the Creative Commons Attribution 4.0 License.
the Creative Commons Attribution 4.0 License.
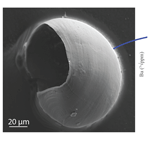
A step towards measuring connectivity in the deep sea: elemental fingerprints of mollusk larval shells discriminate hydrothermal vent sites
Christophe Pecheyran
Fanny Claverie
Cécile Cathalot
Marjolaine Matabos
Yoan Germain
Olivier Rouxel
Didier Jollivet
Thomas Broquet
Thierry Comtet
Deep-sea hydrothermal vent systems are under investigation for base and precious metal exploitations. The impact of mining will depend critically on the ability of larval dispersal to connect and replenish endemic populations. However, assessing connectivity is extremely challenging, especially in the deep sea. Here, we investigate the potential of elemental fingerprinting of mollusk larval shells to discriminate larval origins between multiple hydrothermal sites in the southwest Pacific Ocean. The gastropod Shinkailepas tollmanni represents a suitable candidate as it uses capsules to hold larvae before dispersal, which facilitates sampling and ensures mineralization occurs on the site of origin. Multielemental microchemistry was performed using cutting-edge femtosecond laser ablation inductively coupled plasma mass spectrometry analysis to obtain individual measurements on 600 encapsulated larval shells. We used classification methods to discriminate the origin of individuals from 14 hydrothermal sites spanning over 3500 km, with an overall success rate of 70 %. When considering fewer sites within more restricted areas, reflecting dispersal distances reported by genetic and modeling approaches, the success rate increased up to 86 %. We conclude that individual larval shells register site-specific elemental signatures that can be used to assess their origin. These results open new perspectives to get direct estimates on population connectivity from the geochemistry of pre-dispersal shells of recently settled juveniles.
- Article
(4119 KB) - Full-text XML
-
Supplement
(1637 KB) - BibTeX
- EndNote
Deep-sea hydrothermal activity produces massive polymetallic sulfide deposits from the ascent and precipitation of hydrothermal fluids enriched in metals originating from the oceanic crust (Fouquet et al., 1991; Binns and Scott, 1993; Humphris et al., 1995; Hannington et al., 2011). Vent sites are economically interesting to mining companies (Hoagland et al., 2010; Petersen et al., 2016; Radziejewska et al., 2022), whose initiatives are currently under evaluation worldwide (Boschen et al., 2013; Dunn et al., 2018). Deep-sea mining is expected to strongly disturb hydrothermal vent ecosystems (Van Dover, 2014; Danovaro et al., 2017, 2020), yet very little is known about the resilience of their associated communities (Dunn et al., 2018; Suzuki et al., 2018).
Hydrothermal vent fields are ephemeral and patchy habitats, separated by hundreds of meters to thousands of kilometers. The resilience of vent populations therefore depends critically on their level of connectivity, i.e., the migration of individuals between vent sites. As a basic process of population dynamics, connectivity is key to understanding the persistence of vent communities as well as the potential of vent species to colonize new areas and habitats (e.g., Adams et al., 2012; Levin et al., 2016). As such, it is of major interest for biodiversity conservation and the design of deep-sea marine protected areas (Combes et al., 2021), for example in the framework of the 30 × 30 initiative, which aims to protect at least 30 % of the world's oceans by 2030 (O'Leary et al., 2019). In the particular context of deep-sea mining, connectivity has been identified as a key scientific knowledge gap (Gollner et al., 2017; Miller et al., 2018; Smith et al., 2020; Amon et al., 2022) that should be tackled taking into account its interactions with climate change (Levin et al., 2020). For vent benthic species, pelagic larvae are the major, if not the only, vector of dispersal between distant and well-separated populations living at active sites and a means to colonize new territories. Accurately estimating larval dispersal is therefore critical to understand the demographic trajectory of hydrothermal metapopulations, especially in the context of habitat destruction (Amon et al., 2022). It is however extremely difficult to catch minute-sized larvae released at great depths (although some successful attempts have been reported: Comtet et al., 2000; Mullineaux et al., 2005), and it is almost impossible to directly track their journey between their initial release and final settlement locations.
Indirect methods exist to assess connectivity but have some limitations. Larval dispersal modeling aims at estimating the spatial extent of larval transport in a given area (Mitarai et al., 2016; Vic et al., 2018; Yearsley et al., 2020). It uses physical oceanographic data and species-specific biological features, such as the planktonic larval duration and larval behavior (e.g., vertical migration), but biological and hydrodynamic parameters are hardly constrained, inducing uncertainties in the larval traveling distance (McVeigh et al., 2017). Other indirect methods based on genetic data (Baco et al., 2016; Breusing et al., 2016; Tran Lu Y et al., 2022) are often inconclusive in broadcast-spawning species because large and very fertile populations experience weak genetic drift, resulting in low population differences regardless of migration rates (Gagnaire et al., 2015). The genetic approach can use more direct methods, e.g., based on parentage analyses, but these are applicable only when a significant fraction of individuals is sampled (Gagnaire et al., 2015), which is unworkable in the deep sea.
Although used for many years to assess directly larval dispersal in coastal environments (Levin, 1990; Levin et al., 1993; Zacherl et al., 2003; Becker et al., 2005, 2007; Carson, 2010; Fodrie et al., 2011; Kroll et al., 2016; Honig et al., 2020; Bounket et al., 2021), elemental fingerprinting of biogenic carbonate structures (such as otoliths or shells) has never been applied to vent species (Mullineaux et al., 2018; Cunha et al., 2020), even though its interest for deep-sea environments has been suggested over 15 years ago (Levin, 2006). This approach relies on the record in calcified structures of the chemical composition of the water in which they have formed (Thorrold et al., 2007). When spatial differences occur in seawater composition, these can be imprinted in the biominerals of organisms living there, with potential modifications due to environmental conditions (e.g., temperature; Thorrold et al., 2007) and animal metabolism (Mouchi et al., 2020). In fish and mollusks, calcified structures produced during the early larval stage and preserved thereafter can be used to infer their natal geographic origin, provided that they have been built in chemically contrasting sites (Becker et al., 2007; Thorrold et al., 2007). This approach sounds promising in hydrothermal systems for which different chemical signatures are expected at various spatial scales (Hannington et al., 1991; Le Bris et al., 2003; Toffolo et al., 2020). Besides, newly developed cutting-edge analytical methods now allow precise geochemical measurements on individual 100 µm size larval shells. Assigning the origin of juveniles from elemental fingerprints will provide rare evidence of single-generation connectivity ranges and identify which sites correspond to sources and sinks for populations over a wide area. This type of information can subsequently be used to identify sites of higher priority for preservation and protection from disturbance to ensure the resilience of populations (e.g., no-mining areas known as Areas of Particular Environmental Interest, or APEIs; International Seabed Authority, 2011; Dunn et al., 2018; Preservation Reference Zones and Impact Reference Zones; International Seabed Authority, 2019). Moreover, identifying source and sink populations and migration rates for many species would therefore help identify the species vulnerable to disturbance or even destruction of a specific habitat.
The first step towards using elemental fingerprinting to estimate connectivity in the deep sea is to determine whether the elemental composition of larval shells records a distinct chemical signature between hydrothermal vent populations. Here, we define the elemental fingerprints of shells of encapsulated larvae of the gastropod Shinkailepas tollmanni (Beck, 1992) at multiple hydrothermal sites over 3500 km in the southwest Pacific (Fig. 1). Shinkailepas tollmanni (Fig. 2) is an abundant vent limpet which lays eggs in capsules deposited on the shell of the larger symbiotic gastropod Ifremeria nautilei (Bouchet and Warén, 1991) living in diffuse venting areas. Within these capsules, eggs develop into veliger larvae whose carbonate shells incorporate elements from the surrounding habitat water before dispersing. The challenge is to get high-quality geochemical measurements from the 100 µm size encapsulated larval shells (Fig. 2f) to highlight the potential of this approach to discriminate sites of origin. To explore the use of elemental fingerprints of larval shells in this species, several questions were investigated. Are there enough chemical contrasts between hydrothermal vent sites for elemental fingerprinting? Do elemental fingerprints of larval shells reflect their natal place? What are the main elements responsible for the elemental fingerprint of larval shells? At which spatial scale is elemental fingerprinting more accurate? Does the elemental fingerprint of larval shells correspond to that of habitat water, in the sense that the habitat water fingerprint could be used as a reference for the shell fingerprint?
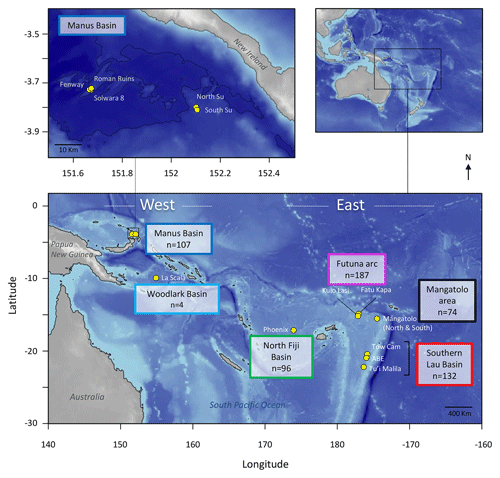
Figure 1Geographic map of the 14 sampling locations in the southwest Pacific. The number of encapsulated larvae is indicated for each area. Although the newly discovered Mangatolo site is located in the northern part of the Lau Basin, we considered it as a separate area due to its greater distance from the other Lau sites. Maps generated using the marmap package for R (Pante and Simon-Bouhet, 2013) and bathymetry data from the NOAA ETOPO1 database (Amante and Eakins, 2009).
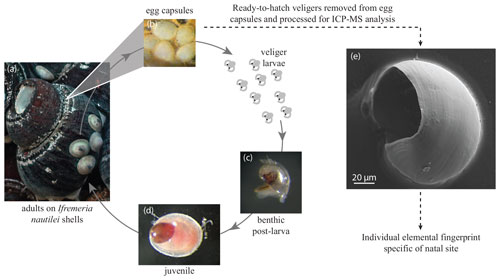
Figure 2Life cycle of Shinkailepas tollmanni. Ifremeria nautilei shell grooves (a) serve as a depository of S. tollmanni egg capsules (b) housing encapsulated larvae. When the capsules open, the shelled larvae disperse in seawater until metamorphosis when the individual reaches a hydrothermal site (settlement; c). The new recruit grows a juvenile shell while preserving its larval shell (d). A scanning electron microscope picture of an encapsulated larval shell (corresponding to the protoconch I; e) is presented (secondary electron mode, 15 kV).
2.1 Shinkailepas tollmanni
Shinkailepas tollmanni (Beck, 1992), first described from the Manus basin (Beck, 1992), is an abundant phenacolepadid gastropod widely distributed in the western Pacific back-arc basins, covering depths ranging from 1300 to 3300 m (Sasaki et al., 2010; Collins et al., 2012; Boulart et al., 2022; Poitrimol, 2022). Adults live in the three faunal assemblages common to these areas, i.e., the Bathymodiolus spp., Ifremeria nautilei, and Alviniconcha spp. communities (Podowski et al., 2009; Collins et al., 2012; Poitrimol, 2022), with higher abundances on shells of I. nautilei (Poitrimol, 2022). As earlier hypothesized by Beck (1992), S. tollmanni likely grazes on free-living bacteria or organic matter from vent surfaces and mollusk shells it lives on (Van Audenhaege et al., 2019; Suh et al., 2022). Shinkailepas tollmanni is gonochoric with continuous gametogenesis (Poitrimol et al., 2024), and fertilization is internal (Sasaki et al., 2010). Females lay egg capsules on shells of living Ifremeria nautilei as first hypothesized by Beck (1992), up to the veliger stage 170–180 µm length (Yahagi et al., 2020). Whether the relationship between S. tollmanni and I. nautilei for egg-laying is obligate is unknown: to our knowledge the presence of egg capsules of S. tollmanni on Bathymodiolus or Alviniconcha shells, or on vent surfaces, has never been reported, but Yahagi et al. (2020) reported egg capsules on conspecific adult shells. Based on morphological characteristics (e.g., size of encapsulated veligers vs. size of postlarvae), larvae of S. tollmanni are considered planktotrophic and are assumed to disperse for up to 1 year (Yahagi et al., 2020). Although no direct evidence exists, larvae of S. tollmanni are assumed to disperse in surface waters as shown for other Phenacolepadidae (Yahagi et al., 2020). Such dispersal abilities could explain the genetic homogeneity observed among populations of S. tollmanni at the scale of the study area (Yahagi et al., 2020; Poitrimol et al., 2022). Recruitment is likely continuous, although episodic larval supply could also occur (Poitrimol et al., 2024).
2.2 Biological sampling
Egg capsules of S. tollmanni were sampled from the apex and spire crests of the shell of large symbiotic gastropods (Ifremeria nautilei) that were collected during the CHUBACARC oceanographic cruise (Hourdez and Jollivet, 2019) on board the research vessel L'Atalante in spring 2019. Site coordinates are indicated in Sect. S1 in the Supplement. Individuals of I. nautilei were collected using the hydraulic claw of the remotely operated vehicle (ROV) Victor 6000 and brought back to the surface in an insulated basket (this corresponds to one biobox or sampling replicate). On board, their shells were examined for egg capsules containing living shelled embryos of S. tollmanni using a dissecting microscope. To this extent, egg capsules were kept submerged using the local deep-sea water contained in bioboxes before opening, and larvae that had reached the veliger stage (swimming larvae with visible shell) were recovered with a Pasteur pipette. The presence of a calcified shell was confirmed after examining the collected larvae under polarized light. All the plastic material was washed with nitric acid and rinsed with pure water before use. After collection, the larvae were stored dry at −20 ∘C in groups of 10 to 100 in 2 mL microtubes until processed according to the protocol described below.
2.3 Habitat water composition analysis
Habitat water surrounding the Ifremeria communities was sampled in one point using the in situ fluid sampler (PIF) manipulated by the ROV arm at each surveyed vent site during the cruise prior to animal collection. Note that not all sites investigated for water composition in the Ifremeria communities comprised S. tollmanni individuals. As described in previous studies (Cotte et al., 2015), such in situ samplers allow us to recover low-temperature fluids with minimal metal contamination due to the use of metal-free materials and to perform if needed in situ filtration. Here, habitat water samples were filtered during sampling by mounting on-line Acrodisc® syringe filters incorporating a 0.22 µm Supor® hydrophilic polyethersulfone membrane. Samples were then acidified to pH 1.8 with ultrapure HCl and stored in LDPE bottles. Compositions of both major and minor elements were measured by high-resolution inductively coupled plasma mass spectrometry (HR-ICPMS) Element XR operated at Ifremer following previously described methods (Rouxel et al., 2018; Konn et al., 2022). In short, isotopes 44Ca, 39K, 24Mg, 32S, 28Si, 56Fe, 55Mn, 63Cu, 66Zn, 27Al, 51V, and 31P are measured in medium-mass-resolution mode, while isotopes 7Li, 11B, 85Rb, 88Sr, 89Y, 98Mo, and 238U are measured in low-mass-resolution mode. Analyses were done on aliquots, diluted 100-fold with 0.28 M HNO3 containing an internal spike of In (at 2 ng g−1 each). Solutions were introduced into the plasma torch using a quartz spray chamber system equipped with a microconcentric PFA nebulizer operating at a flow rate of about 100 µL min−1. Precision and accuracy were determined for each analytical run by repeat analysis of an internal reference material of similar range in composition as the samples (trace-metal-doped seawater) also spiked with In (2 ng g−1). For each element, ICPMS sensitivity was calibrated using a set of matrix-matched in-house standard solutions corresponding to seawater matrices and IAPSO Standard Seawater. Precision was better than 5 % (2 SD) for reported elements. Detection limit was determined as 3 standard deviations of the repeated reagent blank's signal processed through the same protocol as for unknown samples.
2.4 Larval shell preparation
Details of the shell preparation protocol are described in Mouchi et al. (2023a). Briefly, all plastic materials handled during the cleaning and preparation of the samples were acid-cleaned using 10 % Primar Plus Trace analysis grade HNO3 (Fisher Chemicals, 10098862) and rinsed with ultrapure water in Teflon beakers in a clean lab under a laminar flow cabinet (ISO5). No metallic objects were used in contact of the samples at any stage of the sample cleaning. A protocol modified from Becker et al. (2005) was used to remove the soft body from the shells. A solution of Optima-grade H2O2 at 30 % (Merck KGaA, 107298) was buffered with 0.05 mol L−1 NaOH (Suprapur) to obtain a pH of 8.5. The final H2O2 concentration was approximately 15 %. This solution was used to digest the larval tissues in a glass container overnight. The resulting cleaned shells were then rinsed with ultrapure water and collected by a sable brush to place them on Extra Pure carbon adhesive tabs (Science Services) on a microscope slide. After the remaining ultrapure water dried out on the tabs, the slides were placed in a clean airtight plastic box until analysis.
2.5 Individual larval shell elemental analysis
The elemental composition of cleaned larval shells was measured at the Institut des Sciences Analytiques et de Physico-Chimie pour l'Environnement et les Matériaux (Université de Pau et des Pays de l'Adour), with an Agilent 8900 ICP-MS Triple Quad coupled with a femtosecond laser ablation system (Novalase SA). The femtosecond (360 fs) laser ablation system was set to generate pulses of 23 µJ at 50 Hz. It is equipped with a 2D galvanometric scanner which allows the laser beam to be moved at the surface of the sample at high speed. Each shell was ablated following the trajectory of a scanned disk of 100 µm diameter (with 7.5 µm step) at 1 mm s−1 speed rate. For each acquisition, the disk was performed twice to ensure the total ablation of the larval shell while preventing excessive ablation of the tape. Some zones of the tape without sample were also ablated separately in the same conditions in order to check for potential contamination. No evidence of critical signal was observed on this tape for the elements of interest. Helium, at a 450 mL min−1 flow rate, was chosen to transport the ablated particles to the ICPMS. The ICPMS was operated in MS/MS mode with 10 mL min−1 of H2. Measured elements were 24Mg, 52Cr, 55Mn, 56Fe, 63Cu, 66Zn, 75As, 88Sr, 114Cd, 120Sn, 121Sb, 138Ba, 208Pb, and 43Ca as the internal standard to control the ablated volume. Dwell time was 0.005 s for each element. Since each sample represented an extremely small amount of material, on the order of micrograms, the complete ablation of the sample took place in only a few seconds (Sect. S2). Calibration was performed by the successive measurements of the reference glass materials NIST SRM 610, 612, and 614 at the beginning, the middle, and the end of each analytical session using 43Ca as the internal standard. Data reduction was performed using the in-lab-developed software FOCAL 2.41. Accuracy of the measurements was checked by measuring the otolith certified reference materials (CRMs) FEBS-1 and NIES-22 against the preferred values from the GeoReM database (Jochum et al., 2005), when available.
2.6 Data processing
All data were processed using the MATLAB software (MathWorks v. R2022a, https://www.mathworks.com (last access: January 2023)). Correlation between habitat water and larval shell compositions was assessed using the Pearson determination coefficient r2 and the Spearman correlation coefficient rho. Principal component analysis (PCA) was performed to explore the variance of habitat water and larval shell compositions according to hydrothermal sites. For PCA, habitat water and larval shell data were transformed to the cubic root (Chen and Deo, 2004) to improve data dispersion and interpretation and were normalized. Larval shell measurements were only transformed to the cubic root for classifications. Classification models (for site and area discrimination based on larval shell geochemistry; Sect. S3) were performed using the MATLAB “ClassificationLearner” application and five equal folds (i.e., disjoint divisions) of the dataset for cross-validation: each fold was successively used as a validation fold to assess the model based on the remaining four folds, and a mean classification rate was calculated from the five validation folds. Although linear discriminant analysis has been widely used as the preferred classification method for assessing connectivity from elemental fingerprints in coastal environments (Becker et al., 2005; Gomes et al., 2016), alternative, more accurate and successful methods have been proposed (Mercier et al., 2011; Dixon and Brereton, 2009). We here applied 31 classification methods (available in “ClassificationLearner”) on the chemistry of encapsulated larval shells in order to identify the best-fitting model (obtained from one classification method and a specific list of predictors) to determine their geographical origin. For each dataset (all sites, sites in Manus and Woodlark, sites in the east region and Woodlark, and areas in the east region and Woodlark), we successively performed all models with different numbers and combinations of elements. Model significance was assessed by comparing its classification success rate with that of randomized data (1000 runs) using a modified version of the MATLAB code (Sect. S4) by White and Ruttenberg (2007), which was initially restricted to the discriminant analysis.
3.1 Are there enough chemical contrasts between hydrothermal vent sites for elemental fingerprinting?
Shinkailepas tollmanni egg capsules are in contact with the mixture between seawater and hydrothermal fluid associated with the habitat of Ifremeria nautilei in proportions that vary depending on the vent activity, local currents, and turbulence (Podowski et al., 2010). This mixture will hereafter be referred to as the “habitat water”. Because habitat water likely influences the composition of biogenic carbonates, we first investigated the potential differences in the elemental composition of the habitat water surrounding Ifremeria communities, which may be defined by the geological nature of the underlying substrate and by phase separation processes (Hannington et al., 2013) leading to the extensive precipitation of metal sulfide and oxide minerals. Distinct water compositions will be an indication that larval shells formed in the capsules may record potentially useful differences in their elemental composition.
Habitat water elemental composition differed among only some of the sampled sites (Fig. 3a and b and Sect. S5), as shown by a principal component analysis (PCA) conducted on 40 samples with 19 elements. Amongst the most differentiated sites, the Mangatolo Triple Junction is well separated from the other sites (Fig. 3a), together with two nearby chimneys at the sites Aster'X and Stéphanie sampled in the field of Fatu Kapa (Futuna). Habitat water compositions were however overlapping for the other sites despite the great geographic distances separating them, especially in the western region (Manus and Woodlark basins; Fig. 3a). These results indicated that the chemistry of the habitat water was variable enough to distinguish some sites, depending on the geographic scale considered. The next question was thus to assess to what extent encapsulated shells record these slight differences and at what scale.
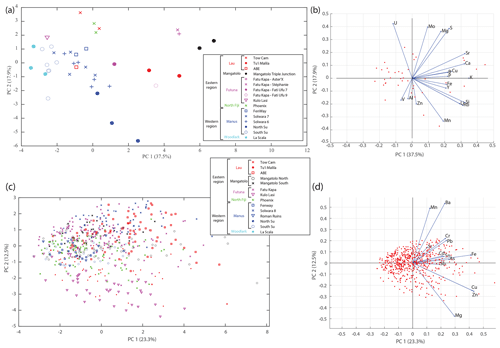
Figure 3Principal component analysis of Ifremeria habitat water compositions (a, b) and Shinkailepas tollmanni larval shell compositions (c, d) between sites, showing principal components one and two. The legend gathers all sites by area (Lau, Mangatolo, Futuna, northern Fiji, Manus, and Woodlark) and by region (east and west). The variance explained by each principal component is given in parentheses (55.4 % and 35.8 % on the first two axes for habitat water and S. tollmanni larval shells, respectively).
We therefore analyzed a total of 600 encapsulated larval shells of S. tollmanni collected from 14 vent sites in the southwest Pacific. The femtosecond laser ablation system coupled ICPMS–MS (8900 in semiconductor configuration) allowed us to measure 13 elements (Ca excluded) from each encapsulated larval shell despite their minute size (100 µm empty spheres, approx. 2 µm thickness; Fig. 2e). Measured abundances (Sect. S1) ranged from 0.5 ng g−1 (for Sb) to 10.2×103 µg g−1 (for Mg).
Considering the abundance variations in each element (Sect. S6), no single element or pair of elements is discriminant, although some substantial differences exist, enabling particular sites to be identified. This was particularly visible for Kulo Lasi (a volcanic caldera) where larval shells are strongly depleted in Mn. Some elements, namely Mg, Fe, Zn, Ba, and Pb, displayed a clear site-to-site variation, but values systematically overlapped at several sites, even across distant areas. Other elements had low geographic power. The abundance homogeneity of Cr, As, Cd, and Sb across sites was probably due to their extremely low values in shells (generally in the range 1–10 µg g−1; Sect. S1). Copper displayed slightly higher and more variable abundances, between 0.7 and 643.5 µg g−1 (Sect. S1).
Contrary to the habitat water data presented above, a simple PCA from the larval shell composition showed no obvious geographic clustering (Fig. 3c and d). Still, some geographic signal was visible on PC2, which appeared to reflect Mn and Ba in particular. Shells from Kulo Lasi and Tu'i Malila generally exhibit lower abundances of these elements compared to those of the other sites. Thus, in a second step we turned to more powerful, discriminant approaches to assess whether there is enough signal in the data to assign the origin of individual shells. That approach was possible due to the significant number of measurements from each site, which was not the case for habitat waters.
3.2 Do elemental fingerprints of larval shells reflect their natal place?
Identification of the site of origin of individual larvae was possible with a 70.0 % mean accuracy (Fig. 4), based on classification methods using Mg, Mn, Fe, Zn, Sr, Ba, and Pb as predictors (Sect. S3). For comparison, the success rate by random assignment was 20.1 % using randomized origins (p<0.001). This success rate appeared to be similar to or better than those obtained from models of coastal environments at such a scale (Becker et al., 2005; Simmonds et al., 2014; Gomes et al., 2016). With this model (i.e., results of a classification, represented by a confusion matrix as illustrated in Fig. 4), correct individual assignment ranged from 25.0 % for La Scala (Woodlark) to 93.5 % for Kulo Lasi (Futuna). Looking at these two extremes, the weak assignment success to La Scala may be due to the small number of specimens sampled at this location (n=4), whereas the high assignment success to Kulo Lasi is mainly due to the low abundance of Mn in larval shells (Sect. S6), in compliance with the very low concentration of this element at this site (Sect. S5). Phoenix (north Fiji) displayed the most heterogeneous larval shell composition, as illustrated by the wide distribution of values for each element in shells from this location compared to the others (see boxplots in Sect. S6). As a consequence, numerous larvae from other sites were wrongly assigned to this location, and a third of larvae originating from this site were misassigned to other locations by the model (69.8 % accuracy for Phoenix). Caution must therefore be taken when assigning a larva to Phoenix.
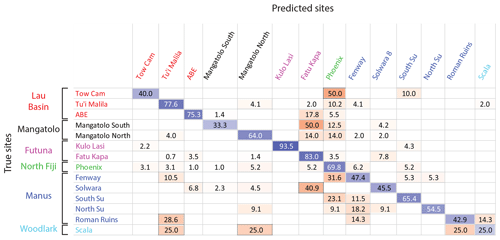
Figure 4Confusion matrix of the classification of Shinkailepas tollmanni encapsulated larvae to vent sites based on shell geochemistry. For each line (corresponding to one site), classification rates are indicated, with correct classification rates on the diagonal in blue and incorrect classification rates outside the diagonal in red. The intensity of these colors is directly correlated to the classification rate (correct or incorrect). This classification was made using quadratic support vector machines, reaching an overall 70.0 % accuracy.
However, a significant improvement in the classification was obtained when focusing on smaller geographic scales, which is justified when considering additional information on population connectivity based on other methods, such as larval transport modeling or population genetics. In particular, simulations of larval transport have suggested that Manus and the eastern (Lau, Fiji, Futuna) basins are not directly connected by larval dispersal and that Woodlark acts as a sink area for both regions (Mitarai et al., 2016). Population genetics reported partially congruent conclusions: migration was found to be strongly limited between these two regions for several gastropod species with a pelagic larval phase (Tran Lu Y et al., 2022; Plouviez et al., 2019; Breusing et al., 2023), although Woodlark could act as a “stepping stone” allowing limited connectivity (Boulart et al., 2022; Poitrimol et al., 2022). For S. tollmanni, although no population differentiation has been observed between these regions based on the mitochondrial genome (Poitrimol et al., 2022; Yahagi et al., 2020), genome-wide data obtained from RAD sequencing recently identified a strong genetic break indicating a lack of dispersal between Manus and the eastern region (Tran Lu Y, 2022). The Woodlark ridge was however a recipient for migrants from the two regions, representing a tension zone (Tran Lu Y, 2022, pp. 87–89). Based on this information, we split our data in two datasets, comprising sites of the western region (Manus and Woodlark) on the one hand and the eastern region plus Woodlark on the other hand (considering that a larva settling in the eastern region could originate from Woodlark but not from Manus). Within the western region, the overall accuracy reached 86.5 %, using Mn and Ba as predictors (Fig. 5a) compared to 26.1 % by random assignment (p<0.001). Site accuracy ranged from 54.5 % for north Su to 100 % for Solwara 8. Similarly, the best-fitting model for the eastern sites (including La Scala in the Woodlark Basin) showed an overall accuracy of 77.1 % with Mg, Mn, Fe, Zn, Sr, Ba, and Pb as predictors (Fig. 5b) compared to 23.7 % by chance (p<0.001). Another model using the same dataset, method, and predictors but focusing on areas instead of sites (Fig. 5c) obtained a slightly lower accuracy (75.3 % vs. 35.3 % by chance; p<0.001). The site model presents better accuracy due to the strong difference in Mn abundance between the sites from Futuna (Kulo Lasi and Fatu Kapa) that are considered one single class by the area model.
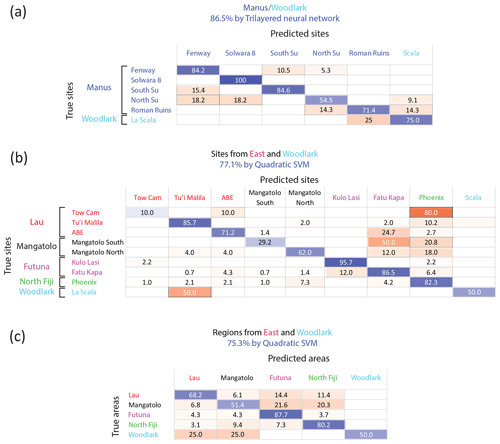
Figure 5Confusion matrices of the classification of Shinkailepas tollmanni encapsulated larvae based on shell geochemistry at the regional scale. (a) Using Mn and Ba as predictors for sites sampled in the Manus and Woodlark areas only (western region). (b) Using Mg, Mn, Fe, Zn, Sr, Ba, and Pb as predictors for sites from Woodlark, north Fiji, Futuna, Lau, and Mangatolo areas (eastern region and Woodlark). (c) Using Mg, Mn, Fe, Zn, Sr, Ba, and Pb as predictors for all areas except Manus (eastern region and Woodlark). For each line, corresponding to one site (a, c) or one area (b), classification rates are indicated, with correct classification rates on the diagonal in blue and incorrect classification rates outside the diagonal in red. The intensity of these colors is directly correlated to the classification rate (correct or incorrect).
In the future, if additional alternative information points to dispersal restricted within a single area in the eastern region, the assignment success to the site of origin for each area would reach 97.7 %, 98.6 %, and 100 % for Lau Basin, Mangatolo, and Futuna volcanic arc, respectively (Fig. 6), while random assignment would be correct for 53.7 % (p<0.001), 66.8 % (p<0.001), and 75.4 % (p<0.001) of the larvae, respectively. This would be particularly interesting as genomic data failed to provide good individual assignments at such small spatial scales because of both the size of the populations and their level of exchange (Tran Lu Y et al., 2022).
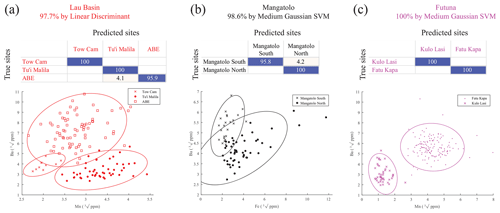
Figure 6Confusion matrices of the classification of Shinkailepas tollmanni encapsulated larvae based on shell geochemistry between sites for each eastern area and scatter plots of corresponding datasets (a Lau; b Mangatolo; c Futuna volcanic arc). For each line of the matrices (corresponding to one site), percentages of correct classifications are indicated in blue and incorrect classifications in red. For each matrix, the precision and type of model are indicated. The elements kept to build these models are Mn and Ba for Lau Basin and Futuna volcanic arc and Fe and Ba for Mangatolo, as illustrated by the scatter plots.
Selecting specific elements for classification appears to be a prerequisite for individual assignments, as it was previously suggested (Mercier et al., 2011); all predictors had a different impact on the determination of origin. Some had a negative impact, especially those with low values that tended to induce noise rather than a discriminatory signal, as their accuracy is probably reduced. This was the case here for Cr, As, Cd, Sb, and, to a lesser extent, Cu and Sn. Removing these elements systematically improved the validation of geographic models. Moreover, our selected predictors differed depending on the spatial scale under scrutiny. Indeed, tracking back the origin of a specimen from the eastern region to the Lau Basin can be performed with seven predictors (Fig. 5c), whereas only two are needed to identify the site of origin within the Lau Basin with a much better success rate (Fig. 6a), under the hypothesis that the individual originates from that area.
In most cases, the support vector machine (SVM) method appeared to generate the best classification model (Sect. S3). Dixon and Brereton (2009) explored different model types from various datasets and concluded that the SVM method generally performs better than other methods when data normality is not met, which is the case here. Linear discriminant analysis, widely used in the literature, was selected as the most appropriate method for only one of the seven models presented in this work, with a well-defined clustering dataset (Fig. 6a; see Sect. S3).
We aimed to check whether there is, indeed, a site-specific fingerprint. Our dataset of larval shells therefore included at least two different sampling replicates from Ifremeria communities from one site for more than half of the sampled locations. This sampling strategy clearly showed that shell compositions were more variable between sites than within sites (Sect. S7), which strengthened the fact that most vent sites have their own elemental signatures.
There are, however, some limitations. First, the temporal variations in the elemental composition of the habitat water (linked to the vent activity) are unknown and would require temporal monitoring with a series of samples over several generations of larvae. This would allow us to assess the stability of the reference elemental fingerprints and thus minimize the misassignment risk associated with the use of elemental fingerprints on individuals collected at different periods. A relatively stable vent activity has been monitored over 7 years within a vent mussel assemblage at the Eiffel Tower edifice (Lucky Strike vent field, Mid-Atlantic Ridge; Van Audenhaege et al., 2022), although data on back-arc basin contexts are not currently available. This can be of particular interest for species such as S. tollmanni, which exhibit continuous reproduction (Poitrimol, 2022; Poitrimol et al., 2024): the elemental fingerprinting for such hydrothermal contexts should be stable at the scale of several consecutive cohorts of larvae. Second, our models are restricted to the sites we sampled, and other hydrothermal sites may still have to be discovered at the scale of a given area. Our sampling is however substantial and represents the largest effort done so far in deep-sea vents, with 14 hydrothermal sites, including two newly discovered vent fields (La Scala, in the Woodlark Basin (Boulart et al., 2022), and Mangatolo). To circumvent such a limitation, which is not specific to deep-sea settings but is also found in coastal systems, Simmonds et al. (2014) proposed to interpolate elemental fingerprints using a kriging method in locations where no samples are available. However, if this method is of interest in coastal environments where shell fingerprints could rely on the distance from specific sources (e.g., rivers), it is not relevant here. Indeed, hydrothermal vent systems are patchy environments, separated by hundreds to thousands of kilometers of background seawater, and the composition of these vents are often specific and strongly dependent on the subsurface phase separation processes (Charlou et al., 2000) that cannot be interpolated. Still, our models should at least help provide the area of origin, even if the exact site is unknown. Additional samples collected from other sites are therefore needed to complement the models for future assignment of post-dispersal stage individuals.
3.3 Can habitat water composition be used as reference for larval shell elemental fingerprinting?
As suspected, element concentrations in habitat water appeared to be poor predictors of their concentrations in shells of encapsulated larvae. The comparison of the element concentrations in habitat water and in shells of encapsulated larvae was carried out for six elements (Mg, Mn, Fe, Cu, Zn, and Sr) measured in both the water and the shells for 13 sites. Using elemental ratios to Ca, commonly reported for carbonates (e.g., Thorrold et al., 2007), a weak but significant positive correlation between water and shells was observed only for (Pearson correlation) and (Pearson and Spearman correlations) (Table 1), which suggested that element concentrations in habitat water are poor predictors of their concentrations in shells of encapsulated larvae. Because hydrothermal vent fluids are released in pulses and not continuously, a bias might occur during fluid sampling. To avoid such bias, we used Mn as a proxy for the hydrothermal fluid dilution in seawater (as this element is mainly introduced in seawater from hydrothermal activity) and compared the elemental ratios to Mn between habitat water and larval shells. A weak but significant positive correlation was noted for , , , and (r2=0.43–0.64, p value<0.001; Table 2), while was not significantly correlated. This showed that the elemental composition of the vent fluid influences the elemental fingerprint in the larval shells, at least for some elements.
Discrepancies between fluid and carbonate elemental composition have already been observed, including in molluskan larvae (Strasser et al., 2008a), and is likely due to vital effects (Weiner and Dove, 2003; Ulrich et al., 2021). Several factors can be at play to cause this difference. Firstly, S. tollmanni larval shells are formed in capsules, laid by the females, which contain an intracapsular fluid of unknown composition, particularly regarding the elemental composition. In particular, as shown above, was not significantly correlated between shells and water, which might be explained by a fraction of fluid Fe being trapped in the formation of Fe oxides that are not present in the S. tollmanni capsules. These capsules also represent a barrier against the surrounding environment, with an unknown permeability to elements, which may change during larval development, as shown in the coastal gastropod Crepidula fornicata (Maeda-Martínez, 2008). Secondly, metabolic activity requires specific elements (Mg, Ca, Fe, Cu, …) sampled from the environment to operate (Abreu and Cabelli, 2010; Vest et al., 2013), which may hamper their incorporation in the shell. Thirdly, larvae may control the chemistry of the fluid located between their body and the calcifying carbonate (i.e., where the shell is built) as shown in mussel larvae (Ramesh et al., 2017). During this process, they are able to change the pH of the fluid to increase calcification rate (Ramesh et al., 2017), which in turn favors Ca substitutions with other metals (Watson, 2004; DePaolo, 2011). Lastly, mineralogy is also responsible for the preferred uptake of some elements over others in the carbonate lattice because of their atomic radii and charges. For instance, Sr displays a significantly higher (several orders of magnitude) incorporation coefficient in aragonite (which is the calcium carbonate mineralogy of mollusk larval shells; Weiss et al., 2002) compared to calcite due to their spatial structure (Littlewood et al., 2017). Following this line, our data are clearly evidence that, although influencing the elemental composition of larval shells, the elemental concentrations of habitat water are not reliable direct predictors of shell chemistry, and thus habitat water cannot be used as a reference to assign the origin of individuals, as reported in fish otoliths and larval molluskan shells (Kroll et al., 2016; Strasser et al., 2008b; Bouchoucha et al., 2018). Therefore, references of habitat water composition cannot reliably replace references from larval shells for the identification of the natal origin of migrants. Only measurements from larval shells, such as the dataset we provide here, can be used as references for the identification of the natal origin of migrants.
The impact of upcoming deep-sea mining programs on hydrothermal vent communities throughout the world will strongly depend on the connectivity of populations. Our study assessed the accuracy of elemental fingerprinting of deep-sea hydrothermal mollusk larval shells, which is the first step to determine the geographic origin of individuals at different spatial scales. Sampled hydrothermal vent fields were characterized by distinct chemical compositions that are partially reflected in shells of encapsulated S. tollmanni larvae.
The composition of the preserved larval shell of a migrant can therefore be used to identify its origin with an accuracy ranging from 70 % to 100 % over 3500 km in the southwest Pacific Ocean. The most accurate classifications corresponded to geographically restricted vent fields and required fewer predictors. This approach is justified if larval dispersal potential is known to be spatially restricted by complementary information (typically from larval dispersal models or population genetics), therefore providing an easier-to-perform and more precise determination of natal sites when the genetic data fail to provide robust assignments. Alternatively, determining the proportion of self-recruitment at a site of interest can be a means to estimate the impact of habitat destruction and recovery (Mullineaux et al., 2010). This can be achieved for sites with high classification success rates, such as Kulo Lasi and Solwara 8. The next step is now to analyze the preserved pre-dispersal larval shell from juveniles after settlement (which corresponds to the encapsulated larval shells analyzed here) to determine their origin from our references. This method is promising and makes it possible to examine the proportion of self-recruitment at any site and assess the origin of migrants to study the connectivity and the ability for a population to recover after perturbations. This information will be of utmost importance for understanding the potential impacts of deep-sea mining ventures.
All data are available in the main text, in the Supplement, and at https://doi.org/10.5281/zenodo.7828332 (Mouchi et al., 2023b).
The supplement related to this article is available online at: https://doi.org/10.5194/bg-21-145-2024-supplement.
VM, CC, MM, OR, DJ, TB, and TC contributed to the conceptualization of the study; VM, CP, FC, and TC designed the methodology; VM, FC, YG, and OR participated in the investigation; VM performed the formal analysis and data curation; VM, DJ, TB, and TC completed the writing of the original draft; and VM, CP, FC, CC, MM, OR, DJ, TB, and TC reviewed and edited the manuscript.
The contact author has declared that none of the authors has any competing interests.
A CC-BY public copyright license has been applied by the authors to the present document and will be applied to all subsequent versions up to the author accepted manuscript arising from this submission, in accordance with the grant's open-access conditions. Preprints are available at https://www.biorxiv.org/content/10.1101/2023.01.03.522618v1 (last access: January 2024).
Publisher's note: Copernicus Publications remains neutral with regard to jurisdictional claims made in the text, published maps, institutional affiliations, or any other geographical representation in this paper. While Copernicus Publications makes every effort to include appropriate place names, the final responsibility lies with the authors.
We would like to thank the captains and crews of the French research vessel L'Atalante and the team in charge of the ROV Victor 6000 during the two parts of the CHUBACARC cruise (Hourdez and Jollivet, 2019, https://doi.org/10.17600/18001111), without whom sampling would not have been possible. We also wish to thank Nicolas Gayet for his help on board with the PIF and Camille Poitrimol for her help with collecting I. nautilei. Ship time and scientist travels were supported by the Flotte Océanique Française and the Centre National de la Recherche Scientifique (CNRS).
This research was funded and carried out under the ANR CERBERUS project (ANR-17-CE02-0003).
This paper was edited by Jack Middelburg and reviewed by Lisa Levin and Steffen Kiel.
Abreu, I. A. and Cabelli, D. E.: Superoxide dismutases – a review of the metal-associated mechanistic variations, BBA-Proteins Proteom., 1804, 263–274, https://doi.org/10.1016/j.bbapap.2009.11.005, 2010.
Adams, D., Arellano, S., and Govenar, B.: Larval Dispersal: Vent Life in the Water Column, Oceanography, 25, 256–268, https://doi.org/10.5670/oceanog.2012.24, 2012.
Amante, C. and Eakins, B. W.: ETOPO1 Global Relief Model converted to PanMap layer format, https://doi.org/10.1594/PANGAEA.769615, 2009.
Amon, D. J., Gollner, S., Morato, T., Smith, C. R., Chen, C., Christiansen, S., Currie, B., Drazen, J. C., Fukushima, T., Gianni, M., Gjerde, K. M., Gooday, A. J., Grillo, G. G., Haeckel, M., Joyini, T., Ju, S.-J., Levin, L. A., Metaxas, A., Mianowicz, K., Molodtsova, T. N., Narberhaus, I., Orcutt, B. N., Swaddling, A., Tuhumwire, J., Palacio, P. U., Walker, M., Weaver, P., Xu, X.-W., Mulalap, C. Y., Edwards, P. E. T., and Pickens, C.: Assessment of scientific gaps related to the effective environmental management of deep-seabed mining, Mar. Policy, 138, 105006, https://doi.org/10.1016/j.marpol.2022.105006, 2022.
Baco, A. R., Etter, R. J., Ribeiro, P. A., Heyden, S., Beerli, P., and Kinlan, B. P.: A synthesis of genetic connectivity in deep-sea fauna and implications for marine reserve design, Mol. Ecol., 25, 3276–3298, https://doi.org/10.1111/mec.13689, 2016.
Beck, L. A.: Two new neritacean limpets (Gastropoda: Prosobranchia: Neritacea: Phenacolepadidae) from active hydrothermal vents at Hydrothermal Field 1 “Wienerwald” in the Manus Back-Arc Basin (Bismarck Sea, Papua-New Guinea), Annalen des Naturhistorischen Museums in Wien. Serie B für Botanik und Zoologie, 93, 259–275, 1992.
Becker, B. J., Fodrie, F. J., McMillan, P. A., and Levin, L. A.: Spatial and temporal variation in trace elemental fingerprints of mytilid mussel shells: A precursor to invertebrate larval tracking, Limnol. Oceanogr., 50, 48–61, https://doi.org/10.4319/lo.2005.50.1.0048, 2005.
Becker, B. J., Levin, L. A., Fodrie, F. J., and McMillan, P. A.: Complex larval connectivity patterns among marine invertebrate populations, P. Natl. Acad. Sci. USA, 104, 3267–3272, https://doi.org/10.1073/pnas.0611651104, 2007.
Binns, R. A. and Scott, S. D.: Actively forming polymetallic sulfide deposits associated with felsic volcanic rocks in the eastern Manus back-arc basin, Papua New Guinea, Econ. Geol., 88, 2226–2236, https://doi.org/10.2113/gsecongeo.88.8.2226, 1993.
Boschen, R. E., Rowden, A. A., Clark, M. R., and Gardner, J. P. A.: Mining of deep-sea seafloor massive sulfides: A review of the deposits, their benthic communities, impacts from mining, regulatory frameworks and management strategies, Ocean Coast. Manage., 84, 54–67, https://doi.org/10.1016/j.ocecoaman.2013.07.005, 2013.
Bouchet, P. and Warén, A.: Ifremeria nautilei, nouveau gastéropode d'évents hydrothermaux, probablement associé à des bactéries symbiotiques, C.R. Acad. Sci., 312, 495–501, 1991.
Bouchoucha, M., Pécheyran, C., Gonzalez, J. L., Lenfant, P., and Darnaude, A. M.: Otolith fingerprints as natural tags to identify juvenile fish life in ports, Estuar. Coast. Shelf S., 212, 210–218, https://doi.org/10.1016/j.ecss.2018.07.008, 2018.
Boulart, C., Rouxel, O., Scalabrin, C., Le Meur, P., Pelleter, E., Poitrimol, C., Thiébaut, E., Matabos, M., Castel, J., Tran Lu Y, A., Michel, L. N., Cathalot, C., Chéron, S., Boissier, A., Germain, Y., Guyader, V., Arnaud-Haond, S., Bonhomme, F., Broquet, T., Cueff-Gauchard, V., Le Layec, V., L'Haridon, S., Mary, J., Le Port, A.-S., Tasiemski, A., Kuama, D. C., Hourdez, S., and Jollivet, D.: Active hydrothermal vents in the Woodlark Basin may act as dispersing centres for hydrothermal fauna, Commun. Earth Environ., 3, 64, https://doi.org/10.1038/s43247-022-00387-9, 2022.
Bounket, B., Tabouret, H., Gibert, P., Bareille, G., Pecheyran, C., Carrel, G., Argillier, C., and Morat, F.: Spawning areas and migration patterns in the early life history of Squalius cephalus (Linnaeus, 1758): Use of otolith microchemistry for conservation and sustainable management, Aquat. Conserv., 31, 2772–2787, https://doi.org/10.1002/aqc.3682, 2021.
Breusing, C., Biastoch, A., Drews, A., Metaxas, A., Jollivet, D., Vrijenhoek, R. C., Bayer, T., Melzner, F., Sayavedra, L., Petersen, J. M., Dubilier, N., Schilhabel, M. B., Rosenstiel, P., and Reusch, T. B. H.: Biophysical and Population Genetic Models Predict the Presence of “Phantom” Stepping Stones Connecting Mid-Atlantic Ridge Vent Ecosystems, Curr. Biol., 26, 2257–2267, https://doi.org/10.1016/j.cub.2016.06.062, 2016.
Breusing, C., Johnson, S. B., Mitarai, S., Beinart, R. A., and Tunnicliffe, V.: Differential patterns of connectivity in Western Pacific hydrothermal vent metapopulations: A comparison of biophysical and genetic models, Evol. Appl., 16, 22–35, https://doi.org/10.1111/eva.13326, 2023.
Carson, H. S.: Population connectivity of the Olympia oyster in southern California, Limnol. Oceanogr., 55, 134–148, https://doi.org/10.4319/lo.2010.55.1.0134, 2010.
Charlou, J. L., Donval, J. P., Douville, E., Jean-Baptiste, P., Radford-Knoery, J., Fouquet, Y., Dapoigny, A., and Stievenard, M.: Compared geochemical signatures and the evolution of Menez Gwen ( N) and Lucky Strike ( N) hydrothermal fluids, south of the Azores Triple Junction on the Mid-Atlantic Ridge, Chem. Geol., 171, 49–75, https://doi.org/10.1016/S0009-2541(00)00244-8, 2000.
Chen, W. W. and Deo, R. S.: Power Transformations to Induce Normality and their Applications, J. Roy. Stat. Soc. B, 66, 117–130, https://doi.org/10.1111/j.1467-9868.2004.00435.x, 2004.
Collins, P. C., Kennedy, R., and Dover, C. L. V.: A biological survey method applied to seafloor massive sulphides (SMS) with contagiously distributed hydrothermal-vent fauna, Mar. Ecol.-Prog. Ser., 452, 89–107, https://doi.org/10.3354/meps09646, 2012.
Combes, M., Vaz, S., Grehan, A., Morato, T., Arnaud-Haond, S., Dominguez-Carrió, C., Fox, A., González-Irusta, J. M., Johnson, D., Callery, O., Davies, A., Fauconnet, L., Kenchington, E., Orejas, C., Roberts, J. M., Taranto, G., and Menot, L.: Systematic Conservation Planning at an Ocean Basin Scale: Identifying a Viable Network of Deep-Sea Protected Areas in the North Atlantic and the Mediterranean, Frontiers in Marine Science, 8, 611358, https://doi.org/10.3389/fmars.2021.611358, 2021.
Comtet, T., Jollivet, D., Khripounoff, A., Segonzac, M., and Dixon, D. R.: Molecular and morphological identification of settlement-stage vent mussel larvae, Bathymodiolus azoricus (Bivalvia: Mytilidae), preserved in situ at active vent fields on the Mid-Atlantic Ridge, Limnol. Oceanogr, 45, 1655–1661, https://doi.org/10.4319/lo.2000.45.7.1655, 2000.
Cotte, L., Waeles, M., Pernet-Coudrier, B., Sarradin, P.-M., Cathalot, C., and Riso, R. D.: A comparison of in situ vs. ex situ filtration methods on the assessment of dissolved and particulate metals at hydrothermal vents, Deep-Sea Res. Pt. I, 105, 186–194, https://doi.org/10.1016/j.dsr.2015.09.005, 2015.
Cunha, M., Génio, L., Pradillon, F., Henry, M. C., Beaulieu, S., Birch, J., Campuzano, F., Carretón, M., Leo, F. D., Gula, J., Laming, S., Lindsay, D., Matos, F., Metaxas, A., Meyer-Kaiser, K., Mills, S., Queiroga, H., Rodrigues, C., Sarrazin, J., Watanabe, H., Young, R., and Young, C.: Foresight Workshop on Advances in Ocean Biological Observations: a sustained system for deep-ocean meroplankton, Research Ideas and Outcomes, 6, e54284, https://doi.org/10.3897/rio.6.e54284, 2020.
Danovaro, R., Aguzzi, J., Fanelli, E., Billett, D., Gjerde, K., Jamieson, A., Ramirez-Llodra, E., Smith, C. R., Snelgrove, P. V. R., Thomsen, L., and Dover, C. L. V.: An ecosystem-based deep-ocean strategy, Science, 355, 452–454, https://doi.org/10.1126/science.aah7178, 2017.
Danovaro, R., Fanelli, E., Aguzzi, J., Billett, D., Carugati, L., Corinaldesi, C., Dell'Anno, A., Gjerde, K., Jamieson, A. J., Kark, S., McClain, C., Levin, L., Levin, N., Ramirez-Llodra, E., Ruhl, H., Smith, C. R., Snelgrove, P. V. R., Thomsen, L., Van Dover, C. L., and Yasuhara, M.: Ecological variables for developing a global deep-ocean monitoring and conservation strategy, Nat. Ecol. Evol., 4, 181–192, https://doi.org/10.1038/s41559-019-1091-z, 2020.
DePaolo, D. J.: Surface kinetic model for isotopic and trace element fractionation during precipitation of calcite from aqueous solutions, Geochim. Cosmochim. Ac., 75, 1039–1056, https://doi.org/10.1016/j.gca.2010.11.020, 2011.
Dixon, S. J. and Brereton, R. G.: Comparison of performance of five common classifiers represented as boundary methods: Euclidean Distance to Centroids, Linear Discriminant Analysis, Quadratic Discriminant Analysis, Learning Vector Quantization and Support Vector Machines, as dependent on data structure, Chemometr. Intell. Lab., 95, 1–17, https://doi.org/10.1016/j.chemolab.2008.07.010, 2009.
Dunn, D. C., Van Dover, C. L., Etter, R. J., Smith, C. R., Levin, L. A., Morato, T., Colaço, A., Dale, A. C., Gebruk, A. V., Gjerde, K. M., Halpin, P. N., Howell, K. L., Johnson, D., Perez, J. A. A., Ribeiro, M. C., Stuckas, H., Weaver, P., and SEMPIA Workshop Participants: A strategy for the conservation of biodiversity on mid-ocean ridges from deep-sea mining, Sci. Adv., 4, eaar4313, https://doi.org/10.1126/sciadv.aar4313, 2018.
Fodrie, F. J., Becker, B. J., Levin, L. A., Gruenthal, K., and McMillan, P. A.: Connectivity clues from short-term variability in settlement and geochemical tags of mytilid mussels, J. Sea Res., 65, 141–150, https://doi.org/10.1016/j.seares.2010.09.001, 2011.
Fouquet, Y., Stackelberg, U. V., Charlou, J. L., Donval, J. P., Erzinger, J., Foucher, J. P., Herzig, P., Mühe, R., Soakai, S., Wiedicke, M., and Whitechurch, H.: Hydrothermal activity and metallogenesis in the Lau back-arc basin, Nature, 349, 778–781, https://doi.org/10.1038/349778a0, 1991.
Gagnaire, P., Broquet, T., Aurelle, D., Viard, F., Souissi, A., Bonhomme, F., Arnaud-Haond, S., and Bierne, N.: Using neutral, selected, and hitchhiker loci to assess connectivity of marine populations in the genomic era, Evol. Appl., 8, 769–786, https://doi.org/10.1111/eva.12288, 2015.
Gollner, S., Kaiser, S., Menzel, L., Jones, D. O. B., Brown, A., Mestre, N. C., van Oevelen, D., Menot, L., Colaço, A., Canals, M., Cuvelier, D., Durden, J. M., Gebruk, A., Egho, G. A., Haeckel, M., Marcon, Y., Mevenkamp, L., Morato, T., Pham, C. K., Purser, A., Sanchez-Vidal, A., Vanreusel, A., Vink, A., and Martinez Arbizu, P.: Resilience of benthic deep-sea fauna to mining activities, Mar. Environ. Res., 129, 76–101, https://doi.org/10.1016/j.marenvres.2017.04.010, 2017.
Gomes, I., Peteiro, L., Albuquerque, R., Nolasco, R., Dubert, J., Swearer, S., and Queiroga, H.: Wandering mussels: using natural tags to identify connectivity patterns among Marine Protected Areas, Mar. Ecol. Prog. Ser., 552, 159–176, https://doi.org/10.3354/meps11753, 2016.
Hannington, M., Herzig, P., Scott, S., Thompson, G., and Rona, P.: Comparative mineralogy and geochemistry of gold-bearing sulfide deposits on the mid-ocean ridges, Mar. Geol., 101, 217–248, https://doi.org/10.1016/0025-3227(91)90073-D, 1991.
Hannington, M., Jamieson, J., Monecke, T., Petersen, S., and Beaulieu, S.: The abundance of seafloor massive sulfide deposits, Geology, 39, 1155–1158, https://doi.org/10.1130/G32468.1, 2011.
Hannington, M. D., Jonasson, I. R., Herzig, P. M., and Petersen, S.: Physical and Chemical Processes of Seafloor Mineralization at Mid-Ocean Ridges, in: Geophysical Monograph Series, edited by: Humphris, S. E., Zierenberg, R. A., Mullineaux, L. S., and Thomson, R. E., American Geophysical Union, Washington, D. C., 115–157, https://doi.org/10.1029/GM091p0115, 2013.
Hoagland, P., Beaulieu, S., Tivey, M. A., Eggert, R. G., German, C., Glowka, L., and Lin, J.: Deep-sea mining of seafloor massive sulfides, Mar. Policy, 34, 728–732, https://doi.org/10.1016/j.marpol.2009.12.001, 2010.
Honig, A., Etter, R., Pepperman, K., Morello, S., and Hannigan, R.: Site and age discrimination using trace element fingerprints in the blue mussel, Mytilus edulis, J. Exp. Mar. Biol. Ecol., 522, 151249, https://doi.org/10.1016/j.jembe.2019.151249, 2020.
Hourdez, S. and Jollivet, D.: CHUBACARC cruise, L'Atalante R/V, French Oceanographic Cruises, https://doi.org/10.17600/18001111, 2019.
Humphris, S. E., Herzig, P. M., Miller, D. J., Alt, J. C., Becker, K., Brown, D., Brügmann, G., Chiba, H., Fouquet, Y., Gemmell, J. B., Guerin, G., Hannington, M. D., Holm, N. G., Honnorez, J. J., Iturrino, G. J., Knott, R., Ludwig, R., Nakamura, K., Petersen, S., Reysenbach, A.-L., Rona, P. A., Smith, S., Sturz, A. A., Tivey, M. K., and Zhao, X.: The internal structure of an active sea-floor massive sulphide deposit, Nature, 377, 713–716, https://doi.org/10.1038/377713a0, 1995.
International Seabed Authority: Environmental management plan for the Clarion-Clipperton Zone, ISBA/17/LTC/7, https://www.isa.org.jm/documents/isba-17-ltc-7/ (last access: January 2024), 2011.
International Seabed Authority: Report of ISA workshop on the design of “Impact Reference Zones” and “Preservation Reference Zones” in deep-sea mining contract areas, ISA Technical Study No. 21, https://www.isa.org.jm/publications/technical-study-21- report-of-isa-workshop-on-the-design-of-impact-reference- zones-and-preservation-reference-zones-in-deep-sea-mining-contract-areas/ (last access: January 2024), 2019.
Jochum, K. P., Nohl, U., Herwig, K., Lammel, E., Stoll, B., and Hofmann, A. W.: GeoReM: A New Geochemical Database for Reference Materials and Isotopic Standards, Geostand. Geoanal. Res., 29, 333–338, https://doi.org/10.1111/j.1751-908X.2005.tb00904.x, 2005.
Konn, C., Donval, J. P., Guyader, V., Germain, Y., Alix, A.-S., Roussel, E., and Rouxel, O.: Extending the dataset of fluid geochemistry of the Menez Gwen, Lucky Strike, Rainbow, TAG and Snake Pit hydrothermal vent fields: Investigation of temporal stability and organic contribution, Deep-Sea Res. Pt. I, 179, 103630, https://doi.org/10.1016/j.dsr.2021.103630, 2022.
Kroll, I., Poray, A., Puckett, B., Eggleston, D., and Fodrie, F.: Environmental effects on elemental signatures in eastern oyster Crassostrea virginica shells: using geochemical tagging to assess population connectivity, Mar. Ecol.-Prog. Ser., 543, 173–186, https://doi.org/10.3354/meps11549, 2016.
Le Bris, N., Sarradin, P.-M., and Caprais, J.-C.: Contrasted sulphide chemistries in the environment of 13∘ N EPR vent fauna, Deep-Sea Res. Pt. I, 50, 737–747, https://doi.org/10.1016/S0967-0637(03)00051-7, 2003.
Levin, L. A.: A review of methods for labeling and tracking marine invertebrate larvae, Ophelia, 32, 115–144, https://doi.org/10.1080/00785236.1990.10422028, 1990.
Levin, L. A.: Recent progress in understanding larval dispersal: new directions and digressions, Integr. Comp. Biol., 46, 282–297, https://doi.org/10.1093/icb/icj024, 2006.
Levin, L. A., Huggett, D., Myers, P., Bridges, T., and Weaver, J.: Rare earth tagging methods for the study of larval dispersal by marine invertebrates, Limnol. Oceanogr., 38, 346–360, https://doi.org/10.4319/lo.1993.38.2.0346, 1993.
Levin, L. A., Baco, A. R., Bowden, D. A., Colaco, A., Cordes, E. E., Cunha, M. R., Demopoulos, A. W. J., Gobin, J., Grupe, B. M., Le, J., Metaxas, A., Netburn, A. N., Rouse, G. W., Thurber, A. R., Tunnicliffe, V., Van Dover, C. L., Vanreusel, A., and Watling, L.: Hydrothermal Vents and Methane Seeps: Rethinking the Sphere of Influence, Front. Mar. Sci., 3, 72, https://doi.org/10.3389/fmars.2016.00072, 2016.
Levin, L. A., Wei, C.-L., Dunn, D. C., Amon, D. J., Ashford, O. S., Cheung, W. W. L., Colaço, A., Dominguez-Carrió, C., Escobar, E. G., Harden-Davies, H. R., Drazen, J. C., Ismail, K., Jones, D. O. B., Johnson, D. E., Le, J. T., Lejzerowicz, F., Mitarai, S., Morato, T., Mulsow, S., Snelgrove, P. V. R., Sweetman, A. K., and Yasuhara, M.: Climate change considerations are fundamental to management of deep-sea resource extraction, Glob. Change Biol., 26, 4664–4678, https://doi.org/10.1111/gcb.15223, 2020.
Littlewood, J. L., Shaw, S., Peacock, C. L., Bots, P., Trivedi, D., and Burke, I. T.: Mechanism of Enhanced Strontium Uptake into Calcite via an Amorphous Calcium Carbonate Crystallization Pathway, Cryst. Growth Des., 17, 1214–1223, https://doi.org/10.1021/acs.cgd.6b01599, 2017.
Maeda-Martínez, A. N.: Osmotic and ionic concentration of the egg capsule fluid of Crepidula fornicata in relation to embryonic development, Mar. Biol., 154, 643–648, https://doi.org/10.1007/s00227-008-0957-4, 2008.
McVeigh, D. M., Eggleston, D. B., Todd, A. C., Young, C. M., and He, R.: The influence of larval migration and dispersal depth on potential larval trajectories of a deep-sea bivalve, Deep-Sea Res. Pt. I, 127, 57–64, https://doi.org/10.1016/j.dsr.2017.08.002, 2017.
Mercier, L., Darnaude, A. M., Bruguier, O., Vasconcelos, R. P., Cabral, H. N., Costa, M. J., Lara, M., Jones, D. L., and Mouillot, D.: Selecting statistical models and variable combinations for optimal classification using otolith microchemistry, Ecol. Appl., 21, 1352–1364, https://doi.org/10.1890/09-1887.1, 2011.
Miller, K. A., Thompson, K. F., Johnston, P., and Santillo, D.: An Overview of Seabed Mining Including the Current State of Development, Environmental Impacts, and Knowledge Gaps, Front. Mar. Sci., 4, 418, https://doi.org/10.3389/fmars.2017.00418, 2018.
Mitarai, S., Watanabe, H., Nakajima, Y., Shchepetkin, A. F., and McWilliams, J. C.: Quantifying dispersal from hydrothermal vent fields in the western Pacific Ocean, P. Natl. Acad. Sci. USA, 113, 2976–2981, https://doi.org/10.1073/pnas.1518395113, 2016.
Mouchi, V., Godbillot, C., Forest, V., Ulianov, A., Lartaud, F., de Rafélis, M., Emmanuel, L., and Verrecchia, E. P.: Rare earth elements in oyster shells: provenance discrimination and potential vital effects, Biogeosciences, 17, 2205–2217, https://doi.org/10.5194/bg-17-2205-2020, 2020.
Mouchi, V., Broquet, T., and Comtet, T.: Preparation of mollusc larval shells for individual geochemical analysis v2, Protocols.io, https://doi.org/10.17504/protocols.io.bp2l61jwkvqe/v2, 2023a.
Mouchi, V., Pecheyran, C., Claverie, F., Cathalot, C., Matabos, M., Germain, Y., Rouxel, O., Jollivet, D., Broquet, T., and Comtet, T.: Elemental data from individual larval shells, Zenodo [data set], https://doi.org/10.5281/zenodo.7828333, 2023b.
Mullineaux, L., Mills, S., Sweetman, A., Beaudreau, A., Metaxas, A., and Hunt, H.: Vertical, lateral and temporal structure in larval distributions at hydrothermal vents, Mar. Ecol.-Prog. Ser., 293, 1–16, https://doi.org/10.3354/meps293001, 2005.
Mullineaux, L. S., Adams, D. K., Mills, S. W., and Beaulieu, S. E.: Larvae from afar colonize deep-sea hydrothermal vents after a catastrophic eruption, P. Natl. Acad. Sci. USA, 107, 7829–7834, https://doi.org/10.1073/pnas.0913187107, 2010.
Mullineaux, L. S., Metaxas, A., Beaulieu, S. E., Bright, M., Gollner, S., Grupe, B. M., Herrera, S., Kellner, J. B., Levin, L. A., Mitarai, S., Neubert, M. G., Thurnherr, A. M., Tunnicliffe, V., Watanabe, H. K., and Won, Y.-J.: Exploring the Ecology of Deep-Sea Hydrothermal Vents in a Metacommunity Framework, Front. Mar. Sci., 5, 49, https://doi.org/10.3389/fmars.2018.00049, 2018.
O'Leary, B. C., Allen, H. L., Yates, K. L., Page, R. W., Tudhope, A. W., McClean, C., Rogers, A. D., Hawkins, J. P., and Roberts, C. M.: 30x30: A blueprint for ocean protection, Umweltstiftung Greenpeace, https://www.greenpeace.org/30x30 (last access: January 2024), 2019.
Pante, E. and Simon-Bouhet, B.: marmap: A Package for Importing, Plotting and Analyzing Bathymetric and Topographic Data in R, PLOS ONE, 8, e73051, https://doi.org/10.1371/journal.pone.0073051, 2013.
Petersen, S., Krätschell, A., Augustin, N., Jamieson, J., Hein, J. R., and Hannington, M. D.: News from the seabed – Geological characteristics and resource potential of deep-sea mineral resources, Mar. Policy, 70, 175–187, https://doi.org/10.1016/j.marpol.2016.03.012, 2016.
Plouviez, S., LaBella, A. L., Weisrock, D. W., von Meijenfeldt, F. A. B., Ball, B., Neigel, J. E., and Van Dover, C. L.: Amplicon sequencing of 42 nuclear loci supports directional gene flow between South Pacific populations of a hydrothermal vent limpet, Ecol. Evol., 9, 6568–6580, https://doi.org/10.1002/ece3.5235, 2019.
Podowski, E., Ma, S., Luther, G., Wardrop, D., and Fisher, C.: Biotic and abiotic factors affecting distributions of megafauna in diffuse flow on andesite and basalt along the Eastern Lau Spreading Center, Tonga, Mar. Ecol. Prog. Ser., 418, 25–45, https://doi.org/10.3354/meps08797, 2010.
Podowski, E. L., Moore, T. S., Zelnio, K. A., Luther, G. W., and Fisher, C. R.: Distribution of diffuse flow megafauna in two sites on the Eastern Lau Spreading Center, Tonga, Deep-Sea Res. Pt. I, 56, 2041–2056, https://doi.org/10.1016/j.dsr.2009.07.002, 2009.
Poitrimol, C.: Distribution et partitionnement de la biodiversité hydrothermale dans un système discontinu de dorsales : le cas des bassins arrière-arc de l'ouest Pacifique, These de doctorat, Sorbonne université, HAL repository ID: tel-03900421, 2022.
Poitrimol, C., Thiébaut, É., Daguin-Thiébaut, C., Le Port, A.-S., Ballenghien, M., Tran Lu Y, A., Jollivet, D., Hourdez, S., and Matabos, M.: Contrasted phylogeographic patterns of hydrothermal vent gastropods along South West Pacific: Woodlark Basin, a possible contact zone and/or stepping-stone, PLoS ONE, 17, e0275638, https://doi.org/10.1371/journal.pone.0275638, 2022.
Poitrimol, C., Matabos, M., Veuillot, A., Ramière, A., Comtet, T., Boulart, C., Cathalot, C., and Thiébaut, É.: Reproductive biology and population structure of three hydrothermal gastropods (Lepetodrilus schrolli, L. fijiensis and Shinkailepas tollmanni) from the South West Pacific back-arc basins, Mar. Biol., 171, 31, https://doi.org/10.1007/s00227-023-04348-4, 2024.
Radziejewska, T., Błażewicz, M., Włodarska-Kowalczuk, M., Jóźwiak, P., Pabis, K., and Węsławski, J. M.: Benthic biology in the Polish exploration contract area of the Mid-Atlantic Ridge: The knowns and the unknowns. A review, Front. Mar. Sci., 9, 898828, https://doi.org/10.3389/fmars.2022.898828, 2022.
Ramesh, K., Hu, M. Y., Thomsen, J., Bleich, M., and Melzner, F.: Mussel larvae modify calcifying fluid carbonate chemistry to promote calcification, Nat. Commun., 8, 1709, https://doi.org/10.1038/s41467-017-01806-8, 2017.
Rouxel, O., Toner, B., Germain, Y., and Glazer, B.: Geochemical and iron isotopic insights into hydrothermal iron oxyhydroxide deposit formation at Loihi Seamount, Geochim. Cosmochim. Ac., 220, 449–482, https://doi.org/10.1016/j.gca.2017.09.050, 2018.
Sasaki, T., Warén, A., Kano, Y., Okutani, T., and Fujikura, K.: Gastropods from Recent Hot Vents and Cold Seeps: Systematics, Diversity and Life Strategies, in: The Vent and Seep Biota: Aspects from Microbes to Ecosystems, edited by: Kiel, S., Springer Netherlands, Dordrecht, 169–254, https://doi.org/10.1007/978-90-481-9572-5_7, 2010.
Simmonds, S., Kinlan, B., White, C., Paradis, G., Warner, R., and Zacherl, D.: Geospatial statistics strengthen the ability of natural geochemical tags to estimate range-wide population connectivity in marine species, Mar. Ecol. Prog. Ser., 508, 33–51, https://doi.org/10.3354/meps10871, 2014.
Smith, C. R., Tunnicliffe, V., Colaço, A., Drazen, J. C., Gollner, S., Levin, L. A., Mestre, N. C., Metaxas, A., Molodtsova, T. N., Morato, T., Sweetman, A. K., Washburn, T., and Amon, D. J.: Deep-Sea Misconceptions Cause Underestimation of Seabed-Mining Impacts, Trends Ecol. Evol., 35, 853–857, https://doi.org/10.1016/j.tree.2020.07.002, 2020.
Strasser, C. A., Mullineaux, L. S., and Thorrold, S. R.: Temperature and salinity effects on elemental uptake in the shells of larval and juvenile softshell clams Mya arenaria, Mar. Ecol. Prog. Ser., 370, 155–169, https://doi.org/10.3354/meps07658, 2008a.
Strasser, C. A., Mullineaux, L. S., and Walther, B. D.: Growth rate and age effects on Mya arenaria shell chemistry: Implications for biogeochemical studies, J. Exp. Mar. Biol. Ecol., 355, 153–163, https://doi.org/10.1016/j.jembe.2007.12.022, 2008b.
Suh, Y. J., Kim, M.-S., Lee, W.-K., Yoon, H., Moon, I., Jung, J., and Ju, S.-J.: Niche partitioning of hydrothermal vent fauna in the North Fiji Basin, Southwest Pacific inferred from stable isotopes, Mar. Biol., 169, 149, https://doi.org/10.1007/s00227-022-04129-5, 2022.
Suzuki, K., Yoshida, K., Watanabe, H., and Yamamoto, H.: Mapping the resilience of chemosynthetic communities in hydrothermal vent fields, Sci. Rep.-UK, 8, 9364, https://doi.org/10.1038/s41598-018-27596-7, 2018.
Thorrold, S., Zacherl, D., and Levin, L.: Population Connectivity and Larval Dispersal Using Geochemical Signatures in Calcified Structures, Oceanography, 20, 80–89, https://doi.org/10.5670/oceanog.2007.31, 2007.
Toffolo, L., Nimis, P., Tret'yakov, G. A., Melekestseva, I. Y., and Beltenev, V. E.: Seafloor massive sulfides from mid-ocean ridges: Exploring the causes of their geochemical variability with multivariate analysis, Earth-Sci. Rev., 201, 102958, https://doi.org/10.1016/j.earscirev.2019.102958, 2020.
Tran Lu Y, A.: La phylogéographie comparée d'espèces hydrothermales du Pacifique Ouest à l'heure de la génomique des populations, These de doctorat, Université de Montpellier, https://ged.biu-montpellier.fr/florabium/jsp/nnt.jsp?nnt=2022UMONG017 (last access: January 2024), 2022.
Tran Lu Y, A., Ruault, S., Daguin-Thiébaut, C., Castel, J., Bierne, N., Broquet, T., Wincker, P., Perdereau, A., Arnaud-Haond, S., Gagnaire, P., Jollivet, D., Hourdez, S., and Bonhomme, F.: Subtle limits to connectivity revealed by outlier loci within two divergent metapopulations of the deep-sea hydrothermal gastropod Ifremeria nautilei, Mol. Ecol., 31, 2796–2813, https://doi.org/10.1111/mec.16430, 2022.
Ulrich, R. N., Guillermic, M., Campbell, J., Hakim, A., Han, R., Singh, S., Stewart, J. D., Román-Palacios, C., Carroll, H. M., De Corte, I., Gilmore, R. E., Doss, W., Tripati, A., Ries, J. B., and Eagle, R. A.: Patterns of Element Incorporation in Calcium Carbonate Biominerals Recapitulate Phylogeny for a Diverse Range of Marine Calcifiers, Front. Earth Sci., 9, 641760, https://doi.org/10.3389/feart.2021.641760, 2021.
Van Audenhaege, L., Fariñas-Bermejo, A., Schultz, T., and Lee Van Dover, C.: An environmental baseline for food webs at deep-sea hydrothermal vents in Manus Basin (Papua New Guinea), Deep-Sea Res. Pt. I, 148, 88–99, https://doi.org/10.1016/j.dsr.2019.04.018, 2019.
Van Audenhaege, L., Matabos, M., Brind'Amour, A., Drugmand, J., Laës-Huon, A., Sarradin, P.-M., and Sarrazin, J.: Long-term monitoring reveals unprecedented stability of a vent mussel assemblage on the Mid-Atlantic Ridge, Prog. Oceanogr., 204, 102791, https://doi.org/10.1016/j.pocean.2022.102791, 2022.
Van Dover, C. L.: Impacts of anthropogenic disturbances at deep-sea hydrothermal vent ecosystems: A review, Mar. Environ. Res., 102, 59–72, https://doi.org/10.1016/j.marenvres.2014.03.008, 2014.
Vest, K. E., Hashemi, H. F., and Cobine, P. A.: The Copper Metallome in Eukaryotic Cells, in: Metallomics and the Cell, vol. 12, edited by: Banci, L., Springer Netherlands, Dordrecht, 451–478, https://doi.org/10.1007/978-94-007-5561-1_13, 2013.
Vic, C., Gula, J., Roullet, G., and Pradillon, F.: Dispersion of deep-sea hydrothermal vent effluents and larvae by submesoscale and tidal currents, Deep-Sea Res. Pt. I, 133, 1–18, https://doi.org/10.1016/j.dsr.2018.01.001, 2018.
Watson, E. B.: A conceptual model for near-surface kinetic controls on the trace-element and stable isotope composition of abiogenic calcite crystals, Geochim. Cosmochim. Ac., 68, 1473–1488, https://doi.org/10.1016/j.gca.2003.10.003, 2004.
Weiner, S. and Dove, P. M.: An Overview of Biomineralization Processes and the Problem of the Vital Effect, Rev. Mineral. Geochem., 54, 1–29, https://doi.org/10.2113/0540001, 2003.
Weiss, I. M., Tuross, N., Addadi, L., and Weiner, S.: Mollusc larval shell formation: amorphous calcium carbonate is a precursor phase for aragonite, J. Exp. Zool., 293, 478–491, https://doi.org/10.1002/jez.90004, 2002.
White, J. and Ruttenberg, B.: Discriminant function analysis in marine ecology: some oversights and their solutions, Mar. Ecol. Prog. Ser., 329, 301–305, https://doi.org/10.3354/meps329301, 2007.
Yahagi, T., Thaler, A. D., Van Dover, C. L., and Kano, Y.: Population connectivity of the hydrothermal-vent limpet Shinkailepas tollmanni in the Southwest Pacific (Gastropoda: Neritimorpha: Phenacolepadidae), PLoS ONE, 15, e0239784, https://doi.org/10.1371/journal.pone.0239784, 2020.
Yearsley, J. M., Salmanidou, D. M., Carlsson, J., Burns, D., and Van Dover, C. L.: Biophysical models of persistent connectivity and barriers on the northern Mid-Atlantic Ridge, Deep-Sea Res. Pt. II, 180, 104819, https://doi.org/10.1016/j.dsr2.2020.104819, 2020.
Zacherl, D., Manríquez, P., Paradis, G., Day, R., Castilla, J., Warner, R., Lea, D., and Gaines, S.: Trace elemental fingerprinting of gastropod statoliths to study larval dispersal trajectories, Mar. Ecol.-Prog. Ser., 248, 297–303, https://doi.org/10.3354/meps248297, 2003.