the Creative Commons Attribution 4.0 License.
the Creative Commons Attribution 4.0 License.
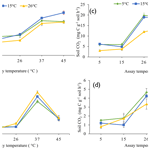
Differential temperature sensitivity of intracellular metabolic processes and extracellular soil enzyme activities
Adetunji Alex Adekanmbi
Laurence Dale
Liz Shaw
Predictions concerning the feedback of soil heterotrophic respiration to a warming climate often do not differentiate between the extracellular and intracellular steps involved in soil organic matter decomposition. This study examined the temperature sensitivities of intracellular metabolic processes and extracellular soil enzyme activities and how they are influenced by previous temperatures. We pre-incubated soils at 5, 15, or 26 ∘C to acclimatize the microbial communities to different thermal regimes for 60 d before measuring potential activities of β-glucosidase and chitinase (extracellular enzymes), glucose-induced respiration (intracellular metabolic processes), and basal respiration at a range of assay temperatures (5, 15, 26, 37, and 45 ∘C). A higher pre-incubation temperature decreased the soil pH and ratio and decreased β-glucosidase potential activity and respiration but not chitinase potential activity. It is likely that this legacy effect on β-glucosidase and respiration is an indirect effect of substrate depletion rather than physiological acclimatation or genetic adaptation. Pre-incubation temperature effects on temperature sensitivity were subtle and restricted to extracellular activities, perhaps because of the short (60 d) duration of the pre-incubation at temperatures that were below the initial optimum (∼ 30 ∘C) for the mesophilic soil community. However, we found that the intracellular and extracellular steps differ in their temperature sensitivity, and this observation differs depending on the range of temperature used for Q10 estimates of temperature sensitivity. Between 5 and 15 ∘C intracellular and extracellular processes show equal temperature sensitivity, but between 15 and 26 ∘C intracellular metabolic processes were more temperature sensitive than extracellular enzyme activity, and between 26 and 37 ∘C extracellular enzyme activity was more temperature sensitive than intracellular metabolic processes. This result implies that depolymerization of higher molecular weight carbon is more sensitive to temperature changes at higher temperatures (e.g. higher temperatures on extremely warm days), but the respiration of the generated monomers is more sensitive to temperature changes at moderate temperatures (e.g. mean daily maximum soil temperature). However, studies using multiple soil types and a greater range of pre-incubation temperatures are required to generalize our results. Nevertheless, since climate change predictions currently indicate that there will be a greater frequency and severity of hot summers and heatwaves, it is possible that global warming may reduce the importance of extracellular depolymerization relative to intracellular metabolic processes as the rate-limiting step of soil organic matter mineralization. We conclude that extracellular and intracellular steps are not equally sensitive to changes in soil temperature and that the previous temperature a soil is exposed to may influence the potential activity, but not temperature sensitivity, of extracellular and intracellular processes.
- Article
(1063 KB) - Full-text XML
-
Supplement
(958 KB) - BibTeX
- EndNote
Understanding the temperature sensitivity of soil organic matter (SOM) decomposition will help predict how soils might respond to climate change. There are two major enzymatically mediated steps involved in the decomposition of SOM to produce CO2 (Bárta et al., 2014; Maire et al., 2013; Blagodatskaya et al., 2016). The first step, extracellular depolymerization, requires extracellular enzymes of microbial (and also plant and animal) origin to depolymerize macromolecular constituents of SOM and produce soluble low-molecular-weight microbial substrates (Maire et al., 2013). The second step, intracellular metabolism, results in the release of CO2 after substrates are absorbed and catabolized by microbial cells, involving a multitude of intracellular metabolic processes.
Many ecological studies have examined the temperature sensitivity of SOM decomposition, but most of them measure the end product as respired CO2 (e.g. Wang et al., 2013) or mass loss of C substrate (e.g. Kirwan et al., 2014), which does not differentiate between the temperature sensitivity of contributing extracellular and intracellular processes. Temperature sensitivity, defined as the rate of change in reaction rate with respect to temperature, is the first derivative of the relationship between temperature and reaction rate (Alster et al., 2020). Temperature–rate relationships are typically unimodal, reflecting rising reaction rates with temperature due to thermodynamic effects and then a decline in rate with further increase in temperature related to thermal effects on enzyme activation and ultimately denaturation (Alster et al., 2020). Parameters describing the temperature–rate relationship have been shown to vary both with respect to extracellular enzyme type and between microbial taxa for the same enzyme type (Alster et al., 2016). Extracellular enzyme activity, rather than intracellular metabolic processes, is widely thought to be the rate-limiting step for respiration of organic matter in soils (Jan et al., 2009; Bradford, 2013), but very few studies have explicitly compared the temperature sensitivity of extracellular and intracellular processes to understand how each step might respond to increases in temperature and whether the magnitude of dependence of intracellular catabolism and CO2 respiration on extracellular enzyme activities for supply of substrate increases or decreases with increasing temperature. Ultimately, this lack of information limits our predictive understanding of how the soil carbon cycle will respond to future global temperature changes (Blagodatskaya et al., 2016).
As already stated, decomposition of SOM is a function of the heterotrophic microbial community and the extracellular enzymes it produces. If the microbial community and its enzyme production adapt to warming (or cooling), this might result in variation in the size of microbial enzyme (and biomass) pools (Fanin et al., 2022). In addition, thermal adaptation of the microbial community may, for a given enzyme-catalysed reaction, modulate the temperature–reaction rate relationship (e.g. manifest as a shift in the temperature optimum of reaction rates) and thus temperature sensitivity of extra- and intracellular processes depending on soil thermal history (Wallenstein et al., 2010). Adaptation at the level of the microbial community may be through acclimation (phenotypic or physiological change to respond to thermal regime, including production of different isozymes within taxa), evolutionary changes within taxa leading to novel isozymes, or species sorting where taxa (including their enzyme systems) already better adapted to a certain temperature competitively exclude those less adapted (Birgander et al., 2013). Whether adaptive processes modulate the activity and temperature response relationship to the same extent for intracellular and extracellular processes is not known. Since extracellular enzymes catalyse what is believed to be the rate-limiting step in SOM decomposition (Duly and Nannipieri, 1998; Alvarez et al., 2018), any thermal adaptation of extracellular enzymes will then determine how much substrate is available for subsequent uptake and respiration and represents an important control on the response of ecosystems to warming (Bradford, 2013).
In this study, we measured potential extracellular enzyme activity and glucose-induced respiration, as a proxy for intracellular metabolic processes, at five assay temperatures (5, 15, 26, 37, and 45 ∘C) following pre-incubation for 60 d at 5, 15, or 26 ∘C. The aim was to compare the temperature sensitivity of the extra- and intracellular steps of organic matter decomposition in soils that have previously been incubated at different thermal regimes, alongside measurements of key soil properties that we consider may lead to changes in potential enzyme activity. The pre-incubation temperatures were selected to be realistic for the site where the soil was sampled. We hypothesized that (i) extracellular and intracellular processes are not equally sensitive in their response to increasing temperature, given the involvement of different enzymes and (for intracellular catabolism) biochemical networks, and (ii) extracellular and intracellular processes, and their temperature sensitivity, are influenced by pre-incubation temperature due to thermal adaptation of the soil microbial community.
2.1 Soil sampling and pre-incubation
Soil samples were collected from a depth of 3–10 cm from a permanent grassland field at Sonning, UK (latitude 51∘28.564′, longitude 000∘54.198′), sieved with a 4 mm sieve, mixed, and homogenized before randomly allocating to replicates. Four “field moist” (soil moisture content = 0.13 g H2O g soil−1) replicate sub-samples (750 g) were pre-incubated at 5, 15 ∘C (similar to the mean daily minimum (6.8 ∘C) and the mean daily maximum (15.0 ∘C) temperatures measured at the University of Reading Atmospheric Observatory, close to the sampling location, between 2009 and 2019), and 26 ∘C (typical of a temperature measured on a warm summer day at the University of Reading Atmospheric Observatory; Fig. S1 in the Supplement) for a period of 60 d in plastic containers with the cover of each container loosely closed. Soil moisture content was adjusted to the initial field moist condition every 2 weeks for soils incubated at 5 and 15 ∘C and weekly for soils incubated at 26 ∘C. The soil is a slightly acidic loamy soil, classified as Chromic Endoskeletic Luvisol. A detailed description of the site is provided by Adekanmbi et al. (2020).
2.2 Experimental design
The experimental design included three pre-incubation temperatures (5, 15, and 26 ∘C), replicated four times, resulting in 12 experimental units. At the end of the 60 d pre-incubation period, soils were sub-sampled for determination of basal respiration and substrate-induced respiration using glucose as the substrate (Sect. 2.3) and the potential activity of β-glucosidase (β-1,4-glucosidase) and chitinase (N-acetyl β-D-glycosaminidase) extracellular enzymes (Sect. 2.4), all measured at five assay temperatures (5, 15, 26, 37, and 45 ∘C). Assays were performed on all experimental units within the same week to minimize variability due to the time of assay. Incubation temperatures were randomized to prevent systematic bias in the results. A portion of the soil from each replicate sample was also analysed for total C, total N, pH, and microbial biomass carbon (Sect. 2.5).
2.3 Basal and substrate-induced respiration
For each replicate sample, 15 g (13.31 g dry weight equivalent) of soil was weighed into a 50 mL centrifuge tube. Glucose solution (2 mL) was added at concentrations of 0 (deionized water only) or 10 mg g−1 soil (an initially saturating concentration of glucose as determined in a preliminary experiment; see Supplement), thus bringing the soil to 58 % of its water-holding capacity. The soil was then mixed to distribute the solution throughout. Following soil-substrate mixing, the tube was ventilated by blowing in lab air with a 20 mL syringe. The tubes were then sealed with septum stoppers, and 15 mL of lab air was injected. The headspace was flushed by moving the syringe plunger up and down several times before sampling 15 mL of headspace gas (as the T0 sample) and injecting it into an evacuated 12 mL exetainer vial, creating overpressure, using a tap and needle attached to the syringe. Soil samples were incubated for 1 h at either 5, 15, 26, 37, or 45 ∘C. It is likely that during the first few minutes of the assay the soils were changing temperature from room temperature to the assay temperature. At the end of the incubation, the process of injecting air, flushing, and sampling was repeated (T1 sample). Headspace gas samples were stored at 20 ∘C prior to analysis by an Agilent 7890B gas chromatograph. After calibrating with CO2 gas standards, the concentration of CO2 in mg L−1 was converted to C-CO2 in mg C g−1 soil h−1 as described by Salazar-Villegas et al. (2016):
where V is the volume of the headspace in the centrifuge tube, T1 is the CO2 concentration after a 1 h incubation in mg L−1, T0 is the CO2 concentration before a 1 h incubation in mg L−1, W is the dry weight of the soil, and t is the time between T0 and T1 measurements in hours.
2.4 Extracellular enzyme assays
Extracellular enzyme assay methods were based on Eivazi and Tabatabai (1988) and Parham and Deng (2000) for β-1,4-glucosidase (β-glucosidase) and N-acetyl β-D-glycosaminidase (chitinase), respectively. For each experimental replicate, 1 g of soil was weighed into a 50 mL centrifuge tube and mixed with 4 mL of room temperature pre-incubated 4-methylumbelliferone (MUB) buffer (pH 6) and either 1 mL 25 mM p-nitrophenyl-β-D-glucopyranoside or 10 mM p-nitrophenyl-N-acetyl-b-D-glucosaminide solution to assess β-glucosidase and chitinase activity, respectively. Samples were incubated at 5, 15, 26, 37, or 45 ∘C for 30 min, after which 1 mL 0.5 M CaCl2 and 4 mL tris buffer (pH 12) were added to stop the reaction. It is likely that during the first few minutes of the assay the soils were changing temperature from room temperature to the assay temperature. Samples were mixed by swirling, then filtered with Whatman no. 2 filter paper. Additionally, two blanks (for each run) were created by adding substrate to tubes containing the mixture after the reaction had stopped. The colour intensity of the filtrate was measured using a spectrophotometer at 400 nm and blank-corrected sample absorbance converted to micrograms of p-nitrophenol per reaction using p-nitrophenol standard solutions (0, 10, 20, 30, 40, and 50 µg p-nitrophenol). Potential enzyme activities were expressed as mg p-nitrophenol g−1 dry soil h−1. The 30 min assay incubation time was within the time range where product accumulation was linear with incubation time, according to a preliminary experiment (see Supplement).
2.5 Measurement of total carbon, total nitrogen, pH, and microbial biomass carbon
Microbial biomass carbon was measured using the fumigation and extraction method described by Vance et al. (1987). Four replicates from each pre-incubation temperature were weighed to the moist mass equivalent to 50 g oven-dried soil in beakers and placed in a vacuum desiccator lined with damp paper towel to ensure high humidity, along with a beaker containing about 50 mL ethanol-free chloroform and several anti-bumping granules. The desiccator was evacuated, and the chloroform was allowed to boil for 2 min before the valve was closed and the desiccator kept in the dark for 24 h. Before extraction, the chloroform was removed, the desiccator evacuated three times, and the samples left to vent to ensure no chloroform remained in the soil.
Extraction was carried out on both fumigated soil and non-fumigated duplicates. Samples of both were placed into 350 mL polypropylene bottles, to which 200 mL 0.5 M K2SO4 was added, before being placed on an oscillating shaker for 30 min. The suspension was then filtered into polypropylene universal tubes before being stored in a freezer prior to analysis. After removal from the freezer, samples were diluted by a factor of 10 and filtered to remove CaSO4 that had precipitated, before analysis for total organic carbon (TOC) using a Shimadzu TOC 5000. Also analysed were method blanks consisting of K2SO4 that had not been used to extract soil, to correct for any part of the reading not due to organic carbon content. TOC extracted from fumigated and non-fumigated samples was converted to a biomass carbon value by multiplying the difference (Ec) by 2.64, following Vance et al. (1987). The TOC of the non-fumigated soil before conversion represents the K2SO4 extractable carbon.
Total C and N were determined using the dry combustion method; 2 mm sieved soil samples were ground for 3 min in an agate ball mill. From the residue, 10 mg duplicates were weighed out using a five-point balance and placed in tinfoil capsules for measurement. C and N concentrations were analysed using a elemental analyser (Thermo Flash 2000 EA). The ratio was calculated from total C and N.
pH was determined in water (10 g air-dried soil: 25 mL deionized water) following end-over-end shaking (30 rpm, 15 min) and using a calibrated (pH 4.0 and pH 7.0) pH meter.
2.6 Temperature sensitivity
Temperature sensitivity (Q10) of both the intra- (glucose-induced respiration) and extracellular (chitinase and β-glucosidase) enzyme activities was calculated using the equal time measurement method, as described by Karhu et al. (2014). Q10 was calculated at three temperature ranges (, , and ). The primary reason why we calculated Q10 at different temperatures is because we found that temperature sensitivity was different at different temperature ranges. This meant that there was not a good linear relationship between the natural log of enzyme intracellular metabolic processes or extracellular enzyme activity and temperature, apart for β-glucosidase, as demonstrated in the Supplement. A similar range of incubation temperatures was used by Wang et al. (2013). Arrhenius enzyme activation energy (Ea) was calculated from the slope of the relationship between and the natural logarithm of the rate of enzyme activity (R0, the gas universal constant: 8.314 J mol−1; T, temperature in Kelvin), as described by Li et al. (2015). Ea was calculated using assays within the 5–26 ∘C temperature range for all four assays to ensure that the data used to calculate Ea conformed to the Arrhenius functional form (Schulte, 2015).
2.7 Statistical analysis
Two-way analysis of variance (ANOVA) was carried out to assess the effects of pre-incubation temperature and assay temperature on basal respiration, glucose-induced respiration, and extracellular enzyme activities. We also assessed whether intracellular and extracellular steps were equally sensitive to temperature, and whether this was influenced by pre-incubation temperature, by performing a two-way ANOVA on the Ea and Q10 values using assay type and pre-incubation temperature as factors. One-way ANOVA was carried out to assess the effect of pre-incubation temperature on soil properties. ANOVA was performed in Minitab version 18. Tukey pairwise comparisons were used to assess the significance of differences between individual treatment means.
3.1 Impact of pre-incubation temperature on selected soil properties
The effects of soil pre-incubation temperature on the soil total C, total N, ratio, pH, and microbial biomass carbon are presented in Fig. 1. Pre-incubation temperature did not have a statistically significant impact on C (P=0.641) or N (P=0.439). However, the soil ratio was significantly (P<0.05) higher in soil pre-incubated at 15 and 5 ∘C, compared to soil pre-incubated at 26 ∘C. Also, pre-incubation temperature significantly (P<0.05) influenced soil pH, which decreased in the order 5 ∘C > 15 ∘C > 26 ∘C. There was no statistically significant effect of soil pre-incubation temperature on soil microbial biomass (P = 0.206).
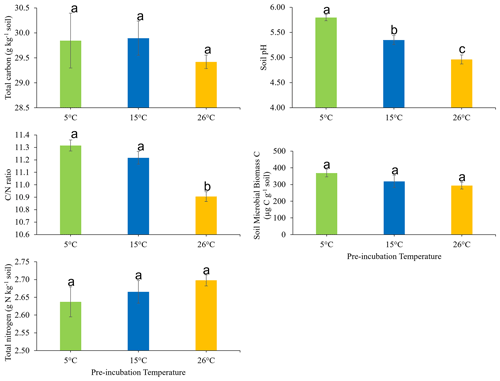
Figure 1Effects of pre-incubation temperature on the soil total carbon, total nitrogen, carbon-to-nitrogen () ratio, pH, and microbial biomass carbon. Each bar and error bar represent the mean and standard error of four replicate samples at each pre-incubation temperature. Means with the same letter are not significantly different (P>0.05).
3.2 Responses of intracellular and extracellular processes to pre-incubation temperature and assay temperature
The influence of pre-incubation temperature on the potential activities of β-glucosidase (Fig. 2a) and chitinase (Fig. 2b) extracellular enzymes, the rate of glucose-induced respiration (representing intracellular metabolic processes) (Fig. 2c), and the basal respiration rate (Fig. 2d) across the full range of assay temperatures (5 to 45 ∘C) are presented in Fig. 2.
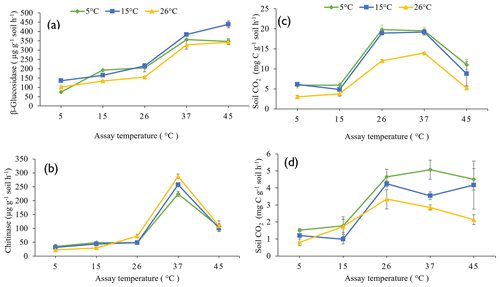
Figure 2Response of β-glucosidase activity (a), chitinase activity (b), glucose-induced respiration (c), and basal respiration (d) to five assay temperatures (5, 15, 26, 37, and 45 ∘C) undertaken on soils pre-incubated at three different temperatures (5, 15, and 26 ∘C). Each symbol and error bar represent the mean and standard error of four replicate samples.
The pre-incubation temperature (P<0.0001), assay temperature (P<0.0001), and their interaction (P = 0.001) significantly influenced potential β-glucosidase activity in soil. Soils pre-incubated at 26 ∘C had a lower potential β-glucosidase activity compared to those pre-incubated at 15 or 5 ∘C. Increasing assay temperature increased β-glucosidase activity up to the maximum assay temperature of 45 ∘C (Fig. 2a). Pre-incubating soils at 15 ∘C resulted in significantly greater potential β-glucosidase activity at the higher assay temperatures (45 and 37 ∘C) than by pre-incubating soils at 5 or 26 ∘C.
Both assay temperature (P<0.0001) and the interaction between assay and pre-incubation temperatures (P<0.001) significantly influenced potential chitinase activity, but pre-incubation temperature (P=0.077) did not. Chitinase activity increased with increasing assay temperature, reaching a maximum when assayed at 37 ∘C, but was lower when assayed at 45 ∘C (Fig. 2b). Pre-incubating soil at 26 ∘C and assaying at 37 ∘C resulted in a significantly (P=0.001) greater chitinase activity than pre-incubating at 5 ∘C and assaying at 37 ∘C. When assayed at 5 or 15 ∘C, pre-incubation at 26 ∘C resulted in lower chitinase activities than pre-incubation at 15 or 5 ∘C.
Both the pre-incubation temperature (P<0.0001) and assay temperature (P<0.0001) significantly influenced glucose-induced respiration but not their interaction (P=0.130). Similarly, the pre-incubation temperature (P=0.001) and assay temperature (P<0.0001) significantly influenced basal respiration but not their interactions (P=0.250). With or without glucose addition, pre-incubating soil at 26 ∘C resulted in lower soil respiration compared to pre-incubating soil at 5 or 15 ∘C (Fig. 2c and d). Glucose-induced respiration increased with increasing assay temperature, reaching maximum between 26 and 37 ∘C, but was significantly lower at 45 ∘C. Basal respiration increased with increasing assay temperature up to 26 ∘C then declined only slightly. The addition of 10 mg per gram of soil of glucose led to about a 4-fold increase in CO2 respired, compared to no addition of glucose substrate.
3.3 Effect of pre-incubation temperature and assay type (intra- or extracellular) on temperature sensitivity of metabolic processes
3.3.1 Temperature coefficient (Q10)
The effects of pre-incubation temperature and enzyme type on , , and are presented in Fig. 3. There was no overall significant effect (P>0.05) of pre-incubation temperature on Q10 calculated using all three temperature intervals. There was also no significant effect of assay type (P=0.393), or the interaction between assay type and pre-incubation temperature (P=0.700), on . However, significantly differed with assay type (P<0.0001) but not for the interactions between assay type and pre-incubation temperature (P=0.160). The was significantly lower for both extracellular enzymes (chitinase and β-glucosidase) than for glucose-induced respiration or basal respiration, irrespective of pre-incubation temperature. This result indicates that intracellular metabolic processes are more temperature sensitive than extracellular enzymes in this soil between 15 and 26 ∘C. Furthermore, was significantly affected by assay type (P<0.0001) but exhibited the opposite pattern to . for chitinase activity and β-glucosidase activity was significantly (P<0.05) greater than the for glucose-induced respiration and basal respiration. This finding indicates that extracellular enzymes are more temperature sensitive than intracellular metabolic processes in this soil between 26 and 37 ∘C. for chitinase activity was significantly (P<0.05) greater than for β-glucosidase activity. There was also a significant interaction between enzyme type and pre-incubation temperature (P=0.018). Chitinase activity was less temperature sensitive when soil was pre-incubated at 26 ∘C compared to when pre-incubated at 15 or 5 ∘C.
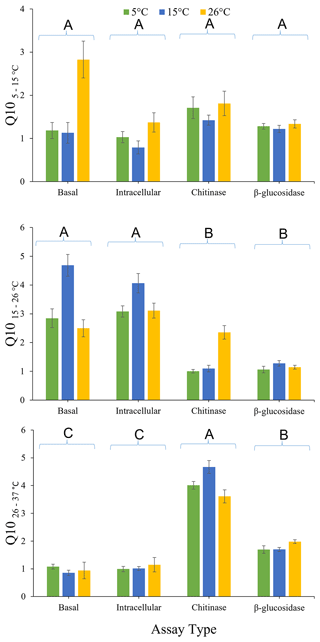
Figure 3Effects of pre-incubation temperature on temperature sensitivity (, , and ) of basal respiration rate, glucose-induced respiration, and extracellular (chitinase and β-glucosidase) enzyme activity. Each bar and error bar represent the mean and standard error of four replicate samples each pre-incubated at one of three different pre-incubation temperatures (5, 15, or 26 ∘C). Assay types sharing the same uppercase letters are not significantly different (P>0.05).
3.3.2 Arrhenius activation energy (Ea)
The activation energy (Ea), derived from the fit of the Arrhenius equation (Fig. 4) to assays performed between 5 and 26 ∘C, differed significantly with assay type (P<0.0001) and pre-incubation temperature (P=0.002), and there was a significant interaction between assay type and pre-incubation temperature (P=0.029). Ea increased with increasing pre-incubation temperature, with soils pre-incubated at 26 ∘C exhibiting the highest Ea and soils pre-incubated at 5 ∘C exhibiting the lowest. β-glucosidase activity and chitinase activity had a significantly (P<0.05) lower Ea than intracellular metabolic activity and basal respiration, though chitinase had similar Ea with intracellular metabolic activity when soils were pre-incubated at 26 ∘C.
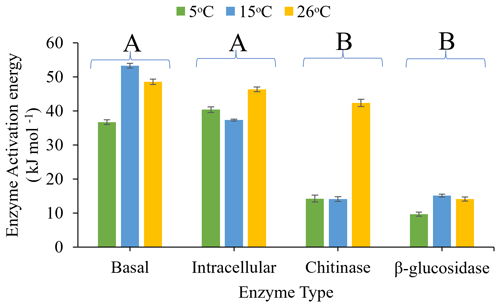
Figure 4Effects of pre-incubation temperature (5, 15, or 26 ∘C) on Arrhenius enzyme activation energy (Ea) for basal respiration rate, intracellular (glucose-substrate-induced respiration) enzyme activity, and extracellular (chitinase and β-glucosidase) enzyme activity. Ea was calculated based on assays undertaken between 5 and 26 ∘C for all four assays. Each bar and error bar represent the mean and standard error of four replicates. Enzyme types sharing the same uppercase letters are not significantly different (P>0.05).
Understanding whether soil intracellular and extracellular processes, which each play a distinct role in SOM decomposition, are equally sensitive to temperature changes was the major motivation for this study. We also examined whether pre-incubation temperature drives thermal adaption of the soil microbial community and results in differential alteration of temperature sensitivity of intracellular and extracellular enzyme activity. Therefore, we pre-incubated soil samples at three different temperatures to expose the soil microbial community to a particular thermal regime and then assayed intracellular processes (as respiration induced by a saturating concentration of glucose) and potential extracellular enzyme activity. Because extracellular enzymes were assayed in soil slurries and also in the presence of excess substrate, it was assumed that substrate diffusion or substrate concentration did not limit reaction rates and that the observed potential reaction rate was thus a function of enzyme properties and enzyme concentration (Wallenstein and Weintraub, 2008). Alongside intracellular and extracellular enzyme steps we measured basal respiration as a reference. We assume that the rate of basal respiration will represent the intracellular metabolic processes as supplied by substrate from extracellular enzyme activity and that the rate of respiration may be limited by substrate availability (to extracellular processes) and its supply (to intracellular processes) by extracellular activity and diffusion. Thus, any differential effects of pre-incubation temperature on temperature sensitivity of basal respiration cannot be interpreted solely as a function of differences in the cellular physiology of the microbial communities present.
Examining the general shape of the response of potential activity to assay temperature, we found that activity of β-glucosidase increased with increasing incubation temperature to our highest assay temperature of 45 ∘C. Our result is consistent with the increase in β-glucosidase activity with temperature reported in other studies using assay temperatures as low as 2 ∘C and as high as 65 ∘C (Steinweg et al., 2013) or 70 ∘C (Trasar-Cepeda et al., 2007) which show increases in activity up to and beyond 45 ∘C. The potential activity of chitinase also increased with temperature, but, in contrast to β-glucosidase, the response over the range of assay temperatures was non-monotonic, reaching a maximum activity between 37 and 45 ∘C. This observed non-monotonic response to increasing temperature is interpreted in terms of three distinct phases: (i) a rising phase where temperature increases lead to increasing reaction rate due to thermodynamic effects, (ii) a plateau which represents the optimum temperature, and (iii) a steep falling phase where rate declines beyond the optimum temperature (Schulte, 2015), attributed to thermal denaturation of proteins. Our optimum for chitinase (37 to 45 ∘C) is relatively consistent with the report of a maximum activity for soil chitinase of 45.5 ∘C (as assayed through quantification of N-acetyl-glucosamine released from added chitin; Rodriguez-Kabana et al., 1983) but contrasts to the optimum of ∼ 63 ∘C reported in the study by Parham and Deng (2000) using the same p-nitrophenol-based assay as used here. Differences in these optimum temperature activity responses between soils may be due to differences in microbial composition (and thus microbial-produced chitinase isozymes) between soils. Optimum temperatures varying between 40 and 60 ∘C have been recorded for chitinases (partially) purified from soil microorganisms (Gao et al., 2008; Alster et al., 2016; Du et al., 2021; Thakur et al., 2021). Additionally, soil-type-dependent stabilization of enzyme structure against thermal denaturation through interaction with soil surfaces might also mediate differential temperature responses (Sarkar et al., 1989). It is presumed that β-glucosidase activity in our study soil had a temperature optimum beyond the maximum tested, and our finding that the optimum temperature for chitinase activity was lower than that of β-glucosidase is likely due to between-enzyme family differences in protein structural properties conferring thermal stability, resulting in differential susceptibility of different enzyme families to thermal denaturation or degree of stabilization in soil. Our finding that intracellular metabolic processes increased with increasing assay temperature up to an optimal temperature between 26 and 37 ∘C, followed by a significant decline thereafter, is very likely due to the inability of the microbial population to function optimally above 37 ∘C due to impairments in their physiological processes (Todd-Brown et al., 2012; Maire et al., 2013) and uncoupling of relative rates of constituent enzymes leading to regulatory compromise (Prentice et al., 2020). The optimum temperature recorded here is greater than the annual average temperature but is within the range of the maximum soil temperatures experienced for this soil. These findings are in agreement with other studies on temperate soils recording an optimum temperature for microbial growth of ∼ 30 ∘C (Bárcenas-Moreno et al., 2009), although the basal respiration rate has been shown to increase with increasing temperature to 45 ∘C and not to be coupled to microbial growth (Pietikäinen et al., 2005). Prentice et al. (2020) identify a relationship between the inflection point of the rising phase of the thermal response of intracellular enzyme activities and the growth rate of the organism. This inflection seems to occur between 15 and 26 ∘C for intracellular metabolic processes and between 26 and 37 ∘C for extracellular enzymes in our experiment, and pre-incubation temperature does not consistently affect the temperature at which this inflection point occurs (Fig. 2). However, the chitinase enzyme activity does not clearly demonstrate the bell-shaped thermal response expected by macromolecular rate theory. This response may have been observed if assays were undertaken at more temperatures between 26 and 45 ∘C.
We found that the intracellular metabolic processes and extracellular enzyme activities differ in their temperature sensitivity, and this observation differs depending on the range of temperature used for Q10 estimates of temperature sensitivity. Intracellular metabolic processes were more sensitive to temperature changes at a moderate temperature range (15 and 26 ∘C) than extracellular enzymes. Conversely, extracellular enzymes were more sensitive than intracellular processes to temperature changes at a higher temperature range (26 and 37 ∘C). These results imply that, in the soil we studied, extracellular depolymerase activity was more temperature sensitive at higher temperatures, and intracellular metabolic processes were more temperature sensitive at moderate temperatures. At the site where the soil was collected for this experiment, the annual mean daily maximum soil temperature was approximately 15 ∘C, whereas 26 ∘C reflected a typical hot summer day. Therefore, assuming the absence of any thermal adaptation, we might expect intracellular metabolic processes to be more sensitive to global-warming-induced increases in the mean daily maximum soil temperature, but extracellular enzymes might be more sensitive to increased maximum temperatures on extremely warm days. The findings described above support our first hypothesis that the potential rate of extracellular depolymerization and intracellular catabolism are not equally temperature-sensitive steps in the mineralization of organic matter in soils. As far as we are aware, only one other study (Blagodatskaya et al., 2016) has considered intra- and extracellular steps involved in organic matter decomposition and their responses to temperature separately. Our finding that intracellular metabolic processes are more temperature sensitive at moderate temperatures is in agreement with Blagodatskaya et al. (2016) who calculated a for intracellular glucose oxidation of 5.1 and for chitinase and β-glucosidase activity of 1.9 and 2, respectively. Other previous research (Trasar-Cepeda et al., 2007) has compared intracellular metabolic processes (via dehydrogenase assay) and extracellular enzyme activity responses to a wider range of temperatures (5–70 ∘C), but, not necessarily under substrate-excess conditions for intracellular metabolic processes, as we have done here. Thus, further experiments are required to evaluate the applicability of our finding of a greater temperature sensitivity of extracellular activities at higher (26 and 37 ∘C) temperature ranges to other soil types.
Climate change predictions currently indicate that there will be a greater frequency and severity of hot summers and heatwaves in Europe (Meehl and Tebaldi, 2004; Christidis et al., 2015), including southeast England, where the soil was collected for this study. Therefore, our findings imply that, in the absence of substrate availability (or other, e.g. moisture) limitations to activity, the rate of extracellular depolymerase-catalysed reactions will increase during heatwaves to a greater extent than the rate of intracellular metabolic processes. Depending on the relative sizes of the intra- and extracellular enzyme pools and substrate availability, it is possible that global warming may reduce the importance of extracellular depolymerization relative to intracellular metabolic processes as the rate-limiting step of SOM mineralization under in situ conditions. Such a switch in rate limitation, if applicable generally across all extra- and intracellular reactions, would result in a buildup of low-molecular-weight substrates in the soil and thus potential for greater losses of C from the soil profile as dissolved organic carbon, an often overlooked component of terrestrial carbon budgets (Evans et al., 2014; Cook et al., 2018).
The temperature sensitivity of C mineralization is generally found to decrease with temperature (Niklińska and Klimek, 2007; Wang et al., 2013), and this trend has been observed in a synthesis of soil respiration measurements from laboratory studies which revealed that Q10 correlates negatively with the range of temperatures used to generate the Q10 value below 25 ∘C (Hamdi et al., 2013). This is consistent with kinetic theory of temperature dependence of reaction rates that explains that the fraction of molecules with sufficient energy to react decreases in relative terms as temperature increases (Davidson and Janssens, 2006). However, similar results to our study have been reported for mineralization of (labile) C where calculated Q10 values were lower in the 0–10 or 5–15 ∘C range than 10–20 or 15–25 ∘C range, respectively (Howard and Howard, 1993; Wang et al., 2013). These findings possibly reflect that CO2 production is not a function of a single non-enzyme-catalysed chemical reaction but is subject to moderation by the temperature sensitivity of other components in the involved biochemical network (for example, reduced membrane fluidity at lower temperature), with implications for substrate uptake and function of membrane-embedded proteins (Schulte, 2015). Also, based on kinetic theory, it is suggested that substrates that are more recalcitrant should have higher temperature sensitivities (Davidson and Janssens, 2006). It might be initially supposed that the substrates that are hydrolysed by chitinase and β-glucosidase enzymes in depolymerization reactions might be more recalcitrant than glucose and other lower-molecular-weight substrates for intracellular respiration and, consequently, the extracellular-catalysed reactions should have higher temperature sensitivities. This supposition is supported by the data but not for Q10 calculated using the other temperature ranges. However, it should be recognized that chitinase and β-glucosidase have relatively simple dimeric or trimeric substrates in nature and are assayed using artificial and simple substrates that may not be more recalcitrant than those used in intracellular metabolism. In addition, the theoretical predictions refer to chemical decomposition reactions and not necessarily those involving enzyme catalysis (Blagodatskaya et al., 2016). Indeed, comparison of intracellular versus extracellular estimated activation energies (Fig. 4) suggested that the extracellular enzyme substrates had similar or lower (for β-glucosidase) recalcitrance. The activation energy values we obtained were in broad agreement with those reported in other studies (Trasar-Cepeda et al., 2007).
In addition to the differences between the temperature sensitivity of extra- and intracellular processes, the extracellular activities were not equally temperature sensitive to each other according to and activation energy (integrating the temperature response between 5 and 26 ∘C), with chitinase being more sensitive than β-glucosidase. Previous studies have also shown that the temperature sensitivity of particular classes of enzyme differs within the same soil environment (Wallenstein et al., 2010), although specific comparisons between β-glucosidase and chitinase have not always revealed significant differences between these enzyme classes (e.g. Nottingham et al., 2016; Min et al., 2014, 2019; Wei et al., 2021). Therefore, the sign and magnitude of within-soil differences may not be consistent across soil types. In the case of differential temperature sensitivity with respect to assay type, there are implications for temperature-dependent variation in the quality of monomeric SOM constituents supplying respiration (Wallenstein and Burns, 2011). In the chitinase vs. β-glucosidase example here, the relative activity of these enzymes would change with temperature (assuming no change in enzyme or substrate concentration), altering the relative production of glucose and N-acetyl-glucosamine monomers and thus C and N resource availability to soil microbial communities (Min et al., 2014).
In respect of the second hypothesis, the observation that pre-incubation at 26 ∘C resulted in significantly lower activity, when considered across all assay temperatures, for β-glucosidase and intracellular catalytic enzymes (as well as basal respiration), compared to pre-incubation at 5 or 15 ∘C, suggests possible adaptation of these processes to the direct or indirect effects of temperature. The indirect effects could be due to temperature-induced changes in soil properties during pre-incubation, with consequences for soil microbial activities (Sinsabaugh, 1994; Sinsabaugh et al., 1991; Adeli et al., 2005; Sinsabaugh et al., 2008; Puissant et al., 2019). It was evident in our results that pre-incubating soils at 26 ∘C reduced the ratio when compared to pre-incubation at 5 or 15 ∘C. This probably reflects enhanced decomposition of organic matter at the warmer pre-incubation temperature and the resulting mass loss of CO2-C and enrichment of N (on a mass basis), leading to the statistically significant effect when expressed in ratio form. Temperature-induced changes in the ratio have been reported previously (Bárta et al., 2014; Souza and Billings, 2022). The lower intracellular enzyme activity after 2 months' exposure to a higher pre-incubation temperature is likely due to a lower (indicated but not statistically significant) microbial biomass (and thus a reduced intracellular enzyme pool) responding to depleted relative C availability. The lower activity of β-glucosidase for 26 ∘C pre-incubated soil most likely also reflects a lower enzyme pool size, given the nature of the potential assay used to measure reaction rate and its relationship to enzyme concentration (Wallenstein and Weintraub, 2008). It is likely that such indirect effects of pre-incubation temperature on the microbial enzyme pool size mask any direct thermal acclimatation or genetic adaptation of the soil microbial community and subsequent change in the temperature sensitivity of the enzymes it produces. It is often found that substrate depletion plays a greater role in the response of soil microbial communities to warming than physiological or genetic shifts (Domeignoz-Horta et al., 2023). Compared to β-glucosidase, there was less evidence of an effect of pre-incubation temperature on the potential enzyme activity of chitinase (no significance of pre-incubation as a main effect). The potential activity of an enzyme in soil is a function of production versus turnover rate. Accordingly, the balance between these two processes, for β-glucosidase, must have been differentially influenced by pre-incubation temperature, probably both directly and indirectly via, for example, reduced microbial biomass, and complex enzyme regulation in response to altered C availability relative to other nutrients (Allison and Vitousek, 2005; de Almeida et al., 2015).
Whilst pre-incubation at 26 ∘C reduced the rate of intracellular metabolic processes, it did not lead to an alteration of community intracellular temperature response traits (i.e. the shape of the temperature response) as evidenced by the non-significant interaction between pre-incubation and assay temperature or a pre-incubation effect on temperature sensitivity as evaluated by calculation of Q10 (Fig. 3) or Ea (Fig. 4). This result agrees with another study that showed minimal adaptation of the temperature response (of microbial growth) to pre-incubation temperature when the temperature was below the initial optimum (∼ 30 ∘C) for the mesophilic soil community (Bárcenas-Moreno et al., 2009), although pre-incubation above the optimum led to corresponding increases in the optimum for microbial growth. Minimal adaptive response to pre-incubation substantially below the initial optimum (i.e. 5 and 15 ∘C in our study) is explained in terms of a rate of species sorting (ultimately favouring a community better adapted to the pre-incubation conditions) being too slow to manifest within the 60 d pre-incubation period due to slow microbial generation times at colder temperatures (Bárcenas-Moreno et al., 2009). In contrast to intracellular metabolic processes, there was some evidence (significant interaction between pre-incubation temperature and assay temperature) of a pre-incubation effect on the temperature response for potential extracellular enzyme activity, although effects were quite subtle and only systematic with pre-incubation temperature for chitinase where activity assayed at 37 ∘C decreased in the order of decreasing pre-incubation temperature (also discernible in effects of pre-incubation on and Ea). The lower Ea for chitinase for soil samples pre-incubated at the lower temperatures is consistent with the concept that cold adaptation of microorganisms leads to the production of cold-adapted enzymes, by adjustment of chemical structure of the active site, with lower activation energies (Wallenstein and Burns, 2011). However, the few previous experimental studies examining the temperature response and/or sensitivity of extracellular enzyme potentials in soils exposed to differing thermal regimes have suggested no difference in temperature sensitivity (Schindlbacher et al., 2015; Jing et al., 2019) and therefore an absence of thermal adaptation of temperature sensitivity. However, these experiments involved long-term field-based warming treatments, and it is suggested that the effects of the experimental warming were negligible against the effects of wide seasonal temperature variations (Jing et al., 2019). Other studies, however, have demonstrated seasonal changes in temperature sensitivity of extracellular enzymes (Wallenstein and Burns, 2011; Wallenstein et al., 2009). These changes likely result from temporal changes in production of isoenzymes (by different organisms or within the same organism transcribing alternative enzyme-encoding genes), but whether these patterns represent an adaptation to seasonally varying temperature or are driven by other factors that change seasonally (e.g. substrate availability) is not clear (Wallenstein and Burns, 2011).
Our results advance understanding of how SOM decomposition will change under future global warming conditions. We show that the potential rates of the intracellular and extracellular steps of SOM decomposition are not equally sensitive to changes in temperature and that individual extracellular enzymes have different temperature sensitivities. Specifically, for our individual grassland soil pre-incubated at just three representative temperatures, we have demonstrated that potential activities of extracellular depolymerase enzymes (β-glucosidase and chitinase) have greater sensitivity to increases in temperature in the range of temperatures experienced on extremely warm days (between 26 and 37 ∘C) than the temperature sensitivity of intracellular metabolic processes involved in the catabolism of monomeric (e.g. glucose) substrates to CO2. Since a greater prevalence of extremely hot days and heatwaves are predicted, the importance of intracellular metabolic processes may increase, and the importance of extracellular enzyme activity may decrease as the rate-limiting step in SOM decomposition.
For the extracellular enzymes studied, we found differential temperature sensitivity with respect to enzyme class. Here, the implications are for temperature-dependent variation in the quality of monomeric SOM substrates supplying respiration and potential feedbacks to the soil microbial community composition, given taxa-specific competitive utilization of substrates (Wallenstein and Burns, 2011). Whilst interpretation should be within the context of the pre-incubation conditions (60 d at temperatures less than the optimum for activity of the mesophilic community), we have also shown that the thermal history (i.e. pre-incubation temperature) of a soil might modulate the relative responses in reaction rates to current temperature. This is both through enzyme-dependent reduction in potential activity across assay temperatures in 26 ∘C pre-incubated soil (for intracellular enzymes and β-glucosidase but not chitinase) and also subtle adaptation of the temperature response trait to pre-incubation temperature (for extracellular enzymes but not intracellular metabolic processes). Measurements of CO2 alone as a response variable while studying the effect of warming may obscure our understanding of the temperature sensitivity of the intracellular and extracellular steps of SOM decomposition.
Data associated with this publication have been uploaded to the Mendeley Data repository: https://doi.org/10.17632/xvr3dzvdcw.2 (Adekanmbi et al., 2023).
The supplement related to this article is available online at: https://doi.org/10.5194/bg-20-2207-2023-supplement.
AAA, LD, LS, and TS conceptualized the research and designed the experiment. AAA and LD carried out the laboratory work. AAA and LD analysed the data with support from TS and LS. AAA and LD undertook data visualization. AAA wrote the original draft of the paper. TS, LS, LD, and AAA undertook subsequent reviewing and editing. TS supervised the project, and LS co-supervised the project.
The contact author has declared that none of the authors has any competing interests.
Publisher's note: Copernicus Publications remains neutral with regard to jurisdictional claims in published maps and institutional affiliations.
This article is part of the special issue “Global change effects on terrestrial biogeochemistry at the plant–soil interface”. It is not associated with a conference.
The authors gratefully acknowledge the Technical Services staff within the Environmental Science Research Division at the University of Reading for technical support and assistance in this work.
This research has been supported by the Commonwealth Scholarship Commission (grant no. NGCS-2017-416).
This paper was edited by Alberto Canarini and reviewed by two anonymous referees.
Adekanmbi, A. A., Shaw, L. J., and Sizmur, T.: Effect of Sieving on Ex Situ Soil Respiration of Soils from Three Land Use Types, J. Soil Sci. Plant Nut., 20, 912–916, https://doi.org/10.1007/s42729-020-00177-2, 2020.
Adekanmbi, A. A., Dale, L., Shaw, L., and Sizmur, T.: Data for: Differential Temperature Sensitivity of Intracellular and Extracellular Soil Enzyme Activities, V2, Mendeley Data [data set], https://doi.org/10.17632/xvr3dzvdcw.2, 2023.
Adeli, A., Sistani, K. R., Bal'a, M. F., and Rowe, D. E.: Phosphorus Dynamics in Broiler Litter-Amended Soils, Commun. Soil Sci. Plan., 36, 1099–1115, https://doi.org/10.1081/CSS-200056876, 2005.
Allison, S. D. and Vitousek, P. M.: Responses of extracellular enzymes to simple and complex nutrient inputs, Soil Biol. Biochem., 37, 937–944, https://doi.org/10.1016/j.soilbio.2004.09.014, 2005.
Alster, C. J., Baas, P., Wallenstein, M. D., Johnson, N. G., and von Fischer, J. C.: Temperature sensitivity as a microbial trait using parameters from macromolecular rate theory, Front. Microbiol., 7, 1–10, https://doi.org/10.3389/fmicb.2016.01821, 2016.
Alster, C. J., von Fischer, J. C., Allison, S. D., and Treseder, K. K.: Embracing a new paradigm for temperature sensitivity of soil microbes., Glob. Change Biol., 26, 3221–3229, https://doi.org/10.1111/gcb.15053, 2020.
Alvarez, G., Shahzad, T., Andanson, L., Bahn, M., Wallenstein, M. D., and Fontaine, S.: Catalytic power of enzymes decreases with temperature: new insights for understanding soil C cycling and microbial ecology under warming, Glob. Change Biol., 24, 4238–4250, https://doi.org/10.1111/gcb.14281, 2018.
Bárcenas-Moreno, G., Brandón, M. G., Rousk, J., and Bååth, E.: Adaptation of soil microbial communities to temperature: Comparison of fungi and bacteria in a laboratory experiment, Glob. Change Biol., 15, 2950–2957, https://doi.org/10.1111/j.1365-2486.2009.01882.x, 2009.
Bárta, J., Šlajsová, P., Tahovska, K., Picek, T., and Santruckova, H.: Different temperature sensitivity and kinetics of soil enzymes indicate seasonal shifts in C, N and P nutrient stoichiometry in acid forest soil, Biogeochemistry, 117, 525–537, https://doi.org/10.1007/s10533-013-9898-1, 2014.
Birgander, J., Reischke, S., Jones, D. L., and Rousk, J.: Temperature adaptation of bacterial growth and 14C-glucose mineralisation in a laboratory study, Soil Biol. Biochem., 65, 294–303, https://doi.org/10.1016/j.soilbio.2013.06.006, 2013.
Blagodatskaya, E., Blagodatsky, S., Khomyakov, N., Myachina, O., and Kuzyakov, Y.: Temperature sensitivity and enzymatic mechanisms of soil organic matter decomposition along an altitudinal gradient on Mount Kilimanjaro, Sci. Rep., 6, 1–11, https://doi.org/10.1038/srep22240, 2016.
Bradford, M. A.: Thermal adaptation of decomposer communities in warming soils, Front. Microbiol., 4, 1–16, https://doi.org/10.3389/fmicb.2013.00333, 2013.
Christidis, N., Jones, G. S., and Stott, P. A.: Dramatically increasing chance of extremely hot summers since the 2003 European heatwave, Nat. Clim. Change, 5, 46–50, https://doi.org/10.1038/nclimate2468, 2015.
Cook, S., Whelan, M. J., Evans, C. D., Gauci, V., Peacock, M., Garnett, M. H., Kho, L. K., Teh, Y. A., and Page, S. E.: Fluvial organic carbon fluxes from oil palm plantations on tropical peatland, Biogeosciences, 15, 7435–7450, https://doi.org/10.5194/bg-15-7435-2018, 2018.
Davidson, E. A. and Janssens, I. A.: Temperature sensitivity of soil carbon decomposition and feedbacks to climate change, Nature, 440, 165–173, https://doi.org/10.1038/nature04514, 2006.
de Almeida, R. F., Naves, E. R., and da Mota, R. P.: Soil quality: Enzymatic activity of soil β-glucosidase, Global Journal of Agricultural Research and Reviews, 3, 146–150, 2015.
Domeignoz‐Horta, L. A., Pold, G., Erb, H., Sebag, D., Verrecchia, E., Northen, T., Louie, K., Eloe‐Fadrosh, E., Pennacchio, C., Knorr, M. A., and Frey, S. D.: Substrate availability and not thermal acclimation controls microbial temperature sensitivity response to long‐term warming, Glob. Change Biol., 29, 1574–1590, 2023.
Du, J., Duan, S., Miao, J., Zhai, M., and Cao, Y.: Purification and characterization of chitinase from Paenibacillus sp., Biotechnol. Appl. Bioc., 68, 30–40, https://doi.org/10.1002/bab.1889, 2021.
Duly, O. and Nannipieri, P.: Intracellular and extracellular enzyme activity in soil with reference to elemental cycling, Z. Pflanz. Bodenkunde, 161, 243–248, https://doi.org/10.1002/jpln.1998.3581610310, 1998.
Eivazi, F. and Tabatabai, M. A.: Glucosidases and Galactosidases in Soils, Soil Biol. Biochem., 20, 601–606, https://doi.org/10.1016/0038-0717(88)90141-1, 1988.
Evans, C. D., Page, S. E., Jones, T., Moore, S., Gauci, V., Laiho, R., Hruska, J., Allot, T. E., Billet, M. F., Tipping, E., Freeman, C., and Garnett, M. H.: Contrasting vulnerability of drained tropical and high-latitude peatlands to fluvial loss of stored carbon, Global Biogeochem. Cy., 28, 1215–1234, https://doi.org/10.1002/2013GB004782, 2014.
Fanin, N., Mooshammer, M., Sauvadet, M., Meng, C., Alvarez, G., Bernard, L., Bertrand, I., Blagodatskaya, E., Bon, L., Fontaine, S., Niu, S., Lashermes, G., Maxwell, T. L., Weintraub, M. N., Wingate, L., Moorhead, D., and Nottingham, A. T.: Soil enzymes in response to climate warming: Mechanisms and feedbacks, Funct. Ecol., 36, 1378–1395, https://doi.org/10.1111/1365-2435.14027, 2022.
Gao, X. A., Ju, W. T., Jung, W. J., and Park, R. D.: Purification and characterization of chitosanase from Bacillus cereus D-11, Carbohyd. Polym., 72, 513–520, https://doi.org/10.1016/j.carbpol.2007.09.025, 2008.
Hamdi, S., Moyano, F., Sall, S., Bernoux, M., and Chevallier, T.: Synthesis analysis of the temperature sensitivity of soil respiration from laboratory studies in relation to incubation methods and soil conditions, Soil Biol. Biochem., 58, 115–126, https://doi.org/10.1016/j.soilbio.2012.11.012, 2013.
Howard, D. M. and Howard, P. J. A.: Relationships between CO2 evolution, moisture content and temperature for a range of soil types, Soil Biol. Biochem., 25, 1537–1546, https://doi.org/10.1016/0038-0717(93)90008-Y, 1993.
Jan, M. T., Roberts, P., Tonheim, S. K., and Jones, D. L.: Protein breakdown represents a major bottleneck in nitrogen cycling in grassland soils, Soil Biol. Biochem., 41, 2272–2282, https://doi.org/10.1016/j.soilbio.2009.08.013, 2009.
Jing, X., Wang, Y., Chung, H., Mi, Z., Wang, S., Jing, X., Wang, Y., and Chung, H.: No temperature acclimation of soil extracellular enzymes to experimental warming in an alpine grassland ecosystem on the Tibetan Plateau, Biogeochemistry, 117, 39–54, https://doi.org/10.1007/s10533-013-9844-2, 2019.
Karhu, K., Auffret, M. D., Dungait, J. A. J., Hopkins, D. W., Prosser, J. I., Singh, B. K., Subke, J. A., Wookey, P. A., Agren, G. I., Sebastià, M. T., Gouriveau, F., Bergkvist, G., Meir, P., Nottingham, A. T., Salinas, N., and Hartley, I. P.: Temperature sensitivity of soil respiration rates enhanced by microbial community response, Nature, 513, 81–84, https://doi.org/10.1038/nature13604, 2014.
Kirwan, M. L., Guntenspergen, G. R., and Langley, J. A.: Temperature sensitivity of organic-matter decay in tidal marshes, Biogeosciences, 11, 4801–4808, https://doi.org/10.5194/bg-11-4801-2014, 2014.
Li, J., He, N., Wei, X., Gao, Y., and Zuo, Y.: Changes in temperature sensitivity and activation energy of soil organic matter decomposition in different Qinghai-Tibet Plateau grasslands, PLoS One, 10, 1–14, https://doi.org/10.1371/journal.pone.0132795, 2015.
Maire, V., Alvarez, G., Colombet, J., Comby, A., Despinasse, R., Dubreucq, E., Joly, M., Lehours, A.-C., Perrier, V., Shahzad, T., and Fontaine, S.: An unknown oxidative metabolism substantially contributes to soil CO2 emissions, Biogeosciences, 10, 1155–1167, https://doi.org/10.5194/bg-10-1155-2013, 2013.
Meehl, G. A. and Tebaldi, C.: More intense, more frequent, and longer lasting heat waves in the 21st century, Science, 305, 994–997, https://doi.org/10.1126/science.1098704, 2004.
Min, K., Lehmeier, C. A., Ballantyne, F., Tatarko, A., and Billings, S. A.: Differential effects of pH on temperature sensitivity of organic carbon and nitrogen decay, Soil Biol. Biochem., 76, 193–200, https://doi.org/10.1016/j.soilbio.2014.05.021, 2014.
Min, K., Bagchi, S., Buckeridge, K., Billings, S. A., Ziegler, S. E., and Edwards, K. A.: Temperature sensitivity of biomass-specific microbial exo-enzyme activities and CO2 efflux is resistant to change across short- and long-term timescales, Glob. Change Biol., 25, 1793–1807, https://doi.org/10.1111/gcb.14605, 2019.
Niklińska, M. and Klimek, B.: Effect of temperature on the respiration rate of forest soil organic layer along an elevation gradient in the Polish Carpathians, Biol. Fert. Soils, 43, 511–518, https://doi.org/10.1007/s00374-006-0129-y, 2007.
Nottingham, A. T., Turner, B. L., Whitaker, J., Ostle, N., Bardgett, R. D., McNamara, N. P., Salinas, N., and Meir, P.: Temperature sensitivity of soil enzymes along an elevation gradient in the Peruvian Andes, Biogeochemistry, 127, 217–230, https://doi.org/10.1007/s10533-015-0176-2, 2016.
Parham, J. A. and Deng, S. P.: Detection, quantication and characterization of b-glucosaminidase activity in soil, Soil Biol. Biochem., 32, 1183–1190, https://doi.org/10.1016/S0038-0717(00)00034-1, 2000.
Pietikäinen, J., Pettersson, M., and Bååth, E.: Comparison of temperature effects on soil respiration and bacterial and fungal growth rates, FEMS Microbiol. Ecol., 52, 49–58, https://doi.org/10.1016/j.femsec.2004.10.002, 2005.
Prentice, E. J., Hicks, J., Ballerstedt, H., Blank, L. M., Liang, L. L., Schipper, L. A., and Arcus, V. L.: The inflection point hypothesis: The relationship between the temperature dependence of enzyme-catalyzed reaction rates and microbial growth rates, Biochemistry, 59, 3562–3569, https://doi.org/10.1021/acs.biochem.0c00530, 2020.
Puissant, J., Jones, B., Goodall, T., Mang, D., Blaud, A., Gweon, H. S., Malik, A., Jones, D. L., Clark, I. M., Hirsch, P. R., and Griffiths, R.: The pH optimum of soil exoenzymes adapt to long term changes in soil pH, Soil Biol. Biochem., 138, 107601, https://doi.org/10.1016/j.soilbio.2019.107601, 2019.
Rodriguez-Kabana, R., Godoy, G., Morgan-Jones, G., and Shelby, R. A.: The determination of soil chitinase activity: Conditions for assay and ecological studies, Plant Soil, 75, 95–106, 1983.
Salazar-Villegas, A., Blagodatskaya, E., and Dukes, J. S.: Changes in the size of the active microbial pool explain short-term soil respiratory responses to temperature and moisture, Front. Microbiol., 7, 1–10, https://doi.org/10.3389/fmicb.2016.00524, 2016.
Sarkar, J. M., Leonowicz, A., and Bollag, J. M.: Immobilization of enzymes on clays and soils, Soil Biol. Biochem., 21, 223–230, https://doi.org/10.1016/0038-0717(89)90098-9, 1989.
Schindlbacher, A., Schnecker, J., Takriti, M., Borken, W., and Wanek, W.: Microbial physiology and soil CO2 efflux after 9 years of soil warming in a temperate forest – no indications for thermal adaptations, Glob. Change Biol., 21, 4265–4277, https://doi.org/10.1111/gcb.12996, 2015.
Schulte, P. M.: The effects of temperature on aerobic metabolism: Towards a mechanistic understanding of the responses of ectotherms to a changing environment, J. Exp. Biol., 218, 1856–1866, https://doi.org/10.1242/jeb.118851, 2015.
Sinsabaugh, R. L., Antibus, R. K., and Linkins, A. E.: An enzymic approach to the analysis of microbial activity during plant litter decomposition, Agr. Ecosyst. Environ., 34, 43–54, https://doi.org/10.1016/0167-8809(91)90092-C, 1991.
Sinsabaugh, R. L., Lauber, C. L., Weintraub, M. N., Ahmed, B., Allison, S. D., Crenshaw, C., Contosta, A. R., Cusack, D., Frey, S., Gallo, M. E., Gartner, T. B., Hobbie, S. E., Holland, K., Keeler, B. L., Powers, J. S., Stursova, M., Takacs-Vesbach, C., Waldrop, M. P., Wallenstein, M. D., Zak, D. R., and Zeglin, L. H.: Stoichiometry of soil enzyme activity at global scale, Ecol. Lett., 11, 1252–1264, https://doi.org/10.1111/j.1461-0248.2008.01245.x, 2008.
Sinsabaugh, R. S.: Enzymic analysis of microbial pattern and process, Biol. Fert. Soils, 17, 69–74, https://doi.org/10.1007/BF00418675, 1994.
Souza, L. F. T. and Billings, S. A.: Temperature and pH mediate stoichiometric constraints of organically derived soil nutrients, Glob. Change Biol., 28, 1630–1642, https://doi.org/10.1111/gcb.15985, 2022.
Steinweg, M. J., Jagadamma, S., Frerichs, J., and Mayes, M. A.: Activation Energy of Extracellular Enzymes in Soils from Different Biomes, PLoS One, 8, e59943, https://doi.org/10.1371/journal.pone.0059943, 2013.
Thakur, N., Nath, A. K., Chauhan, A., and Gupta, R.: Purification, characterization, and antifungal activity of Bacillus cereus strain NK91 chitinase from rhizospheric soil samples of Himachal Pradesh, India, Biotechnol. Appl. Bioc., 69, 1830–1842, https://doi.org/10.1002/bab.2250, 2021.
Todd-Brown, K., Hopkins, F. M., Kivlin, S. N., Talbot, J. M., and Allison, S. D.: A framework for representing microbial decomposition in coupled climate models, Biogeochemistry, 109, 19–33, https://doi.org/10.1007/s10533-011-9635-6, 2012.
Trasar-Cepeda, C., Gil-Sotres, F., and Leirós, M. C.: Thermodynamic parameters of enzymes in grassland soils from Galicia, NW Spain, Soil Biol. Biochem., 39, 311–319, https://doi.org/10.1016/j.soilbio.2006.08.002, 2007.
Vance, E. D., Brookes, P. C., and Jenkinson, D. S.: An Extraction method for measuring Soil Microbial Biomass C, Soil Biol. Biochem., 19, 703–707, https://doi.org/10.1016/0038-0717(87)90052-6, 1987.
Wallenstein, M., Allison, S. D., Ernakovich, J., Steinweg, J. M., and Sinsabaugh, R.: Controls on the Temperature Sensitivity of Soil Enzymes: A Key Driver of In Situ Enzyme Activity Rates, in: Soil Enzymology, edited by: Shukla, G. and Varma, A., Springer Berlin Heidelberg, Berlin, Heidelberg, 245–258, https://doi.org/10.1007/978-3-642-14225-3_13, 2010.
Wallenstein, M. D. and Burns, R. G.: Ecology of extracellular enzyme activities and organic matter degradation in soil: a complex community‐driven process, Methods of soil enzymology, 9, 35–55, 2011.
Wallenstein, M. D. and Weintraub, M. N.: Emerging tools for measuring and modeling the in situ activity of soil extracellular enzymes, Soil Biol. Biochem., 40, 2098–2106, https://doi.org/10.1016/j.soilbio.2008.01.024, 2008.
Wallenstein, M. D., McMahon, S. K., and Schimel, J. P.: Seasonal variation in enzyme activities and temperature sensitivities in Arctic tundra soils, Glob. Change Biol., 15, 1631–1639, 2009.
Wang, G., Zhou, Y., Xu, X., Ruan, H., and Wang, J.: Temperature Sensitivity of Soil Organic Carbon Mineralization along an Elevation Gradient in the Wuyi Mountains, China, PLoS One, 8, e53914, https://doi.org/10.1371/journal.pone.0053914, 2013.
Wei, L., Zhu, Z., Liu, S., Xiao, M., Wang, J., Deng, Y., Kuzyakov, Y., Wu, J., and Ge, T.: Temperature sensitivity (Q10) of stable, primed and easily available organic matter pools during decomposition in paddy soil, Appl. Soil Ecol., 157, 103752, https://doi.org/10.1016/j.apsoil.2020.103752, 2021.