the Creative Commons Attribution 4.0 License.
the Creative Commons Attribution 4.0 License.
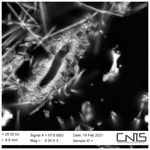
Biogenic calcium carbonate as evidence for life
Sara Ronca
Francesco Mura
Marco Brandano
Angela Cirigliano
Francesca Benedetti
Alessandro Grottoli
Massimo Reverberi
Daniele Federico Maras
Rodolfo Negri
Ernesto Di Mauro
Teresa Rinaldi
The history of the Earth is a story of the co-evolution of minerals and microbes: not only have numerous rocks arisen from life but also life itself may have formed from rocks. To understand the strong association between microbes and inorganic substrates, we investigated the moonmilk, a calcium carbonate deposit of possible microbial origin, occurring in the Iron Age Etruscan necropolis of Tarquinia, in Italy. These tombs provide a unique environment where the hypogeal walls of the tombs are covered by this speleothem. To study moonmilk formation, we investigated the bacterial community in the rock in which the tombs were carved: calcarenite and hybrid sandstone. We present the first evidence that moonmilk precipitation is driven by microbes within the rocks and not only on the rock surfaces. We also describe how the moonmilk produced within the rocks contributes to rock formation and evolution. The microbial communities of the calcarenite and hybrid sandstone displayed, at the phylum level, the same microbial pattern of the moonmilk sampled from the walls of the hypogeal tombs, suggesting that the moonmilk originates from the metabolism of an endolytic bacterial community. The calcite moonmilk is the only known carbonate speleothem on Earth with undoubted biogenic origin, thus representing a robust and credible biosignature of life. Its presence in the inner parts of rocks adds to its characteristics as a biosignature.
- Article
(4545 KB) - Full-text XML
-
Supplement
(22628 KB) - BibTeX
- EndNote
Whether other planets witnessed life like what is seen on Earth remains a complete mystery. The search for traces of extra-terrestrial life suffers from the lack of durable and credible biosignatures. Some breakthroughs may happen soon from the current planetary explorations. The NASA Perseverance rover successfully landed on planet Mars in the Jezero Crater in 2021 and has been collecting many specimens. Future missions are planned to retrieve those specimens, although not before 2031, hence opening the possibility to search for evidence of life from the first-ever samples returned from Mars. The European Space Agency (ESA) ExoMars programme is also planning to address the question of whether life has ever existed on Mars.
Earth has evolved through a long process of co-evolution between minerals and microbes (Cosmidis and Benzerara, 2022; Grosch et al., 2015; Cuadros, 2017; Hazen et al., 2008), and terrestrial rocks constitute an ideal system for the investigation of durable signs of life. Carbonates are good candidates as host rocks because, on Earth, they are largely of biogenic origin. Nevertheless, they did not receive the attention needed because of limited and sporadic evidence on Mars: lithologies revealed the presence of carbonates in the Nili Fossae region (Ehlmann et al., 2008), at the Mars Phoenix landing site (Boynton, et al., 2009), in the Columbia Hills of Gusev Crater (Morris et al., 2010), and in deep rocks exposed by meteor impacts (Michalski et al., 2010). Recently, the analysis of weathering profiles revealed the widespread distribution on Mars of carbonates associated with hydrated minerals, providing evidence of past liquid water presence (Bultel et al., 2019). Carbonates were also detected in asteroids and meteorites, contributing to the understanding of the formation and evolution of our solar system (Lee et al., 2014; Kaplan et al., 2020; Pilorget et al., 2021; Voosen, 2020).
Carbonate rocks on Earth are of abiogenic or biogenic origin and have arisen since the early Archean (> 3 giga-annum, Ga) Eon, when hydrothermal systems were ubiquitous. At that time, the carbonate rocks originated by massive carbonatisation, silicification, and potassium (K) (±Sodium) metasomatism of intermediate to ultramafic silicate precursors (Veizer et al., 1989). In contrast, lower Archean marine carbonates are rare; they occur as very thin, discontinuous, and extensively mineralised beds generally replaced by chert as a result of intense microbial iron (Fe) cycling (Pomar, 2020). The 3.4 Ga old stromatolite structures (sensu Riding, 2011) are widely regarded as among the oldest biogenic carbonate production associated with carbon dioxide (CO2) sequestration (Allwood et al. 2006). During the Proterozoic Eon (< 2.4 Ga), shallow water carbonate production expanded, favouring the development of carbonate platforms where abiogenic and biogenic carbonate precipitation took place (Grotzinger and James, 2000). The seawater was supersaturated with both calcite and aragonite, as evidenced by the well-preserved pseudomorphs of “abiogenic” aragonite and calcite (Pomar, 2020). According to Sumner and Grotzinger (1996), the rise in oxygen concentration at 2.2–1.9 Ga led to the removal of Fe2+, a strong calcite-precipitation inhibitor, from seawater and resulted in a shift from Archean to Proterozoic carbonates that are dominated by microbial activity (Pomar, 2020). Starting from the Cambrian Period, the benthic microbialites (sensu Riding, 2011), the prime carbonate factory since the Late Archean, were still important but progressively decreased, while the biologically controlled carbonates appeared and expanded. The calcification of sessile, mostly colonial, metazoans and algae promoted the accumulation of biogenic carbonate sediments and the appearance and expansion of reefs (Pomar, 2020).
Carbonates are common constituents of the near-surface Earth crust, although carbonate phases may also occur deep in the mantle. They are compounds formed by the anionic complex, carbonate (CO3)2−), combined with metal ions such as calcium (Ca), magnesium (Mg), iron, manganese, sodium, barium, aluminium, zinc, copper, lead, uranium, or rare-earth elements. Uncommonly, carbonate phases are hydrated, contain hydroxyl or halogen ions, or may include silicate, sulfate, or phosphate radicals. Due to the high availability of Ca and Mg in crustal reservoirs (Hartmann et al., 2012), the CaCO3 polymorphs, calcite, aragonite, and dolomite (CaMg(CO3)2) are the most widespread carbonate minerals, whose formation on Earth near-surface environments is widely related to biogenic or bio-mediated processes (Görgen et al., 2021).
Carbonate biomineralisation or organomineralisation (sensu Dupraz et al., 2009) results in the formation of several mineral phases, the most common of which are the CaCO3 anhydrous polymorphs calcite, aragonite, and vaterite, the last being a metastable transitional phase; the hydrated forms of monohydrocalcite (CaCO3•H2O) and ikaite (CaCO3•6H2O); and various amorphous phases (ACC). Moreover, in specific environments (saline lakes, coastal lagoons) (Diloreto et al., 2021; Kaczmarek et al., 2017), microbial activity may promote the formation of dolomite (ordered phase CaMg(CO3) by passing through the precursor phases of high Mg calcite (disordered 4–36 mol % MgCO3), disordered dolomite (disordered > 36 mol % MgCO3), and proto-dolomite (weakly ordered > 36 mol % MgCO3). Carbonate mineral formation seems to proceed from amorphous or disordered phases towards the more stable and ordered forms (Asta et al., 2020); crystal growth and morphologies are controlled by the medium composition, the microbial extracellular polymeric substances (EPS), the Mg Ca ratio, and the presence of other ions. Thus, the mineral phases resulting from abiogenic or biogenic activity are indistinguishable, and the identification of irrefutable biosignatures, evidence of past or present life, remains absent (Changela et al., 2021; Javaux, 2019).
Carbonate rocks, being mostly of biogenic origin, could be considered in this perspective as a possible biosignature, but even if specific analyses could discriminate between biogenic and abiogenic carbonate rocks (Blanco et al., 2013), the attempt to unequivocally distinguish between carbonates of biogenic or abiogenic origin remains vacuous, especially in the search for evidence of life on other planets. So far, the presence of organic materials in carbonate has not been an incontrovertible indicator of biogenicity (Berg et al., 2014).
Consideration for calcium carbonate minerals as possible biosignatures can be found by the study of a secondary calcite deposit, called moonmilk, formed by nanofibres of calcite, commonly found on karst cave surfaces. Calcium carbonate deposits, consisting of thin crystal fibres, have been observed in various vadose environments (soils, karstic caves, and other hypogeal spaces), and an exhaustive classification of calcite fibre morphology is presented in Cailleau et al. (2009). Although widely studied, the origin of such deposits is still a debated matter, being attributed to physicochemical processes (Kolesàr and Čurlik, 2015; Jones and Peng, 2014) or to various biogenic processes (Cañaveras et al., 2006; Millière et al., 2019). In the past, inorganic precipitation mechanisms were thought to be either controlled by climatic variations, as in the case of the Caverne de l'Ours (Lacelle et al., 2004) in Canada, or from the heterogeneous nucleation of calcite from supersaturated fluids, as in the Staloti cave (Borsato et al., 2000). More recently, many authors discussed the biogenicity of the moonmilk (Baskar et al., 2011; Braissant et al., 2012; Cacchio et al., 2014; Kondratyeva et al., 2020; Maciejewska et al., 2017; Portillo and Gonzalez, 2011), reporting that the moonmilk precipitation is promoted by the metabolic activities of a microbial community living in environments rich in calcium content (Banks et al., 2010; Cailleau et al., 2009; Cirigliano et al., 2018; Portillo et al., 2011; Compière et al., 2017). Nevertheless, direct evidence that bacteria promote the precipitation of nanofibres of calcite is still lacking. This evidence is essential to define the moonmilk as a bona fide biosignature.
Recently, the moonmilk speleothem was discovered in the hypogeal ancient Etruscan tombs of the Monterozzi necropolis (Tarquinia, Central Italy) (Cirigliano et al., 2018; Tomassetti et al., 2017). This finding provides a unique opportunity to compare the moonmilk which covers the walls and ceilings collected from 12 tombs excavated in two types of rock, the calcarenite and hybrid sandstone. We provided insight into the formation of moonmilk, which can occur rapidly, i.e. between 10 and 50 years, and we reported that this speleothem originates from, and harbours, a microbial community able to induce carbonate precipitation (Cirigliano et al., 2021a, b). Here, we propose that the nanofibre calcite deposit (moonmilk) developing inside the rocks (calcarenite and sandstone) is promoted by a microbial community and by the physio-chemical features of the host rock. We present an example of an ongoing symbiotic co-evolution between rocks and microorganisms: the moonmilk contributes to the evolution of the rock rich in calcium carbonate, while the physio-chemical features of the host rock shape the resident microbial community which induces the moonmilk deposition.
2.1 Site description and sampling
Samples representative of the bedrock were collected from the ancient Etruscan necropolis of Tarquinia, a UNESCO World Heritage Site (Viterbo, Italy), in which more than 200 painted hypogeal tombs (dated from the 7th to the 2nd century BC) were discovered. The tombs were excavated in a sedimentary bedrock belonging to a middle- to upper-Pliocene formation known as macco consisting of yellowish bioclastic calcarenites interbedded with hybrid sandstones (Fig. S1 in the Supplement shows the map of the necropolis in Tarquinia and the sampling locations). From each location, rock samples were collected and kept in plastic bags on ice and transported to the laboratory for analysis. With a sterile hammer and chisel, surface material and samples from outdoor and indoor rocks were first removed to a depth of 3 to 5 cm. The interior of the rock samples was processed for DNA extraction or geological experiments.
2.2 Media and growth condition for calcium carbonate organomineralisation
To study the organomineralisation in the laboratory, bacterial strain cultures are maintained in an LB (Luria Bertani) liquid medium (1 % Bacto Tryptone, 0.5 % di yeast extract, 0.5 % NaCl, and 0.1 % NaOH 1N). The carbonatogenic activity of bacterial strains has been assessed in a solid complete medium YPDuc plates, containing 1 % Bacto Peptone, 1 % yeast extract, 2 % glucose, 4 % urea, 2.5 % CaCl2, and 2 % agar, adjusted to pH 8.0. To assess the carbonatogenic activity of the microbial community, 0.1 g of crushed calcarenite was inoculated in a BPuc medium (0.72 % Bacto-peptone, 4 % urea and 2.5 % CaCl2, pH 8.0) for 1 week at 28 ∘C, at 160 rpm min−1. The experiments were performed in three technical and three biological replicas. These media induce the metabolism of urea of the microorganisms, resulting in a fast organomineralisation in plates or in liquid medium.
2.3 DNA extraction procedures and sequencing of rock and moonmilk samples
For each sample, rock material was collected aseptically using a sterile rock hammer or chisel and stored in sterile collection bags. All samples intended for DNA extraction were collected by discarding the top 3–5 cm layer and then crushing the inner part with a sterile rock hammer and further reducing it to a powder by grinding with a sterile mortar and pestle. Genomic DNA extraction was performed using the DNeasy PowerMax Soil Kit (QIAGEN) following the manufacture manual method using about 10 g of collected material. Spectrophotometric quantification was performed using a Thermo Scientific NanoDrop spectrophotometer (Thermo Scientific), and DNA purity was assessed through the evaluation of and absorbance ratios. PCR amplification was performed on about 50 ng of DNA from each sample, as described by Grottoli et al. (2020). Amplicon sequencing was performed on PCR products deriving from the 16S rRNA sample regions. PCR products were sequenced through Oxford Nanopore Technologies (ONT) by a MinION sequencer. A total amount of 200 ng of PCR products for samples was used for sequencing. Rapid barcoding of PCRs was carried out following the protocol released by ONT (SQK-RBK004) and sequenced by a Flongle Flow Cell (FLO-FLG001). The total run produced ∼15 Mb of data (∼ 34 k reads), including ∼ 2.8 Mb (∼ 4 k reads) for moonmilk of the Tomba Maggi 2, ∼ 2.8 Mb (∼ 4 k reads) for moonmilk of the Tomba degli Scudi, ∼ 2.7 Mb (∼ 11 k reads) for hybrid sandstone, and ∼ 6.4 Mb (∼ 15 k reads) for calcarenite, respectively. The Kraken2 system (Wood et al., 2019) produced a taxonomic classification of 98.83 %, 96.65 %, 44.02 %, and 62.29 % of total reads for each sample, moonmilk of the Tomba Maggi 2, moonmilk of the Tomba degli Scudi, hybrid sandstone, and calcarenite, respectively.
2.4 Petrophysical analysis
Polarised light microscopy (PLM) observations of rock samples and moonmilk speleothems have been carried out on thin sections at the Dipartimento di Scienze della Terra of the Sapienza University of Rome by means of Olympus BX50 (Japan) and Zeiss AxioPhot (Germany) transmitted polarised light microscopes, under plane-polarised light and crossed polars, at 25×, 40×, 100×, 200×, and 400× magnifications. Microscopic images were obtained by using a digital camera. Polished thin sections (28 mm × 48 mm) were prepared from the sample vacuum impregnated with epoxy resin before cutting and thinning. Density and porosity were measured using an Ultrapyc 5000 helium pycnometer from Anton Paar with an accuracy of 0.02 % and repeatability of 0.01 %. Bulk density was obtained by dividing the dry mass of the sample by its total volume. Grain density resulted from the calculation of the mass/measured volume ratio of the pulverised matrix. Both total and effective (open) porosity was measured. The latter was obtained by dividing the difference between the geometric volume and the volume measured by the pycnometer of the sample (Ruggieri and Trippetta, 2020; Trippetta et al., 2020). All laboratory measurements were made in the Earthquake Physics Laboratory at Sapienza Earth Sciences Department. Bulk rock major and trace-element compositions were obtained by lithium metaborate–tetraborate fusion (inductively coupled plasma–atomic emission spectroscopy (ICP-AES) and ICP–mass spectrometry (ICP-MS)), at Activation Laboratories Inc. (Ontario, Canada) according to the Code 4Litho code package on solutions prepared with lithium metaborate fusion. Loss on ignition (LOI) was measured according to standard gravimetric procedures. Details on the precision and accuracy of the analyses are reported in https://actlabs.com/ (last access: 22 November 2022). The calcium carbonate content was assessed by gasometric measurements using a Dietrich–Frühling calcimeter measuring 1 g of the bulk sediment, following Siesser et al. (1971).
2.5 Scanning electron microscopy analysis
Scanning electron microscopy (SEM) was performed on the Moonmilk samples and on the rock thin sections using the field emission scanning electron microscopy (FESEM) Zeiss Auriga 405, with a chamber room that maintains a pressure of about 10–5 to 10–6 mbar. Before mounting the samples inside the microscope, the specimens were coated with 20 nm of chromium using a Quorum Q150T sputter. Chromium has a high X-ray Kα value (5.145 keV), so it does not interfere with lighter elements during the EDX (energy dispersive X-ray) analysis. EDX spectra were obtained using a Bruker QUANTAX detector in point mode for 30 s, with the electron microscope acceleration voltage set at 10 kV and a working distance of 6 mm to optimise the number of the incoming X-ray signals.
3.1 Lithology, mineralogy, and geochemical characterisation of the bedrock
The area of the Monterozzi necropolis offers a favourable chance to investigate the role of bedrock in the genesis of the moonmilk. To this purpose, we analysed samples of bedrock taken from different areas of the necropolis with special focus on the sites where the Tomba dei Vasi dipinti, Tomba Maggi 2, Tomba delle Pantere, and Tomba degli Scudi were carved (Fig. S1). The hypogeal tombs of the Monterozzi necropolis, located on a flat elevated area, are excavated within a sedimentary substrate of middle- to upper-Pliocene age known as macco formation consisting of two main lithofacies showing lateral and vertical heterotopic relationships. Macco s.s. lithofacies is a bioclastic calcarenite represented by packstone to rudstone and floatstone composed of a small volume (< 5 %–10 %) of micrite matrix, coralline algal branches, bryozoans, bivalves (pectinids and oysters), echinoids, benthic foraminifers, and skeletal debris. Rare non-carbonate grains are present. In the inner walls of the intergranular voids, microsparite cement precipitation and/or recrystallisation often occurs in a phreatic marine environment. The second lithofacies is represented by a poorly cemented, crudely stratified, hybrid sandstone. It is fine to medium grain-sized and grain-supported, with a small amount of micrite matrix (< 10 % vol), and nearly devoid of carbonate cement. This lithofacies is characterised by abundant bioturbation, the skeletal assemblage is dominated by small benthic foraminifers, echinoids, serpulids, and bivalves (mainly oysters). Planktonic foraminifers are common. The terrigenous fraction mainly consists of monocrystalline grains of quartz, sedimentary lithoclasts, and subordinate detrital micas and feldspar along with rare glaucony grains and opaques. Calcimetric analyses from the two lithofacies revealed that calcium carbonate contents range from 90 % to 98 % in the macco s.s. calcarenite and from 49 % to 59 % in the hybrid sandstone. Moreover, whole-rock major oxide and trace-element compositions highlight the geochemical difference between the two lithofacies (Table 1), mostly related to the higher proportion of terrigenous fraction in the hybrid sandstone. Helium pycnometry revealed a high open porosity in both lithofacies (∼ 43% for macco s.s.; ∼ 42 % for hybrid sandstone). The macco s.s. lithofacies show a dominance of vuggy porosity and abundant intraparticle, interparticle, and mouldic porosity. The main porosity of hybrid sandstone is represented by interparticle porosity, rarely by mouldic porosity. Thus, despite the differences in composition, the calcarenite and the hybrid sandstone show two essential characteristics required for moonmilk formation: high calcium content, which activates the microbial metabolism leading to organomineralisation, and high porosity, necessary for the exchange of fluids and nutrients in an oligotrophic environment, also providing the space for microbial colonisation. Noteworthily, the characteristics of bedrock porosity (vuggy and mouldic) indicate that the dissolution processes prevail on those of inorganic carbonate precipitation; indeed, meteoric cements as well as speleothems in the largest cavities are absent. Moreover, the bedrock where the tombs are carved is located in the shallow vadose zone (a few metres below the surface). The high magnesium calcite, the dominant mineralogy of the main components, the coralline algae, is a metastable mineral phase of calcite. This phase, upon exposure to meteoric water, dissolves, partially increasing the availability of Ca2+ for the microbial metabolism and for the biogenic carbonate precipitation. It is notable that the area under investigation lies on a high flat relief, where the infiltrated water mostly derives from rainfall, without any groundwater input. Consequently, the infiltrated water can be reasonably assumed to be undersaturated with respect to calcite.
3.2 First report of the inner location of moonmilk
Mostly, moonmilk biogenic deposits develop indifferently within the two distinct lithofacies constituting the bedrock of the Etruscan necropolis of Monterozzi (Mura et al., 2021). Figure 1a shows an example of the hypogeal walls of tombs carved in calcarenite and hybrid sandstone and the walls covered by moonmilk in the Tomba degli Scudi and Tomba Maggi 2 (Fig. 1b). The moonmilk layer originating from hybrid sandstone is thinner than the one observed on a calcarenite substrate, but the scanning electron micrographs of the moonmilk sampled from the walls of the Tomba degli Scudi and Tomba Maggi 2 showed the same nanofibre structure (Fig. 1c). X-ray powder diffraction analysis (XRD) revealed that moonmilk is composed of calcite (Mura et al., 2020).
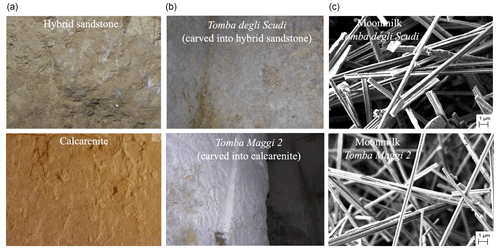
Figure 1In Tarquinia, Italy, during the Iron Age, the ancient Etruscans carved hypogeal tombs into calcarenite and in hybrid sandstone bedrock, whose walls are covered of the moonmilk, a secondary speleothem. (a) Examples of hypogeal walls of tombs carved in hybrid sandstone and calcarenite; the absence of the moonmilk is due to the restoration interventions. (b) Through the centuries, the moonmilk speleothem precipitated as a white patina on the walls and ceilings of the tombs: as an example, the walls covered in moonmilk of the Tomba degli Scudi and the Tomba Maggi 2 are shown, carved in hybrid sandstone and calcarenite, respectively. (c) Scanning electron micrographs of the moonmilk sampled on the walls showed in panel (b) in the Tomba degli Scudi and the Tomba Maggi 2. Regardless of the rock substrate in which the moonmilk is formed, the structure of the nanofibres is similar.
So far, the moonmilk has only been considered to be a deposition covering rock surfaces (Borsato et al., 2000), but the analysis with transmitted polarised light microscopy of thin sections showed that the moonmilk is present inside the calcarenite in the vuggy and mouldic porosity of the calcarenite in the Tomba Maggi 2 (Fig. 2a) and in intergranular and mouldic pores of the hybrid sandstone bedrock collected inside the Tomba degli Scudi (Fig. 2b). The presence of moonmilk inside the rocks is a general phenomenon because it is observed in all samples, irrespective of the type of rock, calcarenite or hybrid sandstone, and collection site (outdoor or indoor) (Figs. S2, S3, and S4). These results are also supported by the discovery of moonmilk deep inside a calcarenite rock sampled at the entrance of the Tomba dei Vasi Dipinti (Fig. S5).
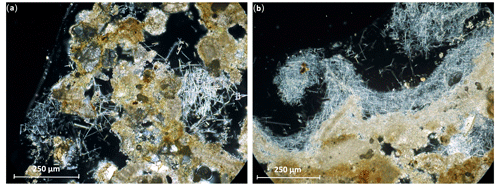
Figure 2The moonmilk is present on the surface and inside the calcarenite and sandstone rocks. Optical microscope thin-section micrographs in cross-polarised transmitted light. (a) A calcarenite sample collected inside the Tomba Maggi 2 and (b) a hybrid sandstone sample collected inside the Tomba degli Scudi.
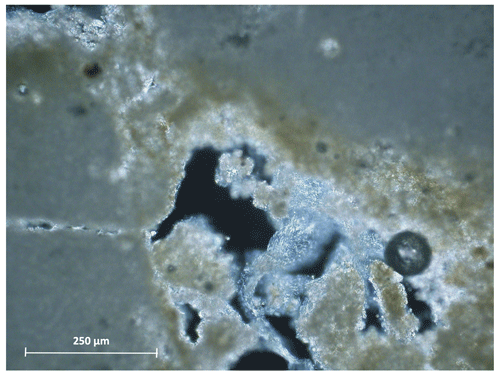
Figure 3The moonmilk contributes to the lithogenic processes. Optical microscope thin-section micrographs (cross-polarised transmitted light) of the moonmilk speleothems grown into the calcarenite sampled outside of the Tomba dei Vasi Dipinti.
The analysis of the rock substrate sampled outside of the Tomba dei Vasi Dipinti also suggests that the moonmilk may be contributing to the authigenic carbonate growth in the host rock, covering the inner walls of the voids (Fig. 3).
3.3 Co-evolution of rocks and microorganisms
If the moonmilk observed inside the rocks (a location that was not reported before) is of biogenic origin, traces of organomineralisation would be expected. Indeed, the SEM analysis on the thin sections of calcarenite sampled outside of the Tomba dei Vasi Dipinti revealed many structures (nanofibres that originate from bacteria encased in a calcite structure) corresponding to organomineralisation (Figs. 4 and S6). Bacterial organomineralisation was also detected in the sandstone sampled inside the Tomba degli Scudi (Fig. S7) and in calcarenite sampled outside of the Tomba delle Pantere and inside of the Tomba Maggi 2 (Fig. S8), suggesting that this is a common phenomenon. Such bacterial organomineralisation is also known as “entombment” (Barton and Northup, 2007), and it is easily observed in laboratory settings when bacterial strains are subjected to environmental conditions favouring calcium carbonate precipitation (Fig. S9a, b, c). In plates, the precipitation of calcium carbonate occurs even at a considerable distance from the bacterial colony, possibly by the diffusion of extracellular enzymes known to be involved in calcium carbonate metabolism (Dhami et al., 2014; Rodriguez-Navarro et al., 2019) (Fig. S9d).
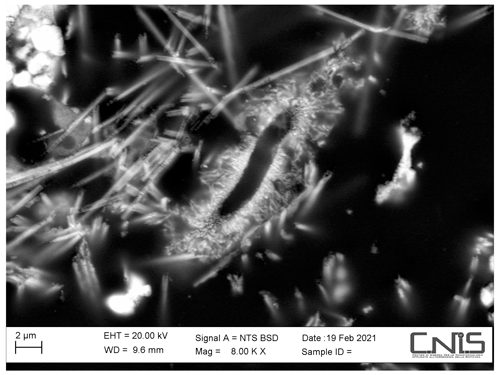
Figure 4Bacterial organomineralisation in the calcarenite. Scanning electron micrograph of a thin section of the calcarenite sampled outside of the Tomba dei Vasi Dipinti.
It remains unclear how the moonmilk nanofibres are produced in natural environmental conditions because to date it has been impossible to reproduce their formation in the laboratory. In fact, bacterial strains cultured from rocks represent only a negligible fraction of the total microorganisms present in the rocks. Instead, the entire microbial community, with a metabolism that sustains the growth in the rock environment, is needed to precipitate and/or dissolve calcium carbonate. Indeed, under laboratory conditions, we have evidence that the ground calcarenite, with its entire microbial community, when present in a medium containing urea and CaCl2, produced calcite. In the same conditions, calcium carbonate is not produced with sterile (autoclaved) ground calcarenite (Fig. S10) (Benedetti et al., 2023). These and previous results (Banerjee and Joshi, 2014) showed that inactivated (dead) cells were unable to precipitate CaCO3 in the laboratory, suggesting that cells need to be metabolically active for calcification and that cell structure alone is not sufficient to promote bioprecipitation. The most studied bacterial metabolism for CaCO3 precipitation is the ureolytic metabolic pathway. This process involves the production of carbamate (NH2COOH) by urea hydrolysis, which spontaneously hydrolyses to form ammonia (NH3) and carbonic acid (H2CO3). These products react with water to form carbonate (CO), ammonium ions (NH), and hydroxyl ions (OH−), finally resulting in an increase of pH. In an alkaline environment, the presence of calcium ions and the bacterial cells, as a nucleation site, allow for the precipitation of calcium carbonate (Anbu et al., 2016; Hammes et al., 2002; Nigro et al., 2022). Thus, it is not the presence of the bacterium alone, as a structure, that provides evidence of precipitation, but also high pH, metabolism, and negative membrane charge. In laboratory conditions (Fig. S9), if urea and calcium are present in the medium, the mechanism is stimulated and accelerated.
In natural environments, bacteria are centres of nucleation for nanofibre formation. They do not control the mineralisation process directly but induce the precipitation of calcium carbonate by changing the chemistry of the environment as a consequence of their metabolic activity and also serve as nuclei for crystallisation. This mechanism is a result of pH increase, a direct effect of the negatively charged bacterial surface, and the presence of a metabolic ureolytic process (Omoregie et al., 2021).
Overall, our results underscore the role of microorganisms in promoting moonmilk deposition, contributing to the rock formation processes. Nevertheless, to propose moonmilk as part of a geological process, the microbial communities of the rocks and those contributing to moonmilk deposition should have a similar composition. Aiming to identify the rock microbial communities, samples from calcarenite and hybrid sandstone were analysed together with the corresponding moonmilk samples from the Tomba Maggi 2 and Tomba degli Scudi. The results of the 16S small subunit (SSU) rRNA amplicon sequencing showed a high abundance of Actinobacteria, Bacteroidetes, Cyanobacteria, Firmicutes, and Proteobacteria (Fig. 5 and additional data). Of note, the Firmicutes phylum is abundant, and several members, such as the Lysinibacillus genus, have extremely high urease activity and therefore greatly enhance carbonate precipitation (Banerjee and Joshi, 2014; Benedetti et al., 2023; Zhu and Dittrich 2016), see also Fig. S9d.
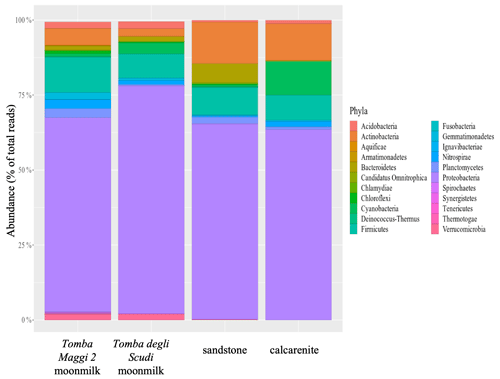
Figure 5Phyla present in the microbial community from moonmilk samples of the Tomba Maggi 2, the Tomba degli Scudi, and their corresponding rocks (calcarenite and hybrid sandstone). The histogram shows the Phylum relative abundance (%) for the analysed samples. Community structure was determined by targeted amplicon sequencing of bacterial 16S rRNA genes. All samples show a high abundance of Actinobacteria, Bacteroidetes, Cyanobacteria, Firmicutes, and Proteobacteria.
Bacterial community diversity was measured by an inverse Simpson index and Shannon index for moonmilk (Tomba degli Scudi and Tomba Maggi 2) and rocks (calcarenite and hybrid sandstone). The indices do not show any significant differences between the samples (Mann–Whitney test, P > 0.05) (Fig. S11). These results show that in moonmilk and rocks the microbial composition is similar, irrespective of rock type (calcarenite and hybrid sandstone) or the environment where the samples were collected (outdoor or indoor). It should be noted that 16S SSU rRNA analysis does not provide information about metabolic activity; thus, these data do not identify microorganisms that are active in CaCO3 deposition, but the overall data demonstrate that the endolytic community of the rocks is promoting moonmilk deposition. The results presented also revealed the presence of organomineralisation and calcite nanofibres that originate from bacterial entombment, not only on the surface but also inside the rocks. The presence of a resident microbial community deep within the rocks possibly evolved with the rocks through geological time. Therefore, no habitat should be considered as extreme for the resident microbial community, and the rocks should not be considered as a “refuge” for escaping extreme environmental conditions. Biological research should focus on microbial community evolution with respect to the geologic substrate in which they are living, considering the natural co-evolution of microbes and rocks, and possibly abstaining from the consideration of the microbial metabolism as an adaptation to adverse environmental conditions.
3.4 Moonmilk as a biosignature
The search for traces of extra-terrestrial life is a complex task often ending with inconclusive results. The co-evolution of minerals and microorganisms has implications for the quest of evidence for life on other planets. The discovery of minerals of undisputable biological origin, rather than organic remains, may provide the most robust signs of biological activity (Hazen et al., 2008). Co-evolution of life and minerals throughout Earth's history lays the foundation for an inclusive search for the presence of life, not only because rocks arose from life but also because life itself may have formed from rocks (Bizzarri et al., 2021; Marshall, 2020; Saladino et al., 2018). Thousands of Earth's minerals owe their existence to the development of life on the planet, and calcium carbonate phases that are massively produced on Earth by microorganisms are the best example (Hazen et al., 2008).
In this work, we have focused on the calcium carbonate nanofibres (moonmilk). Given the tight association and co-evolution between rocks and microbial communities that results in the observed organomineralisation, calcite nanofibres are of interest in the field of astrobiology and are considered to be a potential sign of life. The moonmilk production contributed to rock formation by filling the pores and the cracks in the rocks, while the rock composition and the porosity shaped a microbial community that copes with high calcium content by producing calcite nanofibres. The moonmilk is mainly found in karst caves, but there are also examples of moonmilk bioprecipitation in hypogeal environments carved in different geologic substrates, such as granitoid rocks or sandstone (Miller et al., 2018; Saladino et al., 2018). The moonmilk has also been found in lava tubes, where the microbial communities are similar to those present in the moonmilk that originated from calcarenite (Gonzalez-Pimentel et al., 2021; Miller et al., 2020), raising the possibility of positing the moonmilk as a biosignature also beyond the Earth's calcium carbonate rocks.
The metabarcoding analysis was performed as described in Grottoli et al. (2020).
The sequences were deposited in the NCBI BioProject database under the accession number ID PRJNA1022455 https://www.ncbi.nlm.nih.gov/bioproject/PRJNA1022455 (NCBI, 2023).
The supplement related to this article is available online at: https://doi.org/10.5194/bg-20-4135-2023-supplement.
SR, MB, AC, FB, and FM performed the experiments; MB, SR, and TR collected the samples; RN, AG, and MR supervised and performed the nucleic acid analysis; DFM supervised the sampling in the Etruscan tombs; TR and EDM conceived the study; and TR wrote the paper with contributions from SR, MB, and EDM.
The contact author has declared that none of the authors has any competing interests.
Publisher’s note: Copernicus Publications remains neutral with regard to jurisdictional claims in published maps and institutional affiliations.
This work is dedicated to the memory of Laura Frontali and the restorer Franco Adamo. The authors would like to thank Simone De Amicis for sharing the sampling of the macco quarry of Tarquinia, Carlo Smriglio for the identification of the fossil showed in Fig. S5, Domenico Mannetta for the careful and professional preparation of thin sections, and John Eduard Hallsworth for the discussion and help while preparing the paper. We deeply thank Pierre Zalloua for paper revision and scientific advice. Angela Cirigliano was awarded of the grant Regione Lazio PR FSE 2021–2027. This work was supported by Ateneo Sapienza, 2021 (Sara Ronca).
This research has been supported by Ateneo Sapienza 2021 and DTC Lazio PERGAMO Project 305-2020-35549.
This paper was edited by Chiara Borrelli and reviewed by two anonymous referees.
Allwood, A. C., Walter, M. R., Kamber, B. S., Marshall, C. P., and Burch, I. W.: Stromatolite reef from the Early Archaean era of Australia, Nature, 441, 714–718, https://doi.org/10.1038/nature04764, 2006.
Anbu, P., Kang, C.-H., Shin, Y.-J., and So, J.-S.: Formations of calcium carbonate minerals by bacteria and its multiple applications. SpringerPlus 5, 250, https://doi.org/10.1186/s40064-016-1869-2, 2016.
Asta, M. P., Fernandez-Martinez, A., Alonso, J., Charlet, L., Findling, N., Magnin, V., Ruta, B., Sprung, M., and Westermeier, F.: Nanoscale Ion Dynamics Control on Amorphous Calcium Carbonate Crystallization: Precise Control of Calcite Crystal Sizes, J. Phys. Chem. C., 124, 25645–25656, https://doi.org/10.1021/acs.jpcc.0c08670, 2020.
Banerjee, S. and Joshi, S. R.: Ultrastructural analysis of calcite crystal patterns formed by biofilm bacteria associated with cave speleothems, Journal of Microscopy and Ultrastructure, 2, 217–223, 2014.
Banks, E. D., Taylor, N. M., Gulley, J., Lubbers, B. R., Giarrizzo, J. G., Bullen, H. A, Hoehler, T. M., and Barton, H. A.: Bacterial calcium carbonate precipitation in cave environments: a function of calcium homeostasis, Geomicrobiol. J., 27, 444–454, https://doi.org/10.1080/01490450903485136, 2010.
Barton, H. A. and Northup, D. E.: Geomicrobiology in cave environments: past, current and future perspectives, J. Cave Karst Stud., 69, 163–178, 2007.
Baskar, S., Baskar, R., and Routh, J.: Biogenic evidences of moonmilk deposition in the Mawmluh cave, Meghalaya, India, Geomicrobiol. J., 28, 252–265, https://doi.org/10.1080/01490451.2010.494096, 2011.
Benedetti, F., Kratter, M., Atanasio, P., Mura, F., Beccaccioli, M., Scifo, J., di Sarcina, I., Tomassetti, M. C., Schneider, K., Rossi, M., Cemmi, A., Nigro, L., and Rinaldi, T.: Isolation of carbonatogenic bacteria for biorestoration, J. Cult. Herit., in review, 2023.
Berg, B. L., Ronholm, J., Applin, D. M., Mann, P., Izawa, M., Cloutis, E. A., and Whyte, L. G.: Spectral features of biogenic calcium carbonates and implications for astrobiology, Int. J. Astrobiol., 13, 353, https://doi.org/10.1017/S1473550414000366, 2014.
Bizzarri, B. M., Saladino, R., Delfino, I., García-Ruiz, J. M., and Di Mauro, E.: Prebiotic organic chemistry of formamide and the origin of life in planetary conditions: What we know and what is the future, Int. J. Mol. Sci., 22, 917, https://doi.org/10.3390/ijms22020917, 2021.
Blanco, A., Orofino, V., D'Elia, M., Fonti, S., Mastandrea, A., Guido, A., and Russo, F.: Infrared spectroscopy of microbially induced carbonates and past life on Mars, Icarus, 226, 119–126, https://doi.org/10.1016/j.icarus.2013.05.016, 2013.
Borsato, A., Frisia, S., Jones, B., and Van Der Borg, K.: Calcite moonmilk: crystal morphology and environment of formation in caves in the Italian Alps, J. Sediment. Res., 70, 1171–1182, https://doi.org/10.1306/032300701171, 2000.
Boynton, W. V., Ming, D. W., Kounaves, S. P., Young, S. M. M., Arvidson, R. E., Hecht, M. H., Hoffman, J., Niles, P. B.; Hamara, D. K.; Quinn, R. C., Smith, P. H., Sutter, B., Catling, D. C., and Morris, R. V.: Evidence for calcium carbonate at the Mars Phoenix landing site, Science, 325, 61–64, https://doi.10.1126/science.1172768, 2009.
Braissant, O., Bindschedler, S., Daniels, A. U., Verrecchia, E. P., and Cailleau, G.: Microbiological activities in moonmilk monitored using isothermal microcalorimetry (cave of vers chez le Brandt, Neuchatel, Switzerland), J. Cave Karst Stud., 74, 116–126, https://doi.org/10.4311/2011JCKS0213, 2012.
Bultel, B., Viennet, J. C., Poulet, F., Carter, J., and Werner, S. C.: Detection of carbonates in Martian weathering profiles. J. Geophys. Res.-Sol. Ea., 124, 989–1007, https://doi.org/10.1029/2018JE005845, 2019.
Cacchio, P., Ferrini, G., Ercole, C., Del Gallo, M., and Lepidi, A.: Biogenicity and characterization of moonmilk in the grotta nera (Majella National Park, Abruzzi, Central Italy), J. Cave Karst Stud., 76, 88, https://doi.org/10.4311/2012MB0275, 2014.
Cailleau, G., Verrecchia, E. P., Braissant, O., and Emmanuel, L.: The biogenic origin of needle fiber calcite, Sedimentology, 56, 1858–1875, https://doi.org/10.1111/j.1365-3091.2009.01060.x, 2009.
Cañaveras, J. C., Cuezva, S., Sanchez-Moral, S., Lario, J., Laiz, L., Gonzalez, J. M., and Saiz-Jimenez, C.: On the origin of fiber calcite crystals in moonmilk deposits, Naturwissenschaften, 93, 27–32, https://doi.org/10.1007/s00114-005-0052-3, 2006.
Changela, H. G., Chatzitheodoridis, E., Antunes, A., Beaty, D., Bouw, K., Bridges, J. C., Capova, K. A.; Cockell, C. S., Conley, C. A., Dadachova, E., Dallas, T. D., de Mey, S., Dong, C., Ellery, A., Ferus, M., Foing, B., Fu, X., Fujita, K., Lin, Y., Jheeta, S., Hicks, L. J., Hu, S., Kereszturi, A., Krassakis, A., Liu, Y., Oberst, J., Michalski, J.,Ranjith, P. M., Rinaldi, T., Rothery, D., Stavrakakis, H. A., Selbmann, L., Sinha, R. K., Wang, A., Williford, K., Vaci, Z., Vago, J. L., Waltemathe, M., and Hallsworth, J. E.: Mars: new insights and unresolved questions, Int. J. Astrobiol., 20, 394–426, https://doi.org/10.1017/S1473550421000276, 2021.
Cirigliano, A., Tomassetti, M.C., Di Pietro, M., Mura, F., Maneschi, M. L., Gentili, M. D., Cardazzo, B., Arrighi, C., Mazzoni, C., Negri, R., and Rinaldi, T.: Calcite moonmilk of microbial origin in the etruscan Tomba degli Scudi in Tarquinia, Italy, Sci. Rep., 8, 1–10, https://doi.org/10.1038/s41598-018-34134-y, 2018.
Cirigliano, A., Mura, F., Cecchini, A., Tomassetti, M. C., Maras, D. F., Di Paola, M., Meriggi, N., Cavalieri, D., Negri, R., Quagliariello, A., Hallsworth, J. E., and Rinaldi, T.: Active microbial ecosystem in Iron-Age tombs of the Etruscan civilization, Appl. Environ. Microbiol., 23, 3957-3969, https://doi.org/10.1111/1462-2920.15327, 2021a.
Cirigliano, A., Mura, F., Quagliarello, A., Negri, R., and Rinaldi, T.: Calcium carbonate of microbial origin in the Etruscan tombs of Tarquinia, 1st Italian Space Agency Workshop on Astrobiology. Memorie della Società Astronomica Italiana/ Journal of the Italian Astronomical Society, Fabrizio Serra Editore, Pisa-Roma, 92, 64–66, 2021b.
Compère, P., Carnol, M., Barton, H. A., and Rigali, S.: Assessment of the potential role of Streptomyces in cave moonmilk formation, Front. Microbiol., 8, 1181, https://doi.org/10.3389/fmicb.2017.01181, 2017.
Cosmidis, J. and Benzerara, K.: Why do microbes make minerals?, C. R. Géosci., 354, 1–39, https://doi.org/10.5802/crgeos.107, 2022.
Cuadros, J.: Clay minerals interaction with microorganisms: A review, Clay Miner., 52, 235–261, https://doi.org/10.1180/claymin.2017.052.2.05, 2017.
Dhami, N. K., Reddy, M. S., and Mukherjee, A.: Synergistic role of bacterial urease and carbonic anhydrase in carbonate mineralization, Biotechnol. Appl. Bioc., 172, 2552–2561, https://doi.org/10.1007/s12010-013-0694-0, 2014.
Diloreto, Z. A., Garg, S., Bontognali, T. R., and Dittrich, M.: Modern dolomite formation caused by seasonal cycling of oxygenic phototrophs and anoxygenic phototrophs in a hypersaline sabkha, Sci. Rep., 11, 4170, https://doi.org/10.1038/s41598-021-83676-1, 2021.
Dupraz, C., Reid, R. P., Braissant, O., Decho, A. W., Norman, R. S., and Visscher, P. T.: Processes of carbonate precipitation in modern microbial mats, Earth-Sci. Rev., 96, 141–162, https://doi.org/10.1016/j.earscirev.2008.10.005, 2009.
Ehlmann, B. L., Mustard, J. F., Murchie, S. L., Poulet, F., Bishop, J. L., Brown, A. J., Calvin, W. M., Clark, R. N., Des Marais, D. J., Milliken, R. E., Roach, L. H., Roush, T. L., Swayze, G. A., and Wray, J. J.: Orbital identification of carbonate-bearing rocks on Mars, Science, 322, 1828–1832, https://doi.org/10.1126/science.1164759, 2008.
Gonzalez-Pimentel, J. L., Martin-Pozas, T., Jurado, V., Miller, A. Z., Caldeira, A. T., Fernandez-Lorenzo, O., Sanchez-Moral, S., and Saiz-Jimenez, C.: Prokaryotic communities from a lava tube cave in La Palma Island (Spain) are involved in the biogeochemical cycle of major elements, PeerJ, 9, e11386, https://doi.org/10.7717/peerj.11386, 2021.
Görgen, S., Benzerara, K., Skouri-Panet, F., Gugger, M., Chauvat, F., and Cassier-Chauvat, C.: The diversity of molecular mechanisms of carbonate biomineralization by bacteria, Discov. Mater., 1, 2, https://doi.org/10.1007/s43939-020-00001-9, 2021.
Grosch, E. G. and Hazen, R. M.: Microbes, mineral evolution, and the rise of microcontinents–origin and coevolution of life with early earth, Astrobiology, 15, 922–939, https://doi.org/10.1089/ast.2015.1302, 2015.
Grottoli, A., Beccaccioli, M., Zoppis, E., Fratini, R. S., Schifano, E., Santarelli, M. L., Uccelletti, D., and Reverberi, M.: Nanopore sequencing and bioinformatics for rapidly identifying cultural heritage spoilage microorganisms, Front. Mater. Sci. 7, 14, https://doi.org/10.3389/fmats.2020.00014, 2020.
Grotzinger, J. P. and James, N. P.: Precambrian carbonates: evolution of understanding, in: Carbonate Sedimentation and Diagenesis in the Evolving Precambrian World, edited by: Grotzinger, J. P. and James, N. P., Society for Sedimentary Geology Special Publications, 67, 3–22, ISBN 978-1-56576-072-1, 2000.
Hammes, F. and Verstraete, W.: Key roles of pH and calcium metabolism in microbial carbonate precipitation, Rev. Environ. Sci. Bio., 1, 3–7, https://doi.org/10.1023/A:1015135629155, 2002.
Hartmann, J., Dürr, H. H., Moosdorf, N., Meybeck, M., and Kempe, S.: The geochemical composition of the terrestrial surface (without soils) and comparison with the upper continental crust, Int. J. Earth. Sci., 101, 365–376, https://doi.org/10.1007/s00531-010-0635-x, 2012.
Hazen, R. M., Papineau, D., Bleeker, W., Downs, R. T., Ferry, J. M., McCoy, T. J., Sverjensky, D. A., and Yang, H.: Mineral evolution, Am. Mineral., 93, 1693–1720, https://doi.org/10.2138/am.2008.2955, 2008.
Javaux, E. J.: Challenges in evidencing the earliest traces of life, Nature, 572, 451–460, https://doi.org/10.1038/s41586-019-1436-4, 2019.
Jones, B. and Peng, X.: Abiogenic growth of needle-fiber calcite in spring towers at Shiqiang, Yunnan Province, China, J. Sediment. Res., 84, 1021–1040, https://doi.org/10.2110/jsr.2014.82, 2014.
Kaczmarek, S. E., Gregg, J. M., Bish, D. L., Machel, H. G., Fouke, B. W., MacNeil, A., Lonnee, J., and Wood, R.: Dolomite, very high-magnesium calcite, and microbes – Implication for the microbial model of dolomitization, in: Characterization and Modeling of Carbonates–Mountjoy Symposium 1, SEPM (Society for Sedimentary Geology) 109, edited by: Macneil, A. J., Lonnee, J., Wood, R., https://doi.org/10.2110/sepmsp.109.01, 2017.
Kaplan, H. H., Lauretta, D. S., Simon, A. A., Hamilton, V. E., Della Giustina, D. N., Golish, D. R., Reuter, D. C., Bennett, C. A., Burke, K. N., Campins, H., Connolly Jr., H. C., Dworkin, J. P., Emery, J. P., Glavin, D. P., Glotch, T. D., Hanna, R., Ishimaru, K., Jawin, E. R., Mccoy, T. J., Porter, N., Sandford, S. A., Ferrone, S., Clark, B. E., Li, J.-Y., Zou, X.-D., Daly, M. G., Barnouin, O. S., Seabrook, J. A., and Enos, H. L.: Bright carbonate veins on asteroid (101955) Bennu: Implications for aqueous alteration history, Science, 370, 676, https://doi.org /10.1126/science.abc3557, 2020.
Kolesár, M. and Čurlik, J.: Origin, distribution and transformation of authigenic carbonates in loessic soils, Eur. J. Soil Sci., 4, 38–43, https://doi.org /10.18393/ejss.50910, 2015.
Kondratyeva, L. M., Shadrina, O. S., Litvinenko, Z. N., and Golubeva, E. M.: Biogeochemical investigations of the speleothem moonmilk in the karst Proschalnaya cave (far east, Russia), J. Cave Karst Stud., 82, 95–105, https://doi.org/10.4311/2019MB0106, 2020.
Lacelle, D., Lauriol, B., and Clark, I. D.: Seasonal isotopic imprint in moonmilk from Caverne de l'Ours (Quebec, Canada): implications for climatic reconstruction, Canad. J. Earth Sci., 41, 1411–1423, https://doi.org/10.1139/e04-080, 2004.
Lee, M. R., Lindgren, P., and Sofe, M. R.: Aragonite, breunnerite, calcite and dolomite in the CM carbonaceous chondrites: High fidelity recorders of progressive parent body aqueous alteration, Geochim. Cosmochim. Ac., 144, 126–156, https://doi.org/10.1016/j.gca.2014.08.019, 2014.
Maciejewska, M., Adam, D., Naômé, A., Martinet, L., Tenconi, E., Całusinska, M., Delfosse, P., Hanikenne, M., Baurain, D., Compère, P., Carnol, M., Barton, H. A., and and Rigali, S.: Assessment of the Potential Role of Streptomyces in Cave Moonmilk Formation, Front. Microbiol, 8, 1181, https://doi.org/10.3389/fmicb.2017.01181, 2017.
Marshall, M.: The water paradox and the origins of life, Nature, 588, 210–213, https://doi.org/10.1038/d41586-020-03461-4, 2020.
Michalski, J. R. and Niles, P. B.: Deep crustal carbonate rocks exposed by meteor impact on Mars, Nat. Geosci., 3, 751–755, https://doi.org/10.1038/ngeo971, 2010.
Miller, A. Z., Garcia-Sanchez, A. M., Martin-Sanchez, P. M., Costa Pereira, M. F., Spangenberg, J. E., Jurado, V., Dionísio, A., Afonso, M. J., Iglé sias Chaminé, H. I., Hermosin, B., and Saiz-Jimenez, C.: Origin of abundant moonmilk deposits in a subsurface granitic environment, Sedimentology, 65, 1482–1503, https://doi.org/10.1111/sed.12431, 2018.
Miller, A. Z., García-Sánchez, A. M., Coutinho, M. L., Costa Pereira, M. F., Gázquez, F., Calaforra, J. M., Forti, P., Martínez-Frías, J., Toulkeridis, T., Caldeira, A. T., and Saiz-Jimenez, C.: Colored microbial coatings in show caves from the Galapagos Islands (Ecuador): first microbiological approach, Coatings, 10, 1134, https://doi.org/10.3390/coatings10111134, 2020.
Millière, L., Gussone, N., Moritz, T., Bindschedler, S., and Verrecchia, E. P.: Origin of strontium and calcium in pedogenic needle fibre calcite (NFC), Chem. Geol., 524, 329–344, https://doi.org/10.1016/j.chemgeo.2019.06.022, 2019.
Morris, R. V., Ruff, S. W., Gellert, R., Ming, D. W., Arvidson, R. E., Clark, B. C., Golden, D. C., Siebach, K., Klingelhöfer, G., Schröder, C., Fleischer, I., Yen, A. S., and Squyres, S. W.: Identification of carbonate-rich outcrops on Mars by the Spirit rover, Science, 329, 1189667, https://doi.org/10.1126/science.118966, 2010.
Mura, F., Cirigliano, A., Bracciale, M. P., and Rinaldi, T.: Characterization of Nanostructured Calcium Carbonate found in two ancient Etruscan tombs, AIP Conf. Proc., 2257, 020011, https://doi.org/10.1063/5.0023677, 2020.
Mura, F., Cirigliano, A., Maras, D., and Rinaldi, T.: Analysis of moonmilk nanofibers in the etruscan tombs of Tarquinia, AIP Conf. Proc., 2416, 020014, https://doi.org/10.1063/5.0068804, 2021.
National Center for Biotechnology Information (NCBI): Biogenic calcium carbonate as evidence for life, https://www.ncbi.nlm.nih.gov/bioproject/PRJNA1022455, last access: 29 September 2023.
Nigro, L., Mura, F., Toti, M. P., Cirigliano, A., and Rinaldi, T.: Carbonatogenic bacteria on the “Motya Charioteer” sculpture, J. Cult. Herit., 57, 256–264, https://doi.org/10.1016/j.culher.2022.09.009, 2022.
Omoregie, A. I., Palombo, E. A., and Nissom, P. M.: Bioprecipitation of calcium carbonate mediated by ureolysis: A review, Environ. Eng. Res., 26, 200379, https://doi.org/10.4491/eer.2020.379, 2021.
Pilorget, C., Okada, T., Hamm, V., Brunetto, R., Yada, T., Loizeau, D., Riu, L., Usui, T., Moussi-Soffys, A., Hatakeda, K., Nakato, A., Yogata, K., Abe, M., Aléon-Toppani, A., Carter, J., Chaigneau, M., Crane, B., Gondet, B., Kumagai, K., Langevin, Y., Lantz, C., Le Pivert-Jolivet, T., Lequertier, G., Lourit, L., Miyazaki, A., Nishimura, M., Poulet, F., Arakawa, M., Hirata, N., Kitazato, K., Nakazawa, S., Namiki, N., Saiki, T., Sugita, S., Tachibana, S., Tanaka, S., Yoshikawa, M., Tsuda, Y., Watanabe, S., and Bibring, J. P.: First compositional analysis of Ryugu samples by the MicrOmega hyperspectral microscope, Nat. Astron., 6, 1–5, https://doi.org/10.1038/s41550-021-01549-z, 2021.
Pomar, L.: Carbonate systems Regional Geology and Tectonics: Principles of Geologic Analysis, Elsevier, 1, 235–311, https://doi.org/10.1016/B978-0-444-64134-2.00013-4, 2020.
Portillo, M. C. and Gonzalez, J. M.: Moonmilk deposits originate from specific bacterial communities in Altamira Cave (Spain), Microb. Ecol., 61, 182–189, https://doi.org/10.1007/s00248-010-9731-5, 2011.
Riding, R.: Microbialites, Stromatolites, and Thrombolites, in: Encyclopedia of Geobiology, edited by: Reitner, J. and Thiel, V., Encyclopedia of Earth Sciences Series, Springer, Dordrecht, 635–654, https://doi.org/10.1007/978-1-4020-9212-1_196, 2011.
Rodriguez-Navarro, C., Cizer, Ö., Kudłacz, K., Ibañez-Velasco, A., Ruiz-Agudo, C., Elert, K., Burgos-Cara, A., and Ruiz-Agudo, E.: The multiple roles of carbonic anhydrase in calcium carbonate mineralization, Cryst. Eng. Comm., 21, 7407–7423, https://doi.org/10.1039/C9CE01544B, 2019.
Ruggieri, R. and Trippetta, F.: Petrophysical properties variation of bitumen-bearing carbonates at increasing temperatures from laboratory to model, Geophysics, 5, 1SO-Z24, https://doi.org/10.1190/geo2019-0790.1, 2020.
Saladino, R., Botta, L., and Di Mauro, E.: The prevailing catalytic role of meteorites in formamide prebiotic processes, Life, 8, 6, https://doi.org/10.3390/life8010006, 2018.
Siesser, W. G. and Rogers, J.: An investigation of the suitability of four methods used in routine carbonate analysis of marine sediments, Deep-Sea Res., 16, 135–139, https://doi.org/10.1016/0011-7471(71)90021-0, 1971.
Sumner, D. Y., and Grotzinger, J.P.: Herringbone calcite: petrography and environmental Significance, J. Sediment. Res., 66, 419–429, https://doi.org/10.1306/D4268360-2B26-11D7-8648000102C1865D, 1996.
Tomassetti, M. C., Cirigliano, A., Arrighi, C., Negri, R., Mura, F., Maneschi, M. L., Gentili, M. D., Stirpe, M., Mazzoni, C. and Rinaldi, T.: A role for microbial selection in frescoes' deterioration in Tomba degli Scudi in Tarquinia, Italy. Sci. Rep., 7, 6027, https://doi.org/10.1038/s41598-017-06169-0, 2017.
Trippetta, F., Ruggieri, R., Brandano, M., and Giorgetti, C.: Petrophysical properties of heavy oil-bearing carbonate rocks and their implications on petroleum system evolution: Insights from the Majella Massif, Mar. Petrol. Geol., 111, 350–362, https://doi.org/10.1016/j.marpetgeo.2019.08.035, 2020.
Veizer, J., Hoefs, J., Ridler, R. H., Jensen, L. S., and Lowe, D. R.: Geochemistry of Precambrian carbonates: I. Archean hydrothermal systems, Geochim. Cosmochim. Ac., 53, 845–857, https://doi.org/10.1016/0016-7037(89)90030-6, 1989.
Voosen, P.: NASA mission set to sample carbon-rich asteroid, Science, 370, 158, https://doi.org/10.1126/science.370.6513.158, 2020.
Wood, D. E., Lu, J., and Langmead, B.: Improved metagenomic analysis with Kraken 2, Genome. Biol. 20, 257, https://doi.org/10.1186/s13059-019-1891-0, 2019.
Zhu, T. and Dittrich, M.: Carbonate precipitation through microbial activities in natural environment, and their potential in biotechnology: a review, Frontiers in bioengineering and biotechnology, 4, 4, https://doi.org/10.3389/fbioe.2016.00004, 2016.