the Creative Commons Attribution 4.0 License.
the Creative Commons Attribution 4.0 License.
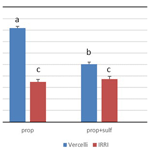
Fractionation of stable carbon isotopes during microbial propionate consumption in anoxic rice paddy soils
Peter Claus
Propionate is an important intermediate during the breakdown of organic matter in anoxic flooded paddy soils. Since there are only a few experiments on carbon isotope fractionation and the magnitude of the isotopic enrichment factors (ε) involved, we measured propionate conversion to acetate, CH4 and CO2 in anoxic paddy soils. Propionate consumption was measured using samples of paddy soil from Vercelli (Italy) and the International Rice Research Institute (IRRI, the Philippines) suspended in a phosphate buffer (pH 7.0) both in the absence and presence of sulfate (gypsum) and of methyl fluoride (CH3F), an inhibitor of aceticlastic methanogenesis. Under methanogenic conditions, propionate was eventually degraded to CH4, with acetate being a transient intermediate. Butyrate was also a minor intermediate. Methane was mainly produced by aceticlastic methanogenesis. Propionate consumption was inhibited by CH3F. Butyrate and CH4 were 13C-depleted relative to propionate, whereas acetate and CO2 were 13C-enriched. The isotopic enrichment factors (εprop) of propionate consumption, determined by Mariotti plots, were in a range of −8 ‰ to −3.5 ‰. Under sulfidogenic conditions, acetate was also transiently accumulated, but CH4 production was negligible. Application of CH3F hardly affected propionate degradation and acetate accumulation. The initially produced CO2 was 13C-depleted, whereas the acetate was 13C-enriched. The values of εprop were −3.5 ‰. It is concluded that the degradation of organic carbon via propionate to acetate and CO2 involves only a little isotope fractionation. The results further indicate a major contribution of Syntrophobacter-type propionate fermentation under sulfidogenic conditions and Smithella-type propionate fermentation under methanogenic conditions. This interpretation is consistent with data regarding the microbial community composition published previously for the same soils.
- Article
(1459 KB) - Full-text XML
-
Supplement
(864 KB) - BibTeX
- EndNote
Propionate is a common intermediate of organic matter degradation in anoxic paddy soils. In the absence of sulfate reduction or methanogenesis, propionate may accumulate to millimolar concentrations (Conrad et al., 2014; Glissmann and Conrad, 2000; Nozoe, 1997). Under methanogenic conditions, propionate is degraded by fermentation. Several different biochemical pathways are conceivable for propionate fermentation (Textor et al., 1997). The major fermentation pathways are those by Syntrophobacter (Boone and Bryant, 1980) and Smithella (Liu et al., 1999), both members of Deltaproteobacteria. Syntrophobacter operates the methylmalonyl-CoA pathway, which results in randomization of the carbon positions of propionate (Houwen et al., 1991). This pathway can also be found in Desulfotomaculum sp. and Pelotomaculum sp. (Chen et al., 2005; DeBok et al., 2005; Imachi et al., 2002; Plugge et al., 2002) and apparently exists in many anoxic environments (Imachi et al., 2006; Krylova et al., 1997; Schink, 1985). Smithella, on the other hand, operates a dismutation pathway, which does not result in randomization (DeBok et al., 2001). This pathway has also been found in many anoxic environments (Gan et al., 2012; Lueders et al., 2004; Xia et al., 2019).
Propionate degradation by randomizing Syntrophobacter proceeds via succinate in the following way:
Propionate degradation by non-randomizing Smithella proceeds by dismutation of propionate:
Butyrate is then syntrophically converted (e.g., by Syntrophomonas; McInerney et al., 1981):
The Smithella pathway, in total, is then
Propionate fermentation is thermodynamically endergonic under standard conditions and therefore requires syntrophic microbial partners that further convert the fermentation products. Under methanogenic conditions, the syntrophic partners are methanogenic archaea, which consume the products acetate and H2. Under sulfidogenic conditions, sulfate-reducing bacteria replace the methanogens. Propionate can also be directly oxidized to CO2 by propionate-degrading sulfate reducers. The overall reaction stoichiometry is the same for Syntrophobacter and Smithella:
Note that the relative production of acetate and H2 is different for Syntrophobacter and Smithella fermentation, being 1:3 and 3:2, respectively. Therefore, aceticlastic methanogenesis contributes relatively more than hydrogenotrophic methanogenesis when propionate is fermented by Smithella rather than Syntrophobacter. Under methanogenic conditions, propionate degradation in anoxic paddy soils operates close to the thermodynamic limits (Krylova and Conrad, 1998; Yao and Conrad, 2001). These restrictions are more severe for Syntrophobacter than for Smithella (Dolfing, 2013).
Using paddy soil from Italy and the Philippines, Liu and their coworkers (Liu et al., 2018a; Liu and Conrad, 2017) have recently shown that propionate consumption under sulfidogenic conditions is mainly achieved by Syntrophobacter species or other Syntrophobacteraceae, which first oxidize propionate to acetate and CO2 and subsequently oxidize the accumulated acetate to CO2. They also showed that Smithella was probably involved in methanogenic propionate degradation. The involvement of Smithella has also been shown for other paddy soils and sediments (Gan et al., 2012; Lueders et al., 2004; Xia et al., 2019). Since we used in the present study the same soils as Liu and their coworkers (Liu et al., 2018a; Liu and Conrad, 2017), we assumed that propionate degradation was achieved by the same microorganisms.
Knowledge of carbon isotope fractionation is important for the assessment of the pathways involved in anaerobic degradation of organic matter (Conrad, 2005; Elsner et al., 2005). The δ13C values of organic carbon, acetate and propionate in various soils and sediments were found to be similar (Conrad et al., 2014). The similarity indicates that the enrichment factors (ε) of the processes involved in both the production and consumption of propionate are probably small. The direct determination of ε values in microbial cultures of one propionate-producing bacterium and one propionate-consuming bacterium also showed low values (Botsch and Conrad, 2011). However, direct determination of ε values in environmental samples is missing. Therefore, we decided to measure isotope fractionation in methanogenic and sulfidogenic paddy soil amended with propionate along with the recording of the production of acetate, CH4 and CO2. We also used the treatment with methyl fluoride (CH3F) to inhibit the consumption of acetate by methanogenic archaea (Janssen and Frenzel, 1997). Recently, we determined the microbial communities in methanogenic and sulfidogenic rice field soils, which were used for the assessment of 13C isotope fractionation during acetate consumption (Conrad et al., 2021). Here, we present analogous data from the same soil suspensions prepared for the propionate degradation experiments.
2.1 Paddy soils and incubation conditions
The soil samples were from the research stations in Vercelli, Italy, and the International Rice research Institute (IRRI) in the Philippines. The sampling and soil characteristics have been described before (Liu et al., 2018b). The main soil characteristics will be given. The Italian soil is a sandy loam with a pH of 5.75, total C of 1.1 % and total N of 0.08 %. The Philippine soil is a silt loam with a pH of 6.3, total C of 1.9 % and total N of 0.2 %.
The experimental setup was exactly the same as during a previous study on acetate consumption (Conrad et al., 2021). Paddy soil was mixed with autoclaved anoxic H2O at a ratio of 1:1 and incubated under N2 at 25 ∘C for 4 weeks. In a second incubation, paddy soil was mixed with autoclaved anoxic H2O (prepared under N2) at a ratio of 1:1, was amended with 0.07 g CaSO4 ⋅ 2H2O and was then incubated under N2 at 25 ∘C for 4 weeks. These two preincubated soil slurries were sampled and stored at −20 ∘C for later molecular analysis (see data in Conrad et al., 2021). The preincubated soil slurries were also used (in three replicates) for the following incubation experiments. Two different sets of incubations were prepared. In the first set (resulting in methanogenic conditions), 5 mL of soil slurry preincubated without sulfate was incubated at 25 ∘C with 40 mL of 20 mM potassium phosphate buffer (pH 7.0) in a 150 mL bottle under an atmosphere of N2. The bottles were then amended with (i) 5 mL H2O, (ii) 5 mL H2O + 4.5 mL CH3F, (iii) 5 mL 50 mM sodium propionate, and (iv) 5 mL 50 mM sodium propionate + 4.5 mL CH3F. In the second set (resulting in sulfidogenic conditions), 5 mL of soil slurry preincubated with sulfate was incubated at 25 ∘C with 40 mL of 20 mM potassium phosphate buffer (pH 7.0) in a 150 mL bottle under an atmosphere of N2. The amendments were the same as above but with the addition of 200 µl of a CaSO4 suspension corresponding to a concentration of 2.5 M (giving a final concentration of 10 mM sulfate).
2.2 Chemical and isotopic analyses
Gas samples for analysis of partial pressures of CH4 and CO2 were taken from the headspace of the incubation bottles after vigorous manual shaking for about 30 s using a gas-tight pressure-lock syringe, which had been flushed with N2 before each sampling. Soil slurries were sampled, centrifuged and filtered through a 0.2 µm cellulose membrane filter and were stored frozen at −20 ∘C for later fatty acid analysis. Chemical and isotopic analyses were performed as described in detail previously (Goevert and Conrad, 2009). Methane was analyzed by gas chromatography (GC) with a flame ionization detector. Carbon dioxide was analyzed after conversion to CH4 with a Ni catalyst. Stable isotope analyses of in gas samples were performed using GC-combustion isotope ratio mass spectrometry (GC-C-IRMS). Propionate, butyrate and acetate were measured using high-performance liquid chromatography (HPLC) linked via a Finnigan LC IsoLink to an IRMS. The isotopic values are reported in the delta notation (δ13C) relative to the Vienna PeeDee Belemnite standard with a ratio (Rstandard) of 0.01118: δ13C = . The precision of the GC-C-IRMS was ± 0.2 ‰, and that of the HPLC-IRMS was ± 0.3 ‰.
2.3 Calculations
Millimolar concentrations of CH4 were calculated from the mixing ratios (1 ppmv ± 10−6 bar) measured in the gas phase of the incubation bottles: 1000 ppmv CH4 correspond to 0.09 µmol mL−1 of liquid. Note that this is the total amount of CH4 in the gas phase relative to the liquid phase.
Fractionation factors for reaction A → B are defined after Hayes (Hayes, 1993) as follows:
also expressed as ε ≡ 1000(1−α) (in ‰). The carbon isotope enrichment factor εprop associated with propionate consumption was calculated from the temporal change in δ13C of propionate as described by Mariotti et al. (Mariotti et al., 1981) from the residual reactant
where δri is the isotopic composition of the reactant (propionate) at the beginning, and δr is the isotopic composition of the residual propionate; both of these are indicated at the instant when f is determined. fprop is the fractional yield of the products based on the consumption of propionate (). Linear regression of δ13C of propionate against ln(1−f) yields εprop as the slope of the best-fit lines. The regressions of δ13C of propionate were done for data in the range of fprop<0.7. The linear regressions were done individually for each experimental replicate (n=3) and were only accepted if r2>0.9. The ε values resulting from the replicate experiments were then averaged (± SE).
The fraction () of CH4 derived from hydrogenotrophic methanogenesis was determined as described before (Conrad et al., 2010) using
where = δ13C of the total CH4 produced; = δ13C of CH4 produced from hydrogenotrophic methanogenesis, which is equivalent to the CH4 produced in the presence of CH3F; and = δ13C of CH4 produced from aceticlastic methanogenesis. The was approximated from the δ13C of acetate in the presence of CH3F assuming that the methyl group of acetate was depleted in 13C by 8 ‰ (Conrad et al., 2014) and that the enrichment factor () for CH4 being produced from acetate–methyl was between 0 and −20 ‰.
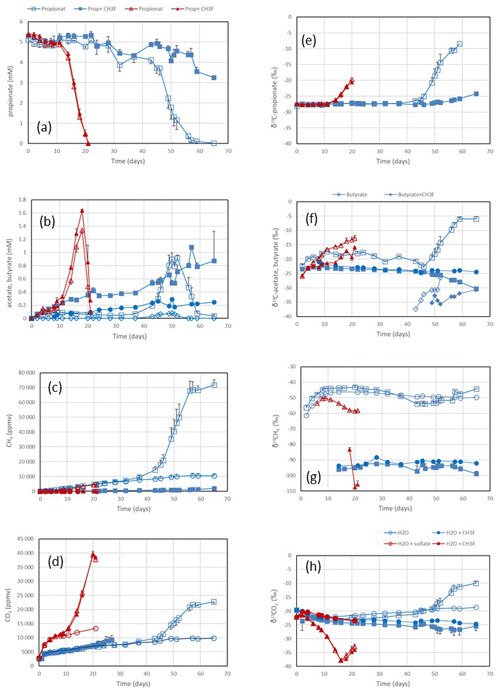
Figure 1Propionate conversion to acetate, butyrate, CH4 and CO2 in suspensions of paddy soil from Vercelli (Italy) after addition of propionate without sulfate (blue squares) or of propionate plus sulfate (gypsum) (red triangles) without CH3F (open symbols) or with CH3F (closed symbols). Controls with the addition of water only (blue or red circles) are only shown occasionally. The panels show the temporal change in (a) concentrations of propionate, (b) concentrations of acetate and butyrate (blue diamonds), (c) mixing ratios of CH4 (1 ppmv = 10−6 bar), (d) mixing ratios of CO2, (e) δ13C of propionate, (f) δ13C of acetate and butyrate, (g) δ13C of CH4, and (h) δ13C of CO2. Shown are the means ± SE (standard error).
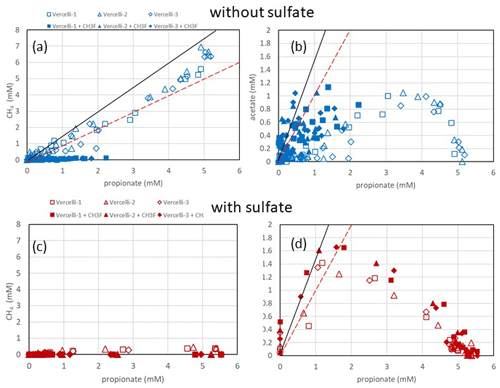
Figure 2Balance of (a, c) produced CH4 and (b, d) produced acetate against the consumed propionate under (a, b) methanogenic and (c, d) sulfidogenic conditions in paddy soil from Vercelli (Italy). The open and closed symbols denote conditions in the absence and the presence of CH3F, respectively. The black and red lines in (a) indicate aceticlastic methanogenesis after generation of acetate by either Smithella (Eq. 4) or Syntrophobacter (Eq. 1). The black and red lines in (b) and (d) indicate transient acetate production by Smithella and Syntrophobacter, respectively. The different symbols indicate three different replicates.
3.1 Conversion of propionate under methanogenic and sulfidogenic conditions
Incubation of buffered suspensions of rice field soil from Vercelli (Fig. 1) and the IRRI (Fig. S1 in the Supplement) resulted in similar patterns of propionate degradation to acetate, CH4 and CO2. Under methanogenic conditions in the absence of sulfate, propionate degradation started after a lag phase of about 20 d (Fig. 1a), resulting in the production of acetate (Fig. 1b), CH4 (Fig. 1c) and CO2 (Fig. 1d). The formation of acetate, CH4 and CO2 in the absence of propionate was only very small. The accumulation of acetate was only transient, except when aceticlastic methanogenesis was inhibited by CH3F (Fig. 1b). Similar observations were made in IRRI soil (Fig. S1a–d). The production of CH4 was roughly equimolar to the consumption of propionate but was nearly zero when aceticlastic methanogenesis was inhibited by CH3F (Fig. 2a). Under these conditions, acetate accumulated to nearly equimolar amounts with the consumed propionate (Fig. 2b), but in IRRI soil, acetate accumulation was less than equimolar (Fig. S2b in the Supplement). Butyrate was also a transient intermediate of propionate degradation and was produced and consumed simultaneously with acetate (Figs. 1b and S1b). However, the accumulated concentrations were small (< 0.1 mM).
In the presence of sulfate, propionate degradation started after a lag phase of only about 10 d (Fig. 1a), resulting in the accumulation of acetate (Fig. 1b) and the production of CO2 (Fig. 1d), but CH4 production was close to zero (Fig. 1c). Similar results were obtained with IRRI soil (Fig. S1a–d). The accumulated acetate was equimolar (slightly less than equimolar in the IRRI soil; Fig. S2d) to the consumption of propionate (Fig. 2d), but CH4 was not accumulated (Fig. 2c). The addition of CH3F had no effect. Butyrate was not detected. The accumulated acetate was subsequently degraded, resulting in further production of CO2 (Fig. 1b and d).
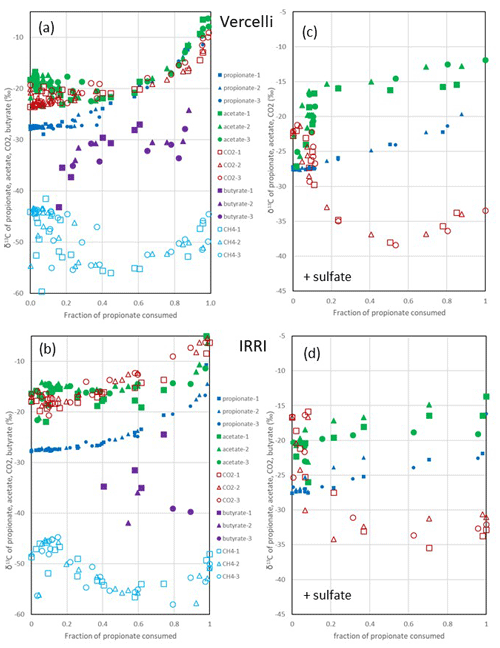
Figure 3Change in δ13C of propionate, acetate, butyrate, CO2 and CH4 relative to the fraction of propionate consumed (fprop) under (a, b) methanogenic and (c, d) sulfidogenic conditions in paddy soil from (a, c) Vercelli (Italy) and (b, d) the IRRI (the Philippines). The different symbols indicate three different replicates.
3.2 Isotope fractionation during propionate degradation
After the onset of propionate degradation, the δ13C of propionate (Fig. 1e) and acetate (Fig. 1f) increased, indicating that the light isotope was preferentially consumed. The δ13C values of CO2 also increased (Fig. 1h). The same was the case for butyrate (Fig. 1f). Similar results were obtained with IRRI soil (Fig. S1e–h). When aceticlastic methanogenesis was inhibited by CH3F, the δ13C values of these compounds increased only slightly or decreased (Fig. 1e, f and h). However, the δ13C of CH4 was much more negative (30–50 ‰) in the presence than in the absence of CH3F (Fig. 1g). The δ13C values of CH4 in unamended soil (H2O control) were similar to those in propionate amended soil (Fig. 1g). To visualize the change in the 13C content of the metabolic products relative to the substrates, the δ13C values were plotted against the increasing fractions (fprop) of propionate consumed in soil from both Vercelli (Fig. 3a) and the IRRI (Fig. 3b). The patterns of δ13C values against the fprop indicated kinetic isotope fractionation. Note that the δ13C values of acetate and CO2 were higher than those of propionate, whereas the values of butyrate and CH4 were lower (Fig. 3a and b). The δ13C of CH4 decreased until about 40 % of the propionate had been consumed and then increased again to its initial (low) values (−50 ‰ to −45 ‰) (Fig. 3a and b).
Under sulfidogenic conditions, only very little CH4 was produced. Similarly to that under methanogenic conditions, the δ13C of propionate (Fig. 1e) and of acetate (Fig. 1f) increased after the onset of propionate degradation, indicating that the light isotope was preferentially consumed. However, the δ13C values of CO2 decreased during the first 10–15 d when acetate was accumulated (Figs. 1h and S1h). Inhibition of aceticlastic methanogenesis by CH3F had no effect on the δ13C of propionate and CO2, but the values of acetate increased less than in the absence of CH3F (Fig. 1f). Also, δ13C of CH4 was lower in the presence than in the absence of CH3F (Fig. 1g), but the amounts of CH4 produced were only very small (Fig. 1c). The values of δ13C of propionate and acetate increased with increasing fprop (Fig. 3c and d). The δ13C of acetate was generally higher by about 5–10 ‰ than the δ13C of propionate but also increased with fprop, indicating kinetic isotope fractionation. However, the δ13C of CO2 did not increase; instead, it decreased after the onset of propionate degradation, reaching about −35 ‰ when 50 % of the propionate had been consumed and when acetate accumulation had reached a maximum (Fig. 3c and d). Thereafter, δ13C of CO2 increased or became constant.
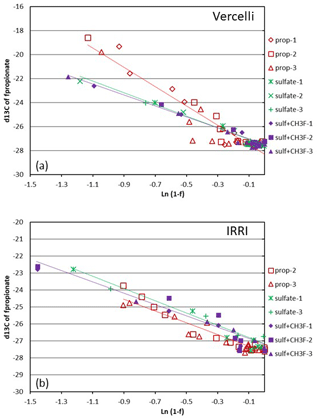
Figure 4Mariotti plots of propionate consumption under methanogenic and sulfidogenic (± CH3F) conditions in paddy soil from (a) Vercelli and (b) the IRRI. The different symbols indicate three different replicates; the lines give the results of linear regression averaged over the replicates.
Mariotti plots of the 13C of propionate as a function of fprop could be created for methanogenic and sulfidogenic incubation conditions, the latter being the case both in the absence and the presence of CH3F (Fig. 4). The lines were straight even when more than 70 % of the propionate was consumed. Nevertheless, enrichment factors (ε) were determined only for fprop < 0.7 and for regressions giving r2 > 0.9. The εprop values were determined for each individual incubation and then averaged over the replicates (n = 2–3). The results for Vercelli and IRRI soils are summarized in Fig. 5. The average εprop values under methanogenic conditions were about −8 ‰ for Vercelli and about −3.5 ‰ for IRRI soil. The average εprop values under sulfidogenic conditions were around −3.5 ‰ in both soils, irrespective of whether CH3F was present or not.
3.3 Hydrogenotrophic methanogenesis
The difference between the δ13C of CH4 in the presence and in the absence of CH3F was used together with the δ13C of acetate to roughly estimate the percentage of CH4 derived from versus acetate (Fig. S3 in the Supplement). The percentage fractions of hydrogenotrophic methanogenesis () in Vercelli soil reached a maximum after 40–50 d when acetate concentrations also reached a maximum (Fig. S3a) and then decreased strongly. The same was the case in IRRI soil after around 35 d (Fig. S3b). When assuming a reasonable isotopic enrichment factor of = −15 ‰, which is in between the of aceticlastic Methanosaeta (Penning et al., 2006; Valentine et al., 2004) and Methanosarcina species (Gelwicks et al., 1994; Goevert and Conrad, 2009), the average values were 0 % for Vercelli soil and 20 % for IRRI soil (Fig. S3c).
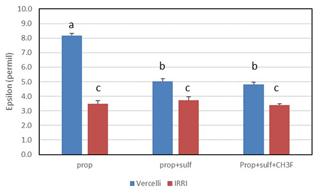
Figure 5Isotopic enrichment factors (εprop, given as negative values) in paddy soils without and with the addition of sulfate (gypsum) and CH3F. Shown are the means ± SE (standard error). The differences between the incubations were examined using Tukey's post hoc test of a one-way analysis of variance (ANOVA). Different letters on top of the bars indicate significant difference (P < 0.05) between the data.
Pathway of propionate degradation
Our results showed that propionate was degraded via acetate as the main transient intermediate, finally resulting in the production of CH4 and CO2 under methanogenic conditions and CO2 under sulfidogenic conditions. These results are consistent with previous observations by Liu and Conrad (Liu and Conrad, 2017) using the same paddy soils. Stable isotope probing and correlation network analysis of the microbial communities have shown that propionate degradation is accomplished by both Syntrophopbacter and Smithella species (Gan et al., 2012; Liu and Conrad, 2017; Lueders et al., 2004). The present study showed that propionate degradation under methanogenic conditions was consistent with the major operation of the Smithella pathway. The main argument for this conclusion is the observation that butyrate was a transient intermediate of propionate degradation, albeit at low concentrations (Figs. 1 and S1). In the Smithella pathway, butyrate is further fermented to acetate and H2. However, production of H2 is smaller in the Smithella pathway than in the Syntrophobacter pathway, while production of acetate is larger. Indeed, aceticlastic methanogenesis explained all the propionate-driven methanogenesis in the paddy soils (Figs. 2a and S2a). The average hydrogenotrophic methanogenesis, by contrast, contributed almost zero in Vercelli soil and only about 20 % in IRRI soil (Fig. S3c). The relatively larger contribution of aceticlastic compared to hydrogenotrophic methanogenesis to methanogenic propionate degradation supports the conclusion that the Smithella pathway was dominant over the Syntrophobacter pathway. Arguments against the Smithella pathway are that the accumulated CH4 amounted to less than the expected 1.75 mole CH4 per mole propionate consumed in Vercelli soil (Fig. 2a) and to even less in IRRI soil (Fig. S2a). With the inhibition of aceticlastic methanogenesis, acetate accumulation in Vercelli soil accounted for about 1 mole acetate per mole propionate, which is in a range that is compatible with propionate fermentation by either Smithella or Syntrophobacter (Fig. 2b). In IRRI soil, however, acetate accumulation accounted for less than 1 mole acetate per mole propionate (Fig. S2b). Note, however, that the accumulation of acetate reflects only that part of propionate fermentation, which was not inhibited by CH3F. Our conclusion that propionate was degraded mainly by Smithella under methanogenic conditions is consistent with the microbial community structures in the paddy soils from Vercelli and the IRRI, which contain not only Syntrophobacter species but also Smithella together with Syntrophomonas, which is able to ferment butyrate (Liu and Conrad, 2017).
Under sulfidogenic conditions, propionate can be oxidized in different ways, either directly by sulfate reducers forming acetate and CO2 or syntrophically, as under methanogenic conditions, but with subsequent oxidation of H2 and acetate by sulfate reducers. Using the same paddy soils, Liu and their coworkers (Liu et al., 2018a; Liu and Conrad, 2017) recently showed that, under sulfidogenic conditions, propionate consumption was mainly achieved by Syntrophobacter spp., which first oxidized propionate to acetate and CO2 and subsequently oxidized the accumulated acetate to CO2. These were exactly the processes observed in the present study, where propionate degradation initially resulted in almost equimolar accumulation of acetate (Fig. 2d) according to
It was interesting that CH3F was not only a strong inhibitor of aceticlastic methanogenesis (which was expected) but also a relatively strong inhibitor of propionate fermentation, though this was only the case under methanogenic conditions and not under sulfidogenic conditions. Inhibition of propionate fermentation under methanogenic conditions has been observed before in three different paddy soils and has been interpreted as being due to the adverse thermodynamic conditions when acetate accumulates (Conrad et al., 2014). However, this interpretation cannot be true since the accumulation of acetate also occurred under sulfidogenic conditions, where CH3F did not inhibit propionate degradation. In fact, it is mainly the accumulation of H2 rather than acetate to which propionate degradation is thermodynamically sensitive. This is the reason why the Smithella pathway is less sensitive to thermodynamic inhibition than the Syntrophobacter pathway (Dolfing, 2013). However, CH3F did not inhibit H2 consumption by methanogens, as seen by the low δ13C of CH4 in the presence of CH3F. Furthermore, the first step of the Smithella-type propionate fermentation does not produce any H2, and therefore, propionate in the presence of CH3F should at least be fermented to butyrate and acetate; however, this was not the case. Hence, the reason why CH3F inhibited propionate fermentation under methanogenic conditions but not under sulfidogenic conditions remains unknown. Perhaps it is Smithella being more sensitive to CH3F than Syntrophobacter.
Fractionation during propionate degradation
The isotopic fractionation of propionate apparently followed Raleigh distillation, which is characteristic for kinetic isotope fractionation in a closed system. The isotopic enrichment factor, which was determined from Mariotti plots, was in the range of εprop = −8 ‰ to −3.5 ‰, which is less than the enrichment factor for methanogenic acetate consumption, which has been found to be εac = −21 ‰ to −17 ‰ (Conrad et al., 2021). The εprop values are on the same order as those predicted from δ13C values of propionate, acetate and organic carbon measured in various methanogenic soils and sediments (Conrad et al., 2014). Propionate degradation resulted in the formation of 13C-enriched acetate and CO2 and 13C-depleted butyrate and CH4. The formation of 13C-depleted butyrate can be explained by the kinetic isotope effect with the preferential utilization of 13C-depleted propionate in the initial dismutation reaction by Smithella. However, the production of 13C-enriched acetate cannot be explained by a linear kinetic isotope effect. We assume that the dismutation of propionate is a branch point (Fry, 2003; Hayes, 2001) at which the carbon flow is split into the production of 13C-enriched acetate and 13C-depleted butyrate. At the branch point, the carbon isotope flow shows a preferential flow of 12C into the product generated by the reaction with the larger fractionation factor, which would be butyrate. The further conversion of butyrate should produce acetate that is depleted in 13C. This acetate together with the acetate produced from propionate dismutation should result in the δ13C–acetate that is observed. The total acetate pool initially had a δ13C that was up to 10 ‰ heavier than the δ13C of propionate. In the end, the δ13C values were about equal. The observation that acetate was 13C-enriched relative to propionate is consistent with δ13C data for various soils and sediments (Conrad et al., 2014) reporting that acetate is, on average, enriched by 6 ‰ relative to propionate. Acetate was further converted to CH4 and to CO2. In Vercelli soil, the δ13C of CH4 was about 25–35 ‰ lighter than the δ13C of acetate. In IRRI soil, 13C depletion was even larger (30–40 ‰). In both soils, the isotopic enrichment factors for acetate consumption were in a range of −12 ‰ to −17 ‰ and, for CH4 production from acetate, they were in a range of −37 ‰ to −27 ‰ (Conrad et al., 2021). Considering that a certain percentage (albeit small) of CH4 was formed from CO2 reduction by hydrogenotrophic methanogenesis, which displays relatively negative enrichment factors (see the δ13C of CH4 in the presence of CH3F – Fig. 1g), the observed difference in the δ13C of CH4 versus acetate is reasonable. In Smithella fermentation, the only CO2 production occurs during the fermentation of butyrate and the aceticlastic conversion of acetate. In both cases, CO2 should be 13C-depleted relative to the substrates. Note that this was not the case. Unfortunately, the 13C contents of the individual C atoms of propionate, butyrate and acetate are not known. The 13C content in the different C positions might also affect the δ13C of CH4 and CO2, which are formed. It is also possible that, besides Smithella fermentation, the Syntrophobacter fermentation contributed to propionate degradation. In summary, the detailed process of isotope fractionation during the pathway of propionate degradation is unclear. However, the magnitude of the enrichment factors involved was relatively small, being on the order of < 10 ‰.
Under sulfidogenic conditions, propionate was most probably degraded by Syntrophobacter spp., first to acetate, then finally to CO2 (Liu et al., 2018a; Liu and Conrad, 2017). The carbon isotope fractionation of propionate consumption was, with an enrichment factor of εprop = −3.5 ‰, comparatively small. Propionate was eventually converted to two carbon products, of which one was depleted (the CO2) and the other was enriched (the acetate) in terms of 13C. In case of Syntrophobacter-type degradation, acetate and CO2 are produced from the conversion of pyruvate, which is generated in the methylmalonyl-CoA pathway. In this pathway, CO2 is first consumed by the conversion of propionyl-CoA to methylmalonyl-CoA and is then produced by the conversion of oxaloacetate to pyruvate. Pyruvate is finally converted to acetate and CO2, which should both be 13C-depleted with respect to pyruvate (DeNiro and Epstein, 1977). However, both acetate and CO2 were initially 13C-enriched relative to propionate (about 2–5 ‰) and then changed in opposite directions, with acetate becoming increasingly 13C-enriched and CO2 becoming increasingly 13C-depleted until the time when acetate accumulation reached a maximum (Fig. 5). Then, δ13C of both acetate and CO2 increased together with the increase in 13C of propionate (Fig. 5). The increase in δ13C of acetate is often explained by consumption, especially through aceticlastic methanogenesis (Heuer et al., 2010; Heuer et al., 2009). However, hardly any CH4 was produced under sulfidogenic conditions, and the 13C enrichment occurred during the phase of acetate accumulation. Therefore, the enrichment likely happened during acetate production from propionate degradation. The increasing 13C depletion of CO2 can also not be explained by consumption but only by the production from propionate. Hence, isotope fractionation during the conversion of propionate, particularly during the conversion of propionate to pyruvate, is unclear. We assume complications during the carboxylation and decarboxylation reactions. Unfortunately, we found hardly any literature data on the isotope fractionation of propionate fermentation. A co-culture of Syntrophobacter fumaroxidans with Methanobacterium formicicum exhibited marginal propionate fractionation with εprop = 0.9 ‰ and the formation of acetate that was slightly 13C-enriched (about 5 ‰) (Botsch and Conrad, 2011), similarly to what is observed here. In summary, the mechanism of isotope fractionation during the conversion of propionate is not completely clear, but the magnitude of isotope fractionation is quite low.
Propionate degradation under sulfidogenic conditions was explained by the metabolism of Syntrophobacteraceae, which, in a first step, converted propionate to 13C-enriched acetate and 13C-depleted CO2. By contrast, propionate degradation under methanogenic conditions was at least partially due to metabolism by Smithella, which, in a first step, converted propionate to 13C-enriched acetate and 13C-depleted butyrate. However, the isotopic enrichment factors (εprop) of propionate consumption in two paddy soils were generally very low (−8 ‰ to −3.5 ‰) under both methanogenic and sulfidogenic conditions. This low range is consistent with literature values of δ13C collected for propionate, acetate and organic carbon in various soils and sediments (Conrad et al., 2014). Fractionation of propionate carbon actually seems to be smaller than fractionation of acetate, which is at least 2 times larger (Conrad et al., 2021). Hence, degradation of organic carbon via propionate to acetate and CO2 apparently involves only a little isotope fractionation on the order of < 10 ‰. By contrast, further degradation of acetate and CO2 (+ H2) to CH4 involves substantial isotope fractionation. This is also the case for chemolithotrophic acetate production (Conrad et al., 2014).
The data are all contained in the figures and in the Supplement.
The supplement related to this article is available online at: https://doi.org/10.5194/bg-20-3625-2023-supplement.
RC designed the experiments, evaluated the data and wrote the paper. PC conducted the experiments.
The contact author has declared that neither of the authors has any competing interests.
Publisher's note: Copernicus Publications remains neutral with regard to jurisdictional claims in published maps and institutional affiliations.
This research has been supported by the Fonds der Chemischen Industrie (grant no. 163468).
The article-processing charges for this open-access publication were covered by the Max Planck Society.
This paper was edited by Jack Middelburg and reviewed by two anonymous referees.
Boone, D. R. and Bryant, M. P.: Propionate-degrading bacterium, Syntrophobacter wolinii sp. nov. gen. nov., from methanogenic ecosystems, Appl. Environ. Microb., 40, 626–632, 1980.
Botsch, K. C. and Conrad, R.: Fractionation of stable carbon isotopes during anaerobic production and degradation of propionate in defined microbial cultures, Org. Geochem., 42, 289–295, 2011.
Chen, S. Y., Liu, X. L., and Dong, X. Z.: Syntrophobacter sulfatireducens sp. nov., a novel syntrophic, propionate-oxidizing bacterium isolated from UASB reactors, Int. J. Syst. Evol. Micr., 55, 1319–1324, 2005.
Conrad, R.: Quantification of methanogenic pathways using stable carbon isotopic signatures: a review and a proposal, Org. Geochem., 36, 739–752, 2005.
Conrad, R., Claus, P., and Casper, P.: Stable isotope fractionation during the methanogenic degradation of organic matter in the sediment of an acidic bog lake, Lake Grosse Fuchskuhle, Limnol. Oceanogr., 55, 1932–1942, 2010.
Conrad, R., Claus, P., Chidthaisong, A., Lu, Y., Scavino, A., Liu, Y., Angel, R., Galand, P., Casper, P., Guerin, F., and Enrich-Prast, A.: Stable carbon isotope biogeochemistry of propionate and acetate in methanogenic soils and lake sediments, Org. Geochem., 73, 1–7, 2014.
Conrad, R., Liu, P., and Claus, P.: Fractionation of stable carbon isotopes during acetate consumption by methanogenic and sulfidogenic microbial communities in rice paddy soils and lake sediments, Biogeosciences, 18, 6533–6546, https://doi.org/10.5194/bg-18-6533-2021, 2021.
DeBok, F. A. M., Stams, A. J. M., Dijkema, C., and Boone, D. R.: Pathway of propionate oxidation by a syntrophic culture of Smithella propionica and Methanospirillum hungatei, Appl. Environ. Microb., 67, 1800–1804, 2001.
DeBok, F. A. M., Harmsen, H. J. M., Plugge, C. M., DeVries, M. C., Akkermans, A. D. L., DeVos, W. M., and Stams, A. J. M.: The first true obligately syntrophic propionate-oxidizing bacterium, Pelotomaculum schinkii sp. nov., co-cultured with Methanospirillum hungatei, and emended description of the genus Pelotomaculum, Int. J. Syst. Evol. Micr., 55, 1697–1703, 2005.
DeNiro, M. J. and Epstein, S.: Mechanism of carbon isotope fractionation associated with lipid synthesis, Science, 197, 261–263, 1977.
Dolfing, J.: Syntrophic propionate oxidation via butyrate: a novel window of opportunity under methanogenic conditions, Appl. Environ. Microb., 79, 4515–4516, 2013.
Elsner, M., Zwank, L., Hunkeler, D., and Schwarzenbach, R. P.: A new concept linking observable stable isotope fractionation to transformation pathways of organic pollutants [review], Environ. Sci. Technol., 39, 6896–6916, 2005.
Fry, B.: Steady state models of stable isotopic distributions, Isot. Environ. Healt. S., 39, 219–232, 2003.
Gan, Y., Qiu, Q., Liu, P., Rui, J., and Lu, Y.: Syntrophic oxidation of propionate in rice field soil at 15 and 30 ∘C under methanogenic conditions, Appl. Environ. Microb., 78, 4923–4932, 2012.
Gelwicks, J. T., Risatti, J. B., and Hayes, J. M.: Carbon isotope effects associated with aceticlastic methanogenesis, Appl. Environ. Microb., 60, 467–472, 1994.
Glissmann, K. and Conrad, R.: Fermentation pattern of methanogenic degradation of rice straw in anoxic paddy soil, FEMS Microbiol. Ecol., 31, 117–126, 2000.
Goevert, D. and Conrad, R.: Effect of substrate concentration on carbon isotope fractionation during acetoclastic methanogenesis by Methanosarcina barkeri and M. acetivorans and in rice field soil, Appl. Environ. Microb., 75, 2605–2612, 2009.
Hayes, J. M.: Factors controlling 13C contents of sedimentary organic compounds: principles and evidence, Mar. Geol., 113, 111–125, 1993.
Hayes, J. M.: Fractionation of carbon and hydrogen isotopes in biosynthetic processes, Stable Isotope Geochemistry, 43, 225–277, 2001.
Heuer, V. B., Pohlman, J. W., Torres, M. E., Elvert, M., and Hinrichs, K. U.: The stable carbon isotope biogeochemistry of acetate and other dissolved carbon species in deep subseafloor sediments at the northern Cascadia Margin, Geochim. Cosmochim. Ac., 73, 3323–3336, 2009.
Heuer, V. B., Krüger, M., Elvert, M., and Hinrichs, K. U.: Experimental studies on the stable carbon isotope biogeochemistry of acetate in lake sediments, Org. Geochem., 41, 22–30, 2010.
Houwen, F. P., Dijkema, C., Stams, A. J. M., and Zehnder, A. J. B.: Propionate metabolism in anaerobic bacteria – determination of carboxylation reactions with 13C-NMR spectroscopy, Biochim. Biophys. Acta, 1056, 126–132, 1991.
Imachi, H., Sekiguchi, Y., Kamagata, Y., Hanada, S., Ohashi, A., and Harada, H.: Pelotomaculum thermopropionicum gen. nov., sp. nov., an anaerobic, thermophilic, syntrophic propionate-oxidizing bacterium, Int. J. Syst. Evol. Micr., 52, 1729–1735, 2002.
Imachi, H., Sekiguchi, Y., Kamagata, Y., Loy, A., Qiu, Y. L., Hugenholtz, P., Kimura, N., Wagner, M., Ohashi, A., and Harada, H.: Non-sulfate-reducing, syntrophic bacteria affiliated with Desulfotomaculum cluster I are widely distributed in methanogenic environments, Appl. Environ. Microb., 72, 2080–2091, 2006.
Janssen, P. H. and Frenzel, P.: Inhibition of methanogenesis by methyl fluoride - studies of pure and defined mixed cultures of anaerobic bacteria and archaea, Appl. Environ. Microb., 63, 4552–4557, 1997.
Krylova, N. I. and Conrad, R.: Thermodynamics of propionate degradation in methanogenic paddy soil, FEMS Microbiol. Ecol., 26, 281–288, 1998.
Krylova, N. I., Janssen, P. H., and Conrad, R.: Turnover of propionate in methanogenic paddy soil, FEMS Microbiol. Ecol., 23, 107–117, 1997.
Liu, P., Pommerenke, B., and Conrad, R.: Identification of Syntrophobacteraceae as major acetate-degrading sulfate reducing bacteria in Italian paddy soil, Environ. Microbiol., 20, 337–354, 2018a.
Liu, P. F. and Conrad, R.: Syntrophobacteraceae-affiliated species are major propionate-degrading sulfate reducers in paddy soil, Environ. Microbiol., 19, 1669–1686, 2017.
Liu, P. F., Klose, M., and Conrad, R.: Temperature effects on structure and function of the methanogenic microbial communities in two paddy soils and one desert soil, Soil Biol. Biochem., 124, 236–244, 2018b.
Liu, Y. T., Balkwill, D. L., Aldrich, H. C., Drake, G. R., and Boone, D. R.: Characterization of the anaerobic propionate-degrading syntrophs Smithella propionica gen. nov., sp. nov. and Syntrophobacter wolinii, Int. J. Syst. Bacteriol., 49, 545–556, 1999.
Lueders, T., Pommerenke, B., and Friedrich, M. W.: Stable-isotope probing of microorganisms thriving at thermodynamic limits: Syntrophic propionate oxidation in flooded soil, Appl. Environ. Microb., 70, 5778–5786, 2004.
Mariotti, A., Germon, J. C., Hubert, P., Kaiser, P., Letolle, R., Tardieux, A., and Tardieux, P.: Experimental determination of nitrogen kinetic isotope fractionation: some principles; illustration for the denitrification and nitrification processes, Plant Soil, 62, 413–430, 1981.
McInerney, M. J., Bryant, M. P., Hespell, R. B., and Costerton, J. W.: Syntrophomonas wolfei gen. nov. sp. nov., an anaerobic, syntrophic, fatty acid-oxidizing bacterium, Appl. Environ. Microb., 41, 1029–1039, 1981.
Nozoe, T.: Effects of methanogenesis and sulfate-reduction on acetogenetic oxidation of propionate and further decomposition of acetate in paddy soil, Soil Sci. Plant Nutr., 43, 1–10, 1997.
Penning, H., Claus, P., Casper, P., and Conrad, R.: Carbon isotope fractionation during acetoclastic methanogenesis by Methanosaeta concilii in culture and a lake sediment, Appl. Environ. Microb., 72, 5648–5652, 2006.
Plugge, C. M., Balk, M., and Stams, A. J. M.: Desulfotomaculum thermobenzoicum subsp. thermosyntrophicum subsp. nov., a thermophilic, syntrophic, propionate-oxidizing, spore-forming bacterium, Int. J. Syst. Evol. Micr., 52, 391–399, 2002.
Schink, B.: Mechanisms and kinetics of succinate and propionate degradation in anoxic freshwater sediments and sewage sludge, J. Gen. Microbiol., 131, 643–650, 1985.
Textor, S., Wendisch, V. F., DeGraaf, A., Mueller, U., Linder, M. I., Linder, D., and Buckel, W.: Propionate oxidation in Escherichia coli – evidence for operation of a methylcitrate cycle in bacteria, Arch. Microbiol., 168, 428–436, 1997.
Valentine, D. L., Chidthaisong, A., Rice, A., Reeburgh, W. S., and Tyler, S. C.: Carbon and hydrogen isotope fractionation by moderately thermophilic methanogens, Geochim. Cosmochim. Ac., 68, 1571–1590, 2004.
Xia, X. X., Zhang, J. C., Song, T. Z., and Lu, Y. H.: Stimulation of Smithella-dominated propionate oxidation in a sediment enrichment by magnetite and carbon nanotubes, Env. Microbiol. Rep., 11, 236–248, 2019.
Yao, H. and Conrad, R.: Thermodynamics of propionate degradation in anoxic paddy soil from different rice-growing regions, Soil Biol. Biochem., 33, 359–364, 2001.