467-020-20470-z, 2021.
Vihma, T., Screen, J., Tjernström, M., Newton, B., Zhang, X., Popova, V., Deser, C., Holland, M., and Prowse, T.: The atmospheric role in the Arctic water cycle: A review on processes, past and future changes, and their impacts, J. Geophys. Res.-Biogeo., 121, 586–620, https://https://doi.org/10.1002/2015JG003132, 2016.
Vonk, J. E., Sánchez-García, L., Semiletov, I., Dudarev, O., Eglinton, T., Andersson, A., and Gustafsson, Ö.: Molecular and radiocarbon constraints on sources and degradation of terrestrial organic carbon along the Kolyma paleoriver transect, East Siberian Sea, Biogeosciences, 7, 3153–3166, https://doi.org/10.5194/bg-7-3153-2010, 2010.
Vonk, J. E., Semiletov, I. P., Dudarev, O. V., Eglinton, T. I., Andersson, A., Shakhova, N., Charkin, A., Heim, B., and Gustafsson, Ö.: Preferential burial of permafrost-derived organic carbon in Siberian-Arctic shelf waters, J. Geophys. Res.-Ocean., 119, 8410–8421, https://https://doi.org/10.1002/2014JC010261, 2014.
Vonk, J. E., Tesi, T., Bröder, L., Holmstrand, H., Hugelius, G., Andersson, A., Dudarev, O., Semiletov, I., and Gustafsson, Ö.: Distinguishing between old and modern permafrost sources in the northeast Siberian land–shelf system with compound-specific δ2H analysis, The Cryosphere, 11, 1879–1895, https://doi.org/10.5194/tc-11-1879-2017, 2017.
Vonk, J. E., Tank, S. E., and Walvoord, M. A.: Integrating hydrology and biogeochemistry across frozen landscapes, Nat. Commun., 10, 3–6, https://doi.org/10.1038/s41467-019-13361-5, 2019.
Walvoord, M. A. and Striegl, R. G.: Increased groundwater to stream discharge from permafrost thawing in the Yukon River basin: potential impacts on lateral export of carbon and nitrogen, Geophys. Res. Lett., 34, L12402, https://https://doi.org/10.1029/2007GL030216, 2007.
Walvoord, M. A. and Kurylyk, B. L.: Hydrologic Impacts of Thawing Permafrost – A Review, Vadose Zone J., 15, 1–20, https://doi.org/10.2136/vzj2016.01.0010, 2016.
Wang, K., Zhang, T., and Yang, D.: Permafrost dynamics and their hydrologic impacts over the Russian Arctic Drainage Basin, Adv. Clim. Change Res., 12, 482–498, https://doi.org/10.1016/j.accre.2021.03.014, 2021a.
Wang, P., Huang, Q., Pozdniakov, S. P., Liu, S., Ma, N., Wang, T., Zhang, Y., Yu, J., Xie, J., Fu, G., Frolova, N. L., and Liu, C.: Potential role of permafrost thaw on increasing Siberian river discharge, Environ. Res. Lett., 16, 034046, https://doi.org/10.1088/1748-9326/abe326, 2021b.
Wild, B., Andersson, A., Bröder, L., Vonk, J., Hugelius, G., and Mcclelland, J. W.: Rivers across the Siberian Arctic unearth the patterns of carbon release from thawing permafrost, P. Natl. Acad. Sci. USA, 116, 10280–10285, https://doi.org/10.1073/pnas.1811797116, 2019.
Winterfeld, M., Laepple, T., and Mollenhauer, G.: Characterization of particulate organic matter in the Lena River delta and adjacent nearshore zone, NE Siberia – Part I: Radiocarbon inventories, Biogeosciences, 12, 3769–3788, https://doi.org/10.5194/bg-12-3769-2015, 2015.
Yang, D., Kane, D. L., Hinzman, L. D., Zhang, X., Zhang, T., and Ye, H.: Siberian Lena River hydrologic regime and recent change, J. Geophys. Res. Atmos., 107, D23, https://doi.org/10.1029/2002JD002542, 2002.
Yang, D., Park, H., Peterson, A., and Liu, B.: Arctic River Water Temperatures and Thermal Regimes, in: Arctic Hydrology, Permafrost and Ecosystems, edited by: Yang, D. and Kane, D. L., Springer, Cham, ISBN: 978-3-030-50928-6, online ISBN: 978-3-030-50930-9, https://doi.org/10.1007/978-3-030-50930-9_10, 2021.
Zhang, S. M., Mu, C. C., Li, Z. L., Dong, W. W., Wang, X. Y., Streletskaya, I., Grebenets, V., Sokratov, S., Kizyakov, A., and Wu, X. D.: Export of nutrients and suspended solids from major Arctic rivers and their response to permafrost degradation, Adv. Clim. Change Res., 12, 466–474, https://doi.org/10.1016/j.accre.2021.06.002, 2021.