the Creative Commons Attribution 4.0 License.
the Creative Commons Attribution 4.0 License.
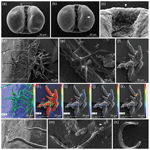
Comment on “The Volyn biota (Ukraine) – indications of 1.5 Gyr old eukaryotes in 3D preservation, a spotlight on the `boring billion' ” by Franz et al. (2023)
Martin J. Head
James B. Riding
Jennifer M. K. O'Keefe
Julius Jeiter
Julia Gravendyck
Franz et al. (2023) report a diverse and three-dimensionally preserved suite of mid-Proterozoic microfossils from miarolitic cavities within the granitic Volyn pegmatite field, a major granitic plutonic complex in NW Ukraine. The biota is dated at between ∼ 1.76 and ∼ 1.5 Ga and includes fungus-like objects. This biota is reported as evidence of organisms living within the continental lithosphere, illuminating part of a ∼ 1.8–0.8-billion-year interval of the Proterozoic Eon characterised by relatively low climatic variability and slow biological evolution. We show that at least some of this putative diversity represents modern contamination including plant hairs, a distinctive pollen grain assignable to the extant conifer genus Pinus, and likely later fungal growth. Comparable diversity is shown to exist in modern museum dust, presented as an example of potential airborne contamination and calling into question whether any part of the Volyn “biota” is biological in origin. We emphasise the need for scrupulous care in collecting, analysing, and identifying Precambrian microfossils.
Franz et al. (2023) recently published a detailed study on what they claim to be a diverse suite of in situ Proterozoic microfossils from the Volyn pegmatite field within the Korosten Pluton, a major granitic complex intruded into continental crust and located WNW of Kyiv, Ukraine. The fossils were extracted from eight kerite samples and one beryl crystal originating from metre-scale crystal-lined (miarolitic) cavities in the pegmatites (Franz et al., 2023, their Table 1; see also Franz et al., 2022a, their Table 1; together compiled in Table 1 here). Kerite is a black, carbon-rich, bitumoid compound found in these cavities in the Korosten Pluton. The origin of kerite has been disputed. Ginzburg et al. (1987), who first described fibrous kerite from the Volyn pegmatite, posited an abiogenic origin from volatile hydrocarbons (see also Luk'yanova et al., 1992), although Gorlenko et al. (2000) considered it to represent the remains of cyanobacterial mats probably of hydrothermal origin. The age of the kerite is less than the 1.76 Ga (latest Paleoproterozoic) radiometric (i.e. crystallisation) age of the pegmatites themselves (Shumlyanskyy et al., 2021) and has a minimum radiometric age of 1.5 Ga (earliest Mesoproterozoic) based on a breccia that contains degraded organic matter (Franz et al., 2017, 2022b). This biota therefore falls within the so-called “boring billion”, a 1.8–0.8 Gyr interval spanning the Mesoproterozoic that was characterised by low oxygen levels, relative climatic stability, sulfidic oceans, primitive life forms, and very slow biological evolution (Mukherjee et al., 2018, and references therein).
Table 1Overview of samples analysed by Franz et al. (2023) and associated information. Compiled from Franz et al. (2022a, 2023).
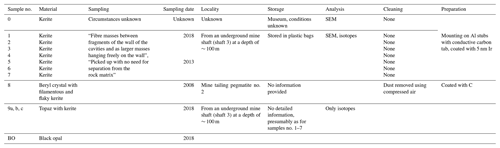
The biota described by Franz et al. (2023) comprises a substantial variety of morphotypes classified by these authors as filaments, hollow objects, irregular objects, and spherical objects, as well as flaky objects and agglutinating filaments interpreted as fossil biofilms. Together these forms are interpreted as representing free-living as well as sessile organisms. It is proposed that this biota lived in large cavities within the pegmatite, therefore representing part of the deep Mesoproterozoic biosphere, and was supposedly fossilised by a rapid influx of hot, mineral-rich fluids associated with a geyser system. These fluids were a mixture of magmatic fluids and meteoric water that caused surficial infiltration of aluminium silicates coupled with encrustations and intergrowths by various minerals, resulting in rapid fossilisation in an exceptional three-dimensional preservational state (Franz et al., 2022a, 2023, Fig. 1b). The biota apparently lived relatively close to the surface.
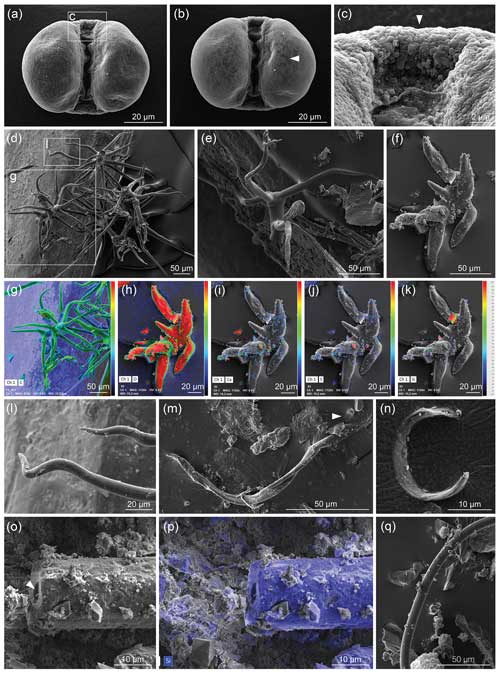
Figure 1Museum dust under the SEM as an example of potential airborne contamination. (a–c) Pinus pollen, (a) imaged with 6 kV accelerating voltage (AV); (b) imaged with 12 kV AV that reveals the infrareticulum of the pollen air sacs (arrowhead), as also seen using a high (10 kV) AV in Fig. 9a–c of Franz et al. (2023); and (c) detail of transitional area between leptoma and cappa with typical microrugulate ornamentation. (d–l) Plant hairs (trichomes) showing various morphologies and views, including (g–k) observed with the SEM combined with energy-dispersive X-ray spectroscopy (EDX) where heat maps depict distributions of elements (C, carbon; O, oxygen; Ca, calcium, S, sulfur; Si, silicon) on each object and (l) detail of sinuous tapering end of trichome. (m–q) Indeterminate filaments, with (m) an additional object (arrowhead) comparable to the arrowed specimen in Fig. 2i of Franz et al. (2023); (n) an unidentified specimen; (o–p) an elongate specimen with a hole (arrowhead) in the middle of the thread-like structure, where (p) reveals mostly silicon (in blue, from silicate) under EDX analysis; and (q) a smooth filament. Dust was mounted on aluminium stubs stickered with conductive carbon tabs and coated with palladium; imaged with a TESCAN CLARA SEM. Images: Julia Gravendyck.
The microfossils were studied and comprehensively illustrated by Franz et al. (2023, their Figs. 3–11) using a scanning electron microscope (SEM) and an electron microprobe analyser. Analysis of carbon and nitrogen stable isotopes and micro-Fourier transform infrared spectroscopy (micro-FTIR) absorption spectra were also undertaken.
We comment on several aspects that point to the inclusion of modern contaminants within the reported Volyn biota, and we present both an SEM analysis of modern museum dust and comparative micro-FTIR absorption spectra, calling into question the high morphological diversity described by Franz et al. (2023).
The 1.76–1.5 Ga age of the Volyn biota places it within a billion-year interval first identified by Buick et al. (1995) as lacking major biological, geological, and climatic events and later coined the “boring billion” (Brasier, 2012). This interval is now typically regarded as extending from ∼ 1.8 to 0.8 Ga (latest Paleoproterozoic–earliest Neoproterozoic, or “mid-Proterozoic”). It follows the final assembly of supercontinent Columbia at ∼ 2.0–1.8 Ga but includes the massive Grenville orogeny and assembly of Rodinia at ∼ 1.3–1.0 Ga (Johnson et al., 2020) prior to the advent of relatively modern-style subduction tectonics at 0.9–0.8 Ga (Santosh and Groves, 2023). Indeed, the interval between 1.850 and ∼ 0.850 Ga was characterised by relatively thin and unusually hot crust (Spencer et al., 2021). However, there were no major glacial events, and atmospheric oxygen levels remained low throughout. Biologically the interval is significant because eukaryotes had evolved by at least 1.7 Ga (Javaux and Lepot, 2018; Miao et al., 2019) and questionably as far back as 2.4 Ga (Barlow et al., 2023), with the appearance of photosynthesising eukaryotes estimated at 1.25 Ga (Gibson et al., 2018). Since all eukaryotes require oxygen to metabolise, the low levels of dissolved oxygen in shallow seas of the mid-Proterozoic (∼ 0.1 % of modern surface ocean levels; Tang et al., 2016) and perhaps other aspects of ocean chemistry including low nutrient trace element concentrations (Mukherjee et al., 2018) explain their restrained evolutionary diversification through much of this time. Short-lived pulses of oxygenation (≥ 4 % in Shang et al., 2019) do not appear to have significantly affected this overall evolutionary pattern, although a moderate increase in diversification during the late Mesoproterozoic–early Neoproterozoic (Javaux and Lepot, 2018) reflects a shift to more favourable conditions.
Franz et al. (2023) described some of the filaments in their Volyn biota as being “fungi-like” and considered molecular clocks with respect to fungi. We note from the more recent literature that clock ages of 887 Myr (Chang et al., 2015) and 1020 Myr (Lutzoni et al., 2018) for the origin of fungi are far younger than the 1.76–1.5 Gyr age of the Volyn biota. Other clocks based on individual fungal groups give younger ages still, for instance with the fungal origins of lichenisation postdating the earliest tracheophytes which appeared in the Ordovician (Nelson et al., 2019). The fungal affinity of filamentous microfossils reported from 1010–890 Ma rocks of Arctic Canada (Loron et al., 2019) was questioned by Berbee et al. (2020) in part because fungal phylogeny does not predict filamentous algae this early. It might be claimed that the fossil record of fungi is poorly sampled, yielding erroneously young estimates of divergence time. However, molecular clocks for the origin of animals, which are close relatives of fungi, approximate 700–800 Myr (Anderson et al., 2023), which is in general accord with recent published clocks for fungi. Unsurprisingly, among the early eukaryotes, uncontested fungi therefore make a relatively late appearance in the fossil record.
Within the mid-Proterozoic context of high crustal heat flow and low overall biotic diversity, the Volyn biota with its wide range of reported morphologies including structures ascribed to fungi-like organisms (Franz et al., 2023) represents an anomaly and therefore calls for scrutiny.
One of the “spherical objects” illustrated by Franz et al. (2023, their Fig. 9a–c) is a bisaccate pollen grain assignable to the extant coniferous genus Pinus (pine). Franz et al. (2023, p. 1910) described this entity as having a “double-ball shape … clearly grown onto the substrate” and bearing “remnants of a sheath [that] points to cell separation”. We note paired air sacs with subtle surface reticulation that reflects internal structure, joined by a central body with a microrugulate surface, all features that characterise this well-known type of pollen (e.g. Moore et al., 1991, their Plates 5, 59; Cojocaru et al., 2022). Bisaccate pollen first entered the fossil record in the late Carboniferous (Traverse, 2008; Fig. 4 in Riding, 2021). The modern native distribution of pine across Eurasia including Germany and Ukraine, the high numbers of airborne pollen produced by the parent tree, the occurrence of this pollen type in museum dust (Fig. 1a–c), and its unique morphology and pristine condition all allow us to confirm it as a modern contaminant.
Of the SEM images presented showing curvilinear filaments with smooth surfaces and circular cross sections, we note that a specimen illustrated by Franz et al. (2023, their Fig. 3j–l) from three views and described as “multiple, conical filaments with claw-like ends, growing from a common center” strongly resembles an abietiform trichome (Payne, 1978). Trichomes are appendages covering plant surfaces including leaves, stems, and fruits and are often microscopic outgrowths of a single epidermal cell (Esau, 1965). Trichomes similar to the specimen shown by Franz et al. (2023) are found for example in extant species of the fagacean genus Quercus (Hardin, 1976) and malvacean genus Alcea (Arabameri et al., 2020). We do not know if this specimen was studied with a backscattered electron (BSE) detector or micro-FTIR analysis to determine whether it was mineralised. However, biomineralisation of plant trichomes is a common phenomenon in angiosperms (e.g. Mustafa et al., 2018; Weigend et al., 2018), with silica, calcium carbonate, calcium oxalate, and calcium phosphate among these biominerals (Lowenstam, 1981; Ensikat et al., 2016). Such biominerals may show complex patterns even within a single-celled trichome (Ensikat et al., 2017) and to the untrained eye might appear similar to the products of fossilisation, especially if only known beyond the context of the plant body. Given that plant trichomes are present in the natural environment and are a common component of household and museum dust (Fig. 1d–l), we consider the extremely well-preserved specimen illustrated by Franz et al. (2023) to be a modern trichome.
Franz et al. (2023) presented micro-FTIR spectra intended to allow speculation on the biological affinities of the fossils recovered. Micro-FTIR is a powerful non-destructive technique yielding fundamental information on the molecular structure of organic and inorganic components in a sample by using an infrared source and analysing the absorption spectrum typically in the mid-infrared (approximately 4000 to 400 cm−1) region (Chen et al., 2015). Franz et al. (2023) measured three 40–60 µm wide translucent dark-brown fragments of kerite from their sample no. 0 (a museum sample from Kyiv), all showing similar spectra (Fig. 13a in Franz et al., 2023; Fig. 2g). These spectra were compared with that of a chitin standard (commercially supplied deacetylated chitin from shrimp shells) and were found to be closely similar if allowing for some thermal maturation of the kerite. The authors take this as evidence of a biological origin of the Volyn biota. Chitin is an essential ingredient of the cell walls of fungi, and its presence would support the claim of Franz et al. (2023) that morphologies displayed within the Volyn biota can be interpreted as fungal in origin. We note, however, that none of the existing micro-FTIR studies published on fossil fungal remains from a variety of ages and thermal maturities (Fig. 2) were included in their analysis or citations. Moreover, no modern fungal chitin standard was shown for comparison. Micro-FTIR spectra of extant, sub-fossil, and fossil fungi (Salman et al., 2010; O'Keefe et al., 2015; Dai et al., 2017; Chen et al., 2013, 2015; Fig. 2) display, with increasing thermal maturity, characteristic changes including reduction in wave height, reduction and eventual loss of the OH stretching band of water, reduction in lipids, and loss and/or alteration of carbohydrates and nucleic acids. These changes are largely absent from the spectra produced by the Volyn biota (Fig. 2g) and some other extremely deep-time possible fungal fossils (Loron et al., 2019; Fig. 2f), excepting the loss of carbohydrates. A spectrum from ∼ 635 Ma fungus-like fossils from China does show the overall reduction in wave height, loss of the OH stretching band, and reduction in lipids, although changes in chemistry producing lower wavenumbers are obscured by a quartz and pyrite overprint (Gan et al., 2021; Fig. 2e). Analysis of Early Devonian Prototaxites from the Bordeaux quarry (Vajda et al., 2022) produced micro-FTIR spectra very similar to those seen in fungus-like fossils from China. Given this variation (Fig. 2) and that CH stretching and bending observed in the Volyn biota spectra are common in many kinds of thermally mature organic matter, including solid bitumen and vitrinite, and in settings impacted by intrusions and hydrothermal alteration (Lis et al., 2005; Presswood et al., 2016; Liu et al., 2019; Teng et al., 2020), micro-FTIR results should not be used as evidence for the presence of fungi. Rock-inhabiting fungi are known from many substrates, including ancient granites with bitumen deposits reminiscent of kerite (Sazanova et al., 2022; Ivarsson et al., 2020a, b, 2021). White-appearing mycelium that is transparent under transmitted light observation is known to be invading rocks that form the Clarkia Lagerstätte (Fig. 3). Together, these observations raise concern that the material Franz et al. (2023) analysed represents (a) thermally mature non-fungal material, (b) younger fungal contamination, or (c) a combination thereof. The authors note that their specimens were translucent, but they provided no light microscope images that would help discern fossil hyphae and/or spores from recent contaminants (presence of nuclear materials, lipids, starches, etc.).
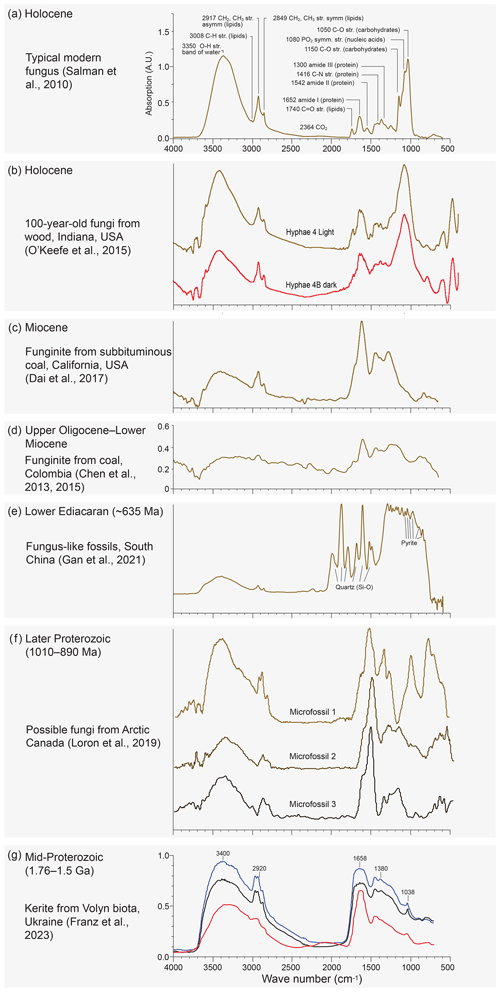
Figure 2Published Micro-FTIR spectra of (a) typical modern fungus spectrum (Fig. 1 in Salman et al., 2010) showing significant absorption bands and their main functional groups (note large bands at ∼ 1050 and 3350 cm−1); (b) latest Holocene hyphae recovered from a log buried in a migrating sand dune for nearly a century (unpublished spectra from O'Keefe et al., 2015); (c) Miocene funginite from subbituminous Sand Bank Coal, Eel River Formation, California (Fig. 7a in Dai et al., 2017), (d) late Oligocene–Early Miocene fungal remains recovered from bituminous C coals, Amagá Formation, Colombia (Figs. 2 and 4b in Chen et al., 2013, 2015); and (e) pyritised fungus-like fossils from the ∼ 635 Ma lower Ediacaran of southern China showing bands probably representing silica and pyrite (Fig. 6b in Gan et al., 2021). (f) Possible fungi from the later Proterozoic of Arctic Canada (Loron et al., 2019, their Fig. 2 and extended data Fig. 3). (g) The Volyn study presented by Franz et al. (2023, their Fig. 13a). Note the general reduction in absorption with increasing melanisation (dark hyphae) (b) and age/thermal maturity (c–e). Note also rising then lowering absorption and minor changes in wavenumber for the amide peak at 1652–1646 cm−1. In the kerite spectra (g) this peak is at 1658 cm−1, somewhat higher than in any of the other spectra. Note also the loss of the carbohydrate/lignin peak at 1082–1050 cm−1 from modern to ancient samples (a–d). This peak is entirely absent in the kerite spectra and microfossils 2 and 3 (f) of Loron et al. (2019). The kerite spectra are overall most similar to the spectrum shown in (c), although they lack peaks at 1446 and 1277 cm−1, and to that shown in (f), although it lacks saccharides.
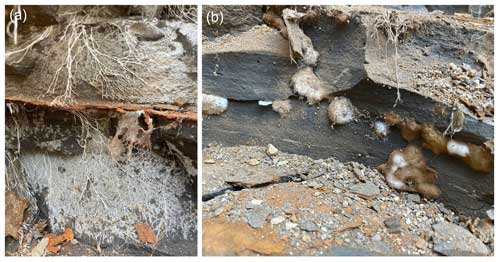
Figure 3Examples of growth habits of extant lithotrophic fungi from the Clarkia Lagerstätte in Idaho, USA. Fungal mycelium in the form of fan-shaped mats of hyphae along a joint opened up during excavation (a) and fungal mycelium forming bulbous structures in voids in the rock, some with melanised spores coating the surface (b). Photos JMKO, July 2021.
Museums are a convenient source of airborne contaminants where they accumulate as dust. Such airborne contamination can compromise samples at any stage from collection (as in a ventilated mine) to analysis. As an illustration of their pervasiveness and diverse composition, we used a SEM combined with energy-dispersive X-ray spectroscopy (EDX) to examine dust from fossil displays of the palaeobotanical collection in the Goldfuß Museum, Bonn (Fig. 1). Our EDX analysis is very similar to the energy-dispersive X-ray analysis EDAX EDS system used by Franz et al. (2023) on selected cross sections (their Fig. 10j–l), but it allows elements comprising or attached to the observed objects to be visualised over the entire surface as imaged by the SEM (Fig. 1). In addition to Pinus pollen (Fig. 1a–c), a number of trichomes (Fig. 1d–l) were observed, along with indeterminate filaments (Fig. 1m–q) reminiscent of the shapes and diversity depicted by Franz et al. (2023) and with similar surface structures, with one consisting primarily of silica showing an opening at its end (Fig. 1o and p vs. Fig. 11 of Franz et al., 2023). We note in particular the appearance of the infrareticulum visible on the surface of air sacs in the Pinus pollen using a high accelerating voltage (12 kV) similar to the 10 kV used by Franz et al. (2023). The variety of trichomes encountered attests to the ease with which they detach from plants and become airborne. The samples examined by Franz et al. (2023) under SEM did not receive special cleaning other than with compressed air on one sample, and in addition to samples collected underground they included a specimen from a museum and another from mine tailings. The underground specimens were simply “picked up” and not separated from the rock matrix. It seems that all samples were able to acquire airborne “dust” and other contaminants before and/or after sampling. Had Franz et al. (2023) used EDX in addition to applying EDAX EDS to selected cross sections, they would have been easily able to determine the elemental surface distribution for all specimens they imaged using a SEM, which could have assisted in discriminating extant contaminants from fossil material.
Stable carbon and nitrogen isotope data obtained by Franz et al. (2023) from bulk samples (all samples were analysed) fall in the range of −31 ‰ to −47 ‰ δ13C and of +3 ‰ to +10 ‰ δ15N. Because modern fungi have a range restricted to −19 ‰ to −29 ‰ δ13C, with the main cluster at −22 ‰ to −28 ‰ δ13C (Mayor et al., 2009; Franz et al., 2023), Franz et al. (2023) explained the much lower values of δ13C in the Volyn biota as reflecting incorporation of carbon from methanogenic bacteria into the fungi-like organisms. These authors concluded that “We exclude an abiotic origin … because of the extremely low δ13C values and the large variation in morphology.” But laboratory experiments have shown that organic compounds produced by abiotic synthesis under hydrothermal conditions can have 13C depletions in the range of biological fractionation, with methane for example yielding a value of −50.3 ‰ (McCollom and Seewald, 2006). Nitrogen is an important component of organic matter, but there is increasing evidence that various carbon-based solid organic compounds including those containing nitrogen can be synthesised in Fischer–Tropsch-type or Friedel–Crafts-type reactions (Ménez et al., 2018; Nan et al., 2021) in hydrogen-rich hydrothermal systems. We therefore consider the carbon and nitrogen isotope data presented by Franz et al. (2023) not to be conclusive evidence of biogenicity for the Volyn kerite.
The Volyn biota at 1.76–1.5 Ga, which includes putative fungus-like organisms, is claimed to represent life in a highly specialised continental setting within subterranean cavities associated with a geyser system. It cannot therefore be compared directly with published mid-Proterozoic records which are mostly marine and include photosynthetic organisms, as Franz et al. (2023) explain. But the hostile ambient conditions in which this biota is proposed to have lived, including high temperatures, lack of sunlight, and high radiation levels, require exceptional proof for these claims to be accepted, especially given the high morphological variation recorded. It would be necessary to consider and eliminate the possibility of modern contamination, given the pristine state of preservation of many components in the Volyn biota and knowledge that fungal remains associated with bitumen in cavities of brecciated granitoids have been shown to be significantly younger than the rocks themselves (Ivarsson et al., 2020b, 2021). Franz et al. (2022a, 2023) do not consider or exclude contamination in any form, yet the age control of other pristine microfossils in igneous rocks is generally lacking or, where present, represents allochthonous material (e.g. Drake et al., 2021).
We identify evidence of modern contamination within the presented Volyn biota, and we raise concern that the presence of chitin-like material is the result of contamination by younger fungi. Franz et al. (2023) discuss the morphological and geochemical similarities of their specimens with fungi, and Bengtson et al. (2017) reported fungus-like objects extending back 2.4 Ga although these present similar concerns as those of the Volyn biota. However, Mesoproterozoic records of such fungus-like fossils (e.g. Loron et al., 2021; Miao et al., 2021) lack the exceptional preservational state of the Volyn biota. While a model was proposed involving hydrothermal fluids to explain rapid preservation for the Volyn biota, this remains a unique process without analogue and therefore requires exceptional supporting evidence.
Documenting evidence of early life requires scrupulous care to minimise and identify any possible contamination. This begins with the careful collection of specimens, ideally from freshly exposed rock surfaces to minimise the possibility of deep penetration by modern fungi. Comparable studies have used petrographic thin sections (Ongeluk Formation, South Africa, 2.4 Ga, Bengtson et al., 2017; Grassy Bay Formation, Canada, 1 Ga, Loron et al., 2019), transmission electron microscopy (Bengtson et al., 2017; Loron et al., 2019), and synchrotron-radiation X-ray tomographic microscopy of discrete blocks (Bengtson et al., 2017) or involve splitting (Strelley Pool, Australia, 3.4 Ga; Alleon et al., 2018) or etching (Onverwacht Group, Australia, 3.5–3.3 Ga; Westall et al., 2001) of the rock prior to its study. Some studies have used light microscopy to reveal the internal structure of the organic component (Loron et al., 2019, 2021; Miao et al., 2021). Airborne contamination can occur in the laboratory, and when pristine three-dimensional fossils are identified, these should be checked using light microscopy to ensure there are no fresh cell contents.
Ultimately, all lines of evidence must be weighed, including micro-FTIR spectra or EDX of all specimens, to exclude the possibility of contamination before claims of ancient biotas possessing unique preservation and morphological diversity are made.
We conclude that the Volyn biota presented by Franz et al. (2023) incorporates modern contaminants including plant hairs and a pollen grain, all present in modern dust from a museum (and no doubt elsewhere), and the micro-FTIR spectra of specimens are not diagnostic of the thermal maturity that might be expected of in situ fungal-like organisms but suggest the presence of younger fungal contamination. Moreover, the carbon and nitrogen isotope data presented by Franz et al. (2023) do not provide conclusive evidence of biological affinity. We do not necessarily challenge the view that the fibrous kerite itself was formed along with the Volyn pegmatites in the mid-Proterozoic. But these carbonaceous fibres have been attributed to postmagmatic abiotic synthesis from hot methane-containing fluids particularly in the vapour phase (Lukjanova and Lovzova, 1994). Such an origin would explain the lack of internal structure found in fibres illustrated by Franz et al. (2023). These processes, while discussed by Franz et al. (2023), now require further consideration, especially in light of “chemical gardening” laboratory experiments that have synthesised fibres resembling purported fossils in both morphology and composition (McMahon, 2019). We have doubts therefore whether any of the in situ Volyn “biota” is organic in origin.
All data are available as figures in the text.
Concept, writing, interpretation: all authors; concept origination: JG; manuscript coordination: MJH; analysis of museum dust: JG; fungal: JMKO'K; trichome: JJ; geological interpretation: MJH, JBR.
The contact author has declared that none of the authors has any competing interests.
Publisher’s note: Copernicus Publications remains neutral with regard to jurisdictional claims made in the text, published maps, institutional affiliations, or any other geographical representation in this paper. While Copernicus Publications makes every effort to include appropriate place names, the final responsibility lies with the authors.
We thank Yaron Malkowsky (University of Bonn) for his support with EDX and SEM work and Maximilian Weigend (University of Bonn, Botanic Gardens) for his consultation on trichomes. Maria Mastalerz (Indiana Geological and Water Survey) kindly gave permission to publish previously unpublished spectra from near-recent fungal fossils obtained during a joint investigation with Jennifer M. K. O'Keefe as part of Fig. 2. We are most grateful to the three journal reviewers, including Nicholas J. Butterfield, for their helpful comments and for bringing additional literature to our attention. James B. Riding publishes with the approval of the Director, British Geological Survey (NERC).
This research has been supported by the Deutsche Forschungsgemeinschaft (grant no. 471591895) and the Natural Sciences and Engineering Research Council of Canada (Discovery Grant).
This open-access publication was funded by the University of Bonn.
This paper was edited by Cindy De Jonge and reviewed by Nicholas J. Butterfield and two anonymous referees.
Alleon, J., Bernard, S., Le Guillou, C., Beyssac, O., Sugitani, K., and Robert, F.: Chemical nature of the 3.4 Ga Strelley Pool microfossils, Geochem. Persp. Lett., 7, 37–42, 2018.
Anderson, R. P., Woltz, C. R., Tosca, N. J., Porter, S. M., and Briggs, D. E. G.: Fossilisation processes and our reading of animal antiquity, Trend. Ecol. Evol., 38, 1060–1071, https://doi.org/10.1016/j.tree.2023.05.014, 2023.
Arabameri, M., Khodayari, H., and Zarre, S.: Trichome micromorphology in Alcea L. and allied genera (Malvaceae) and its systematic implication, Nord. J. Bot., 38, 1–16, https://doi.org/10.1111/njb.02540, 2020.
Barlow, E. V., House, C. H., Liu, M.-C., Wetherington, M. T., and Van Kranendonk, M. J.: Distinctive microfossil supports early Paleoproterozoic rise in complex cellular organisation, Geobiology, 1–23, https://doi.org/10.1111/gbi.12576, 2023.
Bengtson, S., Rasmussen, B., Ivarsson, M., Muhling, J., Broman, C., Marone, F., Stampanomi, M., and Bekker, A.: Fungus-like mycelial fossils in 2.4 billion-year-old vesicular basalt, Nat. Ecol. Evol., 1, 1–6, https://doi.org/10.1038/s41559-017-0141, 2017.
Berbee, M. L., Strullu-Derrien, C., Delaux, P.-M., Strother, P. K., Kenrick, P., Selosse, M.-A., and Taylor, J. W.: Genomic and fossil windows into the secret lives of the most ancient fungi, Nat. Rev. Microbiol., 18, 717–730, https://doi.org/10.1038/s41579-020-0426-8, 2020.
Brasier, M.: Secret Chambers: The Inside Story of Cells and Complex Life, Oxford University Press, p. 211, ISBN 978-0-19-964400-1, 2012.
Buick, R., Des Marais, D. J., and Knoll, A. H.: Stable isotopic compositions of carbonates from the Mesoproterozoic Bangemall group, northwestern Australia, Chem. Geol., 123, 153–171, 1995.
Chang, Y., Wang, S., Sekimoto, S., Aerts, A. L., Choi, C., Clum, A., LaButti, C. M., Lindquist, E. A., Ngan, C. Y., Ohm, R. A., Salamov, A. A., Grigoriev, I. V., Spatafora, J. W., and Berbee, M. L.: Phylogenomic analyses indicate that early fungi evolved digesting cell walls of algal ancestors of land plants, Genome Biol. Evol., 7, 1590–1601, https://doi.org/10.1093/gbe/evv090, 2015.
Chen, Y., Caro, L. D., Mastalerz, M., Schimmelmann, A., and Blandon, A.: Mapping the chemistry of resinite, funginite and associated vitrinite in coal with micro-FTIR, J. Microsc., 249, 69–81, https://doi.org/10.1111/j.1365-2818.2012.03685.x, 2013.
Chen, Y., Zou, C., Mastalerz, M., Hu, S., Gasaway, C., and Tao, X.: Applications of micro-Fourier Transform Infrared Spectroscopy (FTIR) in the geological sciences – A review, Int. J. Mol. Sci., 16, 30223–30250, https://doi.org/10.3390/ijms161226227, 2015.
Cojocaru, R. Mannix, O., Capron, M., Miller, C. G., Jouneau, P.-H., Gallet, B., Falconet, D., Pacureanu, A., and Stukins, S.: A biological nanofoam: The wall of coniferous bisaccate pollen, Sci. Adv., 8, eabd0892, https://doi.org/10.1126/sciadv.abd0892, 2022.
Dai, S., Bartley, R., Bartley, S., Valentim, B., Guedes, A., O'Keefe, J. M. K., Kus, J., Mastalerz, M., and Hower, J. C.: Organic geochemistry of funginite (Miocene, Eel River, Mendocino County, California, USA) and macrinite (Cretaceous, Inner Mongolia, China), Int. J. Coal Geol., 179, 60–71, https://doi.org/10.1016/j.coal.2017.05.015, 2017.
Drake, H., Ivarsson, M., Heim, C., Snoeyenbos-West, O., Bengtson, S., Belivanova, V., and Whitehouse, M.: Fossilized anaerobic and possibly methanogenesis-fueling fungi identified deep within the Siljan impact structure, Sweden, Commun. Earth Environ., 2, 34, https://doi.org/10.1038/s43247-021-00107-9, 2021.
Ensikat, H.-J., Geisler, T., and Weigend, M.: A first report of hydroxylated apatite as structural biomineral in Loasaceae – plants' teeth against herbivores, Sci. Rep., 6, 26073, https://doi.org/10.1038/srep26073, 2016.
Ensikat, H.-J., Mustafa, A., and Weigend, M.: Complex patterns of multiple biomineralization in single-celled plant trichomes of the Loasaceae, Am. J. Bot., 104, 195–206, https://doi.org/10.3732/ajb.1600331, 2017.
Esau, K.: Plant Anatomy, 2nd Edn., John Wiley and Sons, New York, 767 pp., John Wiley and Sons, ISBN: 0471244562, 1965.
Franz, G., Khomenko, V., Vishnyevskyy, A., Wirth, R., Nissen, J., and Rocholl, A.: Biologically mediated crystallization of buddingtonite in the Paleoproterozoic: Organic-igneous interactions from the Volyn pegmatite, Ukraine, Am. Mineral., 102, 2119–2135, 2017.
Franz, G., Lyckberg, P., Khomenko, V., Chournousenko, V., Schulz, H.-M., Mahlstedt, N., Wirth, R., Glodny, J., Gernert, U., and Nissen, J.: Fossilization of Precambrian microfossils in the Volyn pegmatite, Ukraine, Biogeosciences, 19, 1795–1811, https://doi.org/10.5194/bg-19-1795-2022, 2022a.
Franz, G., Sudo, M., and Khomenko, V.: 40Ar 39Ar dating of a hydrothermal pegmatitic buddingtonite–muscovite as- semblage from Volyn, Ukraine, Eur. J. Mineral., 34, 7–18, https://doi.org/10.5194/ejm-34-7-2022, 2022b.
Franz, G., Khomenko, V., Lyckberg, P., Chournousenko, V., Struck, U., Gernert, U., and Nissen, J.: The Volyn biota (Ukraine) – indications of 1.5 Gyr old eukaryotes in 3D preservation, a spotlight on the “boring billion”, Biogeosciences, 20, 1901–1924, https://doi.org/10.5194/bg-20-1901-2023, 2023.
Gan, T., Luo, T., Pang, K., Zhou, C., Zhou, G., Wan, B., Li, G., Yi, Q., Czaja, A. D., and Xiao, X.: Cryptic terrestrial fungus-like fossils of the early Ediacaran Period, Nat. Commun., 12, 641, https://doi.org/10.1038/s41467-021-20975-1, 2021.
Gibson, T. M., Shih, P. M., Cumming, V. M., Fischer, W. W., Crockford, P. W., Hodgskiss, M. S. W., Wörndle, S., Creaser, R. A., Rainbird, R. H., Skulski, T. M., and Halverson, G. P.: Precise age of Bangiomorpha pubescens dates the origin of eukaryotic photosynthesis, Geology, 46, 135–138, https://doi.org/10.1130/G39829.1, 2018.
Ginzburg, A. I., Bulgakov, V. S., Vasilishin, I. S., Luk'yanova, V. T., Solntseva, L. S., Urmenova, A. M., and Uspenskaya, V. A.: Kerite from pegmatites of Volyn, Dokl. Akad. Nauk SSSR, 292, 188–191, 1987 (in Russian).
Gorlenko, V. M., Zhmur, S. I., Duda, V. I., Osipov, G. A., Suzina, N. E., and Dmitriev, V. V.: Fine structure of fossilized bacteria in Volyn kerite, Orig. Life Evol. Biosph., 30, 567–577, 2000.
Hardin, J. W.: Terminology and classification of Quercus trichomes, J. Elisha Mitch. Sci. S., 92, 151–161, 1976.
Ivarsson, M., Drake, H., Bengtson, S., and Rasmussen, B.: A cryptic alternative for the evolution of hyphae, BioEssays, 42, 1900183, https://doi.org/10.1002/bies.201900183, 2020a.
Ivarsson, M., Drake, H., Neubeck, A., Sallstedt, T., Bengtson, S., Roberts, N. M. W., and Rasmussen, B.: The fossil record of igneous rock, Earth-Sci. Rev., 210, 103342, https://doi.org/10.1016/j.earscirev.2020.103342, 2020b.
Ivarsson, M., Drake, H., Neubeck, A., Snoeyenbos-West, O., Belivanova, V., and Bengtson, S.: Introducing palaeolithobiology, GFF, 143, 305–319, https://doi.org/10.1080/11035897.2021.1895302, 2021.
Javaux, E. J. and Lepot, K.: The Paleoproterozoic fossil record: Implications for the evolution of the biosphere during Earth's middle-age, Earth-Sci. Rev., 176, 68–86, https://doi.org/10.1016/j.earscirev.2017.10.001, 2018.
Johnson, T. A., Vervoort, J. D., Ramsey, M. J., Southworth, S., and Mulcahy, S. R.: Tectonic evolution of the Grenville Orogen in the central Appalachians, Precambrian Res., 346, 105740, https://doi.org/10.1016/j.precamres.2020.105740, 2020.
Lis, G. P., Mastalerz, M., Schimmelmann, A., Lewa, M. D., and Stankiewicz, B. A.: FTIR absorption indices for thermal maturity in comparison with vitrinite reflectance Ro in type-II kerogens from Devonian black shales, Org. Geochem., 36, 1533–1552, https://doi.org/10.1016/j.orggeochem.2005.07.001, 2005.
Liu, B., Schieber, J., and Mastalerz, M.: Petrographic and micro-FTIR study of organic matter in the Upper Devonian New Albany Shale during thermal maturation: Implications for kerogen transformation, Chap. 10, in: Mudstone Diagenesis: Research Perspectives for Shale Hydrocarbon Reservoirs, Seals, and Source Rocks, edited by: Camp, W. K., Milliken, K. L., Taylor, K., Fishman, N., Hackley, P. C., and Macquaker, J. H. S., AAPG Memoir, 120, 165–188, https://doi.org/10.1306/13672216M1213380, 2019.
Loron, C. C., François, C., Rainbird, R. H., Turner, E. C., Borensztajn, S., and Javaux, E. J.: Early fungi from the Proterozoic era in Arctic Canada, Nature, 570, 232–235, https://doi.org/10.1038/s41586-019-1217-0, 2019.
Loron, C. C., Halverson, G. P., Rainbird, R. H., Skulski,T., Turner, E.C., and Javaux, E. J.: Shale-hosted biota from the Dismal Lakes Group in Arctic Canada supports an early Mesoproterozoic diversification of eukaryotes, J. Paleontol., 95, 1113–1137, https://doi.org/10.1017/jpa.2021.45, 2021.
Lowenstam, H. A.: Minerals formed by organisms, Science, 211, 1126–1131, https://doi.org/10.1126/science.7008198, 1981.
Lukjanova, V. T. and Lovzova, R.V.: Carbon fibers in nature, Carbon, 32, 777–783, 1994.
Luk'yanova, V. T., Lobzova, R. V., and Popov, V. T.: Filaceous kerite in pegmatites of Volyn, Izvestiya Ross. Akad. Nauk Ser. Geol., 5, 102–118, 1992 (in Russian).
Lutzoni, F., Nowak, M. D., Alfaro, M. E., Reeb, V., Miadlikowska, J., Krug, M., Arnold, A. E., Lewis, L. A., Swofford, D. L., Hibbett, D., Hilu, K., James, T. Y., Quandt, D., and Magalloìn, S.: Contemporaneous radiations of fungi and plants linked to symbiosis, Nat. Commun., 9, 5451, https://doi.org/10.1038/s41467-018-07849-9, 2018.
Mayor, J. R., Schuur, E. A. G., and Henkel, T. W.: Elucidating the nutritional dynamics of fungi using stable isotopes, Ecol. Lett., 12, 171–183, https://doi.org/10.1111/j.1461-0248.2008.01265.x, 2009.
McCollom, T. M., and Seewald, J. S.: Carbon isotope composition of organic compounds produced by abiotic synthesis under hydrothermal conditions, Earth Planet. Sc. Lett., 243, 74–84, https://doi.org/10.1016/j.epsl.2006.01.027, 2006.
McMahon, S.: Earth's earliest and deepest purported fossils may be iron-mineralized chemical gardens, Proc. Roy. Soc. B, 286, 20192410, https://doi.org/10.1098/rspb.2019.2410, 2019.
Ménez, B., Pisapia, C., Andreani, M., Jamme, F., Vanbellingen, Q. P., Brunelle, A., Richard, L., Dumas, P., and Réfrégiers, M.: Abiotic synthesis of amino acids in the recesses of the oceanic lithosphere, Nature, 564, 59–63, https://doi.org/10.1038/ s41586-018-0684-z, 2018.
Miao, L., Moczydłowska, M., Zhu, S., and Zhu, M.: New record of organic-walled, morphologically distinct microfossils from the late Paleoproterozoic Changcheng Group in the Yanshan Range, North China, Precambrian Res., 321, 172–198, https://doi.org/10.1016/j.precamres.2018.11.019, 2019.
Miao, L., Moczydłowska, M., and Zhu, M.: A diverse organic-walled microfossil assemblage from the Mesoproterozoic Xiamaling Formation, North China, Precambrian Res., 360, 106235, https://doi.org/10.1016/j.precamres.2021.106235, 2021.
Moore, P. D., Webb, J. A., and Collinson, M. E.: Pollen Analysis, Second Edition, Blackwell Scientific Publications, Oxford, 216 pp., Blackwell Scientific Publications, ISBN: 0865428956, 1991.
Mukherjee, I., Large, R. R., Corkrey, R., and Danyushevsky, L. V.: The boring billion, a slingshot for complex life on Earth, Sci. Rep., 8, 4432, https://doi.org/10.1038/s41598-018-22695-x, 2018.
Mustafa, A., Ensikat, H.-J., and Weigend, M.: Mineralized trichomes in Boraginales: complex microscale heterogeneity and simple phylogenetic patterns, Ann. Bot., 121, 741–751, https://doi.org/10.1093/aob/mcx191, 2018.
Nan, J., King, H. E., Delen, G., Meirer, F., Weckhuysen, B. M., Guo, Z., Peng, X., and Plümper, O.: The nanogeochemistry of abiotic carbonaceous matter in serpentinites from the Yap Trench, western Pacific Ocean, Geology, 49, 330–334, https://doi.org/10.1130/G48153.1, 2021.
Nelson, M. P., Lücking, R., Boyce, C. K., Lumbsch, H. T., and Ree, R. H.: No support for the emergence of lichens prior to the evolution of vascular plants, Geobiology, 18, 3–13, https://doi.org/10.1111/gbi.12369, 2019.
O'Keefe, J. M. K., Mastalerz, M., Monaghan, G. W., Thompson, T. A., and Argyilan, E. P.: Transformation of wood in the dune environment, Annual Meeting of the Geological Society of America, Abstracts with Program, https://gsa.confex.com/gsa/2015AM/meetingapp.cgi/Paper/269410 (last access: 28 March 2023), 2015.
Payne, W. W.: A glossary of plant hair terminology, Brittonia, 30, 239–255, https://doi.org/10.2307/2806659, 1978.
Presswood, S. M., Rimmer, S. M., Anderson, K. B., and Filiberto, J.: Geochemical and petrographic alteration of rapidly heated coals from the Herrin (No. 6) Coal Seam, Illinois Basin, Int. J. Coal Geol., 165, 243–256, https://doi.org/10.1016/j.coal.2016.08.022, 2016.
Riding, J. B.: A guide to preparation protocols in palynology, Palynology, 45, 1–110, https://doi.org/10.1080/01916122.2021.1878305, 2021.
Salman, A., Tsror, L., Pomerantz, A., Moreh, R., Mordechai, S., and Huleihel, M.: FTIR spectroscopy for detection and identification of fungal phytopathogenes, Spectroscopy, 24, 261–267, https://doi.org/10.3233/SPE-2010-0448, 2010.
Santosh, M. and Groves, D. I.: The Not-So-Boring Billion: A metallogenic conundrum during the evolution from Columbia to Rodinia supercontinents, Earth-Sci. Rev., 236, 104287, https://doi.org/10.1016/j.earscirev.2022.104287, 2023.
Sazanova, K. V., Zelenskaya, M. S., Vlasov, A. D., Bobir, S. Y., Yakkonen, K. L., and Vlasov, D. Y.: Microorganisms in superficial deposits on the stone monuments in Saint Petersburg, Microorganisms, 10, 316, https://doi.org/10.3390/microorganisms10020316, 2022.
Shang, M., Tang, D., Shi, X., Zhou, L., Zhou, X., Song, H., and Jiang, G.: A pulse of oxygen increase in the early Mesoproterozoic ocean at ca. 1.57–1.56 Ga, Earth Planet. Sc. Lett., 527, 115797, https://doi.org/10.1016/j.epsl.2019.115797, 2019.
Shumlyanskyy, L., Franz, G., Glynn, S., Mytrokhyn, O., Voznyak, D., and Bilan, O.: Geochronology of granites of the western Korosten AMCG complex (Ukrainian Shield): implications for the emplacement history and origin of miarolitic pegmatites, Eur. J. Mineral., 33, 703–716, https://doi.org/10.5194/ejm-33-703-2021, 2021.
Spencer, C. J., Mitchell, R. N., and Brown, M.: Enigmatic mid-Proterozoic orogens: hot, thin, and low, Geophys. Res. Lett., 48, e2021GL093312, https://doi.org/10.1029/2021GL093312, 2021.
Tang, D., Shia, X., Wang, X., and Jiang, G.: Extremely low oxygen concentration in mid-Proterozoic shallow seawaters, Precambrian Res., 276, 145–157, https://doi.org/10.1016/j.precamres.2016.02.005, 2016.
Teng, J., Mastalerz, M., Liu, B., Gognat, T., Hauser, E., and McLaughlin, P.: Variations of organic matter transformation in response to hydrothermal fluids: Example from the Indiana part of the Illinois Basin, Int. J. Coal Geol., 219, 103410, https://doi.org/10.1016/j.coal.2020.103410, 2020.
Traverse, A.: Paleopalynology, 2nd Edn., Springer, Dordrecht, 813 pp., https://doi.org/10.1007/978-1-4020-5610-9, 2008.
Vajda, V., Cavalcante, L., Palmgren, K., Krüger, A., and Ivarsson, M.: Prototaxites reinterpreted as mega-rhizomorphs, facilitating nutrient transport in early terrestrial ecosystems, Can. J. Microbiol. 69, 17–31, https://doi.org/10.1139/cjm-2021-0358, 2022.
Weigend, M., Mustafa, A., and Ensikat, H.-J.: Calcium phosphate in plant trichomes: the overlooked biomineral, Planta, 247, 277–285, https://doi.org/10.1007/s00425-017-2826-1, 2018.
Westall, F., de Witt, M. J., Dann, J., van der Gaast, S., de Ronde, C. E. J., and Gerneke, D.: Early Archean fossil bacteria and biofilms in hydrothermally-influenced sediments from the Barberton greenstone belt, South Africa, Precambrian Res., 106, 93–116, https://doi.org/10.1016/S0301-9268(00)00127-3, 2001.