the Creative Commons Attribution 4.0 License.
the Creative Commons Attribution 4.0 License.
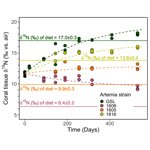
Quantifying the δ15N trophic offset in a cold-water scleractinian coral (CWC): implications for the CWC diet and coral δ15N as a marine N cycle proxy
Josie L. Mottram
Maria G. Prokopenko
Austin Cordova
Veronica Rollinson
Katie Dobkowski
Julie Granger
The nitrogen (N) isotope composition (δ15N) of cold-water corals is a promising proxy for reconstructing past ocean N cycling, as a strong correlation was found between the δ15N of the organic nitrogen preserved in coral skeletons and the δ15N of particulate organic matter exported from the surface ocean. However, a large offset of 8 ‰–9 ‰ between the δ15N recorded by the coral and that of exported particulate organic matter remains unexplained. The 8 ‰–9 ‰ offset may signal a higher trophic level of coral dietary sources, an unusually large trophic isotope effect or a biosynthetic δ15N offset between the coral's soft tissue and skeletal organic matter, or some combinations of these factors. To understand the origin of the offset and further validate the proxy, we investigated the trophic ecology of the asymbiotic scleractinian cold-water coral Balanophyllia elegans, both in a laboratory setting and in its natural habitat. A long-term incubation experiment of B. elegans fed on an isotopically controlled diet yielded a canonical trophic isotope effect of 3.0 ± 0.1 ‰ between coral soft tissue and the Artemia prey. The trophic isotope effect was not detectably influenced by sustained food limitation. A long N turnover of coral soft tissue, expressed as an e-folding time, of 291 ± 15 d in the well-fed incubations indicates that coral skeleton δ15N is not likely to track subannual (e.g., seasonal) variability in diet δ15N. Specimens of B. elegans from the subtidal zone near San Juan Channel (WA, USA) revealed a modest difference of 1.2 ± 0.6 ‰ between soft tissue and skeletal δ15N. The δ15N of the coral soft tissue was 12.0 ± 0.6 ‰, which was ∼6 ‰ higher than that of suspended organic material that was comprised dominantly of phytoplankton – suggesting that phytoplankton is not the primary component of B. elegans' diet. An analysis of size-fractionated net tow material suggests that B. elegans fed predominantly on a size class of zooplankton ≥500 µm, implicating a two-level trophic transfer between phytoplankton material and coral tissue. These results point to a feeding strategy that may result in an influence of the regional food web structure on the cold-water coral δ15N. This factor should be taken into consideration when applying the proxy to paleo-oceanographic studies of ocean N cycling.
- Article
(3144 KB) - Full-text XML
-
Supplement
(1374 KB) - BibTeX
- EndNote
Interactions between ocean circulation and nutrient cycling modulate the marine biological carbon pump, the consequent partitioning of CO2 between atmosphere and ocean, and thus influence planetary climate on centennial to millennial timescales (Sigman and Boyle, 2000). The marine nitrogen (N) cycle is highly sensitive to these interactions, such that knowledge of modern and ancient ocean N cycling can help illuminate drivers of past climate and contextualize modern global change (e.g., Altabet et al., 1994; Francois et al., 1997; Robinson and Sigman 2008; Sigman et al., 1999; Kast et al., 2019).
The main tool to investigate the oceanic N cycle history is the nitrogen (N) isotope composition (i.e., the 15N 14N ratio) of particulate organic nitrogen (PON) exported from the euphotic zone and preserved in various paleo-archives, including bulk sedimentary N in anoxic sediments (reviewed by Robinson et al., 2023). Hereafter, we express the 15N 14N ratio using delta notation (δ15N). The δ15N-PON recorded in paleo-oceanographic archives reflects both regional N cycling processes and the balance of global ocean N source and sink terms (Sigman and Fripiat, 2019; Brandes and Devol, 2002). In regions of the ocean where nitrate is quantitatively consumed, the annually integrated δ15N-PON exported from the surface reflects the isotopic composition of thermocline nitrate (Altabet et al., 1991). The latter is influenced by the circulation history of nitrate (e.g., Marconi et al., 2015), by regional N2 fixation (e.g., Casciotti et al., 2008; Knapp et al., 2008) and by water column denitrification (e.g., Pride et al., 1999; De Pol-Holz et al., 2007). In regions with incomplete consumption of surface nitrate, such as the Southern Ocean, the isotopic discrimination imparted during nitrate assimilation is reflected in the δ15N-PON, which can be used to reconstruct the degree of surface nitrate consumption in the past (e.g., Sigman et al., 1999; Francois et al., 1997).
Accurate interpretation of the N cycle's paleo-history relies on the presumption that the δ15N-PON preserved in various paleo-oceanographic archives is impervious to organic matter diagenesis. Thus, bulk sedimentary δ15N measurements are generally inadequate in this respect, subject to post-depositional processes (Robinson et al., 2012) – barring fast-accumulating organic-rich anoxic sediments with a negligible contribution from terrestrial sources (e.g., Altabet et al., 2002; Ganeshram and Pedersen, 1998). To circumvent this limitation, several “biological” archives of δ15N-PON have been developed that are deemed resistant to diagenetic alteration. These include the organic matter in diatom frustules and foraminifera tests (e.g., Ren et al., 2009; Robinson and Sigman, 2008) and the organic matter in proteinaceous corals (e.g., Sherwood et al., 2009; Williams and Grottoli, 2010). Recently, the δ15N of organic N enclosed within the aragonite mineral lattice of asymbiotic scleractinian (stony) cold-water corals (CWCs) has been found to reflect the δ15N-PON exported from the surface ocean (Wang et al., 2014), offering an exciting new archive of marine N cycling (Wang et al., 2017; Li et al., 2020; Studer et al., 2018; Chen et al., 2023). A robust cold-water coral archive of δ15N-PON can complement the existing suite of nitrogen proxies by reducing the potential biases inevitable in almost any individual proxy, allowing for a broader geographic and temporal reconstruction, and increasing the resolution of the proxy record. Foremost, as with foraminifera and diatom shells, organic material trapped within the coral's original aragonite mineral lattice is largely protected from diagenetic alteration (Drake et al., 2021), and compromised areas can be avoided by inspecting the skeletons for contamination and recrystallization (e.g., borings) using microscopic techniques (Gothmann et al., 2015). CWCs have a broad geographic distribution, being present in all ocean basins from the surface to 5000 m (Freiwald, 2002). CWCs also offer the potential to generate high-resolution records extending relatively far back in time; moreover, corals have continuous skeletal accretion that records ocean conditions at the time of growth, so the analysis of multiple individuals provides enhanced temporal resolution of long-term records (Robinson et al., 2014; Hines et al., 2015). Unlike sediments containing microfossils (e.g., diatoms and foraminifera), CWC skeletons are not subject to bioturbation, and absolute ages of this paleo-archive can be determined with decadal precision on the timescales of glacial–interglacial climate variability through U–Th series dating (Cheng et al., 2000; Goodfriend et al., 1992; Robinson et al., 2014; Li et al., 2020). Remarkably, individual coral samples can archive multiple seawater properties, such that a single CWC specimen can potentially be used to reconstruct deep (e.g., Δ14C, pH, temperature, and circulation proxies such as Ba Ca and εNd) and surface (δ15N) ocean conditions at a precisely known time (U–Th dating), making CWC unique as a paleo-oceanographic archive (Robinson et al., 2014; Thiagarajan et al., 2014; Rae et al., 2018).
However, an outstanding concern about the fidelity of the δ15N of coral-bound organic N is a reported 8 ‰–9 ‰ offset between coral-bound δ15N and the corresponding δ15N-PON exported to regions of coral growth (Wang et al., 2014). The magnitude of this offset substantially exceeds the 3 ‰–3.5 ‰ expected for a single trophic transfer (Minagawa and Wada, 1984), assuming that CWC feed predominantly on algal material exported from the surface ocean. Wang et al. (2014) explained the magnitude of the offset by arguing that CWCs feed on the more abundant pool of surface-derived suspended particulate organic material (SPOM), as the δ15N of SPOM at depth is typically ∼4 ‰–5 ‰ higher than that of sinking PON (Altabet, 1988; Saino and Hattori, 1987). While CWCs are considered generalists with regard to diet (e.g., Mortensen, 2001; Freiwald, 2002; Carlier et al., 2009; Maier et al., 2023), a number of studies suggest that many species of CWC subsist predominantly on metazoan zooplankton prey (e.g., Naumann et al., 2011; Kiriakoulakis et al., 2005; Purser et al., 2010; Tsounis et al., 2010). A zooplankton diet should result in an approximate two-level or more trophic transfer between surface PON and coral tissue (e.g., Sherwood et al., 2008), closer to the observed 8 ‰–9 ‰ offset, potentially rendering coral-bound δ15N sensitive to spatial and temporal differences in the trophic-level food web structure. An alternative explanation for the offset is that there is a large biosynthetic offset between the δ15N of the CWC polyp and its skeletal tissue (Horn et al., 2011; Muscatine et al., 2005), assuming that CWCs' diet derives directly from sinking algal material from the surface ocean. Otherwise, there could be an atypically large N isotope fractionation associated with the trophic-level transfer between the coral diet and its tissue (>3 ‰–3.5 ‰), possibly borne out of intermittent starvation periods (Doi et al., 2017), which is then passed on to the organic matrix within the coral skeleton. The gap in our understanding of how corals record the δ15N-PON exported from the surface ocean raises questions regarding the consistency of the offset in space and time as well as whether it is likely to differ among CWC species or due to intraspecific variations in diet.
Due to the challenges of accessing deep-ocean environments, the trophic ecology of CWCs is sparsely documented, although it is fundamental to understanding their role in cold-water reef ecosystems and to defining their utility as paleo-oceanographic archives of N cycling. The nature of the δ15N offset between CWC skeletal material and exported PON must be explained in order to further validate and potentially improve the use of δ15N of CWC skeletons as a proxy to reconstruct the history of exported PON and to further understand the role of CWCs in benthic ecosystems. To this end, we studied Balanophyllia elegans, an asymbiotic scleractinian cold-water coral found along the west coast of North America that grows as individual polyps (Fadlallah, 1983). We investigated the following questions:
- a.
Is there a large offset in δ15N between coral polyp tissue and coral skeletal tissue?
- b.
Is there an unusually large trophic-level offset between coral tissue and coral diet?
- c.
Does B. elegans feed predominantly on suspended particulate organic matter (SPOM) in situ?
- d.
Does B. elegans feed predominantly on metazoan zooplankton, resulting in a two-level trophic transfer between coral tissue and N of export?
To evaluate question (a), we measured the δ15N of tissue–skeleton pairs of coral samples collected in their natural habitat. To evaluate question (b), we cultured B. elegans corals in the laboratory in experiments in which both the isotopic composition of food and the frequency of feeding was controlled. To evaluate questions (c) and (d), we also investigated the δ15N of various components of the food web at a field site where B. elegans is abundant. Our observations offer novel insights into the growth and trophic ecology of B. elegans, providing unique new data on the N metabolism of CWCs and their feeding ecology. We contextualize our conclusions to inform the use of CWC archives as a paleo-proxy for marine N cycling and ocean biogeochemistry.
2.1 Collection of live-coral specimens
Individual specimens of the cold-water coral Balanophyllia elegans were collected during four sampling campaigns in March and June 2019 and in September and November 2020 from the San Juan Channel near the University of Washington's Friday Harbor Laboratories off the coast of Washington state in the Salish Sea (48.5° N, 123.0° W; Fig. 1). B. elegans is a solitary, asymbiotic cold-water cup coral native to the Pacific Northwest that can be found both in shallow, rocky environments and at depths as great as 500 m (Durham and Barnard, 1952). The genus Balanophyllia is cosmopolitan, and fossil samples as old as the Eocene in age have been used for paleo-environmental study (Muhs et al., 1994; Gothmann et al., 2015; Gagnon et al., 2021). B. elegans's presence at near-surface depths makes it an easy target for culture experiments, and Balanophyllia sp. can be found co-occurring with the similar but more widely applied cold-water coral archive Desmophyllum dianthus (Margolin et al., 2014). Therefore, we consider the asymbiotic Balanophyllia sp. to be generally representative of other deep cold-water coral species.
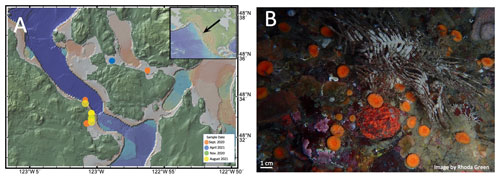
Figure 1(a) Map of the San Juan Islands indicating the collection site of B. elegans specimens and hydrographic measurements (created using http://www.geomapapp.org (last access: 13 December 2023); Ryan et al., 2009). The inset shows where the San Juan Islands are situated within North America. (b) Image of B. elegans from the San Juan Channel near Friday Harbor Laboratories taken by Rhoda Green.
B. elegans specimens were collected at 10–20 m depth by divers who gently removed the corals from vertical rock walls using blunt-tipped diving knives. Of the live corals collected, a subset was immediately frozen at −18 °C for N isotope ratio analyses of soft tissue and organic matter bound in the coral skeleton matrix. Live specimens were shipped overnight in small bags of seawater on ice to St. Olaf College (Minnesota, USA). Corals were cleaned, by gently scraping the exposed skeleton with dental tools to remove encrusting organisms, and placed in incubation bottles with artificial seawater for recovery prior to feeding experiments (described below).
2.2 Live-coral maintenance
Live B. elegans corals were maintained in artificial seawater medium prepared from nitrate-free Instant Ocean® sea salt. Salts were dissolved in deionized water to a salinity of 28.0 ± 0.25 – akin to the conditions at the collection site (Murray et al., 2015) – and sparged with air to achieve atmospheric equilibrium. The pH of the seawater was measured with a YSI brand 4130 pH probe and adjusted using dilute (0.1 N) hydrochloric acid or sodium hydroxide to 8.14 ± 0.05, slightly higher than in situ conditions, to promote skeletal growth. Batch seawater was then allotted to 2 L airtight polypropylene bottles to incubate single coral polyps. Bottles were pre-cleaned with fragrance-free soap and multiple rinses of deionized water. The salinity, pH, and temperature in the incubation bottles were monitored using YSI brand probes (4310-3 conductivity and temperature sensor and 4130 pH probe, respectively), and dissolved oxygen concentrations were measured using an optical sensor (FDO 4410; Fig. S1); a MultiLab 4010–3W was used as the digital meter for the sensors. The bottles containing individual corals were randomly distributed among three recirculating water baths maintained at a constant temperature of 12.5 ± 0.2 °C, akin to the conditions at the collection site (Murray et al., 2015). Small but quasi-systematic differences of ±0.3 °C were observed among the three recirculating tanks (Fig. S2). Corals were sustained on a diet of Artemia salina nauplii (described below), fed twice a week to ensure maximum growth (Crook et al., 2013). Seawater in the incubation bottles was replaced twice a week after the corals were fed, based on observations indicating that seawater pH in the bottles decreased slightly but significantly by ∼0.03 pH units over 3 d due to coral respiration (statistical analysis was performed with RStudio; Welch two-sample t test; t(515.07)=12.8, p value < 0.01; Fig. S3). Dissolved oxygen concentrations remained near atmospheric equilibrium at concentration of 7.5 ± 0.3 mg L−1 (Fig. S1). Nitrate concentrations in the bottles were also monitored from samples taken during each water change, in the freshly prepared seawater and in spent seawater, revealing low variability of 0.7 ± 0.3 µmol L−1 in the NO concentration (Fig. S4). Nitrate concentrations in the incubations were notably lower than ambient levels at the collection site, where concentrations were ∼25 µmol L−1, ensuring that the coral's only source of nitrogen was the Artemia diet (Murray et al., 2015).
2.3 Coral culture experiments
2.3.1 Experiment to quantify the trophic isotope effect
The corals were acclimated to precise incubation conditions for approximately 20 h before initiating feeding experiments. To assess the δ15N of coral soft tissue compared to that of its food source, four experimental groups of individual B. elegans corals were fed respective diets of Artemia salina nauplii with different δ15N values, twice per week for 530 d (Spero et al., 1993). Unhatched Artemia salina sourced from specific geographic locations have widely different δ15N values, owing to the different N isotope dynamics of the environments from which they were collected, which makes these organisms useful for trophic studies (Spero et al., 1993). Eighteen coral specimens were fed Artemia nauplii hatched from cysts from the Great Salt Lake (reference code: GSL) with a δ15N of 17.0 ± 0.3 ‰. Twelve corals were fed hatched nauplii from Lake Ulzhay in Russia (reference code: 1816) with a δ15N of 13.8 ± 0.4 ‰. Twelve corals were fed hatched nauplii from Vinh Chau in Vietnam (reference code: 1805) with a δ15N of 9.9 ± 0.3 ‰. Twelve corals were fed hatched nauplii from Tibet (reference code: 1808) with δ15N of 6.3 ± 0.2 ‰. The GSL Artemia group was procured from Aquatic Foods California Blackworm Co. (Great Salt Lake), whereas all other Artemia groups were obtained from the Artemia Reference Center (Ghent, Belgium). The δ15N of the diet for each treatment was calculated as the mean value measured from each group of unhatched cysts and hatched nauplii (Tables S2 and S3).
Fresh batches of nauplii were hatched from Artemia cysts at approximately monthly intervals, filtered into a concentrated suspension, stored frozen at −18 °C, and thawed immediately before feeding to the corals. Due to low hatch rates of the Artemia group 1808, corals in that treatment group were fed nauplii harvested from decapsulated Artemia cysts from day 151 (19 November 2019) to 245 (22 February 2020). The δ15N of the hatched nauplii ranged from 6.3 ± 0.2 to 17.0 ± 0.3 ‰ (measured by elemental analyzer–isotope ratio mass spectrometry; Table S2). The δ15N of the nauplii did not change significantly over prolonged storage of several months in the freezer (ANOVA test, F(1)=0.07, p value = 0.80; Fig. S5). Artemia nauplii had statistically indistinguishable molar C:N ratios among regional groups, averaging 6.0 ± 0.6 (ANOVA test, F(3)=0.31, p value = 0.82; Table S3). These results show that there was limited variability in the diet of corals due to freezer storage and hatching of multiple individual batches of Artemia (Tables S2, S3; Fig. S5).
Corals were fed their respective nauplii diets by transferring coral individuals from their incubation bottle to a small dish filled with artificial seawater with minimal exposure to air so as not to stress the corals. Each coral was fed 20 µL of thawed nauplii suspension by pipetting the food directly into their oral cavity, making it possible to visually ensure complete consumption and, thus, minimize variability in feeding rates. Each coral was returned to its bottle with a fresh allotment of seawater when its mouth had remained closed for several minutes, signifying that it was finished eating (Fig. 2).
After a shift in the δ15N of the diet, it is expected that coral tissue δ15N will evolve as a function of time until the composition of tissue reaches an equilibrium in line with the new diet. In order to assess the rate (referred to here as the isotopic turnover time) at which this evolution occurs, individual corals were sacrificed at discrete intervals throughout the experiment. Corals were always sacrificed 3 d after feeding to ensure that no food remained in the oral cavity. The corals were removed from their bottles and rinsed with artificial seawater. The coral tissue was then separated from the skeleton using a fine stream of compressed air. The tissue and skeleton were frozen at −18 °C and stored separately until processed for isotope ratio analyses.

Figure 2Photo illustration of a coral feeding sequence. Photo 1 shows coral before food is given. Photo 2 shows food being pipetted onto the coral mouth. Photos 3 through 6 show the coral feeding as the mouth opens to engulf food and closes when finished (about 15 min in total). Corals are ∼1 cm in diameter.
2.3.2 Experiment to evaluate the effects of starvation conditions
An additional 522 d feeding experiment was performed to assess the influence of starvation on the δ15N of the coral soft tissue. Live corals collected during a sampling campaign at the end of November 2020 and shipped live to St. Olaf College were randomly assigned to two treatment groups (starved and not starved). Corals in the starved treatment were fed at 25 % of our normal feeding frequency, or every 2 weeks, whereas those in the not-starved treatment were fed twice a week. These feeding regimes were chosen based on the work of Crook et al. (2013) and Beauchamp et al. (1989), who assumed feeding every 3 d to represent plentiful food supply and feeding every 21 d (close to our starvation condition) to represent minimal maintenance food supply. Both groups were fed Artemia nauplii with a δ15N of 9.9 ± 0.3 ‰, approximately 3 ‰ lower than the coral tissue of average B. elegans collected from Friday Harbor, and thus presumably closest in δ15N to what the corals are eating in the wild given a canonical trophic isotope effect. Coral incubations and feedings were conducted as described above. Individuals were sacrificed over the course of the 522 d experiment, and tissue samples were frozen at −18 °C until isotope analysis.
2.4 Coral preparation for isotope ratio analyses
Frozen coral tissue samples (and hatched nauplii) were freeze-dried using a Labconco FreeZone 4.5 and then powdered using a mortar and pestle. The samples were sent to the University of Connecticut, Avery Point (Groton, CT, USA) for isotope ratio analyses.
Coral skeletons from specimens collected at Friday Harbor were separated from the coral soft tissue and were rinsed and individually ultrasonicated two times in Milli-Q™ (MQ) water for 20 min each in order to remove any residual seawater. Samples were then individually ultrasonicated in a 1 % sodium hypochlorite (bleach) solution for at least two 20 min intervals with fresh bleach for each new ultrasonication interval until no tissue remained on the skeleton, as assessed visually under a dissection microscope. Following this, individual skeletons were rinsed and ultrasonicated for 20 min in MQ another three times (each time with a new batch of MQ water) in order to remove any bleach residue. Skeleton samples were sent to Pomona College (California, USA) for further processing.
It is necessary to isolate organic matter from the coral carbonate matrix in advance of the N isotope measurement methods used here (see Sect. 2.6 below). Organic material in the skeleton matrix was isolated and oxidized to nitrate following the protocol of Wang et al. (2014). Briefly, bulk samples weighing 50–100 mg were ground into coarse powder, and a fraction between 63 and 200 µm was collected by sieving through two metal sieves. The 10–15 mg of sieved powder was rinsed sequentially with sodium polyphosphate and sodiumbicarbonate-buffered dithionite–citrate reagent and then treated with 13.5 % sodium hypochlorite overnight on a shaker. Skeletal material was dissolved in 4 N ultrapure hydrochloric acid and then oxidized to nitrate by autoclaving in basic potassium persulfate solution. Standards of glutamine reference material USGS-40 and USGS-41 (δ15N of −4.52 ‰ vs. air and 47.57 ‰ vs. air, respectively) were oxidized in tandem and used to correct for processing blanks. The resulting nitrate samples were sent to the University of Connecticut for nitrate isotope ratio analysis. The long-term averaged reagent blank was 0.4–0.6 µmol L−1, while the typical samples were 10–15 µmol L−1 (typical amount of nitrogen in skeleton being 2–5 µmol g−1 of aragonite). Samples were typically run in duplicate with an average reproducibility of 0.5 ‰. An internal laboratory standard of ground material of the cold-water colonial scleractinian coral Lophelia pertusa had a long-term δ15N value of 9.4 ± 0.8 ‰ (n=57).
2.5 Hydrographic data
To infer the natural food source of the B. elegans, we collected samples for analysis of the δ15N of the particulate and dissolved N pools in relation to ambient hydrographic variables (temperature and salinity) near Friday Harbor, WA. Seasonal sampling campaigns were conducted in September and November 2020 and in April, June, and August 2021 (Table S1). In all but the August 2021 campaign, particulate and dissolved N samples were collected by divers at unspecified depths between the surface and the depth of coral collection. Samples were stored frozen in 30 mL high-density polyethylene (HDPE) bottles. Surface net tows were performed with a mesh size of 120 µm; materials were stored and shipped frozen and thawed at a later time to be filtered onto pre-combusted GF/F filters (0.7 µm nominal pore size) that were stored frozen pending isotope analysis. No hydrographic variables were recorded during the campaigns except in August 2021.
During the August 2021 campaign, depth profiles of temperature and salinity from the surface to 35 m were characterized with a CastAway® -CTD (conductivity–temperature–depth) profiler. Water samples were collected at 5 m intervals between 5 and 30 m using a Van Dorn water sampler. Water was filtered onto pre-combusted glass-fiber filters (GF/F; 0.7 µm nominal pore size) into pre-cleaned 30 mL HDPE bottles and stored frozen pending analyses of nitrate concentrations and nitrate isotope ratios. The corresponding filters were stored frozen for isotope ratio analysis of suspended particulate organic material (SPOM). Surface (5 m) and deeper (25 m to the surface) net tows were conducted using plankton nets with respective mesh sizes of 150 and 80 µm. Net tow material was filtered directly onto pre-combusted GF/F filters and frozen pending analysis. A portion of the net tow material from the August 2021 campaign was sieved to separate size classes of 80–100 µm, 100–250, ≥250, 250–500, and ≥500 µm. Material from the respective size classes was filtered onto pre-combusted GF/F filters and frozen until isotope analysis.
2.6 Nitrate concentrations and isotope ratio analyses
Nitrate concentrations of oxidized coral skeletons and aqueous samples were measured by reduction to nitric oxide in hot vanadium(III) solution followed by chemiluminescence detection of nitric oxide (Braman and Hendrix, 1989) on a Teledyne chemiluminescence NOx analyzer Model T200 (Thousand Oaks, CA).
The δ15N and δ13C of lyophilized coral tissue samples were analyzed at the University of Connecticut on a Costech elemental analyzer–isotope ratio mass spectrometer (DELTA V) and are expressed in standard delta notation (e.g., for N, δ15N (‰ vs. air) = [[(15N 14Nsample) (15N 14N). Approximately 0.75 mg of lyophilized sample (35 µg N) was allotted into tin cups and analyzed in tandem with recognized glutamine reference materials USGS-40 and USGS-41 with respective δ15N (vs. air) of −4.52 ‰ and 47.57 ‰ and δ13C of −26.39 ‰ and 37.63 ‰ (vs. Pee Dee Belemnite). Replicate analyses of (n≥2) reference materials yielded an analytical precision (±1 SD) of 0.3 ‰ for both δ15N and δ13C.
Nitrate N (and O) isotope ratios of aqueous seawater samples and N isotope ratios of the skeleton matrix samples were analyzed at the University of Connecticut using the denitrifier method (Casciotti et al., 2002; McIlvin and Casciotti, 2011; Sigman et al., 2001). Nitrate sample solutions were injected at target concentrations of 20 nmol for seawater samples and 7 nmol for skeleton matrix samples. N2O was extracted, concentrated, and purified using a custom-modified Thermo GasBench II equipped with a GC PAL autosampler and dual cold traps and was then analyzed on a Thermo DELTA V Advantage continuous-flow isotope ratio mass spectrometer (Casciotti et al., 2002; McIlvin and Casciotti, 2011). Individual analyses were referenced to injections of N2O from a pure gas cylinder and standardized through comparison with potassium nitrate reference material – International Atomic Energy Agency nitrate (IAEA-N3) – and isotopic nitrate reference material – United States Geological Survey 34 (USGS-34) – with respective δ15N of 4.7 ‰ and −1.8 ‰ (vs. air; International Atomic Energy Agency, 1995) and respective δ18O of 25.61 ‰ and −27.9 ‰ (vs. Vienna Standard Mean Ocean Water, VSMOW; Gonfiantini, 1995; Böhlke et al., 2003). To account for bacterial blanks and source linearity, nitrate concentrations of the standard material – diluted in N-free seawater for aqueous seawater samples and air-equilibrated MQ water for skeleton matrix samples – were matched to those of samples within batch analyses, and additional bacterial blanks were also measured (Weigand et al., 2016; Zhou et al., 2022). Replicate measurements (n≥2) of all samples yielded an average analytical precision (±1 SD) of 0.3 ‰ for both δ15N and δ18O.
2.7 N turnover model
We estimate values of the trophic δ15N offset for B. elegans, ϵ, and the rate of isotopic turnover by fitting the data from our trophic isotope experiment to a nonlinear least-squares regression model corresponding to the isotope mixing relationship shown in Eq. (1) below. Equation (1) treats the coral tissue as a single reservoir of N with some initial isotope composition that is evolving to reflect the new diet as a function of time (after Cerling et al., 2007; Ayliffe et al., 2004):
The term δ15Nt=0 is the value of the bulk coral tissue at the onset of the experiment, δ15Ndiet is that of the corals' new Artemia diet (i.e., what it is fed during the experiment), t is the number of days since the start of the experiment, ϵ is the difference between the δ15N of the diet and tissue at equilibrium (i.e., once the isotopic composition of inputs to the system equals the isotope composition of outputs), and λ describes the specific rate at which new N is incorporated into the coral tissue (per day). We use this model to calculate the e-folding time of the system, which is defined as (days) and represents the time at which ∼63 % of the original N reservoir in coral tissue has been replaced with new N from the experimental coral diet.
3.1 Trophic isotope effect
At the onset of the culture experiment, the soft tissue among all experimental corals had a δ15N of 11.7 ± 0.5 ‰. Over the course of the experiment, the δ15N of the tissue increased or decreased in respective treatments depending on the δ15N of the associated Artemia diet (Fig. 3): the tissue δ15N increased in corals fed diets with δ15N values of 17.0 ‰, 13.8 ‰, and 9.9 ‰, whereas the tissue δ15N decreased for the diet of 6.4 ‰. The δ15N of soft tissue in all groups trended towards an asymptotic offset relative to the diet δ15N, as expected for an approach to a new equilibrium. However, at day 530, at the end of the experiment, it appeared as though the coral tissue δ15N had not yet reached a constant offset value, suggesting that the coral tissue had not yet reached an equilibrium with the new diet. Specifically, at the end of the experiment, the coral tissue of the treatment groups reached δ15N values of 9.4 ± 0.3 ‰, 12.6 ± 0.5 ‰, 15.9 ± 0.1 ‰, and 18.1 ± 0.1 ‰ for groups fed the lowest to highest Artemia δ15N values, respectively. The difference between coral soft tissue and diet δ15N ranged from a minimum of 1.0 ± 0.1 ‰ to a maximum of 3.0 ± 0.3 ‰ across the different experimental groups at day 530 (Fig. 3).
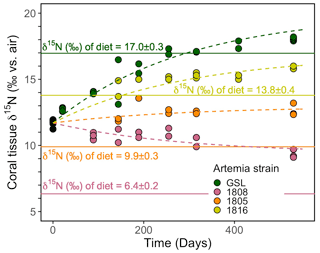
Figure 3Evolution of the coral soft tissue δ15N in response to diet δ15N. Colors correspond to the respective Artemia strains. Dashed lines are the model output of our simultaneous nonlinear least-squares regression fits to the data using Eq. (1). Solid lines mark the diet δ15N ± σ. The mean analytical error on tissue δ15N analyses was ±0.2 ‰.
Despite the fact that coral tissue had not yet reached an equilibrium with the new coral diet at the end of our experiment, we are able to estimate values of the trophic δ15N offset for B. elegans, ϵ, and the rate of isotopic turnover by fitting the data from our trophic isotope experiment to the nonlinear least-squares regression model given Eq. (1) in Sect. 2.7. To more confidently calculate ϵ and λ for each individual experimental group, we generate four equations (one for each experimental group of the form given in Eq. (1) but with different values of δ15Ndiet) and fit them simultaneously using least-squares regression. From this fit, we are able to obtain estimates for both ϵ and λ in B. elegans. An inherent assumption of this approach is that all experimental groups have the same e-folding time and the same trophic isotope effect. We note here that we refer to the e-folding time as the “turnover rate” of N in corals throughout the rest of this text (e.g., Tanaka et al., 2018). Our model fit yielded a trophic isotope effect, ϵ, of 3.0 ‰ with a standard error of 0.1 ‰ between coral tissue and diet. The turnover rate of N (i.e., e-folding time, was 291 d with a standard error of 15 d. The four individual model equations generated by our nonlinear least-squares regression are presented using dashed lines in Fig. 3.
3.2 Effect of starvation
At the onset of the starvation trial, the coral tissue had an average δ15N of 11.5 ± 0.1 ‰. At the end of the 522 d experiment, the starved group (N = 15 coral individuals) had an average δ15N of 12.4 ± 0.4 ‰, while the frequently fed group (N = 15) had a δ15N of 12.7 ± 0.1 ‰ (Fig. 4). The starved group was +2.5 ± 0.4 ‰ compared with the δ15N level of its diet; this was statistically indistinguishable from that of the frequently fed group, which was +2.8 ± 0.1 ‰ compared with the level of its diet (p value = 0.059, pairwise t test).
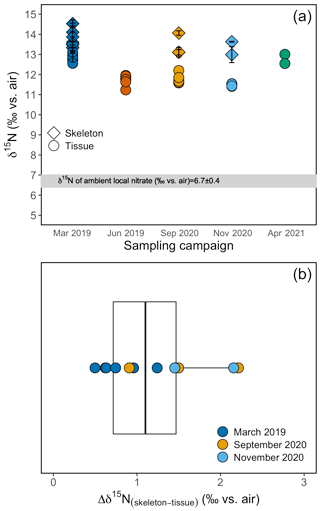
Figure 5(a) Tissue and skeleton δ15N measurements from B. elegans individuals collected during different sampling campaigns. Errors on skeleton data are based on replicate analyses of samples from individual polyps. (b) Box plot of the difference between tissue and skeleton of individual B. elegans corals. The box plot shows the mean, the first and third quartiles, the maxima, and the minima. Individual data points are overlaid on the plot. Colors correspond to respective sampling campaigns.
3.3 δ15N comparison of field specimen polyp tissue and skeleton
The δ15N of the soft tissue from individual B. elegans specimens collected live near Friday Harbor ranged between 11.2 ‰ and 13.1 ‰, averaging 12.0 ± 0.6 ‰ (Fig. 5a). The soft tissue δ15N differed among coral groups collected during different sampling campaigns, with higher values in spring (March 2019 and April 2021) compared with summer and fall (June 2019, September, and November 2020; ANOVA test, F(4)=40.39, p value ≤0.01; post hoc pairwise t test; p value < 0.05). The average δ15N of corresponding skeletal tissue was 13.5 ± 0.7 ‰ and did not differ discernibly among sampling campaigns (ANOVA test, F(2)=0.916, p value = 0.431). The average difference between skeleton and soft tissue δ15N (Δδ15N) among coral individuals for which both soft tissue and skeleton was measured was 1.2 ± 0.6 ‰ (Fig. 5b).
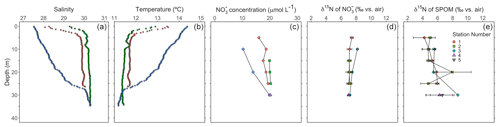
Figure 6Depth profiles during the August 2021 sampling campaign of (a) salinity, (b) temperature, (c) nitrate concentration, (d) the δ15N of nitrate for analytical replicates and (e) the δ15N of SPOM of replicate samples (n≥2). Green and red symbols correspond to flood tide (collected between 11:00 and 14:00 LT, local time, on 2 August 2021), whereas blue symbols correspond to ebb tide (collected at 09:00 LT on 3 August 2021).
3.4 Regional hydrography and N isotope ratios of nitrate and plankton material
Hydrographic profiles recorded at stations near Friday Harbor in August 2021 showed characteristic density structures that were sensitive to tidal phase (Fig. 6a, b; Banas et al., 1999). Profiles collected during flood tide (collected between 11:40 and 14:20 LT on 2 August 2021) were relatively well mixed (salinity 30, temperature 11.8 °C), with fresher and warmer water restricted to the near surface (≤5 m), whereas ebb-tide profiles (collected at 09:00 LT on 2 August 2023) showed a progressive decrease in salinity from 30 to 27 and a corresponding increase in temperature from 11.8 °C at 35 m to 14.5 °C at the surface.
Nitrate concentrations were nearly uniform with depth during flood tide (∼20 µmol L−1), decreasing slightly at 5 m, whereas nitrate concentrations decreased progressively during ebb tide from 20 to 10 µmol L−1 between 30 and 10 m (Fig. 6c). Nitrate concentrations in samples collected during the other sampling campaigns ranged from 12 to 32 µmol L−1 and appeared to be generally higher at stations visited during the September and November 2020 campaigns compared with those visited in April and August 2021 (Fig. S6).
Depth profiles collected in August 2021 revealed uniform nitrate δ15N values of ∼7 ‰ at 30 m among profiles. In well-mixed profiles, nitrate δ15N increased slightly to 7.5 ‰ above 10 m. In stratified profile, nitrate δ15N increased progressively to 8.2 ‰ at 10 m (Fig. 6d). Among all sampling campaigns, the δ15N of nitrate ranged from 6.1 ‰ to 8.2 ‰, with median values of 6.8 ± 0.4 ‰ (Fig. 7a). The relationship between nitrate δ15N and nitrate concentration in August 2021 was fit to a closed-system Rayleigh distillation model (Mariotti et al., 1981), suggesting a nitrate assimilation isotope effect of 1.5 ± 0.1 ‰ (Fig. 8).
The δ15N of SPOM collected at depths above 35 m near Friday Harbor during the different sampling campaigns ranged from 1.6 ‰ to 11.7 ‰, averaging 5.7 ± 1.7 ‰ (Fig. 7b). Values were lowest for the four samples collected in April (4.4 ± 0.4 ‰) and highest for the four samples collected in September and November (6.2 ± 2.6 ‰), although these trends may be an artifact of the low data density in April (n = 4) and in September and November (n=5) relative to August 2021 (n=29), at which time the observed range of δ15N subsumed that in the other two campaigns. Values did not differ coherently with depth in August 2021, although any potential depth structure was obscured by the large variability among sample replicates (Fig. 6e).
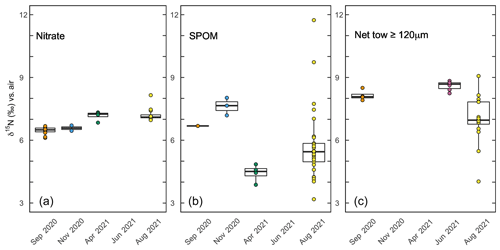
Figure 7Box plots of aqueous and particulate N pools at respective sampling times. (a) The δ15N of nitrate from samples above 30 m collected during respective sampling campaigns. (b) The δ15N of suspended particulate organic matter (SPOM) at sites near Friday Harbor during respective sampling campaigns. (c) The δ15N of net tows (≥120 µm mesh size) conducted during respective sampling campaigns.
The δ15N of material collected in net tows (120 µm mesh size) during sampling campaigns in September 2020 and June 2021 ranged between 7.9 ‰ and 8.8 ‰ (Fig. 7c). Material collected in net tows of 80 and 150 µm mesh size in August 2021 and separated by size class post-collection revealed a coherent δ15N increase with size class (Figs. 7c, 9). The ≥80 µm size class had a mean δ15N of 6.0 ± 0.3 ‰, whereas the ≥500 µm size class had an average δ15N of 8.0 ± 0.8 ‰, which was significantly greater than the δ15N of the other size classes (ANOVA test, p value < 0.05).
This study of B. elegans provides novel constraints on the trophic ecology of scleractinian CWCs. Foremost, our observations of B. elegans collectively suggest that the relatively large global δ15N offset of 8 ‰–9 ‰ between CWC skeletal tissue and the δ15N of PON exported from the surface ocean is neither explained by a large difference between tissue and skeleton δ15N nor by an unusually large trophic isotope effect. Further, controlled feeding experiments yielded direct estimates of the trophic isotope effect and the corresponding N turnover rate of B. elegans soft tissue. Examination of the soft tissue δ15N of wild specimens in relation to regional hydrography and food web components near Friday Harbor leads us to conclude that B. elegans feeds predominantly metazoan zooplankton prey, implicating more than one trophic transfer between exported PON and coral soft tissue. We contextualize our findings to existing studies of CWC trophic ecology and discuss the implications of considering a two-level trophic transfer for paleo-reconstructions of ocean N cycling using B. elegans and CWCs more generally.
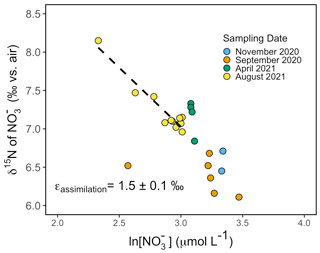
Figure 8Rayleigh plot of nitrate δ15N vs. the natural logarithm (ln) of nitrate concentration for samples collected from the surface to 40 m around Friday Harbor. The isotope effect of ∼1.5 ± 0.1 ‰ corresponds to the slope of the best-fit linear regression line for the August 2021 data, δ15N–1.5 ln [NO].
4.1 Culture experiments revealed a normal trophic isotope effect
We investigated whether the large difference in δ15N between PON export from the surface and coral-skeleton-bound δ15N (8 ‰–9 ‰) observed by Wang et al. (2014) could arise from an unusually large-trophic-level offset specific to CWCs. The long-term feeding experiment of B. elegans polyps revealed a “normal” trophic isotopic offset between coral tissue and diet of ± 0.1 ‰. This value conforms to the expected range of +3.4 ± 1.1 ‰ for a single trophic-level offset for δ15N (Minagawa and Wada, 1984).
To support the above conclusion, we assess the assumptions inherent to the isotope mixing model (Eq. 1) used to derive ε and the corresponding nitrogen turnover rate from our culture data. First, the model only accounts for the turnover of a single pool of N, requiring the assumption that all N in the coral polyp tissues equilibrates at the same rate. This notion is unlikely to be wholly accurate, as fluxes of N may vary among tissue types. However, given the relatively low resolution of our sampling over the course of the culture experiments (necessary due to constraints on numbers of total samples), we are unable to extend our model to one with multiple pools (e.g., as in Ayliffe et al., 2004). As soft tissues of individual coral polyps were homogenized, we suggest that the δ15N values and corresponding estimate of ε thus represent the average of soft tissues with potentially different turnover rates. The estimates of ε and the N turnover rate further rely on the assumption that the nutritional quality of the respective diets among treatments was equivalent, as trophic isotope effects can be sensitive to food type. Diets low in protein can be associated with greater ε values due to internal recycling of nitrogen (Adams and Sterner, 2000; Webb et al., 1998). For instance, locusts fed a low-protein diet were enriched 5.1 ‰ from their diet, compared with 2.3 ‰ for those fed a high-protein diet (Webb et al., 1998). Conversely, a compilation of studies of various metazoan consumers raised on controlled diets suggests that high-protein diets generally result in higher trophic isotope effects (∼3.3 ‰) compared with more herbivorous diets (∼2.2 ‰) – a dynamic ascribed to higher rates of N excretion to assimilation in consumers fed high-protein diets (McCutchan Jr et al., 2003). As noted in Table S3 and in Sect. 2.3.1, our Artemia prey had similar C:N ratios among treatments, in line with our model treatment. Finally, our model assumes that N turnover was dominated by metabolic tissue replacement, rather than net growth, consistent with the observation that adult B. elegans individuals display slow growth (Gerrodette, 1979).
Equation (1) could be invalidated if the corals can access nutritional N sources other than N in Artemia, given that the model assumes that Artemia individuals constitute the only source of N to corals in our experiment. Biological N2 fixation and chemoautotrophy have been detected in association with CWC holobionts, providing some N nutrition to the corals (Middelburg et al., 2016). Our trophic isotope effect estimate was in the range expected for a single trophic transfer, arguably suggesting that N2 fixation, if occurring, was not a substantial contribution to the corals' nutrition; it would otherwise result in a lower value of ε given a δ15N contribution of −1 ‰ to 0 ‰ (Carpenter et al., 1997). That the trophic isotope effect of the poorly fed corals did not differ from that of corals that were fed well also argues for no sources of N other than Artemia, as starved corals would presumably increase their reliance on said source. In a related vein, N recycling between the B. elegans specimens and potential microbial symbionts (e.g., Middelburg et al., 2016) could also dampen the trophic isotope effect relative to the Artemia prey and yield an overestimate of the soft tissue turnover rate for N. The normal trophic isotope effect indicated here suggests a modest role of N retention and recycling by microbial symbionts, in contrast to tropical symbiotic corals wherein bacterial symbionts promote substantial N retention and recycling, and, consequently, lower trophic isotope effects (Tanaka et al., 2018). Finally, the validity of our estimates could be sensitive to differences in feeding rates, which can influence the rate of N turnover of tissues (Martínez del Rio and Carleton, 2012; Rangel et al., 2019). Corals were fed at identical times among treatments, at a relatively high feeding rate (Crook et al., 2013). However, given the limited number of studies on feeding in B. elegans, it is difficult to compare our feeding strategy and that of this species' natural environment. Overall, we consider that the mixing model described by Eq. (1) is appropriate to derive the first-order trophic isotope effect and turnover rate of B. elegans.
Changes in metabolism due to underfeeding or prolonged fasting have the potential to increase trophic-level isotope offsets due to increased protein metabolism (Adams and Sterner, 2000). For instance, extensive amino acid recycling in overwintered adult insect larvae was cited to explain trophic isotope effects upward of 10 ‰ (Scrimgeour et al., 1995). A meta-analysis on the effects of starvation on consumer δ15N revealed that starvation generally led to increased organism δ15N by an average of 0.5 ‰, up to 4.3 ‰ (Doi et al., 2017). This dynamic was documented for the tropical symbiotic coral Stylophora pistillata, where heterotrophically starved corals were enriched in δ15N by ∼0.5 ‰ compared with frequently fed corals (Reynaud et al., 2009). The trophic isotope offset of B. elegans soft tissue relative to its diet, ε, was not discernibly influenced by near starvation; that of corals fed once every other week was similar to that of corals fed twice a week – in spite of visible signs of stress among the former, including relatively more sluggish feeding (Fig. S7) and thinner soft tissue (data not shown). Deep-sea coral reefs are often highly productive environments with high levels of biodiversity, commensurate with a relatively high food supply (Duineveld et al., 2007, 2004; Genin et al., 1986; Roberts et al., 2006; Soetaert et al., 2016; Thiem et al., 2006; Cathalot et al., 2015). Nevertheless, periodicity and spatial heterogeneity in the food supply of CWC reefs implicate periods of lower food density (e.g., Duineveld et al., 2007). High currents, downwelling, and/or vertically migrating zooplankton temporally boost the export of surface organic matter to the seabed, creating “feast” conditions, interspersed with “famine” periods during the nonproductive season (Maier et al., 2023). Regardless, our trials suggest that starvation, if pertinent to CWC communities, does not result in greater-than-expected trophic isotope offsets, at least for B. elegans.
4.2 Turnover rate for B. elegans
We report the first estimate of the nitrogen turnover for a nonsymbiotic cold-water coral: 291 ± 15 d for B. elegans soft tissue. This value falls within the range of existing estimates for tropical symbiotic corals. Pulse–chase experiments with 15N-nitrate conducted with fragments of the tropical symbiotic coral Porites cylindrica yielded an N turnover time of 370 d, whereas this value was 210 d for the tropical symbiotic coral Acropora pulchra (Tanaka et al., 2006, 2018). These relatively long turnover times are attributed to the recycling and retention of N within the coral–symbiont system in nutrient-deplete ecosystems. In comparison, the corresponding carbon turnover in A. pulchra was 18 d – compared with 210 d for N – because the system is ultimately N limited (Tanaka et al., 2006). Tanaka et al. (2018) inferred that the N turnover in P. cylindrica would be substantially faster than 370 d without symbionts, on the order of 56 d based on estimates of polyp-specific N uptake rates. Nevertheless, the N turnover estimated for the tropical symbiotic coral Porites lutea was notably shorter than that of A. pulchra and P. cylindrica, on the order of 87 d (Rangel et al., 2019), implicating different N nutritional strategies among symbiotic coral groups and/or ecosystems. The N turnover for B. elegans estimated here is of the same order as, although still longer than, that for tropical symbiotic corals, suggesting that cold-water species have lower metabolic and growth rates compared with tropical symbiotic species, although efficient N recycling has also been documented previously in CWCs (Middelburg et al., 2016). The slower turnover of CWCs relative to their symbiotic tropical counterparts may reflect the lower temperatures of the former's habitats (Miller, 1995; Thomas and Crowther, 2015).
Constraints on N turnover also allow for calibration of the temporal resolution that is achievable with the CWCs' δ15N proxy for marine N cycling. Corals are constantly accreting skeleton, such that coral proxies have the potential to provide annual-resolution information (e.g., Adkins et al., 2004). In theory, a rapid N turnover in CWCs could record seasonal changes in regional N dynamics. A turnover time of 291 ± 15 d for N in B. elegans soft tissue, however, signifies that the δ15N of coral skeleton is unlikely to provide a faithful record of seasonal differences in the δ15N of the coral diet. Moreover, the turnover of the pool of N that sources the skeletal tissue may be different from that of bulk tissue, and thus decoupled from the soft tissue turnover rate. We suggest that CWCs can likely record changes in their diet on annual or longer timescales, compatible with the ability to date CWCs with subdecadal resolution (Adkins et al., 2004).
4.3 Soft tissue vs. skeleton δ15N
A large biosynthetic δ15N offset between the coral soft tissue and its skeleton could conceivably account for a large δ15N offset between coral-skeleton-bound organic matter and N of export that is not explained by single trophic-level enrichment of ∼3 ‰. However, the mean difference between soft tissue and skeleton-bound δ15N among B. elegans specimens collected at Friday Harbor was relatively modest, on the order of +1.2 ‰, ranging between +0.5 ‰ and +2.2 ‰. The observed range was dictated primarily by the variability in the δ15N of the coral soft tissue, as skeleton-associated δ15N values were relatively invariant among specimens sampled from different locations and field seasons – likely due to the fact that the amount of skeleton analyzed represented multiple years of growth. The amount of skeleton-bound organic N is small relative to aragonite mass (2–5 µmol N g−1 of skeleton in our samples), such that homogenization of 50–100 mg aragonite fragments may alias seasonally driven variability in skeletal δ15N. Soft tissue values in spring were ∼1.5 ‰ higher than in summer and fall, such that they appeared to record seasonal changes in diet (Fig. 5a). In this regard, the asymptotic nature of the two-end-member isotope mixing model (Eq. 1) renders B. elegans's soft tissue sensitive to seasonal changes in prey δ15N but not likely to reach isotopic equilibrium on seasonal timescales – given an N turnover of ∼291 d, as discussed above. Seasonal variations in the δ15N of the food source of B. elegans near Friday Harbor could arise from corresponding differences in the δ15N of nitrate entrained to the surface driven by seasonal hydrographic variability around San Juan archipelago, in the extent of surface nitrate consumption, in food web structure, or from some combination of these. The data density among all but the August 2021 sampling campaign is too sparse to be conclusive in this regard. Otherwise, the observed differences in soft tissue δ15N may result from spatial heterogeneity in the δ15N of the food source among the different collection sites visited for respective campaigns at Friday Harbor.
As documented here for B. elegans, the δ15N difference between coral tissue and skeleton appears to be modest among various scleractinian coral species. Specimens of the symbiotic tropical coral Porites lutea showed a δ15N offset of +1.1 ‰ between skeleton and soft tissue, whereas the symbiotic tropical coral Favia stelligera revealed an insignificant offset of −0.1 ‰ (Erler et al., 2015). Similarly, no offset was observed for proteinaceous CWCs of the genus Lepidisis collected off Tasmania (Sherwood et al., 2009), whereas an offset of −1.9 ± 0.8 ‰ was reported for cold-water proteinaceous corals of the genus Primnoa from the Gulf of Alaska, Isadella from the central California margin, and Kulamanamana from the North Pacific Subtropical Gyre (McMahon et al., 2018). Conversely, a study of numerous species of both symbiotic and nonsymbiotic corals reported a +4 ‰ offset between the skeletal organic matrix and soft tissue among the nonsymbiotic corals specifically but no difference among the symbiotic corals (Muscatine et al., 2005), suggesting that biosynthetic offsets may occur for certain CWC species or conditions.
4.4 Implications for components of CWC diet
Cold-water corals are considered opportunistic feeders, ingesting whatever is available in the water column (Mortensen, 2001; Freiwald, 2002; Duineveld et al., 2004, 2007; Kiriakoulakis et al., 2005; Carlier et al., 2009; Dodds et al., 2009; van Oevelen et al., 2009). They have been reported to feed on zooplankton (Kiriakoulakis et al., 2005; Naumann et al., 2011), including microzooplankton (Houlbrèque et al., 2004); on phytoplankton and phytodetritus, including the bacterial fraction of phytodetritus (Maier et al., 2020; Houlbrèque et al., 2004); and on dissolved organic matter (Mueller et al., 2014; Ferrier, 1991; Al-Moghrabi et al., 1993; Hoegh-Guldberg and Williamson, 1999; Houlbrèque et al., 2004; Grover et al., 2008). Furthermore, the CWC holobiont has been observed to display biological N2 fixation and chemoautotrophy (Middelburg et al., 2016). While it is clear that corals may be able to consume a variety of components within the food web, the soft tissue δ15N of B. elegans specimens collected at Friday Harbor averaged 12.0 ‰, signifying that they fed on material with a δ15N of approximately 9.0 ‰ – accounting for a normal trophic offset relative to their diet (3 ‰), as confirmed by our culture experiment results. Here, we seek to determine the primary nutrition source for B. elegans at Friday Harbor by comparing the δ15N of these corals' expected diet with the measured δ15N of different food web components including SPOM and net tow material.
We first explore whether the SPOM fraction of the food web was the dominant component of B. elegans' diet at Friday Harbor. SPOM is operationally defined as the particulate material retained onto glass-fiber filters (GF/F, 0.7 µm nominal pore size) from filtered aqueous samples. At the ocean surface, including at the stations near Friday Harbor, SPOM is generally dominated by phytoplankton material. At the ocean subsurface, below the euphotic zone, SPOM is derived from organic material exiting the ocean surface but is considered a distinct pool from the ballasted sinking PON collected in sediment traps. The δ15N of SPOM typically increases with depth, with the steepest gradient across the 100–300 m depth interval, reaching upwards of ∼4 ‰–5 ‰ in the ocean subsurface, which are higher values than the corresponding sinking particles at abyssal depths due to recycling and remineralization (Altabet, 1988; Casciotti et al., 2008; Saino and Hattori, 1987). Wang et al. (2014) reasoned that, because the δ15N of SPOM is approximately one trophic level lower that of the N preserved in skeletons of the deep-dwelling (deeper than ∼500 m) CWC Desmophyllum dianthus and as suspended particles are the most abundant form of small particles in the deep ocean, CWCs must feed predominantly on SPOM. However, SPOM collected in the upper 30 m near Friday Harbor had a δ15N of 5.7 ± 1.7 ‰, which is ∼6 ‰ lower than B. elegans soft tissue, a difference greater than expected for a single trophic level. Thus, the SPOM at Friday Harbor was evidently not the predominant food source for B. elegans growing in this depth interval.
Additionally, it has been suggested that CWCs can assimilate dissolved organic nitrogen (DON) (Gori et al., 2014). We do not have δ15N DON measurements from our field study. However, we do not expect the potential assimilation of DON to explain the elevated δ15N of organic tissue that was observed. There are two components of marine DON, refractory and labile (Bronk et al., 2002), which have different δ15N (Knapp et al., 2018). At Friday Harbor, we do not know the partitioning of the δ15N between these pools; however, even if we did, the labile fraction (which would presumably be the pool available to corals) is expected to converge on the δ15N value of SPOM (Bronk et al., 2002; Sigman and Fripiat, 2019, their Fig. 4; Knapp et al., 2018; Zhang et al., 2020), given that the most recently produced DON is generally most labile. As a result, the consumption of DON would not explain the high δ15N of coral organic tissue.
Instead, we suggest that the relatively high δ15N of ∼12 ‰ of B. elegans soft tissue at Friday Harbor results from these corals deriving nutrition predominantly from larger metazoan zooplankton. Indeed, this is supported by a comparison of the δ15N coral tissue and the δ15N of the largest size class of net tow material (≥500 µm) of 8.0 ± 0.8 ‰. This is the only component of the organic matter nitrogen budget that is offset from the coral tissue by ∼3.5 ‰, consistent with one trophic level transfer. Additionally, the net tow material had a molar C:N ratio of 4.4 ± 0.6, compared with 6.5 ± 2.2 for the SPOM (Fig. S8), suggesting that a dietary preference for metazoan zooplankton would provide a higher protein content and nutritional density for these corals (Adams and Sterner, 2000).
Despite evidence that zooplankton is the main dietary source for B. elegans at Friday Harbor, we acknowledge that this feeding strategy may not apply to corals of other species living in habitats that are hundreds to thousands of meters deep. As pointed out in a recent review (Maier et al., 2023), the presence of CWC reefs in the food-limited deep ocean appears paradoxical, and it is not likely that the food available to corals at Friday Harbor looks identical to food available to corals living at >1000 m water depth. Indeed, Maier et al. (2023) suggest that the biodiversity and productivity of CWC reefs in the deep sea are supported by a number of processes, such as CWCs' ability to consume a range of dietary components in addition to zooplankton (dissolved organic matter, DOM; bacterioplankton; and inorganic resources such as inorganic C and ammonium), efficient resource recycling, and their ability to align their feeding strategies and growth with fluctuations in food availability. For example, it has been suggested that sponges, some of which are known to have high δ15N due to efficient internal recycling, generate dissolved and particulate organic nitrogen that is then transferred to other associated deep-sea organisms such as brittle stars (Hanz et al., 2022; Kahn et al., 2018). At the moment, however, we do not have any evidence that this deep-sea “sponge loop” directly influences the N isotope composition of CWCs. Additionally, while we cannot speculate about the flux of DOM to corals living at >1000 m depth, we note that the δ15N of deep DOM has a uniform value of ∼5 ‰, which cannot explain the high δ15N of CWCs (see Sigman and Fripiat, 2019).
Maier et al. (2023) and references therein highlight that most deep CWC reefs occur in regions with higher-than-average annual primary productivity, indicating that these CWC reefs are sustained by inputs of high energy to the system, where there is also evidence of the presence of vertically migrating zooplankton. The vertically migrating zooplankton have been found near both relatively shallow (<200 m; Duineveld et al., 2007; Garcia-Herrera et al., 2022) and deep (∼1000 m; e.g., Carlier et al., 2009) CWC reefs. Moreover, there are a number of other independent studies that reveal a single trophic-level offset between the δ15N of zooplankton prey and the δ15N soft tissue of asymbiotic scleractinian corals at specific sites (Duineveld et al., 2004; Sherwood et al., 2005, 2008, 2009; Carlier et al., 2009; Hill et al., 2014; Maier et al., 2020). Given the “normal” trophic level offset reported for CWCs in our laboratory culture experiment, these published observations underscore that zooplankton could be a dominant dietary component of corals other than B. elegans as well. Additional evidence from lipid biomarkers corroborates the assertion that deep-dwelling CWC species such Lophelia pertusa (recently reclassified as Desmophyllum pertusum) and Madrepora oculata feed predominantly on metazoan zooplankton (Dodds et al., 2009; Kiriakoulakis et al., 2005; Naumann et al., 2015). Some deep-dwelling CWCs (Desmophyllum pertusum, Madrepora oculata, and Dendrophyllia cornigera) exhibit prey preference for larger zooplankton (Da Ros et al., 2022), suggesting that zooplankton prey are an essential component of their diet. Indeed, an exclusive diet of phytodetritus (Maier et al., 2019) and the exclusion of zooplankton from diet (Naumann et al., 2011) led to decreases in coral metabolism. More fundamentally, the shared traits of tentacles and nematocysts are evidence of a predatory life strategy, indicating that zooplankton are an important food source for corals (Lewis and Price, 1975; Sebens et al., 1996). The coral morphology of B. elegans and that of other cold-water scleractinian corals is consistent with an adaptation for the capture of prey of a commensurate size (Fautin, 2009). Correspondingly, D. dianthus is considered to be a generalized zooplankton predator that can prey on medium to large copepods and euphausiids (Höfer et al., 2018). In contrast, gorgonian corals do not capture naturally occurring zooplankton and have a correspondingly low density of nematocysts (Lasker, 1981). In summary, while our data cannot directly indicate that all CWCs, including the deep-dwelling ones, derive their primary nutrition from zooplankton, the results of our trophic experiment and field study (when evaluated in the context of the published literature) suggest that it may be important to consider metazooplankton as a significant component of CWCs' diet and that CWCs' δ15N is likely to be sensitive to food web dynamics. We discuss the implications of these suggestions further in the sections below.
4.5 Does coral-bound δ15N reflect surface ocean processes at Friday Harbor?
The effectiveness of coral-skeleton-bound δ15N as an archive to reconstruct past ocean N cycling depends on its ability to record the δ15N of the surface PON export. In turn, the δ15N imparted to the phytoplankton component of surface particles, from which PON export derives, is highly dependent on surface ocean dynamics that influence the degree of nitrate consumption and associated isotope fractionation. Here, we describe local marine N cycling dynamics in order to evaluate whether coral-bound δ15N recorded in the B. elegans specimens reflects local surface ocean processes.
Given complete assimilation of inorganic N pools, the δ15N of phytoplankton material – the dominant component of SPOM at the surface ocean – converges on the δ15N of the N sources, new nitrate and recycled N sources (Treibergs et al., 2014; Fawcett et al., 2011). At steady state, the δ15N of the sinking PON flux reflects the isotope signature of the nitrate upwelled to the surface (Altabet, 1988). Alternatively, given partial nitrate consumption in the context of a finite pool (Rayleigh dynamic), such as in high-nutrient, low-chlorophyll regions and in upwelling systems, the SPOM δ15N is fractionated relative to the nitrate δ15N as function of the assimilation isotope effect and the extent of nitrate consumption (Sigman et al., 1999). The δ15N of the sinking flux then reflects both the δ15N of nitrate upwelled to the surface and the degree of nitrate consumption (Altabet and François, 1994; François et al., 1997). In this section, we discuss whether coral-bound δ15N reflects the δ15N of nitrate entrained to the surface.
Nitrate assimilation at Friday Harbor appeared to be incomplete, potentially implicating the fractionation of N isotopes between nitrate and biomass. Although depleted nitrate concentrations are generally expected at coastal sites during the summer in density-stratified water column due to phytoplankton assimilation, nitrate concentrations at Friday Harbor in August of 2021 were upwards of 15 µm at the surface and 20 µm at 30 m depth. Indeed, nitrate in the San Juan Channel is replete year-round, even at the surface, due to vigorous mixing within the channel (Mackas and Harrison, 1997; Murray et al., 2015).
The region experiences tidal mixing, designating it as a well-mixed estuary with minimal density stratification (Mackas and Harrison, 1997). The tidal influence is clearly identified from the diurnal patterns of vertical hydrographic structure variability with the salinity/temperature gradients changing with the tidal phase (Fig. 6a, b). The tidal pumping drives vertical mixing between high-nutrient deep water from the Strait of Juan de Fuca and fresher surface water from the Strait of Georgia (Lewis, 1978; Murray et al., 2015; Mackas and Harrison, 1997). Nutrient concentrations in the surface in the Strait of Georgia vary seasonally and are depleted during the summer at the stratified, fresher surface (Del Bel Belluz et al., 2021; Mackas and Harrison, 1997). Our temperature–salinity plot in August 2021 reflects end-member mixing between more saline/colder water from the Strait of Juan de Fuca and fresher/warmer surface water from the Strait of Georgia (Fig. S9). The influence of the Strait of Georgia surface water is recognized by the salinity minima originating from the outflow of the Fraser River (Fig. S10; Mackas and Harrison, 1997). The nitrate profiles in August 2021, although collected with a lower vertical resolution, do show diurnal variability in vertical gradients similar to salinity/temperature, consistent with the tidal mixing effect (Fig. 6c).
The δ15N values of nitrate measured at stations near Friday Harbor also corroborate the mixing of nitrate-rich deeper water with nitrate-deplete surface water from the Strait of Georgia. The apparent isotope effect for nitrate assimilation in August 2021 was ∼1.5 ‰, markedly lower than the canonical value of 5 ‰ associated with nitrate assimilation by surface ocean phytoplankton communities (DiFiore et al., 2006; Sigman et al., 1999; Altabet and François, 1994). A low apparent isotope effect is consistent with the two-end-member mixing of lower-δ15N, nitrate-rich water with highly fractionated (high-δ15N), low-nitrate water (Sigman et al., 1999). Highly fractionated nitrate, in turn, likely originated from the nutrient-depleted Strait of Georgia surface waters entrained into the Channel Islands of California. The linear relationship between salinity and nitrate concentration in August 2021 further substantiates physical mixing as the dominant control on nitrate concentrations and isotope ratios in San Juan Channel (Fig. S10; Mackas and Harrison, 1997). Moreover, the δ15N of nitrate was relatively uniform with depth, indicating effective vertical mixing of the Strait of Georgia and Strait of Juan de Fuca water masses. The relatively slight decrease in nitrate δ15N with depth suggests a secondary influence of local nitrate assimilation on its concentration and isotope ratios.
The corresponding δ15N of SPOM at Friday Harbor covered a broad range, from 4.2 ‰ to 8.7 ‰ in August 2021. The depth distribution of SPOM did not mirror the corresponding nitrate δ15N profile, as could otherwise be expected. At the stratified near-surface (5 m) at station 1, the δ15N of SPOM averaged 4.2 ‰ compared with 7.4 ‰ for nitrate. In the context of Rayleigh fractionation, this result suggests that particulate material at the surface consisted primarily of the instantaneous product of nitrate assimilation (Mariotti et al., 1981). The lower δ15N SPOM values could also reflect some degree of reliance with respect to regenerated N species, which would result in a δ15N of SPOM that is lower than that of incident nitrate (Fawcett et al., 2011; Lourey et al., 2003; Treibergs et al., 2014). Deeper in the water column, the δ15N of SPOM converged on the δ15N of incident nitrate, between 6 ‰ and 7 ‰, suggesting that SPOM was derived from the complete consumption of an incident nitrate pool (even though nitrate was present at these depths). Phytoplankton at these depths may, thus, have originated from surface water entrained from the Strait of Georgia – where nitrate was completely utilized. The above dynamics complicate validation of the offset between the δ15N of exported PON and coral-bound δ15N. However, we find little evidence of nitrate fractionation from partial assimilation on δ15N of phytoplankton SPOM, which suggests that the δ15N imparted on local B. elegans skeletons should reflect the δ15N of nitrate entrained to the surface. The ∼7 ‰ difference between coral skeleton δ15N (∼13.5 ‰) and the entrained nitrate (∼6.5 ‰) is similar to the empirical range of 7 ‰–9 ‰ reported for other CWC species, such as D. pertusum (Kiriakoulakis et al., 2005) and D. dianthus (Wang et al., 2014), and suggests that B. elegans provides a record of the thermocline nitrate δ15N and surface nutrient dynamics at Friday Harbor.
We conclude that the solitary scleractinian cold-water coral B. elegans in Friday Harbor, WA, predominantly derives nutrition from metazoan zooplankton prey. While our study was limited to a shallow field site, our isotope feeding experiment, evaluated alongside previously published studies, points to the possibility that deeper-dwelling CWCs could also rely on zooplankton prey as a fundamental component of their diet. SPOM may contribute to these CWCs' diet, but it cannot be presumed to exclusively account for the large offset between the δ15N of PON export and coral skeleton δ15N documented by Wang et al. (2014). The δ15N of skeletal material recovered from coral archives is, thus, likely to be sensitive to local food web dynamics; for a given δ15N of sinking PON exiting the surface ocean, the δ15N recorded by CWC may differ among individuals of the same species feeding on different zooplankton prey, depending on availability. In fact, Wang et al. (2014) did report a “natural variability” of 1 ‰–1.5 ‰ within a single specimen that might have resulted from some variability in the local food web on a short timescale of few years. Some studies have documented an increase in the degree of carnivory of zooplankton with depth (Dodds et al., 2009; Vinogradov, 1962). For instance, Hannides et al. (2013) recorded a 3.5 ‰ increase in zooplankton δ15N from 150 to 1000 m in the subtropical North Pacific, with the steepest rate of increase from 100 to 300 m. Koppelmann et al. (2009) reported a similar pattern of zooplankton δ15N through the water column. Corals feeding on carnivorous zooplankton that have elevated δ15N at depth could explain small but resolvable (1 ‰–2 ‰) increases in coral δ15N with increasing depth (Wang et al., 2014). The δ15N recorded in CWC skeletons also tends to differ by 1 ‰–2 ‰ among species, as respective species occupy different nutritional niches (Teece et al., 2011). The relationship between CWC species represented in fossil archives to the depth structure of their zooplankton prey warrants further investigation.
Consideration of the possible dependence of coral-bound δ15N on food web dynamics informs the questions that can be competently addressed by this proxy. Although we do not have direct estimates of the δ15N range that can be expected from local food web variability, the scatter around the global compilation of Wang et al. (2014) for the coral-bound δ15N of D. dianthus relative to the δ15N of PON suggests that this range is modest, on the order of ∼1 ‰–2 ‰. Given this range, we suggest that the coral-bound δ15N proxy will be most useful for reconstructing larger environmental δ15N signals and where chosen coral samples belong to the same species and are collected at comparable depths, as has already been successfully demonstrated by Wang et al. (2017), Studer et al. (2018), and Chen et al. (2023). If used in this way, the broad geographic and temporal coverage afforded by CWCs, the opportunity to measure multiple proxies from individual specimens, and the imperviousness of coral-bound δ15N to diagenetic alteration render it a valuable paleo-proxy for reconstructing marine N cycling.
The data presented in this paper are available from https://www.bco-dmo.org/project/893811 (Gothmann et al., 2024).
The supplement related to this article is available online at: https://doi.org/10.5194/bg-21-1071-2024-supplement.
JG, AMG, and MGP conceptualized the research presented in this paper. JLM and AMG designed and carried out culture experiments. MGP and AC prepared coral samples for analysis. JLM and VR analyzed samples. JLM, AMG, JG, and KD collected water samples, SPOM, and net tows. KD collected live corals for culture experiments and field studies. JLM and JG prepared the manuscript with contributions from all co-authors.
The contact author has declared that none of the authors has any competing interests.
Publisher’s note: Copernicus Publications remains neutral with regard to jurisdictional claims made in the text, published maps, institutional affiliations, or any other geographical representation in this paper. While Copernicus Publications makes every effort to include appropriate place names, the final responsibility lies with the authors.
We are grateful to Friday Harbor Laboratories for their assistance with the coral collections and field sampling (especially Pema Kitaeff and Megan Dethier). We acknowledge the valued assistance of the Artemia Reference Center (specifically Gilbert Van Stappen and Christ Mahieu). Coral culture experiments would not have been sustained without the help of St. Olaf College undergraduate students Rachel Raser, Joash Daniel, Qintiantian Nong, YiWynn Chan, Mansha Haque, Natasia Preys, and Miranda Lenz. We are also indebted to Craig Tobias and Peter Ruffino for access to and assistance with the elemental analyzer–isotope ratio mass spectrometer.
This research has been supported by the Directorate for Geosciences (grant nos. OCE-1949984, OCE-1949132, and OCE-1949119).
This paper was edited by Marcel van der Meer and reviewed by Philip Riekenberg and Ulrike Hanz.
Adams, T. S. and Sterner, R. W.: The effect of dietary nitrogen content on trophic level 15N enrichment, Limnol. Oceanogr., 45, 601–607, https://doi.org/10.4319/lo.2000.45.3.0601, 2000.
Adkins, J. F., Henderson, G. M., Wang, S.-L., O'Shea, S., and Mokadem, F.: Growth rates of the deep-sea Scleractinia Desmophyllum cristagalli and Enallopsammia rostrata, Earth Planet. Sc. Lett., 227, 481–490, https://doi.org/10.1016/j.epsl.2004.08.022, 2004.
Al-Moghrabi, S., Allemand, D., and Jaubert, J.: Valine uptake by the scleractinian coral Galaxea fascicularis: characterization and effect of light and nutritional status, J. Comp. Physiol. B, 163, 355–362, https://doi.org/10.1007/BF00265638, 1993.
Altabet, M. A.: Variations in nitrogen isotopic composition between sinking and suspended particles: implications for nitrogen cycling and particle transformation in the open ocean, Deep Sea Res., 35, 535–554, https://doi.org/10.1016/0198-0149(88)90130-6, 1988.
Altabet, M. A. and Francois, R.: Sedimentary nitrogen isotopic ratio as a recorder for surface ocean nitrate utilization, Global Biogeochem. Cy., 8, 103–116, https://doi.org/10.1029/93GB03396, 1994.
Altabet, M. A., Deuser, W. G., Honjo, S., and Stienen, C.: Seasonal and depth-related changes in the source of sinking particles in the North Atlantic, Nature, 354, 136–139, https://doi.org/10.1038/354136a0, 1991.
Altabet, M., Higginson, M., and Murray, D.: The effect of millennial-scale changes in Arabian Sea denitrification on atmospheric CO2, Nature, 415, 159–162, https://doi.org/10.1038/415159a, 2002.
Ayliffe, L. K., Cerling, T. E., Robinson, T., West, A. G., Sponheimer, M., Passey, B. H., Hammer, J., Roeder, B., Dearing, M. D., and Ehleringer, J. R.: Turnover of carbon isotopes in tail hair and breath CO2 of horses fed an isotopically varied diet, Oecologia, 139, 11–22, https://doi.org/10.1007/s00442-003-1479-x, 2004.
Beauchamp, K. A.: Aspects of gametogenesis, development and planulation in laboratory populations of solitary corals and corallimorpharian sea anemones (PhD), University of California, Santa Cruz, United States – California, 1989.
Böhlke, J. K., Mroczkowski, S. J., and Coplen, T. B.: Oxygen isotopes in nitrate: new reference materials for 18O : 17O : 16O measurements and observations on nitrate-water equilibration, Rapid Commun. Mass Sp., 17, 1835–1846, https://doi.org/10.1002/rcm.1123, 2003.
Braman, R. S. and Hendrix, S. A.: Nanogram nitrite and nitrate determination in environmental and biological materials by vanadium(III) reduction with chemiluminescence detection, Anal. Chem., 61, 2715–2718, https://doi.org/10.1021/ac00199a007, 1989.
Brandes, J. A. and Devol, A. H.: A global marine-fixed nitrogen isotopic budget: Implications for Holocene nitrogen cycling, Global Biogeochem. Cy., 16, 1–14, https://doi.org/10.1029/2001GB001856, 2002.
Bronk, D. A.: Dynamics of DON. Biogeochem. Mar. Dissolved Org. Matter, 153–249, 2002.
Brown, B. E. and Bythell, J. C.: Perspectives on mucus secretion in reef corals, Mar. Ecol. Prog. Ser., 296, 291–309, 2005.
Cairns, S. D.: Deep-water corals: an overview with special reference to diversity and distribution of deep-water scleractinian corals, Bull. Mar. Sci., 81, 311–322, 2007.
Carlier, A., Guilloux, E. L., Olu, K., Sarrazin, J., Mastrototaro, F., Taviani, M., and Clavier, J.: Trophic relationships in a deep Mediterranean cold-water coral bank (Santa Maria di Leuca, Ionian Sea), Mar. Ecol. Prog. Ser., 397, 125–137, https://doi.org/10.3354/meps08361, 2009.
Carpenter, E. J., Harvey, H. R., Fry, B., and Capone, D. G.: Biogeochemical tracers of the marine cyanobacterium Trichodesmium, Deep-Sea Res. Pt. I, 44, 27–38, https://doi.org/10.1016/S0967-0637(96)00091-X, 1997.
Casciotti, K. L., Sigman, D. M., Hastings, M. G., Böhlke, J. K., and Hilkert, A.: Measurement of the oxygen isotopic composition of nitrate in seawater and freshwater using the denitrifier method, Anal. Chem., 74, 4905–4912, https://doi.org/10.1021/ac020113w, 2002.
Casciotti, K. L., Trull, T. W., Glover, D. M., and Davies, D.: Constraints on nitrogen cycling at the subtropical North Pacific Station ALOHA from isotopic measurements of nitrate and particulate nitrogen, Deep Sea Res. Pt. II, 55, 1661–1672, https://doi.org/10.1016/j.dsr2.2008.04.017, 2008.
Cathalot, C., Van Oevelen D., Cox, T. J. S., Kutti, T., Lavaleye, M., Duineveld, G., and Meysman, F. J. R.: Cold-water coral reefs and adjacent sponge grounds: hotspots of benthic respiration and organic carbon cycling in the deep sea, Front. Mar. Sci., 2, 37, https://doi.org/10.3389/fmars.2015.00037, 2015.
Cerling, T. E., Ayliffe, L. K., Dearing, M. D., Ehleringer, J. R., Passey, B. H., Podlesak, D. W., Torregrossa, A.-M., and West, A. G.: Determining biological tissue turnover using stable isotopes: the reaction progress variable, Ecophysiology, 151, 175–189, https://doi.org/10.1007/s00442-006-0571-4, 2007.
Chen, W. H., Ren, H., Chiang, J. C. H., Wang, Y. L., Cai-Li, R. Y., Chen, Y. C., Shen, C. C., Taylor, F. W., DeCarlo, T. M., Wu, C. R., Mii, H. S., and Wang, X. T.: Increased tropical South Pacific western boundary current transport over the past century, Nat. Geosci., 16, 590–596, https://doi.org/10.1038/s41561-023-01212-4, 2023.
Cheng, H., Adkins, J., Edwards, R. L., and Boyle, E. A.: U-Th dating of deep-sea corals, Geochim. Cosmochim. Ac., 64, 2401–2416, https://doi.org/10.1016/S0016-7037(99)00422-6, 2000.
Crook, E. D., Cooper, H., Potts, D. C., Lambert, T., and Paytan, A.: Impacts of food availability and pCO2 on planulation, juvenile survival, and calcification of the azooxanthellate scleractinian coral Balanophyllia elegans, Biogeosciences, 10, 7599–7608, https://doi.org/10.5194/bg-10-7599-2013, 2013.
Da Ros, Z., Dell'Anno, A., Fanelli, E., Angeletti, L., Taviani, M., and Danovaro, R.: Food preferences of Mediterranean cold-water corals in captivity, Front. Mar. Sci., 9, 867656, https://doi.org/10.3389/fmars.2022.867656, 2022.
Del Bel Belluz, J., Peña, M. A., Jackson, J. M., and Nemcek, N.: Phytoplankton composition and environmental drivers in the Northern Strait of Georgia (Salish Sea), British Columbia, Canada, Estuar. Coast., 44, 1419–1439, https://doi.org/10.1007/s12237-020-00858-2, 2021.
De Pol-Holz, R., Robinson, R. S., Hebbeln, D., Sigman, D. M., and Ulloa, O.: Controls on sedimentary nitrogen isotopes along the Chile margin, DDeep-Sea Res. Pt. II, 56, 1042–1054 https://doi.org/10.1016/j.dsr2.2008.09.014, 2009.
DiFiore, P. J., Sigman, D. M., Trull, T. W., Lourey, M. J., Karsh, K., Cane, G., and Ho, R.: Nitrogen isotope constraints on subantarctic biogeochemistry, J. Geophys. Res.-Oceans, 111, C08016, https://doi.org/10.1029/2005JC003216, 2006.
Dodds, L. A., Black, K. D., Orr, H., and Roberts, J. M.: Lipid biomarkers reveal geographical differences in food supply to the cold-water coral Lophelia pertusa (Scleractinia), Mar. Ecol. Prog. Ser., 397, 113–124, https://doi.org/10.3354/meps08143, 2009.
Doi, H., Akamatsu, F., and González, A. L.: Starvation effects on nitrogen and carbon stable isotopes of animals: an insight from meta-analysis of fasting experiments, R. Soc. Open Sci., 4, 170633, https://doi.org/10.1098/rsos.170633, 2017.
Drake, J. L., Guillermic, M., Eagle, R. A., and Jacobs, D. K.: Fossil corals with various degrees of preservation can retain information about biomineralization-related organic material, Front. Earth Sci., 9, 643864, https://doi.org/10.3389/feart.2021.643864, 2021.
Druffel, E. R. M.: Geochemistry of corals: Proxies of past ocean chemistry, ocean circulation, and climate, P. Natl. Acad. Sci. USA, 94, 8354–8361, https://doi.org/10.1073/pnas.94.16.8354, 1997.
Duineveld, G. C. A., Lavaleye, M. S. S., and Berghuis, E. M.: Particle flux and food supply to a seamount cold-water coral community (Galicia Bank, NW Spain), Mar. Ecol. Prog. Ser., 277, 13–23, https://doi.org/10.3354/meps277013, 2004.
Duineveld, G., Lavaleye, M., Bergman, M., Stigter, H., and Mienis, F.: Trophic structure of a cold-water coral mound community (Rockall Bank, NE Atlantic) in relation to the near-bottom particle supply and current regime, Bull. Mar. Sci., 81, 449–467, 2007.
Durham, J. W. and Barnard, J. L.: Stony corals of the Eastern Pacific collected by the Velero III and Velero IV, Al1an Hancock Pacific Expeditions, 16, 1–110, 1952.
Erler, D. V., Wang, X. T., Sigman, D. M., Scheffers, S. R., and Shepherd, B. O.: Controls on the nitrogen isotopic composition of shallow water corals across a tropical reef flat transect, Coral Reefs, 34, 329–338, https://doi.org/10.1007/s00338-014-1215-5, 2015.
Fadlallah, Y. H.: Population Dynamics and Life History of a Solitary Coral, Balanophyllia elegans, from Central California, Oecologia, 58, 200–207, 1983.
Fautin, D. G.: Structural diversity, systematics, and evolution of cnidae. Toxicon, Cnidarian Toxins and Venoms, 54, 1054–1064, https://doi.org/10.1016/j.toxicon.2009.02.024, 2009.
Fawcett, S. E., Lomas, M. W., Casey, J. R., Ward, B. B., and Sigman, D. M.: Assimilation of upwelled nitrate by small eukaryotes in the Sargasso Sea, Nat. Geosci., 4, 717–722, https://doi.org/10.1038/ngeo1265, 2011.
Ferrier, M. D.: Net uptake of dissolved free amino acids by four scleractinian corals, Coral Reefs, 10, 183–187, https://doi.org/10.1007/BF00336772, 1991.
François, R., Altabet, M. A., Yu, E.-F., Sigman, D. M., Bacon, M. P., Frank, M., Bohrmann, G., Bareille, G., and Labeyrie, L. D.: Contribution of Southern Ocean surface-water stratification to low atmospheric CO2 concentrations during the last glacial period, Nature, 389, 929–935, https://doi.org/10.1038/40073, 1997.
Freiwald, A.: Reef-Forming Cold-Water Corals. In: Wefer, G., Billett, D., Hebbeln, D., Jørgensen, B. B., Schlüter, M., and van Weering, T. C. E. (Eds.): Ocean Margin Systems, Springer, Berlin, Heidelberg, https://doi.org/10.1007/978-3-662-05127-6_23, 2002.
Gagnon, A. C., Gothmann, A. M., Branson, O., Rae, J. W. B., and Stewart, J. A.: Controls on boron isotopes in a cold-water coral and the cost of resilience to ocean acidification, Earth Planet. Sc. Lett., 554, 116662, https://doi.org/10.1016/j.epsl.2020.116662, 2021.
Ganeshram, R. S. and Pedersen, T. F.: Glacial-interglacial variability in upwelling and bioproductivity off NW Mexico: Implications for Quaternary paleoclimate, Paleoceanography, 13, 634–645, https://doi.org/10.1029/98PA02508, 1998.
Garcia-Herrera, N., Cornils, A., Laudien, J., Niehoff, B., Höfer, J., Försterra, G., González, H. E., and Richter, C.: Seasonal and diel variations in the vertical distribution, composition, abundance and biomass of zooplankton in a deep Chilean Patagonian Fjord, PeerJ, 10, e12823, https://doi.org/10.7717/peerj.12823, 2022.
Genin, A., Dayton, P. K., Lonsdale, P. F., and Spiess, F. N.: Corals on seamount peaks provide evidence of current acceleration over deep-sea topography, Nature, 322, 59–61, https://doi.org/10.1038/322059a0, 1986.
Gerrodette, T.: Equatorial Submergence in a Solitary Coral, Balanophyllia elegans, and the Critical Life Stage Excluding the Species from Shallow Water in the South, Mar. Ecol. Prog-Ser., 1, 227–235, 1979.
Gonfiantini, R., W. Stichler, and Rosanski, K.: Standards and Intercomparison. Materials Distributed by the IAEA for Stable Isotope Measurements, Int. At. Energy Agency, Vienna, 1995.
Goodfriend, G. A., Hare, P. E., and Druffel, E. R. M.: Aspartic acid racemization and protein diagenesis in corals over the last 350 years, Geochim. Cosmochim. Ac., 56, 3847–3850, https://doi.org/10.1016/0016-7037(92)90176-J, 1992.
Gori, A., Grover, R., Orejas, C., Sikorski, S., and Ferrier-Pagès, C.: Uptake of dissolved free amino acids by four cold-water coral species from the Mediterranean Sea, Deep-Sea Res. Pt. II, 99, 42–50, https://doi.org/10.1016/j.dsr2.2013.06.007, 2014.
Gothmann A. M., Stolarski J., Adkins J. F., Schoene, B., Dennis, K. J., Schrag, D. P., Mazur, M., Bender, M. L.: Fossil corals as an archive of secular variations in seawater chemistry since the Mesozoic. Geochim Cosmochim. Ac., 160, 188–208, https://doi.org/10.1016/j.gca.2015.03.018, 2015.
Gothmann, A. M., Granger, J., and Prokopenko, M.: “Collaborative Research: Refining the use of scleractinian cold-water coral skeleton-bound δ15N as a proxy for marine N cycling” Biological and Chemical Oceanography Data Management Office (BCO-DMO), [data set], https://www.bco-dmo.org/project/893811 (last access: 1 February 2024), 2024.
Grover, R. Maguer, J.-F., Allemand, D., and Ferrier-Pagès, C.: Uptake of dissolved free amino acids by the scleractinian coral Stylophora pistillata, J. Exp. Biol., 21, 860–865, doi: https://doi.org/10.1242/jeb.012807, 2008.
Hannides, C. S., Popp, B. N., Choy, C. A., and Drazen, J. C.: Midwater zooplankton and suspended particle dynamics in the North Pacific Subtropical Gyre: A stable isotope perspective, Limnol. Oceanogr., 58, 1931–1946, https://doi.org/10.4319/lo.2013.58.6.1931, 2013.
Hill, T. M., Myrvold, C. R., Spero, H. J., and Guilderson, T. P.: Evidence for benthic–pelagic food web coupling and carbon export from California margin bamboo coral archives, Biogeosciences, 11, 3845–3854, https://doi.org/10.5194/bg-11-3845-2014, 2014.
Hines, S. K. V., Southon, J. R., and Adkins, J. F.: A high-resolution record of Southern Ocean intermediate water radiocarbon over the past 30,000 years, Earth Planet. Sc. Lett., 432, 46–58, https://doi.org/10.1016/j.epsl.2015.09.038, 2015.
Hoegh-Guldberg, O. and Williamson, J.: Availability of two forms of dissolved nitrogen to the coral Pocillopora damicornis and its symbiotic zooxanthellae, Mar. Biol., 133, 561–570, https://doi.org/10.1007/s002270050496, 1999.
Höfer, J., González, H. E., Laudien, J., Schmidt, G. M., Häussermann, V., and Richter, C.: All you can eat: the functional response of the cold-water coral Desmophyllum dianthus feeding on krill and copepods, PeerJ, 6, e5872, https://doi.org/10.7717/peerj.5872, 2018.
Horn, M. G., Robinson, R. S., Rynearson, T. A., and Sigman, D. M.: Nitrogen isotopic relationship between diatom-bound and bulk organic matter of cultured polar diatoms, Paleoceanography, 26, PA3208, https://doi.org/10.1029/2010PA002080, 2011.
Kast, E. R., Stolper, D. A., Auderset, A., Higgins, J. A., Ren, H., Wang, X. T., Martínez-García, A., Haug, G. H., and Sigman, D. M.: Nitrogen isotope evidence for expanded ocean suboxia in the early Cenozoic, Science, 364, 386–389, https://doi.org/10.1126/science.aau5784, 2019.
Kiriakoulakis, K., Fisher, E., Wolff, G. A., Freiwald, A., Grehan, A., and Roberts, J. M.: Lipids and nitrogen isotopes of two deep-water corals from the North-East Atlantic: initial results and implications for their nutrition, edited by: Freiwald, A., Roberts, J. M., Cold-Water Corals and Ecosystems, Erlangen Earth Conference Series, Springer, Berlin, Heidelberg, 715–729, https://doi.org/10.1007/3-540-27673-4_37, 2005.
Knapp, A. N., Casciotti, K. L., and Prokopenko, M. G.: Dissolved Organic Nitrogen Production and Consumption in Eastern Tropical South Pacific Surface Waters, Global Biogeochem. Cy., 32, 769–783, https://doi.org/10.1029/2017GB005875, 2018.
Knapp, A. N., DiFiore, P. J., Deutsch, C., Sigman, D. M., and Lipschultz, F.: Nitrate isotopic composition between Bermuda and Puerto Rico: Implications for N2 fixation in the Atlantic Ocean, Global Biogeochem. Cy., 22, GB3015, https://doi.org/10.1029/2007GB003107, 2008.
Koppelmann, R., Böttger-Schnack, R., Möbius, J., and Weikert, H.: Trophic relationships of zooplankton in the eastern Mediterranean based on stable isotope measurements, J. Plankton Res., 31, 669–686, 2009.
Lasker, H. R.: A comparison of the particulate feeding abilities of three species of Gorgonian soft coral, Mar. Ecol. Prog.-Ser., 5, 61–67, 1981.
Lewis, A. G.: Concentrations of nutrients and chlorophyll on a cross-channel transect in Juan de Fuca Strait, British Columbia, J. Fish. Res. Board Can., 35, 305–314, https://doi.org/10.1139/f78-055, 1978.
Lewis, J. B. and Price, W. S.: Feeding mechanisms and feeding strategies of Atlantic reef corals, J. Zool., 176, 527–544, https://doi.org/10.1111/j.1469-7998.1975.tb03219.x, 1975.
Li, T., Robinson, L. F., Chen, T., Wang, X. T., Burke, A., Rae, J. W. B., Pegrum-Haram, A., Knowles, T. D. J., Li, G., Chen, J., Ng, H. C., Prokopenko, M., Rowland, G. H., Samperiz, A., Stewart, J. A., Southon, J., and Spooner, P. T.: Rapid shifts in circulation and biogeochemistry of the Southern Ocean during deglacial carbon cycle events, Sci. Adv., 6, eabb3807, https://doi.org/10.1126/sciadv.abb3807, 2020.
Lourey, M. J., Trull, T. W., and Sigman, D. M.: Sensitivity of δ15N of nitrate, surface suspended and deep sinking particulate nitrogen to seasonal nitrate depletion in the Southern Ocean, Global Biogeochem. Cy., 17, 1081, https://doi.org/10.1029/2002GB001973, 2003.
Mackas, D. L. and Harrison, P. J.: Nitrogenous nutrient sources and sinks in the Juan de Fuca Strait/Strait of Georgia/Puget Sound estuarine system: Assessing the potential for eutrophication, Estuar. Coast. Shelf Sci., 44, 1–21, https://doi.org/10.1006/ecss.1996.0110, 1997.
Maier, S. R., Bannister, R. J., van Oevelen, D., and Kutti, T.: Seasonal controls on the diet, metabolic activity, tissue reserves and growth of the cold-water coral Lophelia pertusa, Coral Reefs, 39, 173–187, https://doi.org/10.1007/s00338-019-01886-6, 2020.
Maier, S. R., Kutti, T., Bannister, R. J., van Breugel, P., van Rijswijk, P., and van Oevelen, D.: Survival under conditions of variable food availability: Resource utilization and storage in the cold-water coral Lophelia pertusa, Limnol. Oceanogr., 64, 1651–1671, https://doi.org/10.1002/lno.11142, 2019.
Maier, S. R., Brooke, S., De Clippele, L. H., de Froe, E., van der Kaaden, A.-S., Kutti, T., Mienis, F., and van Oevelen, D.: On the paradox of thriving cold-water coral reefs in the food-limited deep sea, Biol. Rev., 98, 1768–1795, https://doi.org/10.1111/brv.12976, 2023.
Marconi, D., Weigand, A. M., Rafter, P. A., Matthew, R. McIlvin, M. R., Forbes, M., Casciotti, K. L., and Sigman, D. M.: Nitrate isotope distributions on the US GEOTRACES North Atlantic cross-basin section: Signals of polar nitrate sources and low latitude nitrogen cycling, Mar. Chem., 177, 143–156, https://doi.org/10.1016/j.marchem.2015.06.007, 2015.
Margolin, A. R., L. F. Robinson, A. Burke, R. G. Waller, K. M. Scanlon, M. L. Roberts, M. E. Auro, and van de Flierdt, T.: Temporal and spatial distributions of cold-water corals in the Drake Passage: Insights from the last 35,000 years. Deep Sea Res. Pt. II, 99, 237–248, https://doi.org/10.1016/j.dsr2.2013.06.008, 2014.
Mariotti, A., Germon, J. C., Hubert, P., Kaiser, P., Letolle, R., Tardieux, A., and Tardieux, P.: Experimental determination of nitrogen kinetic isotope fractionation: Some principles; illustration for the denitrification and nitrification processes, Plant Soil, 62, 413–430, https://doi.org/10.1007/BF02374138, 1981.
Martínez del Rio, C. and Carleton, S. A.: How fast and how faithful: the dynamics of isotopic incorporation into animal tissues, J. Mammal., 93, 353–359, https://doi.org/10.1644/11-MAMM-S-165.1, 2012.
McCutchan Jr, J. H., Lewis, Jr, W. M., Kendall, C., and McGrath, C. C.: Variation in trophic shift for stable isotope ratios of carbon, nitrogen, and sulfur, Oikos, 102, 378–390, https://doi.org/10.1034/j.1600-0706.2003.12098.x, 2003.
McIlvin, M. R. and Casciotti, K. L.: Technical Updates to the Bacterial Method for Nitrate Isotopic Analyses, Anal. Chem., 83, 1850–1856, https://doi.org/10.1021/ac1028984, 2011.
McMahon, K. W., Williams, B., Guilderson, T. P., Glynn, D. S., and McCarthy, M. D.: Calibrating amino acid δ13C and δ15N offsets between polyp and protein skeleton to develop proteinaceous deep-sea corals as paleoceanographic archives, Geochim. Cosmochim. Acta, 220, 261–275, https://doi.org/10.1016/j.gca.2017.09.048, 2018.
Middelburg, J., Mueller, C., Veuger, B. Larsson, A. I., Form, A., and van Oevelen, D.: Discovery of symbiotic nitrogen fixation and chemoautotrophy in cold-water corals. Sci. Rep., 5, 17962, https://doi.org/10.1038/srep17962, 2016.
Miller, M.: Growth of a temperate coral: effects of temperature, light, depth, and heterotrophy, Mar. Ecol. Prog. Ser., 122, 217–225, https://doi.org/10.3354/meps122217, 1995.
Minagawa, M. and Wada, E.: Stepwise enrichment of δ15N along food chains: Further evidence and the relation between δ15N and animal age, Geochim. Cosmochim. Ac., 48, 1135–1140, https://doi.org/10.1016/0016-7037(84)90204-7, 1984.
Mortensen P. B.: Aquarium observations on the deep-water coral Lophelia pertusa (L., 1758) (scleractinia) and selected associated invertebrates, Ophelia, 54, 83-104, https://doi.org/10.1080/00785236.2001.10409457, 2001.
Muhs, D. R., Kennedy, G. L., and Rockwell, T. K.: Uranium-Series Ages of Marine Terrace Corals from the Pacific Coast of North America and Implications for Last-Interglacial Sea Level History, Quat. Res., 42, 72–87, https://doi.org/10.1006/qres.1994.1055, 1994.
Mueller, C. E., Larsson, A. I., Veuger, B., Middelburg, J. J., and van Oevelen, D.: Opportunistic feeding on various organic food sources by the cold-water coral Lophelia pertusa, Biogeosciences, 11, 123–133, https://doi.org/10.5194/bg-11-123-2014, 2014.
Murray, J. W., Roberts, E., Howard, E., O'Donnell, M., Bantam, C., Carrington, E., Foy, M., Paul, B., and Fay, A.: An inland sea high nitrate-low chlorophyll (HNLC) region with naturally high pCO2, Limnol. Oceanogr., 60, 957–966, https://doi.org/10.1002/lno.10062, 2015.
Muscatine, L., Goiran, C., Land, L., Jaubert, J., Cuif, J.-P., and Allemand, D.: Stable isotopes (δ13C and δ15N) of organic matrix from coral skeleton, P. Natl. Acad. Sci. USA, 102, 1525–1530, https://doi.org/10.1073/pnas.0408921102, 2005.
Naumann, M. S., Orejas, C., Wild, C., and Ferrier-Pagès, C.: First evidence for zooplankton feeding sustaining key physiological processes in a scleractinian cold-water coral, J. Exp. Biol., 214, 3570–3576, https://doi.org/10.1242/jeb.061390, 2011.
Naumann, M. S., Tolosa, I., Taviani, M., Grover, R., and Ferrier-Pagès, C.: Trophic ecology of two cold-water coral species from the Mediterranean Sea revealed by lipid biomarkers and compound-specific isotope analyses, Coral Reefs, 34, 1165–1175, https://doi.org/10.1007/s00338-015-1325-8, 2015.
Pride, C., Thunell, R., Sigman, D. M., Keigwin, L., Altabet, M., and Tappa, E.: Nitrogen isotopic variations in the Gulf of California since the Last Deglaciation: Response to global climate change, Paleoceanography, 14, 397–409, https://doi.org/10.1029/1999PA900004, 1999.
Purser, A., Larsson, A. I., Thomsen, L., and van Oevelen D.: The influence of flow velocity and food concentration on Lophelia pertusa (Scleractinia) zooplankton capture rates, J. Exp. Mar. Bio. Ecol., 395, 55–62, https://doi.org/10.1016/j.jembe.2010.08.013, 2010.
Rae, J. W. B.: Boron Isotopes in Foraminifera: Systematics, Biomineralisation, and CO2 Reconstruction, in: Marschall, H. and Foster, G., Boron Isotopes, Advances in Isotope Geochemistry, Springer, Cham., https://doi.org/10.1007/978-3-319-64666-4_5, 2018.
Rangel, M. S., Erler, D., Tagliafico, A., Cowden, K., Scheffers, S., and Christidis, L.: Quantifying the transfer of prey δ15N signatures into coral holobiont nitrogen pools, Mar. Ecol. Prog. Ser., 610, 33–49, https://doi.org/10.3354/meps12847, 2019.
Ren, H., Sigman, D. M., Meckler, A. N., Plessen, B., Robinson, R. S., Rosenthal, Y., and Haug, G. H.: Foraminiferal Isotope Evidence of Reduced Nitrogen Fixation in the Ice Age Atlantic Ocean, Science, 323, 244–248, https://doi.org/10.1126/science.1165787, 2009.
Reynaud, S., Martinez, P., Houlbrèque, F., Billy, I., Allemand, D., and Ferrier-Pagès, C.: Effect of light and feeding on the nitrogen isotopic composition of a zooxanthellate coral: role of nitrogen recycling, Mar. Ecol. Prog.-Ser., 392, 103–110, https://doi.org/10.3354/meps08195, 2009.
Roberts, J. M., Wheeler, A. J., and Freiwald, A.: Reefs of the deep: The biology and geology of cold-water coral ecosystems, Science, 312, 543–547, https://doi.org/10.1126/science.1119861, 2006.
Robinson, R. S., Kienast, M., Albuquerque, A. L., Altabet, M., Contreras, S., Holz, R. D. P., Dubois, N., Francois, R., Galbraith, E., Hsu, T.-C., Ivanochko, T., Jaccard, S., Kao, S.-J., Kiefer, T., Kienast, S., Lehmann, M., Martinez, P., McCarthy, M., Möbius, J., Pedersen, T., Quan, T. M., Ryabenko, E., Schmittner, A., Schneider, R., Schneider-Mor, A., Shigemitsu, M., Sinclair, D., Somes, C., Studer, A., Thunell, R., and Yang, J.-Y.: A review of nitrogen isotopic alteration in marine sediments, Paleoceanography, 27, PA4203, https://doi.org/10.1029/2012PA002321, 2012.
Robinson, L. F., Adkins, J. F., Frank, N., Gagnon, A. C., Prouty, N. G., Roark, B., and van de Flierdt, T.: The geochemistry of deep-sea coral skeletons: A review of vital effects and applications for palaeoceanography, Deep Sea Res Pt. II, 99, 184–198, https://doi.org/10.1016/j.dsr2.2013.06.005, 2014.
Robinson, R. S. and Sigman D. M.: Nitrogen isotopic evidence for a poleward decrease in surface nitrate within the ice age Antarctic, Quat. Sci. Rev., 27, 1076–1090, https://doi.org/10.1016/j.quascirev.2008.02.005, 2008.
Robinson, R. S., Smart, S. M., Cybulski, J. D., McMahon, K. W., Marcks, B., and Nowakowski, C.: Insights from fossil-bound nitrogen isotopes in diatoms, foraminifera, and corals, Annu. Rev. Mar. Sci., 15, 407–430, https://doi.org/10.1146/annurev-marine-032122-104001, 2023.
Ryan, W. B. F., Carbotte, S. M., Coplan, J., O'Hara, S., Melkonian, A., Arko, R., Weissel, R. A., Ferrini, V., Goodwillie, A., Nitsche, F., Bonczkowski, J., and Zemsky, R.: Global Multi-Resolution Topography (GMRT) synthesis data set, Geochem. Geophys. Geosyst., 10, Q03014, https://doi.org/10.1029/2008GC002332, 2009.
Saino, T. and Hattori, A.: Geographical variation of the water column distribution of suspended particulate organic nitrogen and its 15N natural abundance in the Pacific and its marginal seas, Deep Sea Res., 34, 807–827, https://doi.org/10.1016/0198-0149(87)90038-0, 1987.
Scrimgeour, C. M., Gordon, S. C., Handley, L. L., and Woodford, J. A. T.: Trophic levels and anomalous δ15N of insects on raspberry (Rubus Idaeus L.). Isotopes Environ, Health Stud., 31, 107–115, https://doi.org/10.1080/10256019508036256, 1995.
Sebens, K. P., Vandersall, K. S., Savina, L. A., and Graham, K. R.: Zooplankton capture by two scleractinian corals, Madracis mirabilis andMontastrea cavernosa, in a field enclosure, Mar. Biol., 127, 303–317, https://doi.org/10.1007/BF00942116, 1996.
Sherwood, O. A., Heikoop, J. M., Scott, D. B., Risk, M. J., Guilderson, T. P., and McKinney, R. A.: Stable isotopic composition of deep-sea gorgonian corals Primnoa spp.: a new archive of surface processes, Mar. Ecol. Prog.-Ser., 301, 135–148, https://doi.org/10.3354/meps301135, 2005.
Sherwood, O. A., Jamieson, R. E., Edinger, E. N., and Wareham, V. E.: Stable C and N isotopic composition of cold-water corals from the Newfoundland and Labrador continental slope: Examination of trophic, depth and spatial effects. Deep Sea Res., 55, 1392–1402, https://doi.org/10.1016/j.dsr.2008.05.013, 2008.
Sherwood, O. A., Thresher, R. E., Fallon, S. J., Davies, D. M., and Trull, T. W.: Multi-century time-series of 15N and 14C in bamboo corals from deep Tasmanian seamounts: evidence for stable oceanographic conditions, Mar. Ecol. Prog.-Ser., 397, 209–218, https://doi.org/10.3354/meps08166, 2009.
Sigman, D. M., Altabet, M. A., McCorkle, D. C., Francois, R., and Fischer, G.: The δ15N of nitrate in the Southern Ocean: Consumption of nitrate in surface waters, Global Biogeochem. Cy., 13, 1149–1166, https://doi.org/10.1029/1999GB900038, 1999.
Sigman, D. M. and Boyle, E.: Glacial/interglacial variations in atmospheric carbon dioxide, Nature, 407, 859–869, https://doi.org/10.1038/35038000, 2000.
Sigman, D. M., Casciotti, K. L., Andreani, M., Barford, C., Galanter, M., and Böhlke, J. K.: A Bacterial method for the nitrogen isotopic analysis of nitrate in seawater and freshwater, Anal. Chem., 73, 4145–4153, https://doi.org/10.1021/ac010088e, 2001.
Sigman, D. M. and Fripiat, F.: Nitrogen Isotopes in the Ocean, in: Cochran, J. K., Bokuniewicz, H. J., and Yager, P. L., Encyclopedia of Ocean Sciences (Third Edition), Academic Press, Oxford, 263–278, https://doi.org/10.1016/B978-0-12-409548-9.11605-7, 2019.
Soetaert, K., Mohn, C., Rengstorf, A., Grehan, A., and van Oevelen, D.: Ecosystem engineering creates a direct nutritional link between 60 m deep cold-water coral mounds and surface productivity, Sci. Rep., 6, 35057, https://doi.org/10.1038/srep35057, 2016.
Spero, H. J., Andreasen, D. J., and Sorgeloos, P.: Carbon and nitrogen isotopic composition of different strains of Artemia sp., Int. J. Salt Lake Res., 2, 133, https://doi.org/10.1007/BF02905905, 1993.
Studer, A. S., Sigman, D. M., Martinez-Garcia, A., Thole, L. M., Michel, E., Jaccard, S. L., Lippolds, J. A., Mazaud, A., Wang, X. C. T., Robinson, L. F., Adkins, J. F., and Haug, G. H.: Increased nutrient supply to the Southern Ocean during the Holocene and its implications for the pre-industrial atmospheric CO2 rise, Nat. Geosci., 11, 756–761, 2018.
Tanaka, Y., Miyajima, T., Koike, I., Hayashibara, T., and Ogawa, H.: Translocation and conservation of organic nitrogen within the coral-zooxanthella symbiotic system of Acropora pulchra, as demonstrated by dual isotope-labeling techniques, J. Exp. Mar. Biol. Ecol., 336, 110–119, https://doi.org/10.1016/j.jembe.2006.04.011, 2006.
Tanaka, Y., Suzuki, A., and Sakai, K.: The stoichiometry of coral-dinoflagellate symbiosis: carbon and nitrogen cycles are balanced in the recycling and double translocation system, ISME J., 12, 860–868, https://doi.org/10.1038/s41396-017-0019-3, 2018.
Teece, M. A., Estes, B., Gelsleichter, E., and Lirman, D.: Heterotrophic and autotrophic assimilation of fatty acids by two scleractinian corals, Montastraea faveolata and Porites astreoides, Limnol. Oceanogr., 56, 1285–1296, https://doi.org/10.4319/lo.2011.56.4.1285, 2011.
Thiagarajan, N., Subhas, A. V., Southon, J. R., Eiler, J. M., and Adkins, J. F.: Abrupt pre-Bolling-Allerod warming and circulation changes in the deep ocean, Nature, 511, 75–78, https://doi.org/10.1038/nature13472, 2014.
Thiem, Ø., Ravagnan, E., Fosså, J. H., and Berntsen, J.: Food supply mechanisms for cold-water corals along a continental shelf edge, J. Mar. Syst., 60, 207–219, https://doi.org/10.1016/j.jmarsys.2005.12.004, 2006.
Thomas, S. M. and Crowther, T. W.: Predicting rates of isotopic turnover across the animal kingdom: a synthesis of existing data, J. Anim. Ecol., 84, 861–870, https://doi.org/10.1111/1365-2656.12326, 2015.
Treibergs, L. A., Fawcett, S. E., Lomas, M. W., and Sigman, D. M.: Nitrogen isotopic response of prokaryotic and eukaryotic phytoplankton to nitrate availability in Sargasso Sea surface waters, Limnol. Oceanogr., 59, 972–985, https://doi.org/10.4319/lo.2014.59.3.0972, 2014.
Tsounis, G., Orejas, C., Reynaud, S., Allemand, D., and Ferrier-Pagès, C.: Prey-capture rates in four Mediterranean cold water corals, Mar. Ecol. Prog.-Ser., 398, 149–155, https://doi.org/10.3354/meps08312, 2010.
van Oevelen, P., Duineveld, G., Lavaleye, M., Mienis, F., Soetaert, K., and Carlo, H. R.: The cold-water coral community as hotspot of carbon cycling on continental margins: A food-web analysis from Rockall Bank (northeast Atlantic), Limnol. Oceanogr., 54, 1829–1844, https://doi.org/10.4319/lo.2009.54.6.1829, 2009.
Vinogradov, M. E.: Feeding of the deep-sea zooplankton, Rapp. Pv. Reun. Cons. Perm. Int. Exp. Mer., 153, 114–120, 1962.
Wang, X. T., Prokopenko, M. G., Sigman, D. M., Adkins, J. F., Robinson, L. F., Ren, H., Oleynik, S., Williams, B., and Haug, G. H.: Isotopic composition of carbonate-bound organic nitrogen in deep-sea scleractinian corals: A new window into past biogeochemical change, Earth Planet. Sc. Lett., 400, 243–250, https://doi.org/10.1016/j.epsl.2014.05.048, 2014.
Wang, X. T., Sigman, D. M., Prokopenko, M. G., Adkins, J. F., Robinson, L. F., Hines, S. K., Chai, J., Studer, A. S., Martínez-García, A., Chen, T., and Haug, G. H.: Deep-sea coral evidence for lower Southern Ocean surface nitrate concentrations during the last ice age, P. Natl. Acad. Sci. USA, 114, 3352–3357, https://doi.org/10.1073/pnas.1615718114, 2017.
Webb, S., Hedges, R., and Simpson, S.: Diet quality influences the δ13C and δ15N of locusts and their biochemical components, J. Exp. Biol., 201, 2903–2911, https://doi.org/10.1242/jeb.201.20.2903, 1998.
Weigand, M. A., Foriel, J., Barnett, B., Oleynik, S., and Sigman, D. M.: Updates to instrumentation and protocols for isotopic analysis of nitrate by the denitrifier method, Rapid Commun. Mass Spectrom., 30, 1365–1383, https://doi.org/10.1002/rcm.7570, 2016.
Williams, B. and Grottoli, A. G.: Recent shoaling of the nutricline and thermocline in the western tropical Pacific, Geophys. Res. Lett., 37, L22601, https://doi.org/10.1029/2010GL044867, 2010.
Zhang, R., Wang, X. T., Ren, H., Huang, J., Chen, M., and Sigman, D. M.: Dissolved Organic Nitrogen Cycling in the South China Sea from an Isotopic Perspective, Global Biogeochem. Cy., 34, e2020GB006551, https://doi.org/10.1029/2020GB006551, 2020.
Zhou, M., Granger, J., and Chang, B. X.: Influence of sample volume on nitrate N and O isotope ratio analyses with the denitrifier method, Rapid Commun. Mass Sp., 36, e9224, https://doi.org/10.1002/rcm.9224, 2022.