the Creative Commons Attribution 4.0 License.
the Creative Commons Attribution 4.0 License.
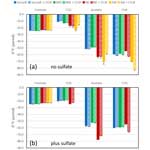
Fractionation of stable carbon isotopes during formate consumption in anoxic rice paddy soils and lake sediments
Peter Claus
Formate is energetically equivalent to hydrogen and thus is an important intermediate during the breakdown of organic matter in anoxic rice paddy soils and lake sediments. Formate is a common substrate for methanogenesis, homoacetogenesis and sulfate reduction. However, how much these processes contribute to formate degradation and fractionate carbon stable isotopes is largely unknown. Therefore, we measured the conversion of formate to acetate, CH4 and CO2 and the δ13C of these compounds in samples of paddy soils from Vercelli, Italy, and the International Rice Research Institute (IRRI) in the Philippines and of sediments from the NE and SW basins of Lake Fuchskuhle, Germany. The samples were suspended in a phosphate buffer (pH 7.0) in both the absence and presence of sulfate (gypsum) and of methyl fluoride (CH3F), an inhibitor of aceticlastic methanogenesis. In the paddy soils, formate was mainly converted to acetate under both methanogenic and sulfidogenic conditions. Methane was only a minor product and was mainly formed from the acetate. In the lake sediments, the product spectrum was similar but only under methanogenic conditions. In the presence of sulfate, however, acetate and CH4 were only minor products. The isotopic enrichment factors (εform) of formate consumption, determined by Mariotti plots, were in the low range of −8 ‰ to −2.5 ‰ when sulfate was absent, and formate was mainly converted to acetate and CH4. However, no enrichment factor was detectable when formate was degraded with sulfate to mainly CO2. The δ13C of acetate was by about 25 ‰–50 ‰ more negative than that of formate, indicating acetate production by chemolithotrophic homoacetogenesis. Hence, formate seems to be an excellent substrate for homoacetogenesis in anoxic soils and sediments, so that this process is competing well with methanogenesis and sulfate reduction.
- Article
(4807 KB) - Full-text XML
-
Supplement
(1048 KB) - BibTeX
- EndNote
Formate is energetically almost equivalent to H2 (Schink et al., 2017) and thus is an important intermediate in the anaerobic degradation of organic matter. Formate is a product of microbial fermentation, where it is produced in pyruvate cleavage by pyruvate formate lyase (Thauer et al., 1977) or by reduction in CO2 (Schuchmann and Müller, 2013). Formate can also be produced in secondary fermentation, such as oxidation of butyrate or propionate (Dong et al., 1994; Sieber et al., 2014). In fact, formate and H2 may be used equivalently as electron shuttles between secondary fermenting bacteria and methanogens (Montag and Schink, 2018; Schink et al., 2017).
Formate can serve alternatively to H2 as a substrate for methanogenesis (Zinder, 1993) (homo)acetogenesis (Drake, 1994) or sulfate reduction (Widdel, 1988), i.e.,
Formate may also be a substrate for syntrophic bacteria, which live from the little Gibbs free energy ( kJ mol−1) that is generated by the conversion of formate to H2 plus CO2 (Dolfing et al., 2008; Kim et al., 2010; Martins et al., 2015), i.e.,
Formate can also be enzymatically equilibrated with H2 and CO2 without energy generation. This reaction happens in any organism possessing the suitable enzymes, such as formate hydrogen lyase or hydrogen-dependent carbon dioxide reductase, and in anoxic sediments (DeGraaf and Cappenberg, 1996; Peters et al., 1999; Schuchmann et al., 2018):
Formate has been identified as an important substrate for methanogenesis, homoacetogenesis or sulfate reduction in lake sediments (DeGraaf and Cappenberg, 1996; Lovley and Klug, 1982; Phelps and Zeikus, 1985), soils (Kotsyurbenko et al., 1996; Küsel and Drake, 1999; Rothfuss and Conrad, 1993), mires (Hausmann et al., 2016; Hunger et al., 2011; Liebner et al., 2012; Wüst et al., 2009) and marine sediments (Glombitza et al., 2015). However, it is not very clear to which extent formate-dependent methanogenesis, homoacetogenesis and sulfate reduction are actually operative and to which extent formate affects stable carbon isotope fractionation. The δ13C values of compounds involved in the degradation process of organic matter provide valuable information on the metabolic pathways involved (Conrad, 2005; Elsner et al., 2005; Hayes, 1993). However, for correct interpretation, the knowledge of the enrichment factors (ε) of the major metabolic processes is also important. The ε values of methanogenesis or homoacetogenesis from H2 plus CO2 are large (Blaser and Conrad, 2016). However, our knowledge of carbon isotope fractionation with formate as a substrate is scarce. In cultures of homoacetogenic bacteria, the carbon in the acetate produced from formate was strongly depleted in 13C ( ‰), almost on the same level as with CO2 as carbon source (Freude and Blaser, 2016). However, it is not known which enrichment factors operate in methanogenic or sulfidogenic environmental samples.
Therefore, we measured isotope fractionation in methanogenic and sulfidogenic rice paddy soils and in lake sediments amended with formate. We recorded the consumption of formate along with the production of acetate, CH4 and CO2 and measured the δ13C of these compounds. We also used the treatment with methyl fluoride (CH3F) to inhibit the consumption of acetate by methanogenic archaea (Janssen and Frenzel, 1997). We used the same environmental samples as we did for the study of carbon isotope fractionation during consumption of acetate (Conrad et al., 2021) and propionate (Conrad and Claus, 2023), i.e., rice paddy soils from Vercelli, Italy, and the International Rice Research Institute (IRRI) in the Philippines and sediments from the NE and SW basins of Lake Fuchskuhle, Germany. The molecular data characterizing the microbial community compositions in these samples are found in Conrad et al. (2021).
2.1 Environmental samples and incubation conditions
The soil samples were from the research stations in Vercelli, Italy, and the International Rice Research Institute (IRRI) in the Philippines. Sampling and soil characteristics were described before (Liu et al., 2018). The lake sediments (top 10 cm layer) were from the NE and SW basins of Lake Fuchskuhle, an acidic bog (pH 4.2–4.6) lake in northeastern Germany (Casper et al., 2003). The lake was artificially divided into four compartments in an ongoing process from 1987 to 1991, resulting in four nearly equal-sized compartments, each with a different catchment area. The NE basin is characterized by higher biomass and activity throughout all trophic levels in the water column than those of the SW basin. The lake sediments were sampled in July 2016 using a gravity core sampler as described before (Kanaparthi et al., 2013). The experiments with rice field soil were carried out in 2016, while the experiments with sediments of Lake Fuchskuhle were carried out in 2017.
The experimental setup was exactly the same as during previous studies of acetate consumption (Conrad et al., 2021) and propionate consumption (Conrad and Claus, 2023). For methanogenic conditions, paddy soil was mixed with autoclaved anoxic H2O (prepared under N2) at a ratio of 1 : 1 and incubated under N2 at 25 °C for 4 weeks. In a second incubation, for sulfidogenic conditions, paddy soil was mixed with autoclaved anoxic H2O at a ratio of 1 : 1, was amended with 0.07 g CaSO4 ⋅ 2H2O, and was then incubated under N2 at 25 °C for 4 weeks. These two preincubated soil slurries were sampled and stored at −20 °C for later molecular analysis (see data in Conrad et al., 2021). The preincubated soil slurries were also used (in three replicates) for the following incubation experiments. Two different sets of incubations were prepared. In the first set (resulting in methanogenic conditions), 5 mL of soil slurry preincubated without sulfate was incubated at 25 °C with 40 mL of 20 mM potassium phosphate buffer (pH 7.0) in a 150 mL bottle under an atmosphere of N2. The bottles were amended with (i) 5 mL H2O, (ii) 5 mL H2O + 4.5 mL CH3F, (iii) 5 mL 200 mM sodium formate, and (iv) 5 mL 200 mM sodium formate + 4.5 mL CH3F. In the second set (resulting in sulfidogenic conditions), 5 mL of soil slurry preincubated with sulfate was incubated at 25 °C with 40 mL of 20 mM potassium phosphate buffer (pH 7.0) in a 150 mL bottle under an atmosphere of N2. The amendments were the same as above but with the addition of 200 µL of a CaSO4 suspension corresponding to a concentration of 2.5 M (giving a final concentration of 10 mM sulfate).
For lake sediments under methanogenic conditions, 5 mL sediment was incubated in three replicates at 10 °C (which is close to the in situ temperature) with 40 mL of 20 mM potassium phosphate buffer (pH 7.0) in a 150 mL bottle under an atmosphere of N2. The bottles were amended with (i) 5 mL H2O, (ii) 5 mL H2O + 4.5 mL CH3F, (iii) 5 mL 200 mM sodium formate, and (iv) 5 mL 200 mM sodium formate + 4.5 mL CH3F. For sulfidogenic conditions, lake sediments were preincubated with sulfate by adding 0.1 g CaSO4 ⋅ 2H2O (gypsum) to 50 mL of sediment and incubating at 10 °C for 4 weeks. For sulfidogenic conditions, 5 mL of the preincubated sediment was incubated in three replicates at 10 °C with 40 mL of 20 mM potassium phosphate buffer (pH 7.0) in a 150 mL bottle under an atmosphere of N2. The bottles were amended as above but also with 200 µL of a CaSO4 suspension, giving a final concentration of 10 mM sulfate. Samples for later molecular analysis were taken from the original lake sediment and from the lake sediment preincubated with sulfate. The samples were stored at −20 °C (see data in Conrad et al., 2021).
2.2 Chemical and isotopic analyses
Gas samples for the analysis of partial pressures of CH4 and CO2 were taken from the headspace of the incubation bottles after vigorous manual shaking for about 30 s using a gas-tight pressure-lock syringe which had been flushed with N2 before each sampling. Soil slurries were sampled, centrifuged and filtered through a 0.2 µm cellulose membrane filter and stored frozen at −20 °C for later fatty acid analysis. Chemical and isotopic analyses were performed as described in detail previously (Goevert and Conrad, 2009). Methane was analyzed by gas chromatography (GC) with a flame ionization detector. Carbon dioxide was analyzed after conversion to CH4 with a Ni catalyst. Stable isotope analyses of in gas samples were performed using GC-combustion isotope ratio mass spectrometry (GC-C-IRMS). Formate and acetate were measured using high-performance liquid chromatography (HPLC) linked via a Finnigan LC IsoLink to an IRMS. The isotopic values are reported in the delta notation (δ13C) relative to the Vienna PeeDee Belemnite standard, having a ratio (Rstandard) of 0.01118: δ13C = 103 (). The precision of the GC-C-IRMS was ±0.2 ‰, and that of the HPLC-IRMS was ±0.3 ‰.
2.3 Calculations
Millimolar concentrations of CH4 were calculated from the mixing ratios (1 ppmv = 10−6 bar) measured in the gas phase of the incubation bottles, with 1000 ppmv CH4 corresponding to 0.09 µmol per milliliter of liquid. Note that this is the total amount of CH4 in the gas phase relative to the liquid phase.
Fractionation factors for reaction A→B are defined after Hayes (1993) as
also expressed as in permil. The carbon isotope enrichment factor εform associated with formate consumption was calculated from the temporal change in δ13C of formate as described by Mariotti et al. (1981) from the residual reactant
where δri is the isotopic composition of the reactant (formate) at the beginning and δr is the isotopic composition of the residual formate, both at the instant when f is determined. fform is the fractional yield of the products based on the consumption of formate (). Linear regression of δ13C of formate against ln (1−f) yields εform as the slope of best-fit lines. The regressions of δ13C of formate were done for data in the range of fform<0.7. The linear regressions were done individually for each experimental replicate (n=3) and were only accepted if r2>0.7. The ε values resulting from the replicate experiments were then averaged (± SE).
3.1 Conversion of formate under methanogenic and sulfidogenic conditions
The rice paddy soils were submerged and preincubated to create methanogenic or sulfidogenic conditions. Samples of these soils were suspended in a buffer at pH 7 and amended with formate. In the Vercelli soil, formate was consumed after a lag phase of 4 d under methanogenic and 5 d under sulfidogenic conditions (Fig. 1a). During this time the pH increased from pH 7 up to pH 8 despite buffering. Formate consumption was not inhibited by CH3F (Fig. 1a). Similar results were obtained with IRRI soil (Fig. S1 in the Supplement). Acetate was produced concomitantly with formate consumption, again without effect by CH3F (Fig. 1b). The production of acetate under sulfidogenic conditions was smaller than under methanogenic conditions. Methane was also produced under both methanogenic and sulfidogenic conditions concomitantly with formate consumption (Figs. 1c; S1c). It is noteworthy that CH3F inhibited the production of CH4 (Figs. 1c; S1c). Finally, CO2 was produced under all conditions without lag phase and without effect by CH3F (Fig. 1d). In Vercelli soil, CO2 production was about 2 times larger under sulfidogenic conditions than under methanogenic conditions (Fig. 1d). In IRRI soil, it was only slightly larger (Fig. S1d). The accumulation of acetate plus CH4 was equimolar to the consumption of formate in terms of electron equivalents, while the accumulation of CH4 alone accounted for only <30 % and in the presence of CH3F even less (Figs. 2a; S2a). Hence, acetate was the more important product of formate consumption. Under sulfidogenic conditions, accumulation of acetate plus CH4 was less than equimolar, especially in Vercelli soil (Fig. 2b), probably since formate was instead converted to CO2. However, acetate formation was still substantial, accounting for 60 %–80 % of formate consumption (Figs. 2b; S2b).
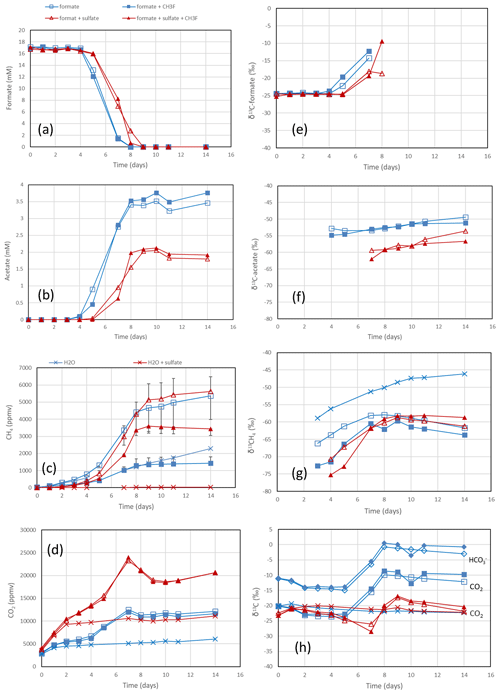
Figure 1Formate conversion to acetate, CH4 and CO2 in suspensions of paddy soil from Vercelli, Italy, after the addition of formate without sulfate (blue squares) or formate plus sulfate (gypsum) (red triangles), without CH3F (open symbols) or with CH3F (closed symbols). Controls with the addition of only water (blue or red × crosses) are only shown occasionally. The panels show the temporal change in (a) concentrations of formate, (b) concentrations of acetate, (c) mixing ratios of CH4 (1 ppmv = 10−6 bar), (d) mixing ratios of CO2, (e) δ13C of formate, (f) δ13C of acetate, (g) δ13C of CH4 and (h) δ13C of CO2. Means ± SE.
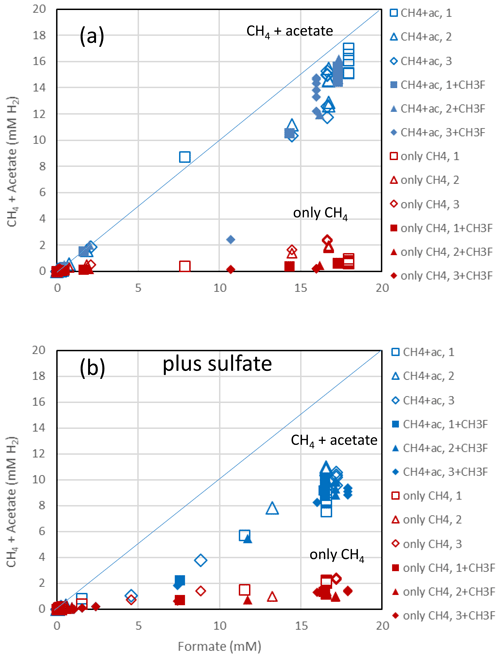
Figure 2Balance of produced acetate plus CH4 (blue symbols) and of only CH4 (red symbols) against the consumed formate in (a) the absence and (b) the presence of sulfate in paddy soil from Vercelli, Italy. Acetate and CH4 are each equivalent to 4 H2, while formate is equivalent to 1 H2. The open and closed symbols denote conditions in the absence and the presence of CH3F, respectively. The different symbols indicate three different replicates. The lines indicate equimolarity (in terms of reducing equivalents between substrate and product).
The sediments from Lake Fuchskuhle were methanogenic in situ so that preincubation of the samples was not required. However, sulfidogenic conditions were created analogously to the paddy soils by preincubtion with sulfate (gypsum). Substantial formate depletion did not start before about 20 d of incubation in sediments from the NE basin (Fig. 3) and the SW basin (Fig. S3). Again, CH3F only inhibited the production of CH4 but not that of acetate or CO2 (Figs. 3; S3). The main difference to the paddy soils was that CH4 was not produced concomitantly with formate consumption but started right from the beginning. However, the amounts of CH4 produced were only small and were apparently due to the little formate that was consumed in the beginning of incubation (i.e., before day 20), as seen by the fact that CH4 production in the water control (not amended with formate) was negligible (Figs. 3c; S3c). Production of CO2 started without lag phase but accelerated together with formate consumption (Figs. 3d; S3d). In the lake sediments, CH4 accounted only for <10 % of formate consumption, while acetate was the main product when sulfate was absent (Figs. 4a, S4a). In contrast to the paddy soils, formate consumption in both lake sediments was much slower under sulfidogenic conditions than under methanogenic conditions (Figs. 3a; S3a). In the sediment from SW basin, formate consumption was very slow; therefore, less than half of the formate was consumed during 80 d of incubation and consumption was not completed until the end of the experiment (Fig. S3a). Very little acetate was produced and no CH4 was formed from formate in both lake sediments, when sulfate was present (Figs. 4b, S4b).
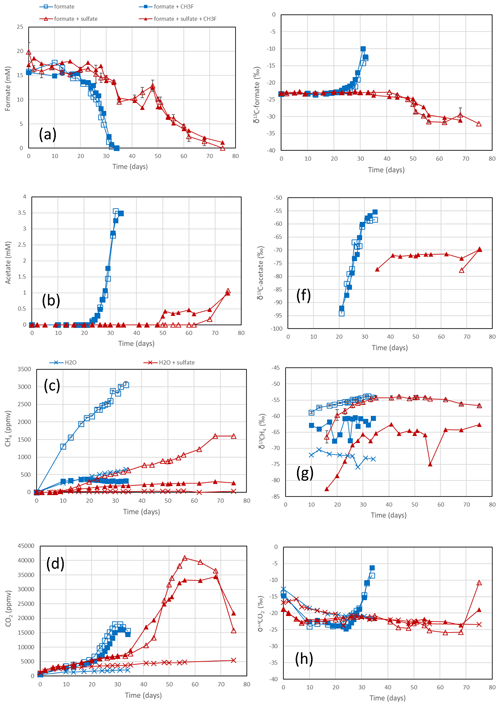
Figure 3Formate conversion to acetate, CH4 and CO2 in suspensions of sediment from the NE basin of Lake Fuchskuhle after the addition of formate without sulfate (blue squares) or formate plus sulfate (gypsum) (red triangles), without CH3F (open symbols) or with CH3F (closed symbols). Controls with the addition of only water (blue or red × crosses) are only shown occasionally. The panels show the temporal change in (a) concentrations of formate, (b) concentrations of acetate, (c) mixing ratios of CH4 (1 ppmv = 10−6 bar), (d) mixing ratios of CO2, (e) δ13C of formate, (f) δ13C of acetate, (g) δ13C of CH4 and (h) δ13C of CO2. Means ± SE.
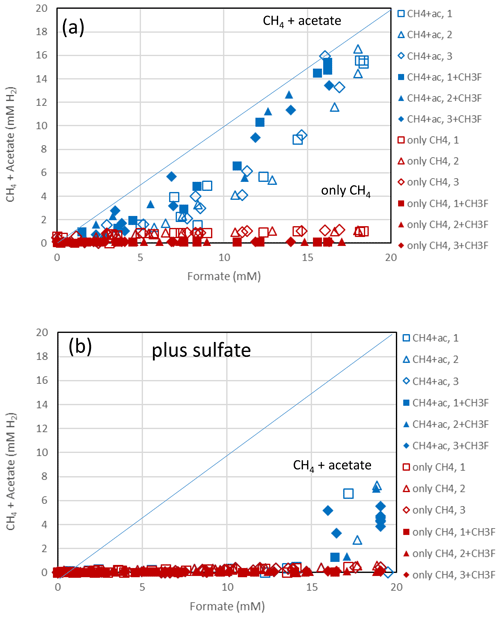
Figure 4Balance of produced acetate plus CH4 (blue symbols) and of only CH4 (red symbols) against the consumed formate in (a) the absence and (b) the presence of sulfate in sediment from the NE basin of Lake Fuchskuhle. Acetate and CH4 are each equivalent to 4 H2, while formate is equivalent to 1 H2. The open and closed symbols denote conditions in the absence and the presence of CH3F, respectively. The different symbols indicate three different replicates. The lines indicate equimolarity (in terms of reducing equivalents between substrate and product).
3.2 Isotope fractionation during formate consumption
In the rice paddy soils, δ13C values of formate increased when formate was consumed, indicating discrimination against the heavy carbon isotope. This process was not affected by CH3F and was similar without and with sulfate (Figs. 1e; S1e). The same was the case with the sediment from the NE lake basin but only in the absence of sulfate (Fig. 3e). With sulfate, the δ13C of formate slowly decreased with time (Fig. 3e). In the sediment from the SW basin, δ13C of formate slowly decreased (without sulfate) or stayed constant with time (with sulfate) (Fig. S3e). Note that formate was not completely consumed in the SW sediment when sulfate was present (Fig. S3a).
Mariotti plots of δ13C of formate as a function of fform resulted in negative slopes (Figs. 4; S5). Hence, the enrichment factors (εform) for the paddy soils, both without and with sulfate, and for the sediments from the NE basin of Lake Fuchskuhle without sulfate showed that the light isotope of formate carbon was preferred. Values of εform were in the range of −8.5 ‰ to −2.5 ‰ (Fig. 6). Under sulfidogenic conditions, however, the Mariotti plots of the sediments from the NE basin (Fig. 5) did not show a negative slope and εform could not be determined. The same was the case for the sediments from the SW basin (Fig. 6).
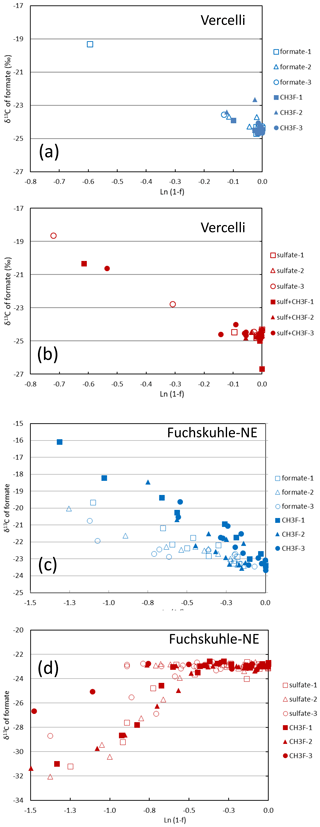
Figure 5Mariotti plots of formate consumption in (a, b) paddy soil from Vercelli and (c, d) sediment from the NE basin of Lake Fuchskuhle under methanogenic (a, c, blue symbols) and sulfidogenic (b, d, red symbols) conditions, both in the absence (open symbols) and in the presence (closed symbols) of CH3F.
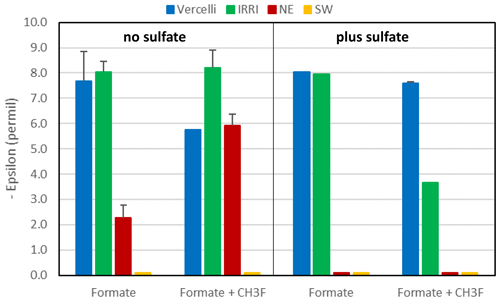
Figure 6Isotopic enrichment factors (εform, given as negative values) in paddy soils from Vercelli and the IRRI (the Philippines) and in lake sediments from the NE and SW basins of Lake Fuchskuhle without (left panel) and with (right panel) the addition of sulfate (gypsum) and CH3F. Means ± SE.
The negative εform indicates that products of formate should be depleted in 13C. Indeed, the δ13C of acetate and CH4 was generally more negative than the δ13C of formate. This was the case in the paddy soils from Vercelli (Fig. 1f) and the IRRI (Fig. S1f), as well as in the sediments from the NE basin (Fig. 3f) and the SW basin (Fig. S3f) of Lake Fuchskuhle. In the sediment of the NE basin, the δ13C of acetate increased from very low level, at −95 ‰, to finally about −57 ‰ in parallel with formate consumption (Fig. 3f). CO2 was also produced during formate degradation to various extent (Reactions R1, R2 and R3). Since the pH was in a range of pH 7 to pH 8, CO2 was also converted to bicarbonate. The δ13C of bicarbonate is generally more positive than the δ13C of CO2 by about 10 ‰ (Stumm and Morgan, 1996). The δ13C of the gaseous CO2 was always close to the δ13C of formate or was more positive. In the paddy soils and the NE basin of Lake Fuchskuhle, the δ13C of CO2 increased in parallel with the increasing δ13C of formate (Figs. 1h, 3h; S1h). The δ13C of the gaseous CO2 produced from the formate-amended samples was initially more negative than that from the unamended samples, but eventually the δ13C increased above these values when formate was completely consumed (Figs. 1h, 3h; S3h).
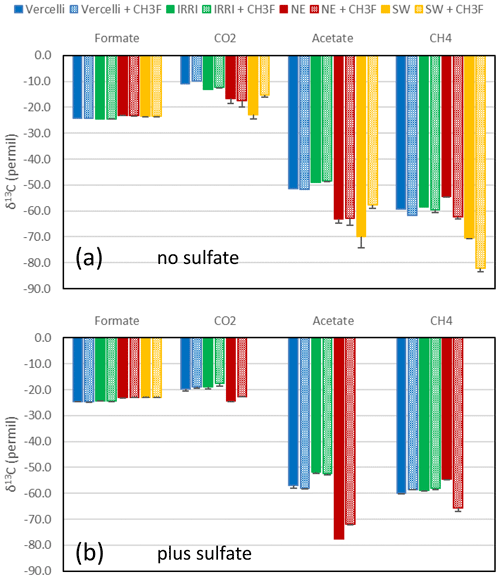
Figure 7Average δ13C of formate (at the beginning of incubation) and of CO2, acetate and CH4 (after the depletion of formate) in paddy soils from Vercelli (blue) and the IRRI (green) and in sediments from the NE basin (red) and the SW basin (yellow) of Lake Fuchskuhle in the absence (filled bars) and the presence (dotted bars) of CH3F. Means ± SE.
The δ13C values of the initial formate were about −24 ‰ (Fig. 5). When formate was completely consumed, the δ13C values of the products acetate and CH4 were always more negative. The average δ13C values of the products after complete consumption of formate are shown in Fig. 7. In the absence of sulfate, δ13C of acetate was in a range of −51 ‰ to −49 ‰ and −70 ‰ to −63 ‰, in the paddy soils and lake sediments, respectively (Fig. 7). In the presence of sulfate, δ13C of acetate was in a range of −57 ‰ to −52 ‰ and −78 ‰ to −72 ‰, in the paddy soils and lake sediments (only NE basin), respectively (Fig. 7). The δ13C of CH4 was in a range of −70 ‰ to −54 ‰ and −60 ‰ to −54 ‰, in the absence and presence of sulfate, respectively (Fig. 7). The δ13C of gaseous CO2 (for bicarbonate plus 10 ‰) was in a range of −23 ‰ to −11 ‰ and −24 ‰ to −19 ‰, in the absence and presence of sulfate, respectively (Fig. 7).
4.1 Formate degradation under acetogenic/methanogenic conditions
In rice paddy soils, formate was consumed within <10 d. The absence of sulfate did not allow sulfidogenic (Reaction R3) degradation but allowed the operation of methanogenic (Reaction R1), homoacetogenic (Reaction R2) or syntrophic (Reaction R4) degradation. Syntrophic degradation is still disputed, since many microorganisms are able to enzymatically equilibrate H2 and formate and thus prohibit exploitation of the difference in the energy content (Montag and Schink, 2018; Schink et al., 2017). Syntrophic formate degradation is exergonic by only a few kilojoules of Gibbs free energy per mole and requires the coupling with methanogenesis or other efficient hydrogen (electron) scavengers. Although formate-driven CH4 production was observed in our study, the production was sensitive to inhibition by CH3F, indicating that CH4 was predominantly produced from acetate rather than from H2. Therefore, syntrophic formate oxidation coupled to CH4 production was probably not a major pathway.
Acetate was the most important product of formate degradation in the paddy soils and the lake sediments. Methane also was a product but was much less important than acetate. Furthermore, it was predominantly produced from acetate, as shown by the inhibition by CH3F and the concomitant decrease in δ13C of CH4, which is characteristic of hydrogenotrophic methanogesis that is not inhibited by CH3F (Conrad et al., 2010). Hence, formate was apparently primarily degraded by homoacetogenesis (Reaction R1). Only part of the produced acetate was immediately used by aceticlastic methanogenesis generating CH4 as secondary product. Although formate is a perfect substrate for homoacetogenic bacteria operating the Wood–Ljungdahl pathway (WLP) (Drake, 1994), the yield of Gibbs free energy per mole formate is less for homoacetogenic than for methanogenic degradation (Dolfing et al., 2008). Thus, it is surprising that formate-driven homoacetogenesis prevailed over methanogenesis. Nevertheless, simultaneous operation of homoacetogenesis and methanogenesis from formate has been observed before in a fen soil (Hunger et al., 2011). Homoacetogenesis prevailing over methanogenesis has also frequently been observed with H2/CO2 as substrate (Conrad et al., 1989; Nozhevnikova et al., 1994), indicating that homoacetogens can take particular advantage from low temperatures (Conrad, 2023) or the availability of secondary substrates (Peters et al., 1998). It is noteworthy that homoacetogens have to invest energy for fixation of formate, while methanogens are able to bypass this step (Lemaire et al., 2020). Perhaps it is such energy investment which makes the homoacetogens to competitive with formate utilizers.
Formate consumption was recorded upon the addition of formate to initial concentrations of about 15 mM, which was much higher than the in situ concentration being typically on the order of a few micromoles per liter (Montag and Schink, 2018). However, the increased concentration allowed stable isotope fractionation, which would not occur under formate limitation. The δ13C of the produced acetate was by about 24 ‰–33 ‰ lower than that of formate. This isotopic discrimination between formate and acetate is similar to that measured in a culture of the homoacetogen Thermoanaerobacter kivui (Freude and Blaser, 2016). However, this discrimination is much larger than the isotopic enrichment factors (εform of −8 ‰ to −2.5 ‰) determined from the change in δ13C during formate consumption. There are two conceivable explanations for this observation. (1) Formate is disproportionated to CO2 and acetate. In the WLP, three formate are oxidized to CO2, one formate is reduced to the methyl group of acetate and one of the produced CO2 is reduced to the carboxyl group of acetate. The disproportionation of formate to acetate and 2CO2 is possibly a branch point (Fry, 2003; Hayes, 2001), at which the carbon flow is split into the production of 13C-enriched CO2 and 13C-depleted acetate, which together result in the εform observed. (2) Formate first is completely converted to CO2 plus H2 (Reaction R5) or other electron equivalents. This reaction displays the εform determined by the Mariotti plots. Acetate is then produced via the WLP by the chemolithotrophic reduction of 2CO2 to acetate, of which the isotopic enrichment factor is typically on the order of about −55 ‰ (Blaser and Conrad, 2016). In any case, it is plausible to assume that acetate was formed via the WLP. In the WLP, oxidation of formate is catalyzed by a formate dehydrogenase, which provides CO2 to the carboxyl branch of the WLP. The methyl branch of the WLP normally starts with formate being converted to formyl-THF. However, it can also start with the reduction of CO2 to formate with a hydrogen-dependent carbon dioxide reductase (HDCD). Homoacetogens (e.g., Acetobacter woodii, T. kivui) contain such a HDCD, which allows the interconversion of formate and H2 plus CO2 (Jain et al., 2020; Schuchmann et al., 2018). The isotope discrimination in our experiments indicates that the CO2 produced from formate has been enriched in 13C rather than depleted, thus supporting the first explanation. The δ13C of CO2 produced from formate was initially lower than that of the unamended soil or sediment being on the order of −20 ‰ to −10 ‰ (Figs. 1h, 3h, S1h, S3h). Eventually, however, δ13C of CO2 reached values of −25 ‰ to −10 ‰ (Fig. 7). The δ13C of bicarbonate is 10 ‰ more positive than that of CO2. This mixed inorganic carbon would be the CO2 substrate for WLP, which together with formate generates the acetate having a δ13C of about −70 ‰ to −50 ‰ (Fig. 7).
Methane was a minor product of formate degradation in all soils and sediments. Since CH4 formation was strongly inhibited by CH3F, it was most likely produced from acetate by aceticlastic methanogens. Since CH4 production from the soils or sediments was much lower without formate amendment, the CH4 must have primarily been produced from the acetate that was generated from formate. The δ13C of CH4 in the soil incubations was more negative than that of acetate (Fig. 7). The difference between the δ13C of CH4 and the δ13C of acetate indicated an isotopic enrichment factor of ‰ to −8 ‰, which is close to the enrichment factor of aceticlastic Methanosaeta (Methanothrix) concilii (Penning et al., 2006). In the lake sediments, the δ13C of CH4 and acetate was not much different, indicating that acetate was instantaneously consumed by methanogens, as it was produced by homoacetogens so that carbon isotopes were not discriminated. Both paddy soils and lake sediments contained mcrA genes (coding for a subunit of methyl CoM reductase) of Methanosaetaceae (Methanotrichaceae) (Conrad et al., 2021).
4.2 Formate degradation under sulfidogenic conditions
In the rice paddy soils, formate was consumed within 10 d when sulfate was present and not quite as fast as without sulfate. In the lake sediments, however, sulfidogenic formate consumption was much slower. Formate degradation by sulfate reduction normally results in complete oxidation to CO2 (Reaction R3). In the lake sediments, CO2 was indeed the main degradation product. However, in the paddy soils, substantial amounts of acetate and even CH4 were also produced. The homoacetogenic bacteria in these soils apparently competed well with the sulfate reducing bacteria, although the soils had been adapted by preincubation in the presence of sulfate. The production of acetate and CH4 was dependent on formate degradation, since no production was observed in the unamended control. Production of CH4 was inhibited by CH3F, indicating that aceticlastic methanogenesis was the main process of CH4 production. The carbon isotope fractionation of formate was similar under non-sulfidogenic conditions, exhibiting a small εform of −8 ‰ to −3.5 ‰ (Fig. 5) and displaying a strong isotope effect with the formation of acetate (δ13C = −57 ‰ to −52 ‰) and CH4 (δ13C = −60 ‰ to −58 ‰). The mechanism of fractionation is probably the same (see above).
In the lake sediments, however, sulfidogenic degradation of formate was much slower than methanogenic/acetogenic degradation. In the sediment of the SW basin, formate was not even completely degraded within 80 d. In the sediments of both lake basins, neither acetate nor CH4 was a major product of sulfidogenic formate degradation. Hence, formate was apparently degraded according to Reaction (R3) forming CO2 as the main carbon product. This formation process displayed no depletion of the heavy carbon isotope, as the Mariotti plots of δ13C of formate did not exhibit a negative slope. The δ13C of the CO2 slowly decreased with an increasing fraction of formate consumed (Figs. 3h; 5c), probably involving isotope exchange between formate and CO2 (DeGraaf and Cappenberg, 1996). The little acetate which was formed displayed a δ13C of −77 ‰ (Fig. 7b), indicating that it was produced by a mechanism similar to the one found in the absence of sulfate, presumably via the WLP.
The strong differences between rice paddy soils and lake sediments were possibly caused by their different microbial communities (Conrad et al., 2021). The differences were seen in the composition of the mcrA and dsrB genes coding for methyl CoM reductase and dissimilatory sulfate reductase, respectively, as well as the gene coding for the bacterial 16S rRNA (data are shown in Conrad et al., 2021). The microbial community structures based on these genes were similar whether the soils and sediments were amended with sulfate or not. However, they were strongly different between soils or sediments were used (Conrad et al., 2021). Unfortunately, these data do not allow for the discrimination of particular taxa of homoacetogenic bacteria. Nevertheless, it is possible that formate-consuming homoacetogens were more prevalent in the soils than in the sediments and competed accordingly with the formate-consuming sulfate reducers, more or less.
4.3 Conclusions
Formate was found to be an excellent substrate for acetate formation in the paddy soils and in the lake sediments, confirming and extending similar observations in a fen soil (Hunger et al., 2011). In the anoxic soils, acetate was the major product even in the presence of sulfate, which would have allowed sulfate reduction. The acetate was strongly depleted in 13C relative to formate, but the consumption of formate itself displayed only a small isotopic enrichment factor. Therefore, it is likely that formate was disproportionated to 13C-depleted acetate and 13C-enriched CO2. The δ13C of CO2 was indeed slightly higher than that of formate. Acetate was most likely produced by homoacetogenesis via the WLP. The produced acetate was then used by aceticlastic methanogens (probably by Methanothrix) but only to a minor extent, resulting in further depletion of 13C. The homoacetogenic bacteria in the paddy soils apparently competed well with both methanogenic and sulfate-reducing microorganisms when formate was the substrate. The preference of homoacetogenesis as a degradation pathway is unexpected, since other substrates, such as acetate and propionate, are degraded in these paddy soils by methanogenesis or sulfate reduction (Conrad et al., 2021; Conrad and Claus, 2023). Only in the lake sediments was formate oxidation by sulfate reduction was more prevalent than homoacetogenesis.
The data are all contained in the figures and in the Supplement.
The supplement related to this article is available online at: https://doi.org/10.5194/bg-21-1161-2024-supplement.
RC designed the experiments, evaluated the data and wrote the manuscript. PC conducted the experiments.
The contact author has declared that neither of the authors has any competing interests.
Publisher’s note: Copernicus Publications remains neutral with regard to jurisdictional claims made in the text, published maps, institutional affiliations, or any other geographical representation in this paper. While Copernicus Publications makes every effort to include appropriate place names, the final responsibility lies with the authors.
This research has been supported by the Fonds der Chemischen Industrie (grant no. 163468).
The article processing charges for this open-access publication were covered by the Max Planck Society.
This paper was edited by Perran Cook and reviewed by two anonymous referees.
Blaser, M. and Conrad, R.: Stable carbon isotope fractionation as tracer of carbon cycling in anoxic soil ecosystems, Curr. Opin. Biotech., 41, 122–129, 2016.
Casper, P., Chan, O. C., Furtado, A. L. S., and Adams, D. D.: Methane in an acidic bog lake: The influence of peat in the catchment on the biogeochemistry of methane, Aquat. Sci., 65, 36–46, 2003.
Conrad, R.: Quantification of methanogenic pathways using stable carbon isotopic signatures: a review and a proposal, Org. Geochem., 36, 739–752, 2005.
Conrad, R.: Complexity of temperature dependence in methanogenic microbial environments, Front. Microbiol., 14, 1232946, https://doi.org/10.3389/fmicb.2023.1232946, 2023.
Conrad, R. and Claus, P.: Fractionation of stable carbon isotopes during microbial propionate consumption in anoxic rice paddy soils, Biogeosciences, 20, 3625–3635, https://doi.org/10.5194/bg-20-3625-2023, 2023.
Conrad, R., Bak, F., Seitz, H. J., Thebrath, B., Mayer, H. P., and Schütz, H.: Hydrogen turnover by psychrotrophic homoacetogenic and mesophilic methanogenic bacteria in anoxic paddy soil and lake sediment, FEMS Microbiol. Ecol., 62, 285–294, 1989.
Conrad, R., Klose, M., Claus, P., and Enrich-Prast, A.: Methanogenic pathway, 13C isotope fractionation, and archaeal community composition in the sediment of two clearwater lakes of Amazonia, Limnol. Oceanogr., 55, 689–702, 2010.
Conrad, R., Liu, P., and Claus, P.: Fractionation of stable carbon isotopes during acetate consumption by methanogenic and sulfidogenic microbial communities in rice paddy soils and lake sediments, Biogeosciences, 18, 6533–6546, https://doi.org/10.5194/bg-18-6533-2021, 2021.
DeGraaf, W. and Cappenberg, T. E.: Evidence for isotopic exchange during metabolism of stable- isotope-labeled formate in a methanogenic sediment, Appl. Environ. Microb., 62, 3535–3537, 1996.
Dolfing, J., Jiang, B., Henstra, A. M., Stams, A. J. M., and Plugge, C. M.: Syntrophic growth on formate: a new microbial niche in anoxic environments, Appl. Environ. Microb., 74, 6126–6131, 2008.
Dong, X. Z., Plugge, C. M., and Stams, A. J. M.: Anaerobic degradation of propionate by a mesophilic acetogenic bacterium in coculture and triculture with different methanogens, Appl. Environ. Microb., 60, 2834–2838, 1994.
Drake, H. L.: Acetogenesis, acetogenic bacteria, and the acetyl-CoA “Wood/Ljungdahl” pathway: past and current perspectives, in: Acetogenesis, edited by: Drake, H. L., Chapman & Hall, New York, 3–60, ISBN 0-412-03211-2, 1994.
Elsner, M., Zwank, L., Hunkeler, D., and Schwarzenbach, R. P.: A new concept linking observable stable isotope fractionation to transformation pathways of organic pollutants, Environ. Sci. Technol., 39, 6896–6916, 2005.
Freude, C. and Blaser, M.: Carbon sotope fractionation during catabolism and anabolism in acetogenic bacteria growing on different substrates, Appl. Environ. Microb., 82, 2728–2737, 2016.
Fry, B.: Steady state models of stable isotopic distributions, Isot. Environ. Healt. S., 39, 219–232, https://doi.org/10.1080/1025601031000108651, 2003.
Glombitza, C., Jaussi, M., Roy, H., Seidenkrantz, M. S., Lomstein, B. A., and Joergensen, B. B.: Formate, acetate, and propionate as substrates for sulfate reduction in sub-arctic sediments of southwest Greenland, Front. Microbiol., 6, 846, https://doi.org/10.3389/fmicb.2015.00846, 2015.
Goevert, D. and Conrad, R.: Effect of substrate concentration on carbon isotope fractionation during acetoclastic methanogenesis by Methanosarcina barkeri and M. acetivorans and in rice field soil, Appl. Environ. Microb., 75, 2605–2612, 2009.
Hausmann, B., Knorr, K. H., Schreck, K., Tringe, S. G., DelRio, T. G., Loy, A., and Pester, M.: Consortia of low-abundance bacteria drive sulfate reduction-dependent degradation of fermentation products in peat soil microcosms, ISME J., 10, 2365–2375, 2016.
Hayes, J. M.: Factors controlling 13C contents of sedimentary organic compounds: principles and evidence, Mar. Geol., 113, 111–125, 1993.
Hayes, J. M.: Fractionation of carbon and hydrogen isotopes in biosynthetic processes, Rev. Mineral. Geochem., 43, 225–277, https://doi.org/10.2138/gsrmg.43.1.225, 2001.
Hunger, S., Schmidt, O., Hilgarth, M., Horn, M. A., Kolb, S., Conrad, R., and Drake, H. L.: Competing formate- and carbon dioxide-utilizing prokaryotes in an anoxic methane-emitting fen soil, Appl. Environ. Microb., 77, 3773–3785, 2011.
Jain, S., Dietrich, H. M., Müller, V., and Basen, M.: Formate is required for growth of the thermophilic acetogenic bacterium Thermoanaerobacter kivui lacking hydrogen-dependent carbon dioxide reductase (HDCR), Front. Microbiol., 11, 59, https://doi.org/10.3389/fmicb.2020.00059, 2020.
Janssen, P. H. and Frenzel, P.: Inhibition of methanogenesis by methyl fluoride – studies of pure and defined mixed cultures of anaerobic bacteria and archaea, Appl. Environ. Microb., 63, 4552–4557, 1997.
Kanaparthi, D., Pommerenke, B., Casper, P., and Dumont, M. G.: Chemolithotrophic nitrate-dependent Fe(II)-oxidizing nature of actinobacterial subdivision lineage TM3, ISME J., 7, 1582–1594, 2013.
Kim, Y. J., Lee, H. S., Kim, E. S., Bae, S. S., Lim, J. K., Matsumi, R., Lebedinsky, A. V., Sokolova, T. G., Kozhevnikova, D. A., Cha, S. S., Kim, S. J., Kwon, K. K., Imanaka, T., Atomi, H., Bonch-Osmolovskaya, E. A., Lee, J. H., and Kang, S. G.: Formate-driven growth coupled with H2 production, Nature, 467, 352–355, 2010.
Kotsyurbenko, O. R., Nozhevnikova, A. N., Soloviova, T. I., and Zavarzin, G. A.: Methanogenesis at low temperatures by microflora of tundra wetland soil, Ant. Leeuwenhoek, 69, 75–86, 1996.
Küsel, K. and Drake, H. L.: Microbial turnover of low molecular weight organic acids during leaf litter decomposition, Soil Biol. Biochem., 31, 107–118, 1999.
Lemaire, O. N., Jespersen, M., and Wagner, T.: CO2-fixation strategies in energy extremophiles: What can we learn from acetogens?, Front. Microbiol., 11, 486, https://doi.org/10.3389/fmicb.2020.00486, 2020.
Liebner, S., Schwarzenbach, S. P., and Zeyer, J.: Methane emissions from an alpine fen in central Switzerland, Biogeochemistry, 109, 287–299, 2012.
Liu, P. F., Klose, M., and Conrad, R.: Temperature effects on structure and function of the methanogenic microbial communities in two paddy soils and one desert soil, Soil Biol. Biochem., 124, 236–244, 2018.
Lovley, D. R. and Klug, M. J.: Intermediary metabolism of organic matter in the sediments of a eutrophic lake, Appl. Environ. Microb., 43, 552–560, 1982.
Mariotti, A., Germon, J. C., Hubert, P., Kaiser, P., Letolle, R., Tardieux, A., and Tardieux, P.: Experimental determination of nitrogen kinetic isotope fractionation: some principles; illustration for the denitrification and nitrification processes, Plant Soil, 62, 413–430, 1981.
Martins, M., Mourato, C., and Pereira, I. A.: Desulfovibrio vulgaris growth coupled to formate-driven H2 production, Environ. Sci. Technol., 49, 14655–14662, 2015.
Montag, D. and Schink, B.: Formate and hydrogen as electron shuttles in terminal fermentations in an oligotrophic freshwater lake sediment, Appl. Environ. Microb., 84, e01572-18, https://doi.org/10.1128/AEM.01572-18, 2018.
Nozhevnikova, A. N., Kotsyurbenko, O. R., and Simankova, M. V.: Acetogenesis at low temperature, in: Acetogenesis, edited by: Drake, H. L., Chapman & Hall, New York, 416–431, ISBN 0-412-03211-2, 1994.
Penning, H., Claus, P., Casper, P., and Conrad, R.: Carbon isotope fractionation during acetoclastic methanogenesis by Methanosaeta concilii in culture and a lake sediment, Appl. Environ. Microb., 72, 5648–5652, 2006.
Peters, V., Janssen, P. H., and Conrad, R.: Efficiency of hydrogen utilization during unitrophic and mixotrophic growth of Acetobacterium woodii on hydrogen and lactate in the chemostat, FEMS Microbiol. Ecol., 26, 317–324, 1998.
Peters, V., Janssen, P. H., and Conrad, R.: Transient production of formate during chemolithotrophic growth of anaerobic microorganisms on hydrogen, Curr. Microbiol., 38, 285–289, 1999.
Phelps, T. J. and Zeikus, J. G.: Effect of fall turnover on terminal carbon metabolism in Lake Mendota sediments, Appl. Environ. Microb., 50, 1285–1291, 1985.
Rothfuss, F. and Conrad, R.: Vertical profiles of CH4 concentrations, dissolved substrates and processes involved in CH4 production in a flooded Italian rice field, Biogeochemistry, 18, 137–152, 1993.
Schink, B., Montag, D., Keller, A., and Müller, N.: Hydrogen or formate: Alternative key players in methanogenic degradation, Env. Microbiol. Rep., 9, 189–202, 2017.
Schuchmann, K. and Müller, V.: Direct and reversible hydrogenation of CO2 to formate by a bacterial carbon dioxide reductase, Science, 342, 1382–1385, 2013.
Schuchmann, K., Chowdhury, N. P., and Müller, V.: Complex multimeric [FeFe] hydrogenases: biochemistry, physiology and new opportunities for the hydrogen economy [review], Front. Microbiol., 9, 2911, https://doi.org/10.3389/fmicb.2018.02911, 2018.
Sieber, J. R., Le, H. M., and McInerney, M. J.: The importance of hydrogen and formate transfer for syntrophic fatty, aromatic and alicyclic metabolism, Environ. Microbiol., 16, 177–188, 2014.
Stumm, W. and Morgan, J. J.: Aquatic Chemistry, 3rd Edn., Wiley, New York, ISBN 978-0-471-51185-4, 1996.
Thauer, R. K., Jungermann, K., and Decker, K.: Energy conservation in chemotrophic anaerobic bacteria, Bacteriol. Rev., 41, 100–180, 1977.
Widdel, F.: Microbiology and ecology of sulfate- and sulfur-reducing bacteria, in: Biology of Anaerobic Microorganisms, edited by: Zehnder, A. J. B., Wiley, New York, 469–585, ISBN 0-471-88226-7, 1988.
Wüst, P. K., Horn, M. A., and Drake, H. L.: Trophic links between fermenters and methanogens in a moderately acidic fen soil, Environ. Microbiol., 11, 1395–1409, 2009.
Zinder, S. H.: Physiological ecology of methanogens, in: Methanogenesis. Ecology, Physiology, Biochemistry and Genetics, edited by: Ferry, J. G., Chapman & Hall, New York, 128–206, ISBN 978-1-4613-6013-1, 1993.