the Creative Commons Attribution 4.0 License.
the Creative Commons Attribution 4.0 License.
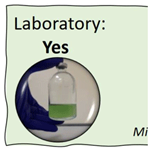
Nitrous oxide (N2O) synthesis by the freshwater cyanobacterium Microcystis aeruginosa
Federico Fabisik
Benoit Guieysse
Jonathan Procter
Pure cultures of the freshwater cyanobacterium Microcystis aeruginosa synthesized nitrous oxide (N2O) when supplied with nitrite (NO) in darkness (198.9 nmol g-DW−1 h−1 after 24 h) and illumination (163.1 nmol g-DW−1 h−1 after 24 h), whereas N2O production was negligible in abiotic controls supplied with NO and in cultures deprived of exogenous nitrogen. N2O production was also positively correlated to the initial NO and M. aeruginosa concentrations but was low to negligible when nitrate (NO) and ammonium (NH) were supplied as the sole exogenous N source instead of NO. A protein database search revealed that M. aeruginosa possesses protein homologous to eukaryotic microalgae enzymes known to catalyze the successive reduction of NO into nitric oxide (NO) and N2O. Our laboratory study is the first demonstration that M. aeruginosa possesses the ability to synthesize N2O. As M. aeruginosa is a bloom-forming cyanobacterium found globally, further research (including field monitoring) is now needed to establish the significance of N2O synthesis by M. aeruginosa under relevant conditions (especially in terms of N supply). Further work is also needed to confirm the biochemical pathway and potential function of this synthesis.
- Article
(592 KB) - Full-text XML
-
Supplement
(423 KB) - BibTeX
- EndNote
Emissions of the potent ozone-depleting greenhouse gas nitrous oxide (N2O) have been reported from various aquatic ecosystems characterized by a high level of photosynthetic activity, and several authors have suggested that N2O emissions from eutrophic lakes could be globally significant (DelSontro et al., 2018; Plouviez et al., 2019a). Noteworthily, DelSontro et al. (2018) determined that N2O emissions from lakes and impoundments could be expected to increase as a function of lake size and chlorophyll a (an indicator of the presence of a primary producer such as microalgae). Because eutrophication is an increasing global issue (DelSontro et al., 2018; Kapsalis and Kalavrouziotis, 2021; Maure et al., 2021), N2O emissions from these ecosystems could also be expected to increase. Several species of microalgae and Cyanobacteria can indeed synthesize N2O (Weathers, 1984; Weathers and Niedzielski, 1986; Bauer et al., 2016; Plouviez et al., 2019a), and a biochemical pathway for this synthesis has been established in the model microalga Chlamydomonas reinhardtii (Plouviez et al., 2017b; Burlacot et al., 2020). Despite these critical advances, the true global significance of microalgal N2O synthesis in microalgae-rich, eutrophic aquatic bodies remains unknown (Plouviez et al., 2019a; Burlacot et al., 2020; Plouviez and Guieysse, 2020). Microcystis species are Cyanobacteria commonly found in eutrophic ecosystems (Xiao et al., 2018; Zhou et al., 2020; Hernandez-Zamora et al., 2021), but the ability of this genus to synthesize N2O is currently unknown. We, therefore, investigated the ability of N2O production by the most notorious bloom-forming cyanobacterium reported in freshwaters and model cyanobacterium Microcystis aeruginosa (Qian et al., 2010; Kataoka et al., 2020; Zhou et al., 2020) under conditions known to induce or impact N2O production in microalgae (Guieysse et al., 2013; Alcántara et al., 2015; Bauer et al., 2016; Plouviez et al., 2017b; Burlacot et al., 2020).
2.1 N2O synthesis bioassays
The ability of M. aeruginosa to synthesize N2O was investigated using a protocol successfully used for the microalgae Chlorella vulgaris and C. reinhardtii (Alcántara et al., 2015; Guieysse et al., 2013; Plouviez et al., 2017b). As can be seen in Fig. 1, N2O production was only recorded in cultures supplied NO, as there was no significant production in the absence of the cyanobacterium (abiotic control) or the absence of NO (negative control). Further assays showed a positive correlation between biomass concentration and N2O production (Fig. 2), confirming the biological origin of N2O synthesis.
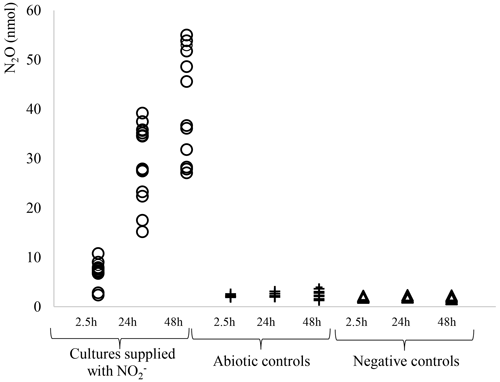
Figure 1Total N2O accumulation (nmol) in M. aeruginosa cultures supplied with 10 mM NO under continuous illumination (∘, n≥12), in the abiotic controls (N-free media supplied with 10 mM NO; +, n≥10), and in the negative controls (M. aeruginosa cultures incubated in N-free media; △, n≥10).
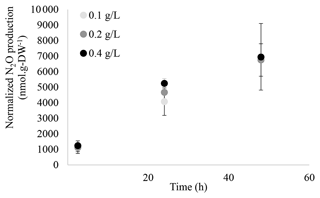
Figure 2Normalized N2O production (nmol g-DW−1) recorded from M. aeruginosa cultures with different biomass concentrations (0.1, 0.2 and 0.4 g-DW L−1; n≥6, n≥12, n=4, respectively) in sealed flasks supplied with light and 10 mM NO. N2O synthesis was statistically different when comparing the rates between 2.5 and 24 h and between 24 and 48 h (p<0.05, two-samples t test). Specific N2O production rates (nmol N2O g-DW−1 h−1) can be found in Table S1.1.
In comparison to cultures supplied with NO, low and negligible N2O synthesis was recorded in cultures supplied with NO and NH, respectively (Table 1). This result showed that NO was the substrate to N2O synthesis, as reported for other microalgae (Weathers, 1984; Weathers and Niedzielski, 1986; Guieysse et al., 2013; Alcántara et al., 2015; Bauer et al., 2016; Plouviez et al., 2017b; Burlacot et al., 2020). The positive correlation between NO concentration and N2O synthesis also confirmed that NO was the substrate to N2O synthesis (Fig. S2.1, Vmax = 185 nmol g-DW−1 h−1 and Km for NO mM) calculated using the Michaelis–Menten equation described by Johnson and Goody (2011).
M. aeruginosa was able to synthesize N2O in both darkness and illumination (Table 1), representing 0.07 % and 0.06 % of the amount of N supplied (g-N-N2O produced g-N−1 supplied ×100) respectively. The N2O produced under illumination was statistically lower than in darkness (p value <0.05, two-samples t test, n=5 replicates from experiments performed on the same day). The negative impact of light was previously observed in C. vulgaris and C. reinhardtii tested under similar conditions (Guieysse et al., 2013; Alcántara et al., 2015; Plouviez et al., 2017b), although N2O production was positively correlated with light supply in C. vulgaris grown outdoors (Plouviez et al., 2017a). The difference we observed during this study may be explained by light-dependent mechanisms impacting enzymatic activities and, consequently, intracellular NO accumulation (e.g., the rates of NO reduction into NH and N2O), as suggested by Plouviez et al. (2017a). However, O2 production during photosynthesis could also influence N2O synthesis. Burlacot et al. (2020) indeed reported that one of the enzymes involved in nitric oxide (NO) reduction to N2O (flavodiiron, as discussed in the next section) can also catalyze the reduction of O2 into H2O. Because of this dual activity and the reactivity of NO with O2, N2O production could be sensitive to O2. Further research is therefore needed to understand if O2 influences N2O production by competitive NO conversion to products such as nitrogen oxides and peroxynitrite and/or by competitive O2 reduction into H2O instead of its reduction to N2O by the enzymes with nitric-reductase ability.
While small, N2O synthesis was statistically significant in M. aeruginosa fed NO as the sole exogenous N source (p value <0.05, two-samples t test when compared with the negative controls). As in C. vulgaris and C. reinhardtii, the intracellular reduction of NO into NO by the enzyme nitrate reductase (narB) is the first step of NO assimilation in M. aeruginosa (Ohashi et al., 2011; Zhou et al., 2020). Hence, intracellular NO production likely generated this substrate for N2O synthesis during NO exogenous supply, but competitive use of NO (for protein synthesis via NH generation) could have competed with N2O synthesis. Intracellular NO production and accumulation is not expected when cells assimilate NH (Plouviez et al., 2019a), explaining the absence of N2O production in the flasks supplied NH as the sole exogenous N source (p value = 0.91, two-samples t test when compared with the negative controls). In M. aeruginosa, NO uptake and the transcriptional regulation of nitrate reductase have been shown to be activated by light, NO and NO (Chen et al., 2009; Ohashi et al., 2011; Chen and Liu, 2015). While the transcriptional and post-translational regulation of nitrate reductase in M. aeruginosa still needs to be investigated in relation to N2O synthesis and varying environmental parameters (e.g., light supply), it is possible that the pattern of N2O synthesis during outdoor M. aeruginosa growth would be similar to that seen in C. vulgaris.
2.2 Putative pathways
In the eukaryotic microalga C. reinhardtii, cytoplasmic NO is sequentially reduced to NO and N2O. The first step, NO reduction into NO, is catalyzed by the dual enzyme nitrate reductase–NO-forming nitrite reductase (NR–NoFNiR) or, potentially, the copper-containing nitrite reductase (NirK). The second step, NO reduction into N2O, can then be catalyzed by cytochrome P450 (CYP55, Plouviez et al., 2017b; Burlacot et al., 2020), flavodiirons (FLVs, Burlacot et al., 2020; Bellido-Pedraza et al., 2020) or, potentially, the hybrid cluster proteins (HCPs, Bellido-Pedraza et al., 2020) involved in nitrogen metabolism (van Lis et al., 2020). Interestingly, NO reduction into NO by nitrate reductase (narB) has been demonstrated in M. aeruginosa (Tang et al., 2011; Song et al., 2017), and here we found that M. aeruginosa possesses homologs of the CYP55, FLVs, and HCPs found in C. reinhardtii (Table 2). While the functions of these proteins need to be confirmed, their presence suggests that N2O synthesis in M. aeruginosa could involve similar NO and NO reduction pathways to those found in C. reinhardtii.
Table 2Summary of BLASTP results for proteins potentially involved in N2O synthesis in Chlamydomonas reinhardtii. Accession numbers were retrieved from Bellido-Pedraza et al. (2020) and used as a query sequence for BLASTP (protein–protein BLAST) protein searches (https://blast.ncbi.nlm.nih.gov/Blast.cgi, last access: 20 September 2022) of the M. aeruginosa (taxid:1126) protein sequences database.
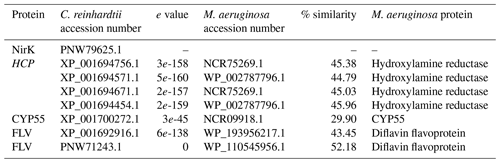
2.3 Metabolic function
The metabolic function of N2O synthesis in eukaryotic microalgae is currently unknown, and it has been suggested that NOreduction into N2O enables cells to expend excess energy or, instead, is the fortuitous result of dual enzymatic activity (Guieysse et al., 2013; Plouviez et al., 2017b). The intermediate NO is a ubiquitous signalling molecule in algae (Astier et al., 2021). Interestingly, in M. aeruginosa NO stimulates the production of secondary metabolites (e.g., linoleic acid) which inhibit the growth of competitors (Song et al., 2017). NO also promotes the growth of this cyanobacterium (Tang et al., 2011). While the link between NO and N2O still needs to be determined, it is possible that the NO and N2O biosynthetic pathways are involved in cell-to-cell communications in M. aeruginosa and, more broadly, in microalgae.
2.4 Potential environmental implications
Microalgae species from at least three divisions (Bacillariophyta, Chlorophyta, Cyanobacteria) have the ability to synthesize NO (Kim et al., 2008; Kumar et al., 2015; Plouviez et al., 2017b; Tang et al., 2011) and/or N2O (Weathers, 1984; Weathers and Niedzielski, 1986; Guieysse et al., 2013; Kamp et al., 2013; Plouviez et al., 2017a, b, this study). All these observations suggest that the ability to synthesize N2O is widely distributed among microalgae. Critically, N2O emissions from aquatic environments where microalgae abound, such as oceans, lakes and engineered cultivation systems, have been repeatedly reported (Bauer et al., 2016; Plouviez et al., 2019a, b; Zhang et al., 2022) even under very low exogenous NO concentrations (Plouviez et al., 2019b). These emissions can be explained by intracellular NO production during reductive nitrate assimilation (Plouviez et al., 2017a, b, 2019b) under conditions when excess NO production (Bristow et al., 2015; French et al., 1983; Mortonson and Brooks, 1980; Schaefer and Hollibaugh, 2018) could support N2O synthesis.
Based on the data available, DelSontro et al. (2018) and Plouviez and Guieysse (2020) estimated that global N2O emissions from eutrophic lakes alone could represent 110 to 450 kt N-N2O yr−1, which represents 14 %–56 % of the natural and anthropogenic N2O emissions reported from inland and coastal waters (Tian et al., 2020). Importantly, DelSontro et al. (2018) predicted that N2O emissions from lakes and impoundments would increase with lake size and chlorophyll a concentration. The N2O synthesis rates reported during our study are in the same order of magnitude as the rate previously reported for members of the green microalgae, Cyanobacteria and diatoms (Bauer et al., 2016; Plouviez et al., 2019a). However, we cannot conclude that M. aeruginosa (or other species) is or is not a major N2O producer in lakes and other aquatic environments without evidence from field measurements. Indeed, high NO concentrations are rare in natural and engineered ecosystem environments, which would suggest insignificant microalgal N2O production in most contexts. Nevertheless, significant N2O emissions were reported from outdoor cultures of C. vulgaris fed NO (Guieysse et al., 2013; Plouviez et al., 2017a), despite this alga also producing much more N2O when fed NO (Guieysse et al., 2013). Plouviez et al. (2017) suggested that this was caused by NO intracellular accumulation under varying light, as this condition is known to have different impacts on the rate of NO reduction into NO by NR and the rate of NO reduction into NH by NiR (nitrite reductase). During our study, N2O emissions under NO supply were low but not negligible. Because NR activity is also influenced by light and the availabilities of NO and NO in M. aeruginosa (Chen et al., 2009; Ohashi et al., 2011; Chen and Liu, 2015), N2O synthesis by this microalga could possibly occur in environments where NO is the main nitrogen source.
Our findings support past predictions of the global relevance of photosynthetic N2O emissions from eutrophic aquatic bodies, as Microcystis is globally found and often the dominant genus in these ecosystems (Qian et al., 2010; Kataoka et al., 2020; Zhou et al., 2020). The work from Weathers and Niedzielski (1986) and our work suggest that Nostoc spp., Aphanocapsa (PCC 6308), Aphanocapsa (PCC 6714) and M. aeruginosa have the ability to synthesize N2O. Consequently, other Cyanobacteria species may also have this ability. Further research is now needed to quantify N2O emissions from eutrophic aquatic ecosystems where Cyanobacteria abound. This is especially timely considering that the frequency and geographic distribution of harmful algae blooms have increased due to anthropogenic activities (Paerl et al., 2018; Kataoka et al., 2020). In addition, algae blooms can lead to the decrease of O2 in oceans, coastal waters and lakes (Jenny et al., 2015; Rabalais and Turner, 2019), a condition that can increase the accumulation of NO in aquatic ecosystems (Schaefer and Hollibaugh, 2018; Bristow et al., 2015). Because microalgal N2O synthesis is rapid and influenced by factors such as the cell biology (Plouviez et al., 2019b) and, as observed during our study, the type and concentration of the nitrogen source microalgae receive, extensive monitoring (i.e., over the long term with wide spatial coverage and high sampling frequency) of several types of microalgae-rich environments are required (e.g., hypoxic waters).
We herein present the first demonstration that M. aeruginosa synthesizes N2O. Microcystis aeruginosa synthesized N2O when supplied with NO in darkness (198.9 nmol g-DW−1 h−1 after 24 h) and illumination (163.1 nmol g-DW−1 h−1 after 24 h), and this production was positively correlated to the initial NO and M. aeruginosa concentrations. A protein database search also revealed that M. aeruginosa possesses proteins homologous to eukaryotic microalgae known to catalyze the successive reduction of NO into NO and N2O. Further studies are needed to confirm the genes and proteins involved, as a better understanding of the biochemical pathway involved during microalgal N2O synthesis is critical to efficiently monitor (i.e., identify the source) and mitigate N2O emissions.
Our study is further evidence of the ability of photosynthetic microorganisms, especially Cyanobacteria, to synthesize N2O. Preliminary estimation showed that N2O emissions from eutrophic lakes alone could represent 110 to 450 kt N-N2O yr−1, which represents 14 %–56 % of the natural and anthropogenic N2O emissions reported from inland and coastal waters. However, how much microalgae contribute to these emissions is currently unknown. As M. aeruginosa is globally distributed, further research (including field monitoring with wide spatial coverage and high sampling frequency from different aquatic ecosystems) is now needed to evaluate the significance of N2O synthesis by these Cyanobacteria under relevant conditions (especially in terms of N supply).
A1 Strain and culture maintenance
Microcystis aeruginosa UTEX 2385 was obtained from the culture collection of the University of Texas at Austin (https://utex.org/, last access: 4 July 2022). Pure cultures were maintained on 100 mL low-phosphate minimal media (Plouviez et al., 2021), incubated at 25 ∘C (INFORS HT Multitron) under continuous illumination (20 µmol cm−2 s−1) and agitation (150 rotation min−1, rpm). Cultures thus incubated for more than a week were supplied with 100 µL of a solution of KH2PO4 K2HPO4 (0.4 M 0.6 M) to prevent P limitation. The purity of the cultures was verified via sequencing (Sect. S3).
A2 Cultivation and bioassays
M. aeruginosa was cultivated on 400 mL low-phosphorus minimal media in 500 mL Duran bottles for 5 d. These cultures were incubated under fluorescent tubes (F15W/GRO, Sylvania Gro-Lux) providing illumination at 20 µmol cm−2 s−1 at the culture surface. Mixing was provided by bubbling filtered (0.22 µm) air at 1.5 L min−1. On the day of the experiment, 15 mL aliquots were withdrawn from the cultures to measure the cell dry weight (DW) according to Béchet et al. (2015). Then, 100–400 mL aliquots were centrifuged at 4400 rpm for 3 min. The supernatants were discarded, and the pellets were re-suspended in an N-free medium to a final concentration of 0.2 g-DW L−1, as previously described (Guieysse et al., 2013). Twenty-five mL aliquots of these suspensions were transferred into 120 mL serum flasks supplied with 1 mL of NaNO2, NaNO3 or NH4Cl stock solutions (250 mM) to reach a final concentration of 10 mM. Sterile abiotic controls were not inoculated but were supplied with 10 mM nitrite (NO), while negative controls were M. aeruginosa cultures incubated in N-free media. The flasks were immediately sealed with rubber septa and aluminum caps and incubated at 25 ∘C under continuous agitation (150 rpm) under either constant illumination (20 µmol cm−2 s−1) or darkness. A similar protocol was used to evaluate the impact of the initial cell (0.1–0.4 g-DW L−1) and of NO (1–10 mM), nitrate (NO, 10 mM) or ammonium (NH, 10 mM) concentrations on N2O synthesis. Unless otherwise stated, each condition was tested in triplicate flasks and repeated at least twice. All glassware and media were autoclaved prior to the experiments. An additional experiment confirmed the purity of the M. aeruginosa stock cultures and the cultures used during the bioassays (Sect. S3).
A3 Analysis
Gas samples (5 mL) were withdrawn from the flask headspace using a syringe equipped with a needle. The headspace N2O concentration in those samples was then quantified using gas chromatography (Shimadzu GC-2010, Shimadzu, Japan). Total N2O was calculated as the sum of gaseous N2O and dissolved N2O, as described by Guieysse et al. (2013). Briefly, assuming the gas and the liquid phase N2O concentrations were at equilibrium at the time of sampling, the total amount of N2O produced in the flask was calculated by summing up the amounts of N2O present in the gas and liquid phases. The amount of dissolved N2O in the liquid phase was calculated using Henry's law at 25 ∘C (Eq. 1):
where is the total amount of N2O produced in the Duran bottle at time t (moles N2O), is the molar fraction of N2O in the gas phase at time t (mol N2O mol gas−1), Pt is the pressure in the gas headspace at time t (typically 101 325 Pa unless otherwise stated), Vg is the volume of gas in the flask (mL), R is the ideal gas constant (8.314 J mol−1 K−1), T is the temperature inside the bottle (298.15 K), is the Henry's law constant of N2O at T (2.5 10−7 mol L−1 Pa−1), and Vl is the volume of liquid in the serum flask (mL).
Data mentioned in Sect. 2.4 can be found in the cited literature, i.e., DelSontro et al. (2018) and Plouviez and Guieysse (2020). All the data from our study are presented numerically in the paper and in the Supplement.
Specific N2O production rates are presented in Table S1.1. The enzymatic kinetic of N2O synthesis in M. aeruginosa is shown in Fig. S2.1. The purity of M. aeruginosa cultures was confirmed by PCR and sequencing; the methodology used and the results obtained are presented in the Supplement S3. The supplement related to this article is available online at: https://doi.org/10.5194/bg-20-687-2023-supplement.
FF performed the investigation, data visualization and curation and contributed to the writing (review and editing) of the paper. MP was involved with the writing (original draft) and contributed to conceptualization, methodology and data curation, as well as visualization with BG. BG and JP were involved in the writing (review and editing) of the paper before submission. Finally, BG, JP and MP were all involved with the funding acquisition.
The contact author has declared that none of the authors has any competing interests.
Publisher’s note: Copernicus Publications remains neutral with regard to jurisdictional claims in published maps and institutional affiliations.
Massey University and the Greenhouse Gas Inventory Research Fund Funding, administrated by the Ministry for Primary Industries (project no. 406614) are gratefully acknowledged. The authors also wish to thank Alexander Cliff for providing a culture aliquot and Trish McLenachan for her assistance with part of the work involving sequencing.
This research has been supported by the Ministry for Primary Industries (grant no. 406614) and by Massey University.
This paper was edited by Tina Treude and Steven Bouillon and reviewed by three anonymous referees.
Alcántara, C., Muñoz, R., Norvill, Z., Plouviez, M., and Guieysse, B.: Nitrous oxide emissions from high rate algal ponds treating domestic wastewater, Bioresour. Technol., 177, 110–117, https://doi.org/10.1016/j.biortech.2014.10.134, 2015.
Astier, J., Rossi, J., Chatelain, P., Klinguer, A., Besson-Bard, A., Rosnoblet, C., Jeandroz, S., Nicolas-Frances, V., and Wendehenne, D.: Nitric oxide production and signalling in algae, J. Exp. Bot., 72, 781–792, https://doi.org/10.1093/jxb/eraa421, 2021.
Bauer, S. K., Grotz, L. S., Connelly, E. B., and Colosi, L. M.: Reevaluation of the global warming impacts of algae-derived biofuels to account for possible contributions of nitrous oxide, Bioresour Technol, 218, 196–201, https://doi.org/10.1016/j.biortech.2016.06.058, 2016.
Béchet, Q., Chambonniere, P., Shilton, A., Guizard, G., and Guieysse, B.: Algal productivity modeling: a step toward accurate assessments of full-scale algal cultivation, Biotechnol. Bioeng., 112, 987–996, https://doi.org/10.1002/bit.25517, 2015.
Bellido-Pedraza, C. M., Calatrava, V., Sanz-Luque, E., Tejada-Jimenez, M., Llamas, A., Plouviez, M., Guieysse, B., Fernandez, E., and Galvan, A.: Chlamydomonas reinhardtii, an Algal Model in the Nitrogen Cycle, Plants, 9, 903, https://doi.org/10.3390/plants9070903, 2020.
Bristow, L. A., Sarode, N., Cartee, J., Caro-Quintero, A., Thamdrup, B., and Stewart, F. J.: Biogeochemical and metagenomic analysis of nitrite accumulation in the Gulf of Mexico hypoxic zone, Limnol. Oceanogr. Lett., 60, 1733–1750, https://doi.org/10.1002/lno.10130, 2015.
Burlacot, A., Richaud, P., Gosset, A., Li-Beisson, Y., and Peltier, G.: Algal photosynthesis converts nitric oxide into nitrous oxide, P. Natl. Acad. Sci. USA, 117, 2704–2709, https://doi.org/10.1073/pnas.1915276117, 2020.
Chen, W. and Liu, H.: Intracellular nitrite accumulation: The cause of growth inhibition of Microcystis aeruginosa exposure to high nitrite level, Phycol. Res., 63, 197–201, https://doi.org/10.1111/pre.12090, 2015.
Chen, W., Zhang, Q., and Dai, S.: Effects of nitrate on intracellular nitrite and growth of Microcystis aeruginosa, J. Appl. Phycol., 21, 701–706, https://doi.org/10.1007/s10811-009-9405-1, 2009.
DelSontro, T., Beaulieu, J. J., and Downing, J. A.: Greenhouse gas emissions from lakes and impoundments: upscaling in the face of global change, Limnol. Oceanogr. Lett., 3, 64–75, https://doi.org/10.1002/lol2.10073, 2018.
French, D. P., Furnas, M. J., and Smayda, T. J.: Diel changes in nitrite concentration in the chlorophyll maximum in the Gulf of Mexico, Deep-Sea Res. Pt. I, 30, 707–722, https://doi.org/10.1016/0198-0149(83)90018-3, 1983.
Guieysse, B., Plouviez, M., Coilhac, M., and Cazali, L.: Nitrous Oxide (N2O) production in axenic Chlorella vulgaris microalgae cultures: evidence, putative pathways, and potential environmental impacts, Biogeosciences, 10, 6737–6746, https://doi.org/10.5194/bg-10-6737-2013, 2013.
Hernandez-Zamora, M., Santiago-Martinez, E., and Martinez-Jeronimo, F.: Toxigenic Microcystis aeruginosa (Cyanobacteria) affects the population growth of two common green microalgae: Evidence of other allelopathic metabolites different to cyanotoxins, J. Phycol., 57, 1530–1541, https://doi.org/10.1111/jpy.13185, 2021.
Jenny, J.-P., Francus, P., Normandeau, A., Lapointe, F., Perga, M.-E., Ojala, A., Schimmelmann, A., and Zolitschka, B.: Global spread of hypoxia in freshwater ecosystems during the last three centuries is caused by rising local human pressure, Glob. Change Biol., 22, 1481–1489, 2015.
Johnson, K. A. and Goody, R. S.: The original Michaelis constant: translation of the 1913 Michaelis-Menten paper, Biochemistry, 50, 8264–8269, https://doi.org/10.1021/bi201284u, 2011.
Kamp, A., Stief, P., Knappe, J., and De Beer, D.: Response of the Ubiquitous Pelagic Diatom Thalassiosira weissflogii to Darkness and Anoxia, PLoS One, 8, 1–11, https://doi.org/10.1371/journal.pone.0082605, 2013.
Kapsalis, V. C. and Kalavrouziotis, I. K.: Eutrophication – A Worldwide Water Quality Issue, in: Chemical Lake Restoration, edited by: Zamparas, M. G. and Kyriakopoulos, G. L., Springer Cham, https://doi.org/10.1007/978-3-030-76380-0_1, 2021.
Kataoka, T., Ohbayashi, K., Kobayashi, Y., Takasu, H., Nakano, S. I., Kondo, R., and Hodoki, Y.: Distribution of the Harmful Bloom-Forming Cyanobacterium, Microcystis aeruginosa, in 88 Freshwater Environments across Japan, Microbes. Environ., 35, ME19110, https://doi.org/10.1264/jsme2.ME19110, 2020.
Kim, D., Kang, Y. S., Lee, Y., Yamaguchi, K., Matsuoka, K., Lee, K.-W., Choi, K.-S., and Oda, T.: Detection of nitric oxide (NO) in marine phytoplankters, J. Biosci. Bioeng., 105, 414–417, https://doi.org/10.1263/jbb.105.414, 2008.
Kumar, A., Castellano, I., Patti, F. P., Palumbo, A., and Buia, M. C.: Nitric oxide in marine photosynthetic organisms, Nitric Oxide, 47, 34–39, https://doi.org/10.1016/j.niox.2015.03.001, 2015.
Maure, E. R., Terauchi, G., Ishizaka, J., Clinton, N., and DeWitt, M.: Globally consistent assessment of coastal eutrophication, Nat. Commun., 12, 6142, https://doi.org/10.1038/s41467-021-26391-9, 2021.
Mortonson, J. A. and Brooks, A. S.: Occurrence of a Deep Nitrite Maximum in Lake Michigan, Can. J. Fish. Aquat., 37, 1025–1027, https://doi.org/10.1139/f80-130, 1980.
Ohashi, Y., Shi, W., Takatani, N., Aichi, M., Maeda, S., Watanabe, S., Yoshikawa, H., and Omata, T.: Regulation of nitrate assimilation in cyanobacteria, J. Exp. Bot., 62, 1411–1424, https://doi.org/10.1093/jxb/erq427, 2011.
Paerl, H. W., Otten, T. G., and Kudela, R.: Mitigating the Expansion of Harmful Algal Blooms Across the Freshwater-to-Marine Continuum, Environ. Sci. Technol., 52, 5519–5529, https://doi.org/10.1021/acs.est.7b05950, 2018.
Plouviez, M. and Guieysse, B.: Nitrous oxide emissions during microalgae-based wastewater treatment: current state of the art and implication for greenhouse gases budgeting, Water Sci. Technol., 82, 1025–1030, https://doi.org/10.2166/wst.2020.304, 2020.
Plouviez, M., Shilton, A., Packer, M. A., and Guieysse, B.: N2O emissions during microalgae outdoor cultivation in 50 L column photobioreactors, Algal. Res., 26, 348–353, https://doi.org/10.1016/j.algal.2017.08.008, 2017a.
Plouviez, M., Wheeler, D., Shilton, A., Packer, M. A., McLenachan, P. A., Sanz-Luque, E., Ocana-Calahorro, F., Fernandez, E., and Guieysse, B.: The biosynthesis of nitrous oxide in the green alga Chlamydomonas reinhardtii, Plant J., 91, 45–56, https://doi.org/10.1111/tpj.13544, 2017b.
Plouviez, M., Shilton, A., Packer, M. A., and Guieysse, B.: Nitrous oxide emissions from microalgae: potential pathways and significance, J. Appl. Phycol., 31, 1–8, https://doi.org/10.1007/s10811-018-1531-1, 2019a.
Plouviez, M., Chambonnière, P., Shilton, A., Packer, M. A., and Guieysse, B.: Nitrous oxide (N2O) emissions during real domestic wastewater treatment in an outdoor pilot-scale high rate algae pond, Algal. Res., 44, e101670, https://doi.org/10.1016/j.algal.2019.101670, 2019b.
Plouviez, M., Fernandez, E., Grossman, A. R., Sanz-Luque, E., Sells, M., Wheeler, D., and Guieysse, B.: Responses of Chlamydomonas reinhardtii during the transition from P-deficient to P-sufficient growth (the P-overplus response): The roles of the vacuolar transport chaperones and polyphosphate synthesis, J. Phycol., 57, 988–1003, https://doi.org/10.1111/jpy.13145, 2021.
Qian, H., Li, J., Pan, X., Sun, Z., Ye, C., Jin, G., and Fu, Z.: Effects of streptomycin on growth of algae Chlorella vulgaris and Microcystis aeruginosa, Environ. Toxicol., 27, 229–237, https://doi.org/10.1002/tox.20636, 2010.
Rabalais, N. N. and Turner, R. E.: Gulf of Mexico Hypoxia: Past, Present, and Future, L&O Bull., 28, 117–124, https://doi.org/10.1002/lob.10351, 2019.
Schaefer, S. C. and Hollibaugh, J. T.: Temperature Decouples Ammonium and Nitrite Oxidation in Coastal Waters, Environ. Sci. Technol., 51, 3157–3164, https://doi.org/10.1021/acs.est.6b03483, 2017.
Song, H., Lavoie, M., Fan, X., Tan, H., Liu, G., Xu, P., Fu, Z., Paerl, H. W., and Qian, H.: Allelopathic interactions of linoleic acid and nitric oxide increase the competitive ability of Microcystis aeruginosa, ISME J., 11, 1865–1876, https://doi.org/10.1038/ismej.2017.45, 2017.
Tang, X., Chen, J., Wang, W. H., Liu, T. W., Zhang, J., Gao, Y. H., Pei, Z. M., and Zheng, H. L.: The changes of nitric oxide production during the growth of Microcystis aerugrinosa, Environ. Pollut., 159, 3784–3792, https://doi.org/10.1016/j.envpol.2011.06.042, 2011.
Tian, H., Xu, R., Canadell, J. G., Thompson, R. L., Winiwarter, W., Suntharalingam, P., Davidson, E. A., Ciais, P., Jackson, R. B., Janssens-Maenhout, G., Prather, M. J., Regnier, P., Pan, N., Pan, S., Peters, G. P., Shi, H., Tubiello, F. N., Zaehle, S., Zhou, F., Arneth, A., Battaglia, G., Berthet, S., Bopp, L., Bouwman, A. F., Buitenhuis, E. T., Chang, J., Chipperfield, M. P., Dangal, S. R. S., Dlugokencky, E., Elkins, J. W., Eyre, B. D., Fu, B., Hall, B., Ito, A., Joos, F., Krummel, P. B., Landolfi, A., Laruelle, G. G., Lauerwald, R., Li, W., Lienert, S., Maavara, T., MacLeod, M., Millet, D. B., Olin, S., Patra, P. K., Prinn, R. G., Raymond, P. A., Ruiz, D. J., van der Werf, G. R., Vuichard, N., Wang, J., Weiss, R. F., Wells, K. C., Wilson, C., Yang, J., and Yao, Y.: A comprehensive quantification of global nitrous oxide sources and sinks, Nature, 586, 248–256, https://doi.org/10.1038/s41586-020-2780-0, 2020.
van Lis, R., Brugiere, S., Baffert, C., Coute, Y., Nitschke, W., and Atteia, A.: Hybrid cluster proteins in a photosynthetic microalga, FEBS J., 287, 721–735, https://doi.org/10.1111/febs.15025, 2020.
Weathers, P. J.: N2O evolution by green algae, Appl. Environ. Microbiol., 48, 1251–1253, 1984.
Weathers, P. J. and Niedzielski, J. J.: Nitrous oxide production by cyanobacteria, Archiv. Microbiol., 146, 204–206, 1986.
Xiao, M., Li, M., and Reynolds, C. S.: Colony formation in the cyanobacterium Microcystis, Biol. Rev. Camb. Philos. Soc., 93, 1399–1420, https://doi.org/10.1111/brv.12401, 2018.
Zhang, Y., Wang, J. H., Zhang, J. T., Chi, Z. Y., Kong, F. T., and Zhang, Q.: The long overlooked microalgal nitrous oxide emission: Characteristics, mechanisms, and influencing factors in microalgae-based wastewater treatment scenarios, Sci. Total Environ., 856, 159153, https://doi.org/10.1016/j.scitotenv.2022.159153, 2022.
Zhou, Y., Li, X., Xia, Q., and Dai, R.: Transcriptomic survey on the microcystins production and growth of Microcystis aeruginosa under nitrogen starvation, Sci. Total Environ., 700, 134501, https://doi.org/10.1016/j.scitotenv.2019.134501, 2020.