the Creative Commons Attribution 4.0 License.
the Creative Commons Attribution 4.0 License.
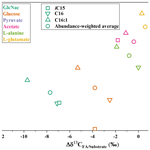
Impact of metabolism and temperature on 2H ∕ 1H fractionation in lipids of the marine bacterium Shewanella piezotolerans WP3
Xin Chen
Weishu Zhao
Liang Dong
Huahua Jian
Lewen Liang
Jing Wang
Fengping Wang
Compound-specific hydrogen isotopes have increasingly been used as a powerful proxy for investigating biogeochemical cycles and climate change over the past 2 decades. Understanding the hydrogen isotope in extant organisms is fundamental for us to interpret such isotope signals preserved in natural environmental samples. Here, we studied the controls on hydrogen isotope fractionation between fatty acids and growth water by an Fe-reducing heterotrophic marine bacterium Shewanella piezotolerans WP3 growing on different organic substrates, including N-acetyl-D-glucosamine (GlcNac), glucose, acetate, pyruvate, L-alanine, and L-glutamate. Meanwhile, we also evaluated the impact of growth temperature on the hydrogen isotope composition of fatty acids using GlcNac as the sole organic substrate. Our results show that the abundance-weighted mean fatty-acid / water fractionations () display considerable variations for cultures grown on different substrates. Specifically, WP3 yielded the most 2H-enriched fatty acids growing on L-glutamate and pyruvate with an of 52 ± 14 ‰ and 44 ± 4 ‰, respectively, and exhibited 2H depletion using GlcNac (−76 ± 1 ‰) and glucose (−67 ± 35 ‰) as sole carbon sources and relatively small fractionations on acetate (23 ± 3 ‰) and L-alanine (−4 ± 9 ‰). Combined with metabolic model analysis, our results indicate that the central metabolic pathways exert a fundamental effect on the hydrogen isotope composition of fatty acids in heterotrophs. Temperature also has an obvious influence on the δ2H values of fatty acids, with strong 2H depletion at an optimal growth temperature (−23 ± 2 ‰ and −23 ‰ growing at 15 and 20 ∘C, respectively) and relatively small fractionations at non-optimal temperatures (4 ± 5 ‰, −4 ± 12 ‰, and 15 ± 41 ‰ at 4, 10, and 25 ∘C, respectively). We hypothesized that this may be associated with temperature-induced enzyme activity for nicotinamide adenine dinucleotide phosphate (NADPH) production. This study helps understand the controlling factors of hydrogen isotope fractionation by marine bacteria, laying the foundation for further interpreting the hydrogen isotope signatures of lipids as an important proxy to decode the biogeochemical cycles and ecological changes in marine sediments.
- Article
(4766 KB) - Full-text XML
-
Supplement
(742 KB) - BibTeX
- EndNote
The hydrogen isotope composition of lipids preserved in sediments is widely applied to trace biogeochemical processes and study regional paleo-hydrological cycles (Hayes, 2001; Huang et al., 2004; Sachse et al., 2012; Sessions, 2016). During the past 2 decades, compound-specific hydrogen isotopes have increasingly been utilized as a valid proxy for reconstructing precipitation δ2H because relatively constant fractionations in terrestrial plants and phototrophic algae are assumed (Sachse et al., 2012; Sessions, 2016; Huang and Meyers, 2019). However, increasing numbers of studies have found that there are large ranges of hydrogen isotope ratios in lipids (up to 700 ‰) from various environmental samples, including microbial mats from hot springs (Naraoka et al., 2010; Osburn et al., 2011), marine particulate organic matter (Jones et al., 2008; Heinzelmann et al., 2016), and marine and lacustrine sediments (Li et al., 2009; Chen et al., 2021). This raises questions as to what factors cause such isotope signals in natural environmental samples. Clues are found in a breakthrough culture study by X. Zhang et al. (2009), who reported that hydrogen isotope compositions of lipids are correlated with microbial metabolisms, with strong depletion in 2H for chemoautotrophs but slight depletion for phototrophs and enrichment for heterotrophs. These features provide potential insights for interpreting hydrogen isotope signals in natural environments, and we can further infer that the metabolism of microbes thrives in modern and ancient environments.
Among these microbes, heterotrophs are of particular concern due to their essential role in the organic carbon cycle on earth (Moran et al., 2022). Understanding the hydrogen isotope composition of lipids in heterotrophs with different central metabolic pathways is important for decoding such isotope signals in geologic archives. Recently, in a systematic laboratory culture, Wijker et al. (2019) showed that aerobic heterotrophic bacteria display the largest range of variations, with values ranging from ‰ to +400 ‰, which are quantitatively related to the central metabolic pathways. In particular, heterotrophic bacteria exhibit the strongest 2H enrichment growing on tricarboxylic acid (TCA) cycle substrates (e.g., acetate, succinate and citrate), but they show 2H depletion on sugars (Wijker et al., 2019). The most plausible explanations of hydrogen isotopic differences are related to central metabolic pathways in the production of nicotinamide adenine dinucleotide phosphate (NADPH), which is the major source of H (>50 %) in fatty acids (Jackson et al., 1999; X. Zhang et al., 2009; Heinzelmann et al., 2015a, b; Wijker et al., 2019). Combined with laboratory experiments and metabolic flux model analysis, values show positive correlations with the relative flux (% of glucose uptake) of Entner–Doudoroff (ED) and TCA cycle pathways, while they show negative correlations with Embden–Meyerhof–Parnas (EMP) and pentose phosphate (PP) pathways (Wijker et al., 2019). However, the most investigated species by previous studies are restricted to model bacteria (e.g., Escherichia coli and Rhizobium radiobacter) and are unlikely to be widely distributed in natural environments. More importantly, understanding the hydrogen isotopes generated in a bacterium with wide distributions and ecological functions is essential for providing further constraints on the isotope signals in natural environments.
Compared with metabolisms, several other factors such as the lipid biosynthetic pathway, growth phase and rate, salinity, and pressure have been thought to potentially influence the hydrogen isotope composition of lipids (Heinzelmann et al., 2015a, b; Zhao et al., 2020; Dirghangi and Pagani, 2013a). For example, long-chain polyunsaturated fatty acids biosynthesized through the polyketide pathway have much lower δ2H values than those of short-chain lengths via the fatty-acid synthase (FAS) pathway (Fang et al., 2014). The values of the C16 fatty acid in Pseudomonas strain LFY10 are increased by only about 10 ‰–15 ‰ from exponential to stationary phases (Heinzelmann et al., 2015b). For environmental factors, Heinzelmann et al. (2015a) showed that the hydrogen isotope ratios of fatty acids exhibited no obvious correlations with salinity in cultures of heterotrophic microbe Pseudomonas strain LFY10, while they were enriched in 2H with increasing salinity in the phototrophic algae Isochrysis galbana. Hydrogen isotope fractionations exhibited an inverse correlation with growth pressure in a deep-sea gram-positive piezotolerant bacterium Sporosarcina sp. DSK25 (Zhao et al., 2020). Still, the variations associated with these factors are rather small (∼10 ‰–20 ‰) compared with metabolisms. Temperature is one of the most important environmental factors, and culture studies have demonstrated its impacts on the hydrogen isotope composition of lipids in phototrophs and archaea (Schouten et al., 2006; Dirghangi and Pagani, 2013b). Until now, however, there have been no cultures focusing on whether or not temperature can affect lipid hydrogen isotopes in heterotrophic bacteria and, if so, by what relationship between fractionations and growth temperatures.
The Shewanella genus is ubiquitously distributed around the globe, such as in surface freshwater and the deepest marine trenches, and can use various electron acceptors for metabolic activities (Hau and Gralnick, 2007; Fredrickson et al., 2008). Shewanella piezotolerans WP3 is a gram-negative, moderately halophilic bacterium within Shewanella genus and has an important role in the biochemical cycle of organic matter in the deep sea (Xiao et al., 2007; Lemaire et al., 2020). In our previous studies, the metabolisms of S. piezotolerans WP3 have been systematically studied through pure cultures and genomics (Wang et al., 2008; Dufault-Thompson et al., 2017). Specifically, S. piezotolerans WP3 can use a variety of organic substrates such as GlcNac, acetate, glucose, pyruvate, and amino acid as sole carbon sources (Xiao et al., 2007). Furthermore, it has relatively wide ranges of growth temperatures from 0–28 ∘C, with an optimal temperature at 15–20 ∘C (Wang et al., 2004). Here, we investigate the compound-specific hydrogen isotopes in S. piezotolerans WP3 growing on different substrates, and also discuss the effect of temperatures on hydrogen isotope fractionations using GlcNac as the sole carbon source. Then, we compare the fractionations with a metabolic flux model to decode the detail fractionation mechanisms in heterotrophic bacteria. Our study can provide the characterization of hydrogen isotope fractionations in S. piezotolerans WP3 lipids and may give insights into the biochemical cycle and ecological functions in Fe-reducing oceanic sediments.
2.1 Strains, media, and growth conditions
S. piezotolerans WP3 was first isolated from west Pacific deep-ocean sediment at a depth of 1914 m (Xiao et al., 2007). S. piezotolerans WP3 cultures have been reported by our previous study (Chen et al., 2022). Briefly, S. piezotolerans WP3 was grown in batch culture in a growth medium containing the following reagents (g L−1): NaCl (26.0), MgCl2⋅6H2O (5.0), CaCl2⋅2H2O (1.4), Na2SO4 (4.0), NH4Cl (1.5), KH2PO4 (0.1), and KCl (0.5) as well as trace minerals and vitamins. To examine the hydrogen fractionations of metabolic type, GlcNac, glucose, sodium acetate, sodium pyruvate, and amino acids were supplemented to each culture as the sole carbon source, with a final concentration of 4 g L−1 and culturing at 20 ∘C and shaking at 200 rpm. WP3 was grown at 4, 10, 15, 20, and 25 ∘C using GlcNac as the sole carbon source under static conditions for investigating the relationship between growth temperature and hydrogen isotope fractionation. All media were passed through a 0.22 µm filter. Final pH was adjusted to about 7.0. For water isotope analysis, 2 mL samples were taken from each culture before incubation. Duplicate cultures were maintained for each condition, and cells were harvested in early stationary phase. Samples of 20 mL were taken, washed with culture salt solutions, and centrifuged for 20 min at 4500 g; cell pellets were stored at −20 ∘C before analysis.
2.2 Extraction of cellular lipids
Cellular lipid extraction and analysis follows the procedure described in Rodríguez-Ruiz et al. (1998). Briefly, about 1–20 mg freeze-dried biomass was transesterified using 1 mL hexane and 2 mL 20 : 1 anhydrous methanol : acetyl chloride; then it was heated at 100 ∘C for 10 min. After cooling at room temperature, 2 mL water was added into the mixture, followed three times by extraction using 3 mL hexane. The extracts were dried under nitrogen at room temperature. The fatty-acid methyl ester (FAME) fraction was purified via a silica gel flash column using dichloromethane (DCM) as the eluent. After purification, the FAMEs were analyzed using a gas chromatography/mass spectrometry (GC-MS) system with an Agilent DB-1 column (30 m × 320 µm × 0.17 µm). Compounds were identified by comparison of mass spectra with the published and library data (Chen et al., 2022).
2.3 Hydrogen isotope analysis
The hydrogen isotope compositions of FAMEs were determined using an HP 6890 gas chromatograph interfaced to a MAT 253 isotope ratio mass spectrometer using a pyrolysis interface. The GC was fit with a wax column () and the temperature program was as follows: the oven temperature was held at 100 ∘C for 1 min, then increased by 20 to 200 ∘C and then followed by a 10 increase up to 310 ∘C and was isothermal for 7 min. The C20 FAME standard with known δ2H values from Indiana University was measured after every sixth sample injection. The low and high relative-abundance FAMEs were analyzed separately to ensure proper signal amplitudes and to obtain accurate hydrogen isotope ratios. The δ2H values of FAMEs were corrected for the isotopic contribution of the hydrogen in the methyl group added during methylation using the following formula: , where n is the number of carbons in the fatty acids and −55.8 ‰ is the δ2H value of the added methyl group. The average standard deviation of the triplicate analysis of each compound was smaller than 5 ‰. Hydrogen isotope fractionations between fatty acids and growth water were calculated as .
Culture water samples were analyzed using a MAT 253 isotope ratio mass spectrometer. The measurement was normalized to the Vienna Standard Mean Ocean Water and the Standard Light Antarctic Precipitation (VSMOW-SLAP) scale using a two-point linear calibration generated from reference waters supplied by IAEA, with an analytical precision and accuracy of ±0.6 ‰ for δ2H.
3.1 Fatty-acid distribution
The composition of fatty acids in S. piezotolerans WP3 growing on different organic substrates has been reported by our previous study (Chen et al., 2022). The lipid compositions are similar, growing on different organic substrates and temperatures. There are no systematic relationships between fatty-acid abundance and growth substrate. Briefly, the carbon chain lengths of n-alkanoic acids vary from C12 to C20, peaking at C16 and C16 : 1. Many kinds of low-melting-point fatty acids including branched-chain fatty acids (iC14, iC15, iC16, and iC17), monounsaturated fatty acids (C14 : 1, C16 : 1, and C18 : 1) and C20 : 5 are found. The relative abundance of these three low-melting-point fatty acids displays a trend towards increasing by decreasing the culture temperature.
3.2 Hydrogen isotope ratio of fatty acids growing on different organic substrates
The hydrogen isotope composition of fatty acids is listed in Table 1. The δ2H values of fatty acids vary considerably between cultures on different substrates. On the whole, the hydrogen isotope composition of fatty acids is most depleted in 2H growing on GlcNac and glucose and slightly 2H-depleted on L-alanine (Table 1, Figs. 1 and S1 in the Supplement). Conversely, fatty acids are slightly enriched in 2H using pyruvate and acetate as the sole carbon, while they are strongly enriched in 2H growing on L-glutamate (Table 1, Figs. 1 and S1). Considerable variations are observed in different chain lengths of fatty acids from the same culture (Table 1). The abundance-weighted mean δ2H values growing on GlcNac are −94 ± 1 ‰ (n=2), and they are a little lower than that on glucose (−86 ± 35 ‰, n=2). Fatty acids have a similar range on cultures when pyruvate (24 ± 4 ‰, n=2) and acetate (3 ± 3 ‰, n=2) are used as the sole organic carbon. The abundance-weighted mean δ2H values are −24 ± 9 ‰ (n=2) and 32 ± 14 ‰ (n=2) growing on L-alanine and L-glutamate, respectively.
Table 1The hydrogen isotopic values of fatty acids in S. piezotolerans WP3 growing on different organic substrates at 20 ∘C and shaking at 200 rpm; “–” indicates that the concentrations are too low for δ2H measurements. Standard deviations of two duplicate cultures are given in parentheses.
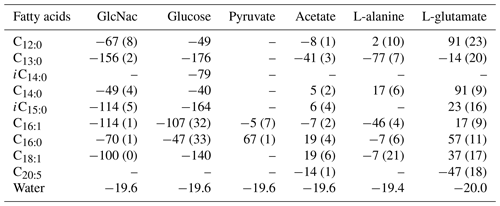
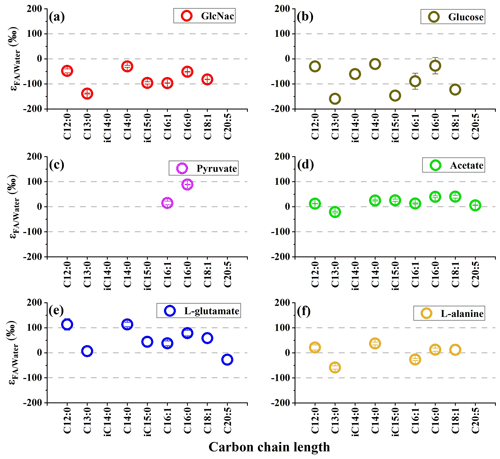
Figure 1The hydrogen isotope fractionation between fatty acids and growth water in S. piezotolerans WP3 growing on different substrates including GlcNac, acetate, D-glucose, pyruvate, and amino acids at 20 ∘C and shaking at 200 rpm. The error bars represent the 1σ standard deviations of duplicate cultures.
3.3 Hydrogen isotope ratios of fatty acids at different growth temperatures
We investigate the hydrogen isotope composition of fatty acids in S. piezotolerans WP3 using GlcNac as the sole carbon growing at different temperatures (4, 10, 15, 20, and 25 ∘C). The abundance-weighted mean δ2H values exhibit considerable ranges, with lower values at optimal growth temperatures (15 and 20 ∘C) and higher values at non-optimal temperatures (Table 2 and Fig. 2). Specifically, the mean δ2H values are −23 ± 2 ‰ (n=2) and −23 ‰ growing at 15 and 20 ∘C, respectively. WP3 growing at 4, 10, and 25 ∘C has mean δ2H values of 4 ± 5 ‰, −4 ± 12 ‰, and to 15 ± 41 ‰.
Table 2The hydrogen isotopic values of fatty acids in S. piezotolerans WP3 growing at different temperatures using GlcNac as the sole carbon source. For better comparison, none of the cultures have been shaken; “–” indicates that the concentrations are too low for δ2H measurements. Standard deviations of two duplicate cultures are given in parentheses.
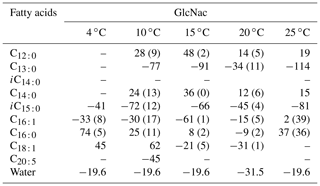
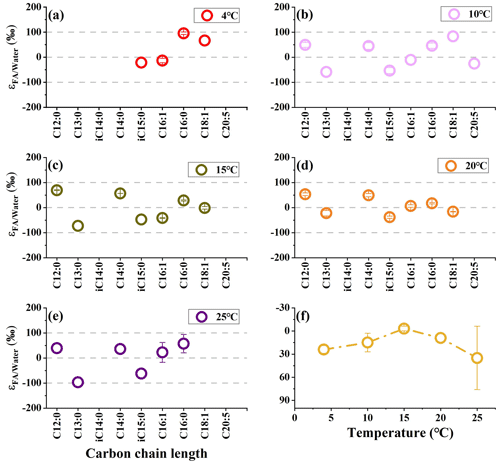
Figure 2The hydrogen isotope composition of fatty acids in S. piezotolerans WP3 growing at different temperatures. Panel (f) shows the abundance-weighted mean values at different temperatures. The error bars represent the 1σ standard deviations of duplicate cultures. None of the cultures have been shaken.
4.1 Hydrogen isotope composition of individual fatty acids
The δ2H values of individual fatty acids vary considerably in the same culture with values ranging from 60 ‰–138 ‰. This indicates biosynthesis pathways have an obvious influence on the δ2H values of individual fatty acids. Specifically, the different chain length of saturated fatty acids have similar δ2H values, suggesting chain elongation processes by enzymes have no obvious influence on hydrogen isotope values. The δ2H values of monounsaturated fatty acids (C16:1) are slightly lower than those of saturated fatty acids (C16) with difference in values from 26 ‰–72 ‰. It is also observed in other heterotrophs that the desaturation process can result in more 2H depletion in monounsaturated fatty acids (X. Zhang et al., 2009; Wijker et al., 2019). Compared with saturated fatty acids, the branched-chain lengths are more depleted in 2H composition, suggesting precursors for anteiso- and iso-fatty acids may have relatively lower δ2H values. The branched acyl-coenzyme A (e.g., isobutyryl-CoA and isovaleryl-CoA) are important precursors for branched-chain fatty-acid biosynthesis, while acetyl-CoA is an important precursor for saturated fatty acids (Hayes, 2001). Wijker et al. (2019) also found that branched fatty acids (iC15 and aC15) have relatively lower δ2H values compared with C16 fatty acid in five species of heterotrophic bacterium. It is interesting to note that C13 and C20:5 in S. piezotolerans WP3 have the lowest δ2H values among these fatty acids. As reported by Fang et al. (2014), polyunsaturated fatty acids (C22:6) synthesized through the polyketide pathway have much lower δ2H values than C16 via the fatty-acid synthase (FAS) pathway in a piezophilic bacterium Moritella japonica DSK1. All these results suggest that fatty-acid biosynthesis processes including desaturation, different precursors, and biosynthetic pathway have considerable influence on the hydrogen isotope composition in S. piezotolerans WP3.
4.2 Variations in fractionation growing on different organic substrates
The range of δ2H values for different fatty-acid structures in heterotrophic bacteria is often large (>100 ‰) (X. Zhang et al., 2009; Osburn et al., 2016). In this study, S. piezotolerans WP3 grown on glucose exhibits a range of δ2H value varying from −176 ‰ to −40 ‰, while the range is relatively smaller on acetate (from −41 ‰–19 ‰) (Table 1 and Fig. 1). To facilitate quantitative comparison between fractionation and different organic substrates, we calculate an abundance-weighted mean hydrogen isotope fractionation between fatty acids and growth water () and standard deviation for each duplicate cultures. Our results show that values are substantially influenced by the type of organic carbon assimilated during growth (Fig. 1). Specifically, fatty acids are mostly enriched in 2H growing on the direct precursor of the TCA cycle such as amino acids (L-glutamate and L-alanine), pyruvate, and acetate with average values from −4 ‰–52 ‰, while fatty acids exhibited 2H depletion using GlcNac and glucose as the sole carbon source with values of −76 ‰ and −67 ‰, respectively. As demonstrated by many previous culture experiments (X. Zhang et al., 2009; Wijker et al., 2019), the variation in lipid δ2H values is not related to organic substrate isotopic composition because relatively little H from organic substrate is incorporate into fatty acids (Sessions et al., 1999; White et al., 2005). In contrast, the fractionation is substantially influenced by the central metabolic pathways, which are driven by the type of organic carbon source assimilated in all analyzed strains (X. Zhang et al., 2009; Dirghangi and Pagani, 2013a; Heinzelmann et al., 2015a, b; Wijker et al., 2019).
Comparison of our results with other aerobic heterotrophic bacterial cultures exhibits consistent fractionation patterns. For example, heterotrophs grown on glucose produced fatty acids were 2H-depleted relative to source water (X. Zhang et al., 2009; Wijker et al., 2019). The metabolism of glucose by S. piezotolerans WP3 involves the Embden–Meyerhof pathway to pyruvate, and then pyruvate is transformed to acetyl-CoA via decarboxylation, and most of the acetyl-CoA enters into TCA cycle (Fig. 3). When grown on pyruvate and a direct precursor (acetate) of the TCA cycle, S. piezotolerans WP3 fatty acids were 2H-enriched compared to water, similar to that observed in Cupriavidus necator strain H16 and Bacillus subtilis cultures (Wijker et al., 2019; X. Zhang et al., 2009). Finally, when amino acids (L-alanine and L-glutamate) were used as organic substrates for heterotrophic bacteria, fatty acids were 2H-enriched relative to water or there was no substantial fractionation. According to the metabolism of these two amino acids in S. piezotolerans WP3 (Fig. 3), L-alanine was transformed to pyruvate that subsequently participates in the TCA cycle, while L-glutamate was directly metabolized via the TCA cycle pathway to acetyl-CoA. All these results indicate that variation in the fractionation in S. piezotolerans WP3 grown on different types of organic substrates is most likely correlated with metabolisms.
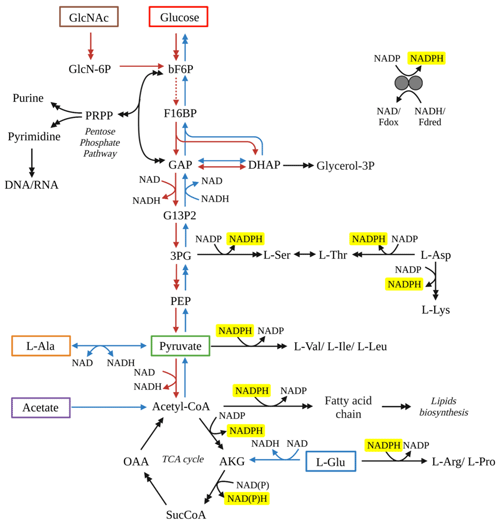
Figure 3Schematic diagram of the metabolic pathways for WP3 by utilizing various substrates. Tested substrates in this study include GlcNac (brown box), glucose (red box), acetate (purple box), pyruvate (green box), L-alanine (orange box) and L-glutamate (blue box). Red arrows represent reactions through glycolysis pathways, while blue arrows represent reactions through glycogenesis pathway. Black arrows represent reactions required no matter which substrate was used. Double arrows indicate that multiple reactions are involved in the conversion between two compounds shown. All reactions with NADPH as a cofactor have been highlighted with yellow background. Abbreviations: 3PG, 3-phospho-D-glycerate; Akg, 2-oxoglutarate; bF6P, beta-D-fructose 6-phosphate; DHAP, dihydroxyacetone phosphate; F16BP, beta-D-fructose 1,6-bisphosphate; G13P2, D-glycerate 1,3-diphosphate; G6P, D-glucose 6-phosphate; GAP, glyceraldehyde 3-phosphate; GlcN-6P, D-glucosamine 6-phosphate; GlcNAc, N-acetyl-D-glucosamine; Glycerol-3P, glycerol 3-phosphate; OAA, oxaloacetate; PEP, phosphoenolpyruvate; Pi, phosphate; PPi, diphosphate; SucCoA, succinate CoA.
Processes associated with NADPH production are crucial in controlling the lipid fractionation (Wijker et al., 2019), as NADPH is the dominant source (>50 %) of hydrogen in fatty acids (Sessions, 2016). The dehydrogenase reactions and transhydrogenases interconversion between nicotinamide adenine dinucleotide (NADH) and NADPH are all associated with a large hydrogen isotopic fractionation (Wijker et al., 2019). Specifically, the NADP+ is reduced through the pentose phosphate and glycolysis pathway resulting in 2H depletion of NADPH; then fatty acids were 2H-depleted relative to source water (Wijker et al., 2019; X. Zhang et al., 2009). In contrast, heterotrophic bacteria growing on TCA cycle organic substrates produce strongly 2H-enriched NADPH via the associated NADPH-reducing reactions and the conversion of NADPH to NADH catalyzed by transhydrogenases.
For S. piezotolerans WP3 growing on GlcNac and glucose, NADPH is mainly produced via the pentose phosphate and glycolysis pathway (Fig. 3). Thus, relatively lower δ2H values of fatty acids are observed growing on GlcNac and glucose; the same order has also been observed previously (X. Zhang et al., 2009; Wijker et al., 2019). However, growth on glucose yields different fractionations in this study, with five heterotrophic bacteria investigated by Wijker et al. (2019). E. coli and B. subtilis fatty acids are −155 ‰ to −131 ‰ 2H-depleted, R. radiobacter and Ensifer meliloti fatty acids are −15 ‰ to −6 ‰ depleted, and Pseudomonas fluorescens is 133 ‰ 2H-enriched compared to growth water (Fig. 4; Wijker et al., 2019). Fatty acids in this study are −67 ‰ 2H-depleted relative to growth water. It is surprising that the glucose metabolism in the above aerobic heterotrophic bacteria produces significantly different isotope ratios of NADPH. A systematic experiment by Wijker et al. (2019) demonstrated that changes in metabolic fluxes have an essential role in controlling the hydrogen isotope composition of lipids, with high ED and TCA fluxes relative to PP and EMP being associated with more 2H-enriched lipids and vice versa (Wijker et al., 2019). Therefore, compared with R. radiobacter, E. meliloti, and P. fluorescens strains, relatively higher PP and EMP fluxes in S. piezotolerans WP3 may cause more 2H-depleted lipids.
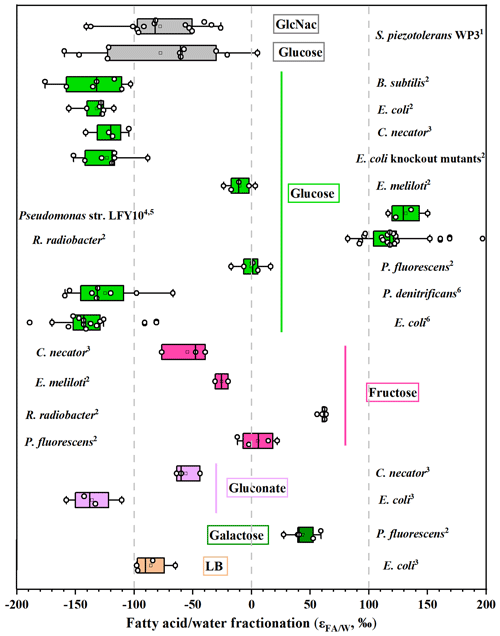
Figure 4Compilation of measured hydrogen isotope fractionations growing on sugars. For each organism, bars represent the range of values for all individual fatty acids. The vertical line is the average value. Data sources: 1 this study; 2 Wijker et al. (2019); 3 X. Zhang et al. (2009); 4,5 Heinzelmann et al. (2015a, b); 6 Osburn et al. (2016).
Growth on pyruvate also yields different fractionations in this study, with six aerobic heterotrophic bacteria investigated by X. Zhang et al. (2009) and Wijker et al. (2019). E. coli fatty acids are −57 ‰ to −52 ‰ 2H-depleted, but B. subtilis, R. radiobacter, P. fluorescens, C. necator, and E. meliloti fatty acids are 18 ‰–220 ‰ 2H-enriched (Fig. 5; Wijker et al., 2019), and fatty acids in the current study are 44 ‰ 2H-enriched compared to growth water. According to the metabolic flux model, one possible explanation is that the portion of pyruvate utilized for various metabolic pathways such as the TCA cycle and the production of amino acids, formate, lipids, and acetyl-CoA vary for different strains. Another is that the conversion of pyruvate to amino acids also consumes NADPH (Fig. 3); this process may affect the hydrogen isotope composition of the NADPH pool. Further, different fractionations are also observed in different aerobic heterotrophic bacteria growing on a TCA cycle intermediate (acetate). Acetate can be metabolized directly to form acetyl-CoA, which is an important intermediate substrate for various biochemical reactions (Fig. 3). As a result, the pool of precursors or intermediates of the TCA cycle and δ2H values of NADPH utilized for lipid synthesis could be different, as reflected by the lipid fractionation. Up to now, however, there are no flux measurements for these investigated species growing on TCA cycle substrates. Therefore, isotope labeling experiments should be used to determine the associated metabolic flux growing on pyruvate and TCA cycle substrates for decoding the variations in fractionation in different strains.
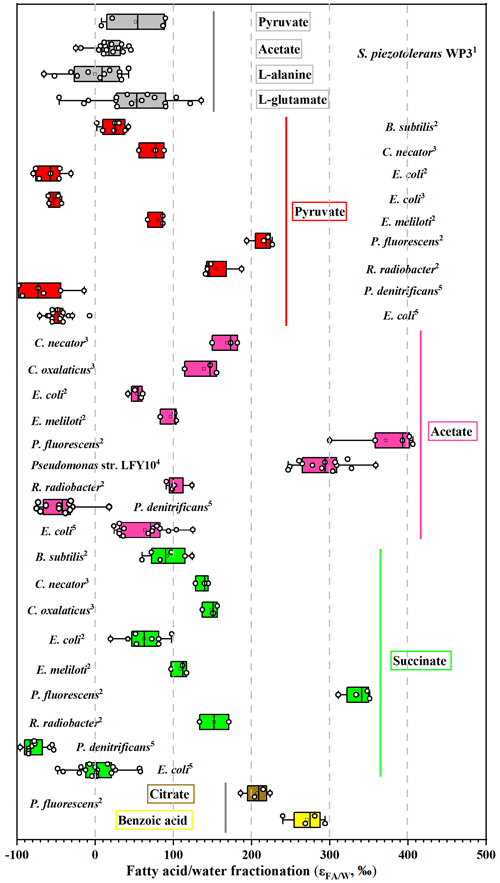
Figure 5Compilation of measured hydrogen isotope fractionations growing on pyruvate and TCA cycle substrates. For each organism, bars represent the range of values for all individual fatty acids. The vertical line is the average value. Data sources: 1 this study; 2 Wijker et al. (2019); 3 X. Zhang et al. (2009); 4,5 Heinzelmann et al. (2015a).
4.3 Influence of growth temperature on the hydrogen isotope composition of fatty acids
In this study, the abundance-weighted mean δ2H values of fatty acids in S. piezotolerans WP3 exhibit considerable ranges, with lower values at optimal growth temperatures (15 and 20 ∘C) and higher values at non-optimal temperatures (4, 10, and 25 ∘C; Fig. S2). Many previous culture experiments have shown that growth temperature exerts significant effects on the hydrogen isotope composition of lipids in photoautotrophic algae (Z. Zhang et al., 2009; Schouten et al., 2006). The lipid δ2H values are decreased by increasing growth temperatures below the optimal growth temperature, while values increase by further increasing temperatures above the optimal growth temperature (Schouten et al., 2006; Z. Zhang et al., 2009) These similar results are also observed in a halophilic archaean Haloarcula marismortui studied by Dirghangi and Pagani (2013b). In contrast, one culture study (Tetrahymena thermophila) indicates fatty acids are more 2H-enriched by increasing growth temperature (Dirghangi and Pagani, 2013b). The mechanisms may be associated with growth rates and enzyme activities in organisms controlled by temperature (Dirghangi and Pagani, 2013b; X. Zhang et al., 2009). The possible mechanisms are that temperature affects lipid hydrogen isotope composition through growth rates and enzyme activities in organisms (Dirghangi and Pagani, 2013b; X. Zhang et al., 2009). The effect of growth rates on hydrogen isotope composition is mainly determined by the proportion of intracellular water from the metabolism (Kreuzer-Martin et al., 2006). Enzyme activities are also strongly related to temperature because organisms produce NADPH through different metabolisms in response to temperature change (X. Zhang et al., 2009). We will discuss these potential factors separately below.
S. piezotolerans WP3 is psychrotolerant and can grow at 0–28 ∘C, with an optimal growth temperature at 15 to 20 ∘C (Wang et al., 2004). The growth rate of S. piezotolerans WP3 is temperature dependent on the highest rates of optimal growth temperature (Xiao et al., 2007). Our results show that relatively larger fractionation is present at a higher growth rate (15 and 20 ∘C), while smaller fractionation is observed at relatively lower rates (4, 10, and 25 ∘C; Fig. 2). This is similar to previous results according to which halophilic archaea yield more 2H-depleted lipids at an optimal growth temperature, while lipids become 2H-enriched (11 ‰) with the temperature increasing above 40 ∘C (Dirghangi and Pagani, 2013a). These observations point to growth rate being the dominant factor causing the observed variations in lipid fractionations. A probable mechanism is that the growth rate affects lipid δ2H via hydrogen atom exchange between NADPH and intracellular water (Wijker et al., 2019). A culture experiment by X. Zhang et al. (2009) reported that lipids showed markedly different δ2H values with two heterotrophic bacteria (C. necator and E. coli) grown on the same organic substrate, with greater fractionation for faster-growing microbes. Hydrogen isotope exchange between NADPH and water in heterotrophs is lower under higher growth rates compared to lower growth rates. However, the strength of hydrogen isotope exchange between NADPH and water depends on the turnover time of NADPH, with a longer cellular residence time for more significant hydrogen isotope exchange. Recently, an important experiment with six heterotrophic bacteria provided evidence that no significant correlation between NADPH turnover time and fractionation was observed, suggesting such exchange effects were minor (Wijker et al., 2019). By contrast, variation in fractionations in different strains growing on glucose is mainly driven by fluxes through NADP+-reducing and NADPH–NADH-balancing reactions. Thus, the observed correlations between fractionation and growth temperature in this study are likely caused by other mechanisms associated with growth rate.
The different central metabolic pathways produce NADPH with a characteristic isotope composition due to differences in the kinetic isotope effects (KIEs) accompanying NADP+ reduction by dehydrogenases and transhydrogenases (X. Zhang et al., 2009). The relative carbon flux associated with G6PDH and 6PGDH enzymes shows a negative relationship with values, while positive correlations are shown for ME (ED pathway) and ICDH (TCA cycle; Wijker et al., 2019). Only increasing carbon flux through G6PDH and 6PGDH can result in decreasing δ2H values of fatty acids. In the S. piezotolerans WP3 strain, the production of NADPH growing on GlcNac is mainly associated with three dehydrogenase enzymes including glucose-6-phosphate dehydrogenase (G6PDH), 6-phosphogluconate dehydrogenase (6PGDH), and isocitrate dehydrogenase (ICDH; Fig. 3). Under the optimal growth temperature, relatively larger isotope fractionations are most likely related to the higher fluxes of NADPH generated by the enzymes G6PDH and 6PGDH. According to the culture experiment and transcriptome data, the expression level of G6PDH and 6PGDH encoding genes at an optimal growth temperature is higher than at non-optimal temperatures (Meng et al., 2020). Given the above constraints, changes in the fluxes of NADP+ reduction by the associated enzymes G6PDH and 6PGDH can plausibly explain the variation in lipid fractionation grown at different temperatures.
The interconversion between NADPH and NADH by transhydrogenase enzymes is also regarded as a potential factor for determining the lipid hydrogen isotope composition (Wijker et al., 2019). The values displayed positive correlations with the NADPH transhydrogenase fluxes in aerobic heterotrophic bacteria, with NADPH underproduction corresponding to lower values (Wijker et al., 2019). The supply of NADPH by enzymatic reactions should be nearly balanced with the demand for NADPH in fatty-acid biosynthesis reactions (Fuhrer and Sauer, 2009). Under high growth rates, the NADPH is overproduced; thus a portion of NADPH should be converted to NADH for balancing between catabolic and anabolic fluxes (Spaans et al., 2015). This process will lead to 2H-enrichment in residual NADPH, indirectly causing 2H-enriched lipids. Therefore, if the predominant interconversion from NADPH to NADH occurs in S. piezotolerans WP3 under high growth rates (optimal growth temperature), the positive relationship between fatty-acid δ2H values and growth rates should be observed. However, the estimates are not in accordance with our measurements, indicating transhydrogenase-enzyme-associated reactions are negligible in this study. According to the above discussions, therefore, we assumed that the effect of growth temperature on the fatty-acid δ2H value is primarily related to the activity of G6PDH and 6PGDH enzymes in S. piezotolerans WP3.
4.4 Geochemical implications
We summarized previously published data (including this study) on the fractionations in aerobic heterotrophic bacteria growing on a variety of organic substrates, including sugars, pyruvate, and TCA cycle substrates (Figs. 4 and 5). On the whole, variations in fractionation are strongly correlated with the central metabolic pathways because different metabolism-associated enzymes produce NADPH with different hydrogen isotope compositions (Wijker et al., 2019). Specifically, fatty acids have average values ranging from −100 ‰ to +100 ‰ using sugars as substrates and from ‰ to +200 ‰ and +100 ‰ to +400 ‰ using pyruvate and TCA cycle substrates, respectively (Figs. 4 and 5). However, considerable variations in fractionation are observed among these investigated strains growing on the same organic substrates, and they are predominantly driven by metabolic fluxes (Wijker et al., 2019). Previous laboratory experiments showed that the average values in P. fluorescens and Rhizobia growing on fructose are about 25 % lower than those growing on glucose (Wijker et al., 2019). The ED pathway is predominant in the glucose metabolism for P. fluorescens, while the flux is only about 54 % through the ED pathway growing on fructose. The metabolic flux analysis can also excellently interpret the fractionations in E. coli growing on gluconate and glucose (X. Zhang et al., 2009). For heterotrophs growing on sugars, B. subtilis and E. coli exhibit the most 2H-depleted fatty acids, while two species (P. fluorescens and Pseudomonas str. LFY10) are slightly enriched in 2H composition (Fig. 4). This is similar to the flux data, according to which B. subtilis and E. coli strongly rely on the EMP pathway for glucose catabolism, while the TCA cycle is predominant in P. fluorescens and Pseudomonas str. LFY10 (Heinzelmann et al., 2015b). For S. piezotolerans WP3 growing on glucose and GlcNac, the average values are −67 ‰ and −76 ‰, respectively, and they are higher than B. subtilis and E. coli but lower than P. fluorescens and Pseudomonas str. LFY10 (Fig. 4). We speculate that the metabolic flux of the TCA cycle in S. piezotolerans WP3 may vary between E. coli and P. fluorescens.
It is interesting to note that aerobic heterotrophs growing on TCA cycle substrates (acetate, succinate, citrate, and benzoic acid) exhibit the largest variations in values (from ‰–400 ‰). Heterotrophs including P. fluorescens and Pseudomonas also have relatively higher values compared with other investigated strains (Fig. 5). The NADPH is predominately produced through the TCA cycle, which results in strong 2H enrichment of NADPH. Moreover, overproduction of NADPH is generally present in heterotrophic bacteria (e.g., E. coli) growing on the direct precursors or intermediates of the TCA cycle (Gerosa et al., 2015; Wijker et al., 2019). The most important thing is that a proportion of NADPH is firstly used as an H− donor for amino acid and protein synthesis, causing residual NADPH enriched in 2H (Fig. 3). Both of these two processes can yield fatty acids with strong 2H enrichment, and the metabolism and range of NADPH overproduction may be responsible for the different values in these species.
Up until now, the hydrogen isotope composition of lipids has been successfully used to infer the metabolism of environmental organisms in a terrestrial hydrothermal environment (Naraoka et al., 2010; Osburn et al., 2011), Antarctic lacustrine sediments (Chen et al., 2021), and marine particulate organic matter (Jones et al., 2008; Heinzelmann et al., 2016). For example, short-chain n-alkanoic acids (C16 and C18) in Antarctic pond sediments have average values ranging from about −150 ‰ to −100 ‰, while mid- and long-chain n-alkanoic acids (C22–C30) vary from ∼0 ‰ to +300 ‰ (Chen et al., 2021). These results indicate that mid- and long-chain compounds originated from heterotrophic microbes, and large variations in values are strongly related to the central metabolic pathway of heterotrophs. Similarly, heterotroph-associated branched fatty acids (e.g., aC15 and iC15) in marine particulate matter and sediments have relatively higher δ2H values than that C20 : 5 n-alkanoic acid from phototrophic algae, and variations in these lipids reflect the community metabolism (Heinzelmann et al., 2016, 2018).
Compound-specific isotope measurements can provide further insights into the biogeochemical cycle and ecological change in deep oceans. Many previous studies reported that C20 : 5 fatty acid can be used as the specific lipids for phytoplankton (Heinzelmann et al., 2016). However, our data showed that S. piezotolerans WP3 can also produce a certain amount of C20 : 5 fatty acid (Wang et al., 2009; Chen et al., 2022). Fatty acids produced from phototrophs have relative larger hydrogen and carbon isotope fractionations with and values ranging from about −200 ‰ to −150 ‰ and about −28 ‰, respectively (Jones et al., 2008; Li et al., 2009; Guan et al., 2019). By contrast, heterotrophs obviously have different fractionations, with and values from about −100 ‰–100 ‰ and −10 ‰–4 ‰. Among heterotrophs, the and are characterized by different values assimilating different types of organic matter (Fig. 6). The carbon and hydrogen isotope compositions of these specific biomarker lipids are a promising tool to infer the community metabolisms and to then decode carbon cycling processes in Fe-reducing oceanic sediments.
Our results showed that the hydrogen isotope composition of individual fatty acids in S. piezotolerans WP3 varies considerably in the same culture. The branched fatty acids and polyunsaturated fatty acid (C20 : 5) have the lowest δ2H values among these compounds, indicating that different biosynthesis pathways have an obvious influence on the δ2H values of different-chain-length fatty acids. The hydrogen isotope fractionations between fatty acids and growth water vary systematically by the type of central carbon metabolic pathway. Specifically, WP3 growing on GlcNac and glucose produce NADPH through pentose phosphate and glycolysis pathways; fatty acids are most depleted in 2H. Fatty acids are slightly enriched in 2H using pyruvate and L-alanine as the sole carbon source and strongly 2H-enriched using TCA cycle substrates (acetate and L-glutamate) because NADP+ is produced through the TCA cycle pathway. The most plausible reasons are that hydrogen isotope fractionations in aerobic heterotrophs are highly correlated to the type of central metabolic pathways due to different enzymes accompanying NADP+ reduction.
We also observed large variations in fractionation in WP3 using GlcNac as the sole carbon source at different temperatures. Fatty acids have relatively lower values at optimal temperatures (−3 ± 3 ‰ and 9 ‰ at 15 and 20 ∘C, respectively), while there are higher values at 4 ∘C (24 ± 5 ‰), 10 ∘C (15 ± 12 ‰), and 25 ∘C (35 ± 41 ‰). We assumed that the influence of growth temperature on the δ2H values of fatty acids is most likely related to the activity of G6PDH and 6PGDH enzymes.
All the data generated in this study are included in Tables 1 and 2 and Figs. 1 and 2 in the text.
The supplement related to this article is available online at: https://doi.org/10.5194/bg-20-1491-2023-supplement.
FW and XC proposed the topic and conceived and designed the study. XC conducted the experiments and prepared the paper with contributions from all co-authors. All the co-authors contributed to the discussion and edited and commented on the paper.
The contact author has declared that none of the authors has any competing interests.
Publisher's note: Copernicus Publications remains neutral with regard to jurisdictional claims in published maps and institutional affiliations.
We thank Yunru Chen and Haining Hu, who provided valuable assistance with laboratory experiments. We are grateful to Denise Akob and two anonymous reviewers, whose comments significantly improved the quality of the paper.
This study was supported by the National Natural Science Foundation of China (grant nos. 42206243, 41921006, and 42141003), the Shanghai Sailing Program (grant no. 22YF1418800), the China Postdoctoral Science Foundation (grant no. 2022M712038), the Shanghai Frontiers Science Center of Polar Science (SCOPS), the Shanghai Pilot Program for Basic Research – Shanghai Jiao Tong University (grant no. 21TQ1400201), and the National Key Research and Development Program of China (grant no. 2020YFA0608300).
This paper was edited by Denise Akob and reviewed by two anonymous referees.
Chen, X., Liu, X., Jia, H., Jin, J., Kong, W., and Huang, Y.: Inverse hydrogen isotope fractionation indicates heterotrophic microbial production of long-chain n-alkyl lipids in desolate Antarctic ponds, Geobiology, 19, 394–404, 2021.
Chen, X., Dong, L., Zhao, W., Jian, H., Wang, J., and Wang, F.: The effects of metabolism and temperature on carbon isotope composition of lipids in marine bacterium Shewanella piezotolerans WP3, Chem. Geol., 606, 120963, https://doi.org/10.1016/j.chemgeo.2022.120963, 2022.
Dirghangi, S. S. and Pagani, M.: Hydrogen isotope fractionation during lipid biosynthesis by Haloarcula marismortui, Geochim. Cosmochim. Ac., 119, 381–390, 2013a.
Dirghangi, S. S. and Pagani, M.: Hydrogen isotope fractionation during lipid biosynthesis by Tetrahymena thermophila, Org. Geochem., 64, 105–111, 2013b.
Dufault-Thompson, K., Jian, H., Cheng, R., Li, J., Wang, F., and Zhang, Y.: A genome-scale model of Shewanella piezotolerans simulates mechanisms of metabolic diversity and energy conservation, Msystems, 2, e00165–00116, 2017.
Fang, J., Li, C., Zhang, L., Davis, T., Kato, C., and Bartlett, D. H.: Hydrogen isotope fractionation in lipid biosynthesis by the piezophilic bacterium Moritella japonica DSK1, Chem. Geol., 367, 34–38, 2014.
Fredrickson, J. K., Romine, M. F., Beliaev, A. S., Auchtung, J. M., Driscoll, M. E., Gardner, T. S., Nealson, K. H., Osterman, A. L., Pinchuk, G., and Reed, J. L.: Towards environmental systems biology of Shewanella, Nat. Rev. Microbiol., 6, 592–603, 2008.
Fuhrer, T. and Sauer, U.: Different biochemical mechanisms ensure network-wide balancing of reducing equivalents in microbial metabolism, J. Bacteriol., 191, 2112–2121, 2009.
Gerosa, L., van Rijsewijk, B. R. H., Christodoulou, D., Kochanowski, K., Schmidt, T. S., Noor, E., and Sauer, U.: Pseudo-transition analysis identifies the key regulators of dynamic metabolic adaptations from steady-state data, Cell Systems, 1, 270–282, 2015.
Guan, H., Chen, L., Luo, M., Liu, L., Mao, S., Ge, H., Zhang, M., Fang, J., and Chen, D.: Composition and origin of lipid biomarkers in the surface sediments from the southern Challenger Deep, Mariana Trench, Geosci. Front., 10, 351–360, 2019.
Hau, H. H. and Gralnick, J. A.: Ecology and biotechnology of the genus Shewanella, Annu. Rev. Microbiol., 61, 237–258, 2007.
Hayes, J. M.: Fractionation of Carbon and Hydrogen Isotopes in Biosynthetic Processes, Rev. Mineral. Geochem., 43, 225–277, 2001.
Heinzelmann, S. M., Chivall, D., M'Boule, D., Sinke-Schoen, D., Villanueva, L., Damsté, J. S. S., Schouten, S., Van der Meer, M. T., and Oren, A.: Comparison of the effect of salinity on the ratio of fatty acids of heterotrophic and photoautotrophic microorganisms, FEMS Microbiol. Lett., 362, 1–6, 2015a.
Heinzelmann, S. M., Villanueva, L., Sinke-Schoen, D., Sinninghe Damsté, J. S., Schouten, S., and Van der Meer, M. T.: Impact of metabolism and growth phase on the hydrogen isotopic composition of microbial fatty acids, Front. Microbiol., 6, 408, 2015b.
Heinzelmann, S. M., Bale, N. J., Villanueva, L., Sinke-Schoen, D., Philippart, C. J. M., Sinninghe Damsté, J. S., Schouten, S., and van der Meer, M. T. J.: Seasonal changes in the ratio of fatty acids of pelagic microorganisms in the coastal North Sea, Biogeosciences, 13, 5527–5539, https://doi.org/10.5194/bg-13-5527-2016, 2016.
Heinzelmann, S. M., Villanueva, L., Lipsewers, Y. A., Sinke-Schoen, D., Damste, J. S. S., Schouten, S., and van der Meer, M. T.: Assessing the metabolism of sedimentary microbial communities using the hydrogen isotopic composition of fatty acids, Org. Geochem., 124, 123–132, 2018.
Huang, X. and Meyers, P. A.: Assessing paleohydrologic controls on the hydrogen isotope compositions of leaf wax n-alkanes in Chinese peat deposits, Palaeogeogr. Palaeocl., 516, 354–363, 2019.
Huang, Y., Shuman, B., Wang, Y., and Webb, T.: Hydrogen isotope ratios of individual lipids in lake sediments as novel tracers of climatic and environmental change: a surface sediment test, J. Paleolimnol., 31, 363–375, 2004.
Jackson, J. B., Peake, S. J., and White, S. A.: Structure and mechanism of proton-translocating transhydrogenase, FEBS Lett., 464, 1–8, 1999.
Jones, A. A., Sessions, A. L., Campbell, B. J., Li, C., and Valentine, D. L.: ratios of fatty acids from marine particulate organic matter in the California Borderland Basins, Org. Geochem., 39, 485–500, 2008.
Kreuzer-Martin, H. W., Lott, M. J., Ehleringer, J. R., and Hegg, E. L.: Metabolic processes account for the majority of the intracellular water in log-phase Escherichia coli cells as revealed by hydrogen isotopes, Biochemistry, 45, 13622–13630, 2006.
Lemaire, O. N., Méjean, V., and Chantal, I. N.: The shewanella genus: ubiquitous organisms sustaining and preserving aquatic ecosystems, FEMS Microbiol. Rev., 44, 155–170, 2020.
Li, C., Sessions, A. L., Kinnaman, F. S., and Valentine, D. L.: Hydrogen-isotopic variability in lipids from Santa Barbara Basin sediments, Geochim. Cosmochim. Ac., 73, 4803–4823, 2009.
Meng, C., Li, S., Fan, Q., Chen, R., Hu, Y., Xiao, X., and Jian, H.: The thermo-regulated genetic switch of deep-sea filamentous phage SW1 and its distribution in the Pacific Ocean, FEMS Microbiol. Lett., 367, fnaa094, https://doi.org/10.1093/femsle/fnaa094, 2020.
Moran, M. A., Kujawinski, E. B., Schroer, W. F., Amin, S. A., Bates, N. R., Bertrand, E. M., Braakman, R., Brown, C. T., Covert, M. W., and Doney, S. C.: Microbial metabolites in the marine carbon cycle, Nat. Microbiol., 7, 508–523, 2022.
Naraoka, H., Uehara, T., Hanada, S., and Kakegawa, T.: δ13C–δD distribution of lipid biomarkers in a bacterial mat from a hot spring in Miyagi Prefecture, NE Japan, Org. Geochem., 41, 398–403, 2010.
Osburn, M. R., Sessions, A. L., Pepe-Ranney, C., and Spear, J. R.: Hydrogen-isotopic variability in fatty acids from Yellowstone National Park hot spring microbial communities, Geochim. Cosmochim. Ac., 75, 4830–4845, 2011.
Osburn, M. R., Dawson, K. S., Fogel, M. L., and Sessions, A. L.: Fractionation of hydrogen isotopes by sulfate-and nitrate-reducing bacteria, Front. Microbiol., 7, 1166, 2016.
Rodríguez-Ruiz, J., Belarbi, E.-H., Sanchez, J. L. G., and Alonso, D. L.: Rapid simultaneous lipid extraction and transesterification for fatty acid analyses, Biotechnol. Tech., 12, 689–691, 1998.
Sachse, D., Billault, I., Bowen, G. J., Chikaraishi, Y., Dawson, T. E., Feakins, S. J., Freeman, K. H., Magill, C. R., McInerney, F. A., and Van Der Meer, M. T.: Molecular paleohydrology: interpreting the hydrogen-isotopic composition of lipid biomarkers from photosynthesizing organisms, Annu. Rev. Earth Pl. Sc., 40, 221–249, 2012.
Schouten, S., Ossebaar, J., Schreiber, K., Kienhuis, M. V. M., Langer, G., Benthien, A., and Bijma, J.: The effect of temperature, salinity and growth rate on the stable hydrogen isotopic composition of long chain alkenones produced by Emiliania huxleyi and Gephyrocapsa oceanica, Biogeosciences, 3, 113–119, https://doi.org/10.5194/bg-3-113-2006, 2006.
Sessions, A. L.: Factors controlling the deuterium contents of sedimentary hydrocarbons, Org. Geochem., 96, 43–64, 2016.
Sessions, A. L., Burgoyne, T. W., Schimmelmann, A., and Hayes, J. M.: Fractionation of hydrogen isotopes in lipid biosynthesis, Org. Geochem., 30, 1193–1200, 1999.
Spaans, S. K., Weusthuis, R. A., Van Der Oost, J., and Kengen, S. W.: NADPH-generating systems in bacteria and archaea, Front. Microbiol., 6, 742, 2015.
Wang, F., Wang, P., Chen, M., and Xiao, X.: Isolation of extremophiles with the detection and retrieval of Shewanella strains in deep-sea sediments from the west Pacific, Extremophiles, 8, 165–168, 2004.
Wang, F., Wang, J., Jian, H., Zhang, B., Li, S., Wang, F., Zeng, X., Gao, L., Bartlett, D. H., and Yu, J.: Environmental adaptation: genomic analysis of the piezotolerant and psychrotolerant deep-sea iron reducing bacterium Shewanella piezotolerans WP3, PLoS one, 3, e1937, https://doi.org/10.1371/journal.pone.0001937, 2008.
Wang, F., Xiao, X., Ou, H.-Y., Gai, Y., and Wang, F.: Role and regulation of fatty acid biosynthesis in the response of Shewanella piezotolerans WP3 to different temperatures and pressures, J. Bacteriol., 191, 2574–2584, 2009.
White, S. W., Zheng, J., Zhang, Y.-M., and Rock, C. O.: The structural biology of type II fatty acid biosynthesis, Annu. Rev. Biochem., 74, 791–831, 2005.
Wijker, R. S., Sessions, A. L., Fuhrer, T., and Phan, M.: variation in microbial lipids is controlled by NADPH metabolism, P. Natl. Acad. Sci. USA, 116, 12173–12182, 2019.
Xiao, X., Wang, P., Zeng, X., Bartlett, D. H., and Wang, F.: Shewanella psychrophila sp. nov. and Shewanella piezotolerans sp. nov., isolated from west Pacific deep-sea sediment, Int. J. Syst. Evol. Micr., 57, 60–65, 2007.
Zhang, X., Gillespie, A. L., and Sessions, A. L.: Large variations in bacterial lipids reflect central metabolic pathways, P. Natl. Acad. Sci. USA, 106, 12580–12586, 2009.
Zhang, Z., Sachs, J. P., and Marchetti, A.: Hydrogen isotope fractionation in freshwater and marine algae: II. Temperature and nitrogen limited growth rate effects, Org. Geochem., 40, 428–439, 2009.
Zhao, W., Fang, J., Huang, X., Zhang, Y., Liu, W., Wang, Y., and Zhang, L.: Carbon and hydrogen isotope fractionation in lipid biosynthesis by Sporosarcina sp. DSK25, Geochemical Perspectives Letters, 14, 9–13, 2020.