the Creative Commons Attribution 4.0 License.
the Creative Commons Attribution 4.0 License.
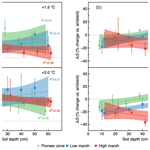
Warming accelerates belowground litter turnover in salt marshes – insights from a Tea Bag Index study
Stefanie Nolte
Kai Jensen
Roy Rich
Julian Mittmann-Goetsch
Peter Mueller
Salt marshes play an important role in the global carbon cycle due to the large amount of organic carbon stored in their soils. Soil organic carbon formation in these coastal wetland ecosystems is strongly controlled by the plant primary production and initial decomposition rates of plant belowground biomass and litter. This study used a field warming experiment to investigate the response of belowground litter breakdown to rising temperature (+1.5 and +3.0 ∘C) across whole-soil profiles (0–60 cm soil depth) and the entire intertidal flooding gradient ranging from the pioneer zone via the low marsh to high marsh. We used standardized plant materials, following the Tea Bag Index approach, to assess the initial decomposition rate (k) and the stabilization factor (S) of labile organic matter inputs to the soil system. While k describes the initial pace at which labile (= hydrolyzable) organic matter decomposes, S describes the part of the labile fraction that does not decompose during deployment in the soil system and stabilizes due to biochemical transformation. We show that warming strongly increased k consistently throughout the entire soil profile and across the entire flooding gradient, suggesting that warming effects on the initial decomposition rate of labile plant materials are independent of the soil aeration (i.e., redox) status. By contrast, negative effects on litter stabilization were less consistent. Specifically, warming effects on S were restricted to the aerated topsoil in the frequently flooded pioneer zone, while the soil depth to which stabilization responded increased across the marsh elevation gradient via the low to high marsh. These findings suggest that reducing soil conditions can suppress the response of belowground litter stabilization to rising temperature. In conclusion, our study demonstrates marked differences in the response of initial decomposition rate vs. stabilization of labile plant litter to rising temperature in salt marshes. We argue that these differences are strongly mediated by the soil redox status along flooding and soil-depth gradients.
- Article
(3591 KB) - Full-text XML
-
Supplement
(535 KB) - BibTeX
- EndNote
Salt marshes provide a multitude of ecosystem services, such as wildlife conservation, flood protection, and water-quality improvement (Barbier et al., 2011; Kirwan and Megonigal, 2013). Recently, salt marshes have additionally been recognized for their ability to store large amounts of organic carbon in their soils, which has been acknowledged by the common use of the term blue carbon (Chmura, 2013; McLeod et al., 2011). Global warming yields the potential to influence carbon sequestration in salt marshes by affecting the balance between organic matter (OM) input to the soil system, through plant primary production, and output, through microbial decomposition of plant OM inputs (Kirwan and Mudd, 2012). Most of the current debate regarding the temperature sensitivity of the salt marsh carbon balance is dealing with aboveground processes, i.e., plant primary production (Kirwan and Blum, 2011; Noyce et al., 2019) and aboveground or surface litter breakdown (Charles and Dukes, 2009; Kirwan and Blum, 2011), whereas the effects on belowground processes remain largely unexplored (but see Mueller et al., 2018; Noyce et al., 2019). Belowground litter input and turnover often are more important drivers of soil carbon sequestration than aboveground processes because aboveground litter gets deposited in an oxic environment and is subject to fast decomposition, whereas belowground litter can get stabilized under reducing soil conditions (Kirwan and Megonigal, 2013; Langley and Megonigal, 2010; Mueller et al., 2018).
Litter decomposition dynamics are commonly quantified using litter bags, which are mesh bags filled with native plant litter of variable quality (e.g., with respect to C:N ratio or labile vs. recalcitrant fractions) that get deployed in the ecosystem or soil system in question. Initial decomposition rates are calculated based on litter mass loss over time. However, the mechanistic insight into climate change effects that can be gained from it is often limited owing to the challenge of separating climate from litter-quality effects (Prescott, 2010). Litter decomposition is controlled by complex interactions between litter quality and climate parameters (Zhang et al., 2008). To separately assess climate effects on litter decomposition, it has proven useful to standardize litter quality. The Tea Bag Index (TBI) sensu Keuskamp et al. (2013) represents a widely used standardized litter bag approach. It allows for the quantification of two key litter breakdown parameters: the initial decomposition rate, k, and the stabilization factor, S.
Warming effects on litter breakdown in coastal wetlands may be strongly controlled by hydrology and resulting soil redox gradients. For instance, warming studies conducted in a boreal peatland demonstrated a dramatic reduction of warming effects on soil decomposition processes in waterlogged and thus strongly reducing subsoils compared to less reducing topsoils (Hopple et al., 2020; Wilson et al., 2016). The authors of these peatland warming studies suggest that the absence of oxygen can inhibit warming effects on soil microbial activity because phenolic compounds accumulate under anoxic conditions and inhibit microbial hydrolytic enzyme activity via the “enzymic latch” mechanism (Freeman et al., 2001). In order to understand warming effects on litter breakdown in coastal wetland ecosystems, it is therefore necessary to use a standard substrate to study temperature effects across gradients in both flooding frequency (i.e., along the marsh elevation gradient) and in relation to soil depth.
The present study uses a unique field experiment to assess the effects of rising temperatures on litter breakdown in relation to soil depth and across the marsh elevation gradient ranging from the pioneer zone via the low marsh to high marsh. The Marsh Ecosystem Response to Increased Temperature (MERIT) experiment operates in a NW European salt marsh and induces active temperature manipulation to 1 m soil depth. We investigated litter breakdown for two consecutive growing seasons during the growing season of year 1 and year 2 of the experiment. We hypothesized that (1) warming will increase the initial litter decomposition rate, k, and decrease the ability of litter to stabilize, S, in soil, and (2) warming effects on k and S will be inhibited by reducing soil conditions and thus vary along gradients of both flooding frequency and soil depth.
2.1 Site description
The MERIT ecosystem warming experiment is located in a NW European salt marsh at Hamburger Hallig, Germany (54∘36′06.2′′ N, 8∘49′00.1′′ E), and has operated since spring 2018. The site is located on the coast of the Schleswig-Holstein Wadden Sea (Fig. 1a and c). The site is exposed to a tidal range of about 3 m, with floodwater salinity ranging from 25 to 29 ppt (Mueller et al., 2023). Mean annual temperature is 10 ∘C, and mean annual precipitation is 850 mm. The vegetation shows a zonation typical for Wadden Sea salt marshes: Spartina anglica is dominant in the pioneer zone, Atriplex portulacoides and Puccinellia maritima are dominant in the low marsh, and Elymus athericus is dominant in the high marsh (Esselink, 2017; Mueller et al., 2020a). The mean elevations of the pioneer zone, low marsh, and high marsh are 136, 174, and 212 cm (NHN, German standard ordnance datum), respectively. The pioneer zone is a typical feature of NW European salt marshes and is defined as the area where pioneer vegetation covers ≥ 5 % (Peterson et al., 2014). In the Wadden Sea region its average surface elevation is below mean high tide. Thus, the pioneer zone is typically flooded twice daily (Esselink, 2017). Soil pH (measured in CaCl2) at the site ranges between 7.0 and 7.5 without pronounced differences between zones (Mueller et al., 2023). Both organic and inorganic carbon stocks increase from the pioneer zone to the high marsh (Mueller et al., 2023). 13C signatures of the soil organic matter demonstrate a strong decrease in allochthonous marine-derived vs. autochthonous vascular plant-derived organic contributions along the flooding gradient from the pioneer zone to the high marsh (Mueller et al., 2023).
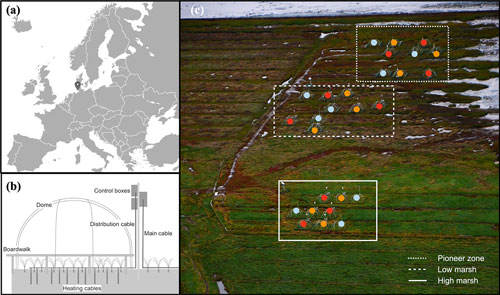
Figure 1Location of the MERIT experimental site (a); diagram of belowground and aboveground heating (b); and plot distribution in the pioneer zone, low marsh, and high marsh (c), aerial photo of the experimental site, courtesy of Norbert Kempf. Color points, i.e., blue, orange, and red, accordingly present the ambient, +1.5, and +3 ∘C treatments in different plots and marsh zones.
2.2 Experimental design
MERIT consists of N=27 experimental plots, each with an area of approx. 7 m2. Belowground active temperature manipulation is conducted using 31 vertical heating cables (GX 088L3100, 9.8Ω m−1, Danfoss, Denmark) per plot, inserted into the ground to 1.0 m soil depth. Aboveground and soil-surface temperature manipulation is achieved using a combination of passive open-top chambers and horizontal heating cables with a length of 52 m per plot deployed at the soil surface (GX 088L3100, 9.8Ω m−1, Danfoss, Denmark) (Fig. 1b). The experiment is conducted across three hydrological zones (pioneer zone, low marsh, and high marsh) and applies three temperature treatments (ambient, +1.5, and +3 ∘C) in a full factorial design (3 zones × 3 temperature treatments × 3 replicates) (Fig. 1c).
Belowground temperature was monitored continuously and logged at 5 min intervals using custom-made thermistors and dataloggers. To control the heating rate evenly throughout the soil profile, sensors were placed at −5, −25, and −75 cm depth below the soil surface. At −5 cm, the highest variation in mean temperature difference across all marsh zones and plots ranged from 1.43 to 1.67 ∘C for the +1.5 treatment and 2.54 to 2.99 ∘C for the +3.0 treatment. At −25 cm depth, the mean temperature-difference values ranged from 1.51 to 1.55 ∘C for the +1.5 ∘C treatment and 2.87 to 3.02 ∘C for the +3.0 ∘C treatment. At −75 cm, temperature-difference values ranged from 1.14 to 1.43 ∘C for the +1.5 ∘C treatment and 1.92 to 2.36 ∘C for the +3.0 ∘C treatment (Rich et al., 2023). We selected the belowground temperature data from −10 to −60 cm of the deployment times in 2018 and 2019 to calculate the average belowground ambient temperature per zone. Mean ambient temperature during the deployment time in 2018 (pioneer zone = 16.45 ∘C, low marsh = 15.99 ∘C, high marsh = 14.54 ∘C) was higher compared to 2019 (pioneer zone = 13.55 ∘C, low marsh = 12.95 ∘C, high marsh = 12.46 ∘C).
2.3 Decomposition of standardized plant litter
The initial decomposition rate (k) and stabilization factor (S) were assessed following the Tea Bag Index (TBI) protocol (Keuskamp et al. 2013). k describes the rate at which the labile (here defined as hydrolyzable) fraction of the plant material decomposes. S describes the part of the labile fraction that did not decompose during deployment in the soil system and stabilized. Twelve polypropylene tea bags, six green tea bags (EAN: 8714100770542; Lipton, Unilever), and six rooibos tea bags (EAN: 8722700188438; Lipton, Unilever) were put into the salt marsh soil using two solid PVC posts per plot, perforated with six holes between 10 and 60 cm soil depth (Fig. 2). Posts contained either green or rooibos tea and were placed at a distance of 20 cm to each other (Fig. 2). The initial weight of the tea bag content was determined by subtracting the mean content weight of five empty bags from the total tea bag weight (green tea: 1.592 ± 0.004 g, rooibos tea: 1.801 ± 0.006 g). Tea bags were deployed in two consecutive growing seasons. In 2018 (year 1 of the experiment), tea bags were deployed from 19 June to 20 September (= 93 d). In 2019 (year 2), tea bags were deployed from 14 May to 17 July (= 93 d). Following deployment, tea bags were removed from the soil, and the tea material was carefully separated from roots and soil, dried for 48 h at 70 ∘C, and weighed. The calculation of k and S followed Mueller et al. (2018), who used a different extraction protocol than the original Keuskamp et al. (2013) publication, yielding slightly higher values for the hydrolyzable (= labile) fractions of green and rooibos tea.
Wr(t) refers to the weight of the rooibos substrate after the incubation time (t in days), ar refers to the labile fraction of the rooibos substrate, and 1−ar refers to the recalcitrant fraction of the rooibos substrate; k is the initial decomposition rate, S is the stabilization factor, ag represents the labile fraction of the green tea substrate, and Hg represents the hydrolyzable fraction of the green tea substrate. The labile fraction of the rooibos substrate is calculated in Eq. (3) based on the hydrolyzable fraction of the rooibos tea substrate (Hr) and S. Hg and Hr values are taken from Tang et al. (2021) because the same tea materials were used.
2.4 Characterization of soil redox conditions
A soil reduction index was determined based on the Indicator of Reduction in Soils technique (IRIS; sensu Jenkinson, 2002; Mueller et al., 2020b; Rabenhorst, 2015). FeCl3-coated PVC sticks were inserted to a soil depth of 30 cm in three zones along the marsh elevation gradient. There were 6 sticks per zone (n = 6) and 18 (N = 6 × 3) sticks per campaign; in total N = 72 (n = 4 campaigns × 18) sticks were analyzed. These measurements were conducted along a transect directly adjacent to the experimental plots. The reduction index describes the fraction of FeCl3 paint that is removed from the PVC stick after 4 weeks of deployment in the field. The IRIS method utilizes the property of the ferrihydrite paint to be reduced from solid-phase Fe(III) to soluble Fe(II) under anoxic soil conditions and in the presence of microbial Fe reducers. The area of removed paint from PVC sticks is used as a proxy for soil reduction (reduction index). Upon the 4-week deployment phase, sticks were removed from the soil and cleaned carefully with tap water to remove soil particles. Each stick was scanned to create digital images for further processing. Image analysis was conducted by applying a supervised classification using the software ArcGIS Pro. RGB color values (0–255) of 4300 randomly set points on a test set of sticks were determined. Points were classified as either reduced, not reduced, or errors (background, scanning effects). The classification was included in a random forest model (confusion matrix 1.5 %) using the software R. This model allowed for a pixel-wise classification of the scanned IRIS sticks. Sticks were analyzed in increments of 5 cm, covering a depth gradient from 0 to 30 cm. The reduction index was calculated as a unitless value ranging from 0 to 1 based on the share of reduced pixels from the total pixels.
2.5 Statistical analyses
Two-way repeated-measures ANOVAs were used to test for the effects of warming (ambient, +1.5, and +3 ∘C), ecosystem zone (pioneer zone, low marsh, and high marsh), and soil depth (within subject/repeated measure) on TBI parameters for each year separately. Because we were not primarily interested in year-to-year differences, ANOVAs were used separately for each year to better understand the interactions between warming, zone, and soil depth. Pairwise comparisons were performed using Tukey's tests. The normal distribution of residuals and homogeneity of variance were assessed visually and met ANOVA assumptions.
The temperature-induced change in k and S was calculated relative to the ambient controls (k0 and S0) for each marsh zone separately: Δk(%) = () × 100 and ΔS(%) = () × 100, where t represents the value under different warming treatments (i.e., +1.5 and +3.0 ∘C). Linear regression was used to analyze the relationships of Δk and ΔS with soil depth.
These analyses were conducted using the statistical software STATISTICA, version 12 (StatSoft Inc, Tulsa, Oklahoma, USA).
Table 1Results of two-way repeated-measures ANOVA testing for the effects of warming (W), marsh zone (Z), soil depth (D), and their interactions on TBI parameters (k is initial decomposition rate and S the stabilization factor) in year 1 (2018) and year 2 (2019) of the experiment. Significant effects (p≤0.05) are shown in bold.
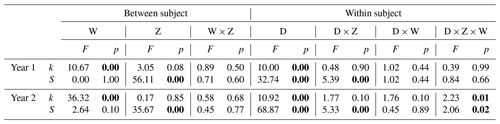
3.1 Decomposition and stabilization dynamics
The initial decomposition rate (k) was increased by warming in both year 1 and year 2 of the experiment (Figs. 3 and 4). The effect of the +3.0 ∘C treatment (+158.6 % in year 1, +234.6 % in year 2) was more pronounced than that of the +1.5 ∘C treatment (+162.3 % in year 1, +170.39 % in year 2). Overall, positive warming effects on k appear to be consistent across marsh zones and soil depths (Figs. 3a–c and 4a–c), despite a significant depth × zone × warming interaction in year 2, as indicated by ANOVA (p<0.01, Table 1). We ascribe this significant three-way interaction term to inconsistent depth trends of the warming responses between the marsh zones (Fig. 4a–c). That is, the magnitude of the +3.0 ∘C warming effect on k decreases linearly with increasing soil depth in the pioneer zone and high marsh, whereas the warming effect in the low marsh was maximized at an intermediate soil depth (Fig. 4a–c).
The stabilization factor (S) was not affected by the warming treatment as a single factor but by the depth × zone × warming interaction in year 2 of the experiment (Table 1). Negative effects of warming on S were pronounced throughout the whole soil in the high marsh (on average −13.6 % at +3.0 ∘C, −8.6 % at +1.5 ∘C) but were restricted to the upper soil layers in the pioneer zone and low marsh (Fig. 4d–f). The soil depth to which S responded to warming treatments increased across the marsh elevation gradient from the pioneer zone via the low to high marsh (Fig. 4). While the magnitude of negative warming effects decreased with soil depth in both the pioneer zone and low marsh, the opposite was true for the high marsh (Fig. 5).
3.2 Soil redox conditions
Pronounced soil redox gradients both with respect to soil depth and flooding frequency exist at the study site (Fig. 6). Reducing soil conditions markedly increase with soil depth and along the flooding gradient from the high marsh to pioneer zone. In the frequently flooded pioneer zone, reducing soil conditions are reached closer to the soil surface than in the high marsh (Fig. 6).
4.1 Warming effects on litter breakdown
The initial decomposition rate (k) was strongly increased by warming across all marsh zones in 2 consecutive years (Table 1). By contrast, warming effects on stabilization (S) were less consistent and only present in year 2. This finding agrees with a large number of studies, from a range of ecosystems, demonstrating that k and S can be decoupled rather than strictly inversely related (Elumeeva et al., 2018; Fanin et al., 2020; Mori et al., 2022; Ochoa-Hueso et al., 2020; Sarneel et al., 2020; Sarneel and Veen, 2017; Tang et al., 2020).
Our results suggest that the decoupling of warming responses in k and S is controlled by hydrology or – more specifically – the soil redox status. Soil depth and marsh zone had no effects on k (two-way ANOVA, p>0.1), which shows that the initial decomposition rate of labile plant inputs is not influenced by hydrology or the soil redox status. This finding agrees with previous studies demonstrating the effects of oxygen availability on OM decomposition depends on OM quality and that labile materials decompose at similar rates in oxic and anoxic environments (Benner et al., 1984; Kristensen et al., 1995). By contrast, S increased with flooding frequency (i.e., from the high marsh to pioneer zone) and with soil depth, indicating that the stabilization of labile materials does depend on the soil redox status. Flooding and soil depth were also the primary constraints of warming effects on S. Specifically, warming effects on S were restricted to the topsoil in the pioneer zone, but the soil depth to which S responded to warming treatments increased across the marsh elevation gradient via the low to high marsh (Fig. 4d–f).
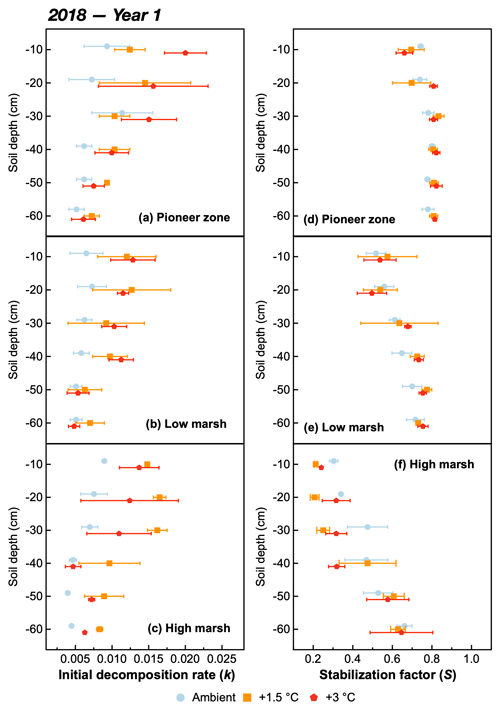
Figure 3The initial decomposition rate (k) (a, b, c) and stabilization factor (S) (d, e, f) at different soil depths of the pioneer, low-marsh, and high-marsh zones under three temperature treatments (ambient, +1.5, and +3 ∘C) in year 1 (2018). Values are means ± SE (n=3).
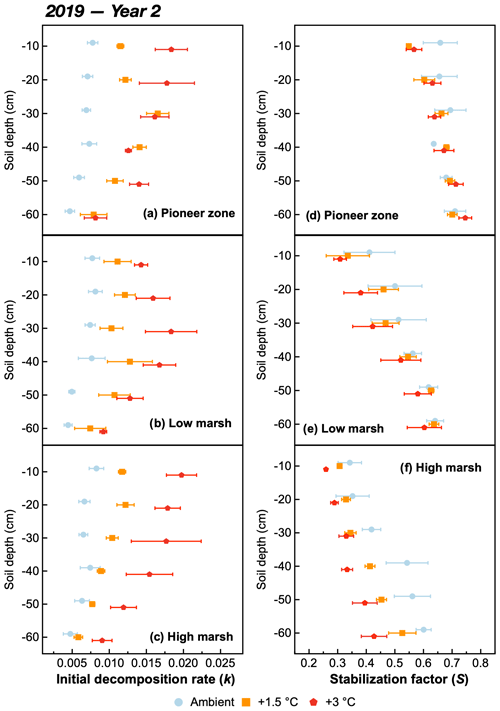
Figure 4The initial decomposition rate (a, b, c) and stabilization factor (d, e, f) at different soil depths of the pioneer, low-marsh, and high-marsh zones under three temperature treatments (ambient, +1.5, and +3 ∘C) in year 2 (2019). Values are means ± SE (n=3).
The here observed redox constraints on warming effects resemble the findings of the Spruce and Peatland Responses Under Changing Environments (SPRUCE) warming experiment operating in a boreal peatland ecosystem in Minnesota, USA. Research in SPRUCE demonstrated a dramatic reduction of warming effects on soil OM decomposition in strongly reducing waterlogged subsoils compared to less reducing topsoils (Hopple et al., 2020; Wilson et al., 2016). It is possible that oxygen constraints on phenol-oxidase activity, following the enzymic-latch hypothesis (Freeman et al., 2001), are responsible for the redox control of warming effects on both the labile OM stabilization observed in our study and soil OM decomposition observed in SPRUCE. The enzymic-latch hypothesis states that phenolic substances accumulate under anoxic conditions, as phenol-oxidase activity requires oxygen, and inhibit the activity of hydrolases responsible for the breakdown of the majority of organic compounds supplied to the soil system. For blue carbon ecosystems, the phenolic-driven reduction of OM decomposition has recently been confirmed for the breakdown of sucrose in seagrass sediments (Sogin et al., 2022), suggesting that even the breakdown of short-chained and labile sugars can be affected by the enzymic latch. The application of the enzymic-latch hypothesis to the findings of the present study is not straightforward, because it is unclear how an accumulation of phenolics could increase the stabilization of labile OM, as well as mitigate warming effects on this process, without decreasing the initial decomposition rate. It is however possible that initial microbial processing increased the secondary chemical recalcitrance of originally labile OM (Prescott, 2010; Lützow et al., 2006), thereby increasing the sensitivity to phenolic inhibition of downstream enzymatic processes.
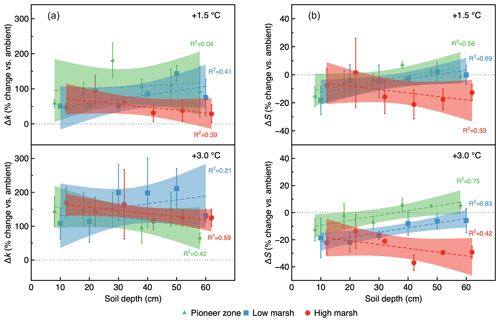
Figure 5Warming treatment effect size (percentage change vs. ambient) as a function of soil depth and marsh zone, shown for the initial decomposition rate, Δk (a), and the stabilization factor, ΔS (b), in year 2 (2019). Linear regression was used to analyze the relationships of Δk and ΔS with soil depth.
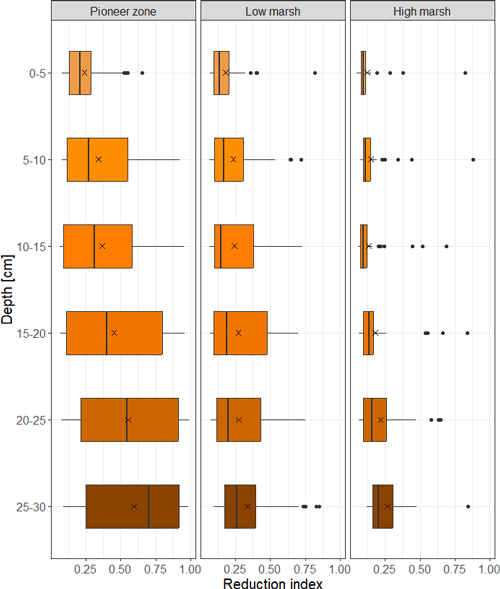
Figure 6Soil reduction in relation to marsh zone and soil depth at the Hamburger Hallig salt marsh site. Shown are median (black bar) and mean values (black X). The box gives the interquartile range, and potential outliers are depicted as black points. Data are based on n = 6 observations per zone, deployed over four consecutive deployment campaigns (July–October). The reduction index (0–1) describes the fraction of FeCl3 paint that is removed from the PVC stick after 4 weeks of deployment in the field.
The current state of science lacks a mechanistic understanding of labile OM processing and stabilization in wetland soils so that we cannot easily build hypotheses to explain the here observed redox control. For terrestrial soil systems, an increasing number of studies highlighted the importance of labile OM stabilization for long-term soil carbon storage (e.g., Cotrufo et al., 2013; Lützow et al., 2006). Along with this, the prevailing concept of terrestrial soil OM formation was called into question stating that primarily recalcitrant OM inputs (i.e., non-hydrolyzable compounds) stabilize in the soil matrix and build the soil OM pool (e.g., Lützow et al., 2006; Schmidt et al., 2011). The Microbial Efficiency-Matrix Stabilization (MEMS) framework hypothesizes that labile plant inputs are the primary source for soil OM formation. Specifically, the MEMS framework considers labile plant inputs the dominant microbial substrate source and thus the dominant source of microbial decomposition products. Microbial decomposition products, in turn, are the main precursors of stabilized soil OM, which forms through aggregation or strong chemical bonding to the mineral matrix in terrestrial soils (Cotrufo et al., 2013). It is questionable if MEMS or related frameworks can be applied to wetland soils, because here physical protection through mineral armoring is largely absent in organic soils and hypothesized to be of little consequence in tidal mineral soils, which often lack aggregates due to low fungal abundance and wet–dry cycles (Kirwan and Megonigal, 2013; but see Spivak et al., 2019). We therefore hypothesize that secondary recalcitrance of originally labile organic compounds via microbial processing (Lützow et al., 2006) plays a larger role in the stabilization of labile OM inputs in many wetland soils than aggregation and other interactions of OM with the mineral matrix.
Our data suggest that warming effects on litter breakdown are not necessarily linear. In year 1 of the experiment, +1.5 ∘C warming had a stronger positive effect on the initial decomposition rate than +3.0 ∘C warming in the high marsh. We doubt that potential warming-induced drought effects in the soil surface were the responsible driver of this pattern because higher k under +1.5 ∘C vs. +3.0 ∘C warming was relatively consistent throughout the 60 cm soil profile. Instead, it is possible that plant–microbe interactions caused the observed pattern. For instance, warming-stimulated nutrient demands and belowground trait responses in plants could have negatively affected microbial communities via competition for nutrients (Noyce et al., 2019).
Warming effects on k and S were qualitatively similar between year 1 and 2. However, effects were generally much more pronounced in year 2 compared to year 1 (Figs. 3–4). The experimentally achieved temperature-difference values were consistent between years (Table S1); however, differences in the actual temperature (not temperature difference) and the seasonal shift of 13 weeks between the two incubation periods of year-1 and year-2 deployment phases could have affected the magnitude of warming effects. Absolute soil temperatures were lower in year 2 than 1 (Fig. S1), which could have resulted in the amplified warming effect. It is also possible that changes in the microbial community with increased treatment duration and/or greater microbial biomass as warming-stimulated plant growth and substrate input to the soil system contributed to the observed effect amplification over time.
4.2 Methodological considerations
The use of PVC posts may have affected drainage and thus redox conditions of the deployed litter materials after flooding events. This could have amplified the redox differences between frequently and rarely flooded vegetation zones we observed. However, we argue that this potential effect on drainage is unimportant for the interpretation of our results, because our study was primarily designed to gain mechanistic insight, not to capture actual rates of litter breakdown.
Compared to most previous studies which used the TBI to investigate litter breakdown in surface (approx. 5 cm depth) soils according to the original TBI protocol (e.g., Fanin et al., 2020; Marley et al., 2019; Mueller et al., 2018; Sarneel et al., 2020), our study used the TBI to assess litter breakdown in whole-soil profiles (10–60 cm depth) in order to improve the mechanistic understanding of belowground carbon turnover. One important caveat in this respect is that we do not know how TBI materials relate to the quality and microbial accessibility of native belowground inputs, namely root litter and rhizodeposits such as exudates. Warming and other climate change drivers are expected to induce changes in the quality of plant litter and other organic matter inputs accumulating in salt marsh soils, for instance through shifts in the plant community composition that can potentially counterbalance or amplify the effects on decomposition processes described here (Mueller et al., 2018, 2020b). Future research within the MERIT project will therefore address litter quality-feedback effects on decomposition processes in order to gain a more complete understanding of warming effects on salt marsh soil carbon cycling.
While the quality of TBI materials is likely to somewhat resemble the quality of root litter, it is questionable if this study can be used to infer anything about the turnover dynamics of root exudates – known to represent a considerable belowground carbon flux (Canarini et al., 2019). In this context, it is also important to note that the TBI, as well as native litter bag techniques, likely reduces the influence of aggregate protection in relation to plant inputs under natural conditions. It is therefore possible that important stabilization mechanisms of terrestrial soils, relying on aggregation or chemical interactions with the mineral matrix, cannot be adequately captured by the method. The importance of these stabilization mechanisms in wetland soils, however, remains to be evaluated (Kirwan and Megonigal, 2013; Spivak et al., 2019).
A number of recent studies have highlighted the importance of dissolved organic carbon and nutrient leaching during early litter breakdown in the context of the TBI (Lind et al., 2022; Marley et al., 2019). Because leaching is a rapid process, particularly in wetlands, we assume that, in the present study, leaching was complete and thus did not contribute to the observed variability in k and S. Indeed, k did not increase, and S did not decrease with flooding (elevation gradient) or soil moisture (depth gradient), suggesting that leaching did not (overly) affect the variability of the data presented here.
Our results show that warming can strongly increase the initial rate of labile litter decomposition but has less consistent effects on the stabilization of this material. This finding suggests that warming may accelerate carbon and nutrient cycling through stimulated initial decomposition rates, whereas soil organic matter formation and carbon sequestration through stabilization may be less consistently affected. We argue that the differential outcome of warming effects on the initial decomposition rate and stabilization factor were mediated by the soil redox status, with redox conditions constraining the warming response of litter stabilization but not its initial decomposition rate. Because belowground organic matter turnover is a key determinant of surface elevation gain and carbon sequestration in blue carbon ecosystems, our findings may yield important implications for the understanding of climate change effects on ecosystem stability and carbon sequestration in the coastal zone.
All data presented in this paper are available upon reasonable request.
The supplement related to this article is available online at: https://doi.org/10.5194/bg-20-1925-2023-supplement.
HT, SN, KJ, and PM designed the TBI decomposition study. SN, KJ, and RR designed the field experiment. HT conducted the TBI assays and analyzed the resulting data. JMG conducted the study on soil reduction. HT and PM wrote the original draft with input from all co-authors.
The contact author has declared that none of the authors has any competing interests.
Publisher’s note: Copernicus Publications remains neutral with regard to jurisdictional claims in published maps and institutional affiliations.
We would like to thank Martin Stock, Armin Jess, and the administration of the Schleswig-Holstein Wadden Sea National Park for support and the opportunity to conduct the experiment at their site. We would like to thank Detlef Böhm and the many colleagues of the Applied Plant Ecology group who assisted with the construction of the experiment and especially Simon Thomsen, Tom Kamin, Jörn Ehlers, and Stefan Knaak for their help and valuable technical expertise.
Hao Tang received financial support from the China Scholarship council (grant no. CSC201606910043). Peter Mueller was funded by the Deutsche Forschungsgemeinschaft (DFG, German Research Foundation) within the Research Training Group (RTG) 2530 (Biota-mediated effects on carbon cycling in estuaries: project number 407270017). Julian Mittmann-Goetsch received funding from the Fischer Stiftung (Stifterverband für die Deutsche Wissenschaft) as part of the Seal-C project (a Stability Assessment of Wadden Sea Blue Carbon Stocks).
This paper was edited by Andreas Ibrom and reviewed by Inge Althuizen, Md. Golam Rakkibu, and one anonymous referee.
Barbier, E. B., Hacker, S. D., Kennedy, C., Koch, E. W., Stier, A. C., and Silliman, B. R.: The value of estuarine and coastal ecosystem services, Ecol. Monogr., 81, 169–193, https://doi.org/10.1890/10-1510.1, 2011.
Benner, R., Maccubbin, A. E., and Hodson, R. E.: Anaerobic biodegradation of the lignin and polysaccharide components of lignocellulose and synthetic lignin by sediment microflora, Appl. Environ. Microbiol., 47, 998–1004, https://doi.org/10.1128/aem.47.5.998-1004.1984, 1984.
Canarini, A., Kaiser, C., Merchant, A., Richter, A., and Wanek, W.: Root exudation of primary metabolites: Mechanisms and their roles in plant responses to environmental stimuli, Front. Plant Sci., 10, 157, https://doi.org/10.3389/fpls.2019.00157, 2019.
Charles, H. and Dukes, J. S.: Effects of warming and altered precipitation on plant and nutrient dynamics of a New England salt marsh, Ecol. Appl., 19, 1758–1773, https://doi.org/10.1890/08-0172.1, 2009.
Chmura, G. L.: What do we need to assess the sustainability of the tidal salt marsh carbon sink?, Ocean Coast. Manag., 83, 25–31, https://doi.org/10.1016/j.ocecoaman.2011.09.006, 2013.
Cotrufo, M. F., Wallenstein, M. D., Boot, C. M., Denef, K., and Paul, E.: The Microbial Efficiency-Matrix Stabilization (MEMS) framework integrates plant litter decomposition with soil organic matter stabilization: Do labile plant inputs form stable soil organic matter?, Glob. Change Biol., 19, 988–995, https://doi.org/10.1111/gcb.12113, 2013.
Elumeeva, T. G., Onipchenko, V. G., Akhmetzhanova, A. A., Makarov, M. I., and Keuskamp, J. A.: Stabilization versus decomposition in alpine ecosystems of the Northwestern Caucasus: The results of a tea bag burial experiment, J. Mt. Sci., 15, 1633–1641, https://doi.org/10.1007/s11629-018-4960-z, 2018.
Esselink, P., van Duin, W.E., Bunje, J., Cremer J., Folmer, E.O., Frikke, J., Glahn, M., de Groot, A. V., Hecker, N., Hellwig, U., Jensen, K., Körber, P., Petersen, J., and Stock, M.: Salt marshes, in: Wadden Sea Quality Status Report, edited by: Kloepper, S. et al., Common Wadden Sea Secretariat, Wilhelmshaven, Germany, https://qsr.waddensea-worldheritage.org/reports/salt-marshes (last access: 18 April 2023), 2017.
Fanin, N., Bezaud, S., Sarneel, J. M., Cecchini, S., Nicolas, M., and Augusto, L.: Relative Importance of Climate, Soil and Plant Functional Traits During the Early Decomposition Stage of Standardized Litter, Ecosystems, 23, 1004–1018, https://doi.org/10.1007/s10021-019-00452-z, 2020.
Freeman, C., Ostle, N., and Kang, H.: An enzymic “latch” on a global carbon store: A shortage of oxygen locks up carbon in peatlands by restraining a single enzymes, Nature, 149, 409, https://doi.org/10.1038/35051650, 2001.
Hopple, A. M., Wilson, R. M., Kolton, M., Zalman, C. A., Chanton, J. P., Kostka, J., Hanson, P. J., Keller, J. K., and Bridgham, S. D.: Massive peatland carbon banks vulnerable to rising temperatures, Nat. Commun., 11, 2373, https://doi.org/10.1038/s41467-020-16311-8, 2020.
Jenkinson, B. J.: Hydrology of sandy soils in northwest Indiana and iron oxide indicators to identify hydric soils, Purdue University, US, https://docs.lib.purdue.edu/dissertations/AAI3104964 (last access: 26 April 2023), 2002.
Keuskamp, J. A., Dingemans, B. J. J., Lehtinen, T., Sarneel, J. M., and Hefting, M. M.: Tea Bag Index: A novel approach to collect uniform decomposition data across ecosystems, Methods Ecol. Evol., 4, 1070–1075, https://doi.org/10.1111/2041-210X.12097, 2013.
Kirwan, M. L. and Blum, L. K.: Enhanced decomposition offsets enhanced productivity and soil carbon accumulation in coastal wetlands responding to climate change, Biogeosciences, 8, 987–993, https://doi.org/10.5194/bg-8-987-2011, 2011.
Kirwan, M. L. and Megonigal, J. P.: Tidal wetland stability in the face of human impacts and sea-level rise, Nature, 504, 53–60, https://doi.org/10.1038/nature12856, 2013.
Kirwan, M. L. and Mudd, S. M.: Response of salt-marsh carbon accumulation to climate change, Nature, 489, 550–553, https://doi.org/10.1038/nature11440, 2012.
Kristensen, E., Ahmed, S. I., and Devol, A. H.: Aerobic and anaerobic decomposition of organic matter in marine sediment: Which is fastest?, Limnol. Oceanogr., 40, 1430–1437, https://doi.org/10.4319/lo.1995.40.8.1430, 1995.
Langley, J. A. and Megonigal, J. P.: Ecosystem response to elevated CO2 levels limited by nitrogen-induced plant species shift, Nature, 466, 96–99, https://doi.org/10.1038/nature09176, 2010.
Lin, Y., Campbell, A. N., Bhattacharyya, A., DiDonato, N., Thompson, A. M., Tfaily, M. M., Nico, P. S., Silver, W. L., and Pett-Ridge, J.: Differential effects of redox conditions on the decomposition of litter and soil organic matter, Biogeochemistry, 154, 1–15, https://doi.org/10.1007/s10533-021-00790-y, 2021.
Lind, L., Harbicht, A., Bergman, E., Edwartz, J., and Eckstein, R. L.: Effects of initial leaching for estimates of mass loss and microbial decomposition – Call for an increased nuance, Ecol. Evol., 12, e9181, https://doi.org/10.1002/ece3.9118, 2022.
Lützow, M. V., Kögel-Knabner, I., Ekschmitt, K., Matzner, E., Guggenberger, G., Marschner, B., and Flessa, H.: Stabilization of organic matter in temperate soils: Mechanisms and their relevance under different soil conditions – A review, Eur. J. Soil Sci., 57, 426–445, https://doi.org/10.1111/j.1365-2389.2006.00809.x, 2006.
Marley, A. C. R. G., Smeaton, C., and Austin, W. E. N.: An Assessment of the Tea Bag Index Method as a Proxy for Organic Matter Decomposition in Intertidal Environments, J. Geophys. Res.-Biogeo., 124, 2991–3004, https://doi.org/10.1029/2018JG004957, 2019.
McLeod, E., Chmura, G. L., Bouillon, S., Salm, R., Björk, M., Duarte, C. M., Lovelock, C. E., Schlesinger, W. H., and Silliman, B. R.: A blueprint for blue carbon: Toward an improved understanding of the role of vegetated coastal habitats in sequestering CO2, Front. Ecol. Environ., 9, 552–560, https://doi.org/10.1890/110004, 2011.
Mori, T., Nakamura, R., and Aoyagi, R.: Risk of misinterpreting the Tea Bag Index: Field observations and a random simulation, Ecol. Res., 37, 381–389, https://doi.org/10.1111/1440-1703.12304, 2022.
Mueller, P., Schile-Beers, L. M., Mozdzer, T. J., Chmura, G. L., Dinter, T., Kuzyakov, Y., De Groot, A. V., Esselink, P., Smit, C., D'Alpaos, A., Ibáñez, C., Lazarus, M., Neumeier, U., Johnson, B. J., Baldwin, A. H., Yarwood, S. A., Montemayor, D. I., Yang, Z., Wu, J., Jensen, K., and Nolte, S.: Global-change effects on early-stage decomposition processes in tidal wetlands-implications from a global survey using standardized litter, Biogeosciences, 15, 3189–3202, https://doi.org/10.5194/bg-15-3189-2018, 2018.
Mueller, P., Mozdzer, T. J., Langley, J. A., Aoki, L. R., Noyce, G. L., and Megonigal, J. P.: Plant species determine tidal wetland methane response to sea level rise, Nat. Commun., 11, 5154, https://doi.org/10.1038/s41467-020-18763-4, 2020a.
Mueller, P., Granse, D., Nolte, S., Weingartner, M., Hoth, S., and Jensen, K.: Unrecognized controls on microbial functioning in Blue Carbon ecosystems: The role of mineral enzyme stabilization and allochthonous substrate supply, Ecol. Evol., 10 , 998–1011, https://doi.org/10.1002/ece3.5962, 2020b.
Mueller P., Kutzbach L., Mozdzer T.J., Jespersen E., Barber D., and Eller F.: Minerogenic salt marshes can function as important inorganic carbon stores, Limnol. Oceanogr., 68, 942–952, https://doi.org/10.1002/lno.12322, 2023.
Noyce, G. L., Kirwan, M. L., Rich, R. L., and Megonigal, J. P.: Asynchronous nitrogen supply and demand produce nonlinear plant allocation responses to warming and elevated CO2, P. Natl. Acad. Sci. USA, 116, 21623–21628, https://doi.org/10.1073/pnas.1904990116, 2019.
Ochoa-Hueso, R., Borer, E. T., Seabloom, E. W., Hobbie, S. E., Risch, A. C., Collins, S. L., Alberti, J., Bahamonde, H. A., Brown, C. S., Caldeira, M. C., Daleo, P., Dickman, C. R., Ebeling, A., Eisenhauer, N., Esch, E. H., Eskelinen, A., Fernández, V., Güsewell, S., Gutierrez-Larruga, B., Hofmockel, K., Laungani, R., Lind, E., López, A., McCulley, R. L., Moore, J. L., Peri, P. L., Power, S. A., Price, J. N., Prober, S. M., Roscher, C., Sarneel, J. M., Schütz, M., Siebert, J., Standish, R. J., Velasco Ayuso, S., Virtanen, R., Wardle, G. M., Wiehl, G., Yahdjian, L., and Zamin, T.: Microbial processing of plant remains is co-limited by multiple nutrients in global grasslands, Glob. Chang. Biol., 26, 4572–4582, https://doi.org/10.1111/gcb.15146, 2020.
Peterson, J., Kers, B., and Stock, M.: TMAP-typology of coastal vegetation in the Wadden Sea area, Common Wadden Sea Secretariat (CWSS), Wilhelmshaven, Germany, https://www.waddensea-secretariat.org (last access: 26 April 2023), 2014.
Prescott, C. E.: Litter decomposition: What controls it and how can we alter it to sequester more carbon in forest soils?, Biogeochemistry, 101, 133–149, https://doi.org/10.1007/s10533-010-9439-0, 2010.
Rabenhorst, M. C.: Using synthesized iron oxides as an indicator of reduction in soils, Methods Biogeochem. Wetl., 10, 723–740, https://doi.org/10.2136/sssabookser10.c37, 2015.
Rich, R., Mueller, P., Fuss, M., Gonçalves, S., Ostertag, E., Reents, S., Tang, H., Tashjian, A., Thomsen, S., Jensen, K., and Nolte, S.: Design and assessment of a novel approach for ecosystem warming experiments in high-energy tidal wetlands, J. Geophys. Res.-Biogeo., in review, 2023.
Sarneel, J. M. J. and Veen, G. F. C.: Legacy effects of altered flooding regimes on decomposition in a boreal floodplain, Plant Soil, 421, 57–66, https://doi.org/10.1007/s11104-017-3382-y, 2017.
Sarneel, J. M., Sundqvist, M. K., Molau, U., Björkman, M. P., and Alatalo, J. M.: Decompositon rate and stabilization across six tundra vegetation types exposed to 20 years of warming, Sci. Total Environ., 724, 138304, https://doi.org/10.1016/j.scitotenv.2020.138304, 2020.
Schmidt, M. W. I., Torn, M. S., Abiven, S., Dittmar, T., Guggenberger, G., Janssens, I. A., Kleber, M., Kögel-Knabner, I., Lehmann, J., Manning, D. A. C., Nannipieri, P., Rasse, D. P., Weiner, S., and Trumbore, S. E.: Persistence of soil organic matter as an ecosystem property, Nature, 478, 49–56, https://doi.org/10.1038/nature10386, 2011.
Sogin, E. M., Michellod, D., Gruber-Vodicka, H. R., Bourceau, P., Geier, B., Meier, D. V., Seidel, M., Ahmerkamp, S., Schorn, S., D'Angelo, G., Procaccini, G., Dubilier, N., and Liebeke, M.: Sugars dominate the seagrass rhizosphere, Nat. Ecol. Evol., 6, 866–877, https://doi.org/10.1038/s41559-022-01740-z, 2022.
Spivak, A. C., Sanderman, J., Bowen, J. L., Canuel, E. A., and Hopkinson, C. S.: Global-change controls on soil-carbon accumulation and loss in coastal vegetated ecosystems, Nat. Geosci., 12, 685–692, https://doi.org/10.1038/s41561-019-0435-2, 2019.
Tang, H., Nolte, S., Jensen, K., Yang, Z., Wu, J., and Mueller, P.: Grazing mediates soil microbial activity and litter decomposition in salt marshes, Sci. Total Environ., 720, 137559, https://doi.org/10.1016/j.scitotenv.2020.137559, 2020.
Tang, H., Liebner, S., Reents, S., Nolte, S., Jensen, K., Horn, F., and Mueller, P.: Plant genotype controls wetland soil microbial functioning in response to sea-level rise, Biogeosciences, 18, 6133–6146, https://doi.org/10.5194/bg-18-6133-2021, 2021.
Wilson, R. M., Hopple, A. M., Tfaily, M. M., Sebestyen, S. D., Schadt, C. W., Pfeifer-Meister, L., Medvedeff, C., Mcfarlane, K. J., Kostka, J. E., Kolton, M., Kolka, R. K., Kluber, L. A., Keller, J. K., Guilderson, T. P., Griffiths, N. A., Chanton, J. P., Bridgham, S. D., and Hanson, P. J.: Stability of peatland carbon to rising temperatures, Nat. Commun., 7, 13723, https://doi.org/10.1038/ncomms13723, 2016.
Zhang, D., Hui, D., Luo, Y., and Zhou, G.: Rates of litter decomposition in terrestrial ecosystems: global patterns and controlling factors, J. Plant Ecol., 1, 85–93, https://doi.org/10.1093/jpe/rtn002, 2008.