the Creative Commons Attribution 4.0 License.
the Creative Commons Attribution 4.0 License.
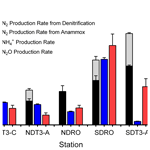
The fate of fixed nitrogen in Santa Barbara Basin sediments during seasonal anoxia
David J. Yousavich
Annie Bourbonnais
Frank Wenzhöfer
Felix Janssen
Tina Treude
David L. Valentine
Despite long-standing interest in the biogeochemistry of the Santa Barbara Basin (SBB), there are no direct rate measurements of different nitrogen transformation processes. We investigated benthic nitrogen cycling using in situ incubations with 15NO addition and quantified the rates of total nitrate (NO) uptake, denitrification, anaerobic ammonia oxidation (anammox), N2O production, and dissimilatory nitrate reduction to ammonia (DNRA). Denitrification was the dominant NO reduction process, while anammox contributed 0 %–27 % to total NO reduction. DNRA accounted for less than half of NO reduction except at the deepest station at the center of the SBB where NO concentration was lowest. NO availability and sediment total organic carbon content appeared to be two key controls on the relative importance of DNRA. The increasing importance of fixed N retention via DNRA relative to fixed N loss as NO deficit intensifies suggests a negative feedback loop that potentially contributes to stabilizing the fixed N budget in the SBB. Nitrous oxide (N2O) production as a fraction of total NO reduction ranged from 0.2 % to 1.5 %, which was higher than previous reports from nearby borderland basins. A large fraction of NO uptake was unaccounted for by NO reduction processes, suggesting that intracellular storage may play an important role. Our results indicate that the SBB acts as a strong sink for fixed nitrogen and potentially a net source of N2O to the water column.
- Article
(1440 KB) - Full-text XML
-
Supplement
(559 KB) - BibTeX
- EndNote
Oxygen minimum zones (OMZs) in the world's ocean, whether they are formed naturally or induced by human activities, have been expanding in the past century (Horak et al., 2016; Oschlies et al., 2017; Stramma et al., 2008). As oxygen (O2) concentration is one of the key controls on biogeochemical processes, including nitrogen (N) cycling, N biogeochemistry in OMZs has been extensively studied (Paulmier and Ruiz-Pino, 2009; Zehr, 2009). Denitrification, the reduction of nitrate (NO) to dinitrogen gas (N2), and anaerobic ammonia oxidation (anammox), where nitrite (NO) and ammonium (NH) are converted into N2 by comproportionation, are two major sinks of the oceanic fixed N budget (Gruber, 2008). These two processes are inhibited by the presence of O2 and sulfide, and their rates are sensitive to O2 at nanomolar concentrations (Dalsgaard et al., 2014; Joye and Hollibaugh, 1995; Caffrey et al., 2019). Because the last step of the sequential reduction of NO during denitrification, N2O reduction, is the most sensitive to O2 (Zumft, 1997), the production of nitrous oxide (N2O) as a byproduct of denitrification is usually elevated under hypoxic conditions, i.e., in the presence of O2 (Firestone et al., 1980; Ji et al., 2015). Additionally, nitrification, i.e., the oxidation of NH and subsequently NO, is another major source of N2O in the ocean (Elkins et al., 1978), and the relative yield of N2O from nitrification is high under low-O2 conditions (<4 µM) (Ji et al., 2018). Under O2 limitation, dissimilatory nitrate reduction to ammonia (DNRA) coupled to organic matter degradation is another important process that results in fixed N retention instead of removal (Burgin and Hamilton, 2007). When viewed as competing processes, DNRA is favored over denitrification under NO-limited conditions where electron donors are in excess (Tiedje et al., 1983). Additionally, under sulfidic conditions, autotrophic DNRA coupled to sulfide oxidation can become a dominant pathway for NO reduction (Shao et al., 2011).
The Santa Barbara Basin (SBB) is one of the borderland basins off the southern part of the coast of California and characterized by high export production (Thunell, 1998). Because the bottom water (maximum depth 586 m) in the SBB is separated from the area outside the basin by relatively shallow sills on the eastern end (∼200 m deep) and the western end (∼475 m deep), O2 concentrations at the basin's bottom are generally low and usually fluctuate between 1 and 30 µM (Bograd et al., 2002; Goericke et al., 2015; Reimers et al., 1990; Sholkovitz and Gieskes, 1971; Myhre et al., 2018). During upwelling seasons (winter and spring), water is advected from outside the basin and replenishes bottom water O2 in the SBB. However, high export production fuels O2 demand that maintains low O2 levels within the basin at depths below the deeper sill (Thunell, 1998). As a consequence, anoxia develops at the bottom of the SBB until the next upwelling event (Goericke et al., 2015), and large coverage of bacterial mats on the sea floor has been reported in the SBB (Valentine et al., 2016).
Using water column NO concentration data collected in the SBB by the California Cooperative Oceanic Fisheries Investigations (CalCOFI) along longitudinal transects (Koslow et al., 2010), Valentine et al. (2016) estimated the benthic NO uptake rate to be as high as 11.7 mmol m−2 d−1, which was one of the highest rates ever reported. However, the fate of the NO in the sediments remains unclear as there are no direct rate measurements of N cycling processes in the SBB. Indirect estimates using analysis of stable isotopes of water column NO suggest that benthic denitrification accounts for >75 % of NO loss in the SBB, and the rates of benthic denitrification were estimated to be the highest among borderland basins in the eastern tropical North Pacific (Sigman et al., 2003). Benthic anammox is expected to occur in the SBB (Prokopenko et al., 2006), but the relative contribution of denitrification and anammox to N2 production has not been assessed. In addition to different dissimilatory processes that reduce NO, the apparent NO drawdown could also be attributed to intracellular storage by both prokaryotes and microbial eukaryotes (Kamp et al., 2015; Bernhard et al., 2012; Schulz et al., 1999). With respect to N2O, these other borderland basins are considered to be a weak sink (Townsend-Small et al., 2014). As the SBB stands out in terms of denitrification, it may be expected that SBB benthic cycling of N2O is also unique.
To decipher the fate of NO taken up by SBB sediments, we performed in situ incubations using benthic flux chambers with added 15NO along the bottom slope traversing north–south across the deeper portion of the SBB. By calculating the rates of N2 production by denitrification and anammox, total N2O production, and DNRA, we assess the overall rates of NO uptake and reduction rates. Accompanying geochemical data are used to explore the controls on the relative importance of NO retention via DNRA.
2.1 In situ incubations with benthic flux chambers
Remotely operated vehicle (ROV) Jason deployed automated benthic flux chambers (BFCs) and conducted sediment push coring at seven stations (Fig. 1) in the SBB along a southern and a northern depth and O2 gradient originating from the depocenter in the deepest point of the basin (Table 1). Station depth, latitude, and longitude were automatically generated by the Jason data processor using navigation data derived from the Doppler velocity log system and the ultrashort baseline positioning system. Bottom water O2 concentration was determined using a type 4831 O2 optode sensor (Aanderaa Data Instruments AS, Bergen, NO) on the ROV and calibrated against Winkler titration measurements of seawater collected from Niskin bottles (Qin et al., 2022). Bottom water was collected using Niskin bottles and stored frozen at −30 °C until lab analysis for nitrate (NO) concentration following the spectrophotometric method described by García-Robledo et al. (2014).
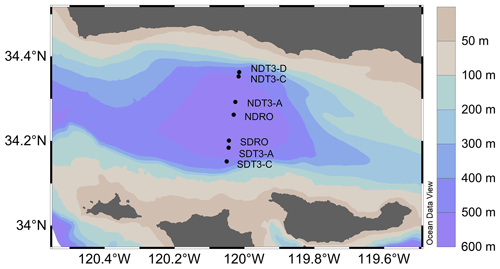
Figure 1Sampling stations in the Santa Barbara Basin. The color contours show bathymetry data from the General Bathymetric Chart of the Oceans at 30 arcsec resolution (Becker et al., 2009) visualized in Ocean Data View v5.6.2 (Schlitzer, 2002).
Table 1Sampling date, latitude, longitude, depth, bottom water concentrations of oxygen and nitrate, chamber volume, total organic carbon (TOC) and nitrogen (TON), and C : N molar ratio of organic matter in the top 2 cm of the sediment (by dry weight %) at the seven sampling stations in the Santa Barbara Basin. Oxygen concentrations below detection limit of the type 4831 (Aanderaa Data Instruments AS, Bergen, NO) oxygen optode sensor (3 µM) and the Winkler titration method (1 µM) are denoted by “bdl”. Note that oxygen concentrations in the bottom water at NDRO and SDRO were confirmed to be zero through additional analytical methods (see Yousavich et al., 2024).
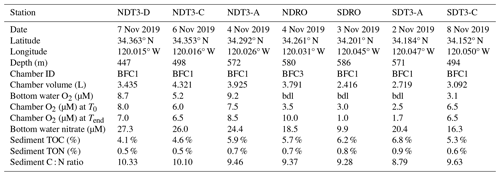
Sediment samples for total organic carbon (TOC) and total organic nitrogen (TON) analyses were subsampled from push cores (polycarbonate, 30.5 cm length, 6.35 cm inner diameter) retrieved by ROV Jason that were sectioned in 1 cm increments up to 10 cm followed by 2 cm increments below 10 cm (Yousavich et al., 2024). Wet sediments were dried for up to 48 h at 50 °C and treated with 6N HCl to dissolve carbonate minerals (Harris et al., 2001). Samples were then washed with ultrapure water and dried again at 50 °C. An aliquot (∼10–15 mg) was then packed into individual 8×5 mm pressed tin capsules and analyzed at the University of California Davis stable isotope facility using a PDZ Europa 20-20 isotope ratio mass spectrometer (Sercon Ltd., Cheshire, UK). TOC and TON were calculated based on the sample peak area corrected against a reference material (alfalfa flour). Molar concentrations, obtained from measured TOC and TON (in wt %), were used to calculate carbon-to-nitrogen (C : N) ratios.
The design of the BFCs has been described previously (Vonnahme et al., 2020). In brief, a stirred cylindrical polycarbonate chamber (inner diameter =19 cm) equipped with conductivity and oxygen sensors in the lid (type 5860 and 4330, respectively, Aanderaa Data Instruments AS, Bergen, NO) was inserted into the sediment to enclose a sediment patch of 284 cm2 together with 2.5 to 4.5 L of overlying water. The chambers were outfitted with a syringe sampler hosting one injection syringe and six sampling syringes to inject into and take samples from the overlying water at approximately 60 min intervals. The injection syringe contained 200 µmol of 15N-labeled potassium nitrate (Cambridge Isotopes) dissolved in 50 mL of deionized water. To minimize the introduction of O2, the 15N-labeled potassium nitrate solution was purged by ultra-high-purity helium at 5 mL min−1 for 60 min prior to being loaded into the injection syringe. The post-injection decrease in salinity in the chamber (as detected by the conductivity sensor) was used to calculate the volume of the benthic flux chamber (Kononets et al., 2021). Depending on the chamber volume, the total concentration of NO ranged between 50 and 100 µM at the beginning of in situ incubations. This level of NO amendment was intended to prevent its depletion before the end of incubations given the potentially high rates of NO uptake estimated by a previous study (Valentine et al., 2016).
After recovery, water samples from the BFC were transferred to evacuated 12 mL vials (Exetainer®, Labco, Lampeter, UK) pre-filled with 0.1 mL of 7 M zinc chloride for preservation. Prior to analysis of the isotopic compositions of N2 and N2O, a 5 mL sample was replaced with ultra-high-purity helium to create a headspace. The concentration and δ15N of dissolved N2 and N2O were determined using a Sercon CryoPrep gas concentration system interfaced to a Sercon 20-20 isotope ratio mass spectrometer (IRMS) at the University of California Davis Stable Isotope Facility. The measurement precision was ±0.2 ‰ for δ15N.
Water samples from the benthic flux chambers for analysis of 15NH were filtered through sterile 47 mm syringe filters (0.2 µm pore size) and frozen immediately. The production of 15NH in seawater samples was measured using a method adapted from Zhang et al. (2007) and described previously (Peng et al., 2016). In brief, NH was first oxidized to NO using hypobromite (BrO−) and then reduced to N2O using an acetic acid azide working solution (McIlvin and Altabet, 2005; Zhang et al., 2007). The δ15N of the produced N2O was determined using an Elementar Americas PrecisION continuous flow, multicollector, isotope ratio mass spectrometer (CF-MC-IRMS) coupled to a custom-built automated gas extraction and preparation system similar to the system described in McIlvin and Casciotti (2011). Calibration and correction were performed as described in Zhang et al. (2007). The measurement precision was ±0.2 ‰ for δ15N. NH solutions (10 µM) from a mixture of 99 % 15NH4Cl (Cambridge Isotopes) and IAEA standard N1 (δ15N =1.2 ‰) with a final δ15N of 135 ‰, 676 ‰, 1351 ‰, 5404 ‰, and 10 806 ‰ were prepared and used as in-house reference standards. The IRMS measurements of these in-house reference standards scaled linearly (R2=0.9996) with their δ15N values.
2.2 Rate calculations and statistics
Production rates of 29N2, 30N2, 15NH, and total N2O were calculated from the slope of the concentrations of the respective species at the syringe sampling time points by fitting a linear regression multiplied by the overlying water column volume and divided by the chamber area. The linear regressions excluded the last one or two sampling time points if they clearly deviated from a linear trend compared to the first four or five sampling time points. The rates of N2 production from denitrification and anammox were calculated following a previously described method (Thamdrup and Dalsgaard, 2002) with modifications to account for coupled DNRA–anammox (Peng et al., 2021). The calculation was set up with denitrification rate (RDN) and anammox rate (RAMX) as unknowns:
where P29 and P30 are the respective production rates of 29N2 and 30N2 that were calculated from measured concentrations stated above, fN is the fraction of 15N in the NO pool, and fA is the fraction of 15N in the NH pool. The solution for RDN and RAMX is
Errors calculated from the linear regression of 29N2 and 30N2 production rates were propagated to RDN and RAMX following established statistical methods (Deming, 1943). Detection limits of the calculated rates were estimated as double the standard deviation from linear regressions. Depending on the in situ NO concentration, the detection limit for total N2 production from denitrification and anammox ranged between 0.04 and 0.17 mmol m−2 d−1 and 0.04 and 0.24 mmol m−2 d−1 (Table S1 in the Supplement), respectively. The detection limit for N2O production ranged between 1.1 and 5.6 µmol m−2 d−1. DNRA rates were calculated as the rates of increase in 15NH divided by f15, where f15 is the fraction of 15N in the NO pool. Because part of the produced 15NH would be adsorbed to sediment minerals, the rates of 15NH production were further multiplied by a factor of 2 (De Brabandere et al., 2015; Laima, 1994). Depending on the in situ NH concentration, the detection limit for total NH production rates ranged between 0.01 and 0.07 mmol m−2 d−1 (Table S1).
3.1 Interpretation of rate measurements from benthic flux chamber incubations
The use of benthic flux chambers to perform 15NO incubation experiments in situ offers multiple advantages over other techniques such as slurry or whole-core incubations, including minimal disturbance of the sediment, maintenance of in situ pressure and temperature, and relatively large surface area which can account for spatial heterogeneity (Aller et al., 1998; Hall et al., 2007; Nielsen and Glud, 1996; Robertson et al., 2019). On the other hand, several limitations of using tracer incubations with benthic flux chambers can lead to either underestimated or overestimated rates. First, the diffusion of added 15NO into sediments and the labeled 15NO reduction products out of sediments in this study was unlikely at steady state. 15NO added to the overlying water of the chambers diffuses into sediment porewater where O2 is depleted within the first few millimeters, sustaining benthic NO reduction. However, a share of the labeled N compounds that are produced will diffuse to pore waters in deeper sediment layers and, hence, cannot be detected in samples taken from the overlying waters. This can lead to an underestimation of NO reduction rates.
Second, the addition of NO at concentrations that were 1.6–6.2 (median =2.3) times as high as ambient concentrations could lead to overestimation of rates. The NO uptake rates calculated as the decrease in total NO concentration over time was 1.9–6.4 (median =3.8) times higher than those measured in parallel chambers deployed at the same time without any added 15NO (Table S2; Yousavich et al., 2024). While the diffusive loss of NO to the sediment porewater is expected to account for the stimulated NO uptake partially, NO addition also likely stimulated the rates of NO reduction and intracellular storage. However, it remains unclear whether the accelerated NO uptake is partitioned between intracellular storage and reduction in the same proportion as under unamended conditions, which would partially depend on the carrying capacity of NO storage vs. reduction.
Third, the slight increase in O2 concentration in benthic chambers could have affected the rates of dissimilatory NO reduction and led to underestimates. O2 in bottom water (and, therefore, also in pore waters) was depleted (below detection of the Winkler titration method, 1 µM) at the deepest stations SDRO and NDRO (southern and northern depocenter radial origin, respectively; Table 1). O2 concentrations in the overlying water in most incubations were slightly increasing over the period of the incubation with an average rate of 0.11±0.44 µmol h−1. The increase is attributed to a release of O2 from the polycarbonate walls and lids of the chambers that were exposed to air until shortly before deployment. The net increase in O2 in the overlying water indicates that rates of O2 provision from the plastics were in most cases higher than the rates of O2 uptake by the enclosed sediment. A release of O2 from plastics has been reported by a previous study which showed rates of O2 provided from polycarbonate to O2-poor waters were among the highest of all plastics tested (Stevens, 1992). The extent to which the artificial elevation of O2 levels in the water overlaying the sediment in the chambers may have affected N transformation pathways and rates will depend on the O2 sensitivity of the respective processes and the penetration depth of O2 into the sediment. This effect was likely insignificant in our incubations in the SBB because the rate of O2 change was minimal compared to ambient O2 concentrations except for station NDRO, where O2 concentrations in the chamber water rose from below detection to 10 µM (Table 1). While there are limitations that can lead to both underestimates and overestimates, there is the possibility that they level each other out and our observations are close to in situ production rates of N2, N2O, and NH. Despite this concern, the relative contribution of different NO reduction processes and the general trend of NO reduction rates across the surveyed transect in the SBB are likely representative of in situ conditions.
The NO reduction rates measured in our experiments represent only the benthic contribution because the water samples in the six sampling syringes were subsampled simultaneously after recovery and no preservative was added inside the sampling syringe to terminate reactions. Therefore, we assume that NO reduction in the overlying water (and in the syringes after respective samples have been taken) contributed equally among all six samples to the production of N2, N2O, and NH and does not interfere with our rate calculations. Separate water incubations would be needed to determine the rates of NO reduction in the water column. To account for NH adsorption which could lead to an underestimate of DNRA, we made the assumption that an amount of 15NH that equals the measured increase in the benthic flux chambers is adsorbed to sediment minerals (Hall et al., 2017; Laima, 1994). The rates determined in this study were determined during seasonal anoxia when bottom water O2 was below detection at the depocenter of the basin. Additional expeditions are required to capture seasonal variations in these N cycling processes.
3.2 Denitrification was the dominant NO reduction pathway
On average, N2 production by denitrification and anammox was dominant over DNRA in this study, accounting for 70.4±16.4 % of total NO reduction (Fig. 2 and Table 2). Total N2 production rates ranged from 0.89 to 3.60 mmol N m−2 d−1, which were lower compared to a previous estimate (∼4.5 mmol N m−2 d−1) based on NO stable isotope mass balance calculations for the SBB (Sigman et al., 2003). Nevertheless, the previous estimate includes large uncertainties, and the rates calculated from stable isotope mass balance represent signals integrated over multiple seasons (Sigman et al., 2003), whereas our measurements represent snapshots obtained in one season of one year when the bottom water NO was not depleted. N2 production rates at seasons more depleted in NO concentrations in the bottom water compared to our study might more closely resemble rates estimated by Sigman et al. (2003). Season-resolving studies are needed in the future to understand the natural variability in the system and assess potential effects of stressors such as deoxygenation and rising temperature.
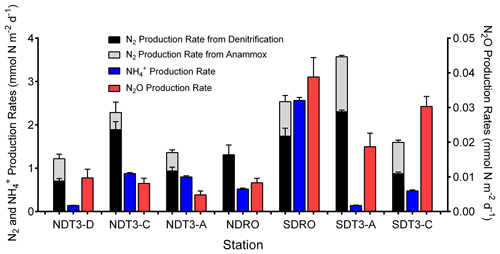
Figure 2Inorganic N-species production rates determined from 15N–NO labeling studies with in situ benthic flux chambers: N2 production rate from denitrification, N2 production rate from anammox, NH production rate, and N2O production rate. Note the lower range (right y axis) for N2O production. Error bars represent standard errors of the calculated slope from linear regressions of N2 and N2O production over time.
N2 production rates in this study were higher than most of those reported in other studies using in situ incubations with benthic flux chambers (Bonaglia et al., 2017; De Brabandere et al., 2015; Hall et al., 2017; van Helmond et al., 2020; Hylén et al., 2022). Elevated rates in the SBB are likely a result of the high organic matter content of sediment (4.1 %–6.8 % total organic carbon; Table 1), supporting high microbial respiration rates, and little (max 20 mm) to zero O2 penetration into the sediment (Yousavich et al., 2024). Compared to the SBB, organic matter content in sediment of previous studies, including the anoxic Eastern Gotland Basin (Hall et al., 2017), the largely pristine and oxygenated Gulf of Bothnia (Bonaglia et al., 2017), and an anoxic fjord basin in the By Fjord on the Swedish west coast (De Brabandere et al., 2015), was lower and the N2 production rates were typically <1 mmol N m−2 d−1. In comparison, N2 production rates reached 1.72±0.77 mmol N m−2 d−1 in the sediment underlying eutrophic waters of the Stockholm archipelago, where organic matter content was similar to SBB sediment (6.3 % ) and O2 penetration depth was <4 mm (van Helmond et al., 2020). Additionally, benthic denitrification rates in the SBB (1.37±0.64 mmol N m−2 d−1) were similar to those reported from the Peruvian OMZ (1.31±0.60 mmol N m−2 d−1) where bottom water O2 was lower than 10 µM and the organic matter content was similar (up to 7.5 % TOC and 0.9 % TON) to that in SBB sediments (Bohlen et al., 2011; Henrichs and Farrington, 1984; Sommer et al., 2016).
Table 2The relative contribution of different processes (total N2 production, N2 from denitrification, N2 from anammox, NH from DNRA, and N2O production) to total NO reduction (upper part) and the relative contribution of total NO reduction to total NO uptake (lower part) in the Santa Barbara Basin. Total N2 production consists of N2 from denitrification and N2 from anammox. Total NO reduction consists of total N2 production, NH from DNRA, and N2O production. Total NO uptake consists of total NO reduction and other NO sinks (e.g., intracellular storage).
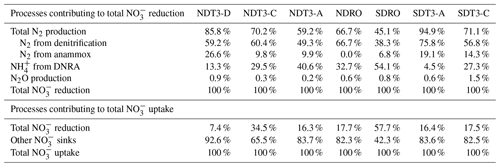
Benthic denitrification rates exceeded anammox rates at all sampling sites (Fig. 2 and Table 2). This relationship agrees with the paradigm that denitrification is typically favored over anammox in organic-rich sediments (Dalsgaard et al., 2005; Devol, 2015). Anammox bacteria can reduce NO to NO, which is then used to oxidize ammonia (NH3) to N2 (Kartal et al., 2007). In our in situ incubations, coupled DNRA–anammox in which DNRA produces a substrate (NH3) required by anammox could result in the production of 30N2 (Prokopenko et al., 2006), which is accounted for by our rate calculation method (detailed in Sect. 2.2). However, because the porewater NH concentration was high (>100 µM) (Yousavich et al., 2024), the fraction of 15N in the NH pool remained low (up to 2.1 % after ∼1 h of incubation and up to 4.3 % after 6 h of incubation). Therefore, the contribution of anammox to 30N2 production was below 2.0 % (Table S3). Overall, anammox contributed up to 26.6 % of NO reduction in the SBB (Table 2), indicating that anammox was a significant process in benthic SBB N cycling. Because the N isotope fractionation during the reduction of nitrite (NO to N2 by anammox bacteria () is lower than that of denitrification used for isotope mass balance calculations (∼25 ‰), anammox likely contributed to the lower-than-expected 15N enrichment in the SBB water column NO pool previously measured (Brunner et al., 2013; Sigman et al., 2003). When NO is not limiting, denitrification typically dominates as the denitrifier population has a shorter generation time than DNRA bacteria (Kraft et al., 2014).
3.3 NO availability and TOC control the relative importance of DNRA
The contribution of DNRA to total NO reduction was lower than denitrification at all stations except for the deepest station SDRO (Fig. 2), where NH production by DNRA contributed more than half of the NO reduction (Table 2). The relative contribution of DNRA to total NO reduction was positively correlated with TOC in the top 2 cm of the sediment (Fig. 3a) and negatively correlated with the bottom water NO concentration (Fig. 3b). These trends are consistent with previous findings showing that DNRA tends to be favored in environments with a high availability of electron donors such as organic carbon (Hardison et al., 2015; Kraft et al., 2014; Tiedje et al., 1983) and limited by NO (van den Berg et al., 2015; Kessler et al., 2018; Peng et al., 2016). Another example where DNRA dominated under limited NO availability is reported from measurements along a bottom water O2 and NO gradient traversing the Peruvian OMZ (Bohlen et al., 2011). One explanation for the increasing importance of DNRA under NO-limited conditions is that the growth yields calculated per mole electron acceptor from DNRA (consumes eight electrons) is higher than from denitrification (consumes five electrons) despite the greater amount of free energy provided by denitrification than DNRA per mole of NO, which was demonstrated by bacterial cultures capable of denitrification and DNRA (Strohm et al., 2007).
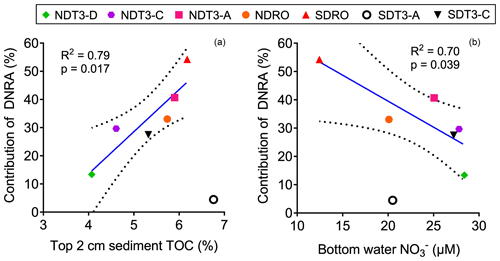
Figure 3The correlation between the contribution of DNRA to NO reduction (in %) and (a) the C : N ratio of sediment organic matter and (b) the bottom water NO concentration in the Santa Barbara Basin. Linear regressions were performed excluding one outlier from station SDT3-A. The solid line represents the best fit, and the dashed lines represent the 95 % confidence interval band.
The regressions we performed between the relative importance of DNRA vs. TOC and bottom water NO concentration excluded one data point from the station SDT3-A that deviated from the overall trend (Fig. 3). The DNRA rate at SDT3-A (0.14±0.005 mmol N m−2 d−1) was similarly low compared to NDT3-D (0.14±0.003 mmol N m−2 d−1), but the N2 production rates by both denitrification and anammox were the highest among all stations (Fig. 2), resulting in the lowest relative importance of DNRA. Porewater sulfide concentration was high at SDT3-A (Yousavich et al., 2024), so the DNRA bacteria should not be limited by the availability of electron donors. Sediments at SDT3-A were characterized by the highest TOC and TON content among all sites (Table 1), which may have fueled the highest rates of denitrification and anammox (Middelburg et al., 1996; Devol, 2015).
The frequency and magnitude of seasonal anoxia in the SBB have been increasing in the past 4 decades, which is expected to intensify fixed N loss and NO deficit in the water column (Goericke et al., 2015). Time-series measurements of water column NO revealed that bottom water NO depletion has become more frequent since 2003 compared to the time between 1986 and 2003. While seasonal flushing of the SBB not only oxygenates the bottom water but also increases bottom water NO, our results suggest that fixed N retention via DNRA will increase in response to NO drawdown even before NO is near depletion, which effectively forms a negative feedback that could potentially prevent the depletion of fixed N in the SBB. On the other hand, when NO is no longer limiting, perhaps due to slowdown of bottom water deoxygenation, the relative importance of DNRA would decrease, allowing denitrification to dominate NO reduction pathways.
3.4 N2O production and saturation
N2O production rates measured by in situ chamber incubations ranged from 4.8±1.1 to 38.8±5.6 µmol m−2 d−1 (Fig. 2). These rates were up to an order of magnitude higher than those measured using shipboard whole-core incubations (3.5±1.0 µmol m−2 d−1) with samples from a similar depth (544 m) in the anoxic part of the Soledad Basin (Townsend-Small et al., 2014). A recent study using in situ chamber incubations with 15NO in the Eastern Gotland Basin reported rates (∼15–68 µmol m−2 d−1) similar to or higher than the rates we measured in the SBB (Hylén et al., 2022). Because the physicochemical context of the Soledad Basin is more similar to the SBB than the Eastern Gotland Basin, we expected the N2O production rates in the Soledad Basin to be close to those in the SBB. The much lower N2O production rates reported from the Soledad Basin may be partially attributed to the whole-core incubations that were not performed in situ.
N2O production as a fraction of total NO reduction ranged from 0.2 % to 1.5 % (Table 2), which fell in the typical range of N2O yield from both nitrification and denitrification (Ji et al., 2015, 2018). Although our measurements do not allow the distinction between N2O production from nitrification and denitrification, it is likely that both processes contributed with the respective share depending on ambient O2 concentration. At the deepest stations where bottom water O2 was depleted (Table 1), denitrification was likely the main source of N2O. At other stations, where bottom water O2 ranged from 3.1–9.2 µM, nitrification likely also contributed to N2O production.
Although we observed N2O production in all in situ 15NO incubations, N2O concentration in the chambers at the start of the incubations was far below saturation level (9 %–12 %) at the two deepest stations SDRO and NDRO (Table S4). In contrast, N2O was either close to or above saturation at all other stations (Table S4). The low concentration of dissolved N2O at the two deepest stations is consistent with our finding that N2 production (i.e., N2O consumption) rates by denitrification were the highest there (Fig. 2), indicating that the deepest part of the SBB typically acts as a sink for N2O. The shallower parts of the SBB were characterized by a lower NO uptake rate (Table S2; Fig. S1), but they had a stronger potential for N2O production than the deepest stations (Fig. S2). In the case of a eutrophication event, enhanced surface primary productivity could stimulate denitrification as well as N2O production in the shallower parts of the SBB where bottom water O2 is not depleted and where benthic N2O production is more likely to contribute to N2O efflux from the water column during upwelling events.
3.5 Total NO uptake suggests high potential for intracellular NO storage
Although the N2 production rates we measured in the SBB were among the highest reported values for any marine sediments, total NO reduction, which also includes DNRA and N2O production, only accounted for 23.9±16.9 % of the total NO uptake in benthic flux chambers amended with 15NO (Table 2). Intracellular NO storage by bacteria and microbial eukaryotes was likely responsible for the majority of the NO uptake unaccounted for by the different NO reduction pathways. Marine Beggiatoa spp. can hyper-accumulate NO intracellularly at concentrations 3000- to 4000-fold above ambient levels (McHatton et al., 1996). Other microbial lineages including Thioploca, foraminifera, and Gromiida are also known to store NO intracellularly (Piña-Ochoa et al., 2010; Zopfi et al., 2001). In two of the porewater profiles sampled during the same cruise, NO concentrations at 1 cm depth reached 80–390 µM, which we interpreted as evidence of NO leakage from bacterial cells during porewater handling (Yousavich et al., 2024). While it is difficult to directly constrain the contribution of intracellular NO storage to total NO uptake, it can be indirectly inferred by calculating the diffusive loss (both upward and downward) of added 15NO if porewater concentrations in sediments underlying the benthic flux chamber were available.
The total NO uptake in the SBB measured from parallel benthic flux chambers without substrate amendment at the same stations (3.26±0.72 mmol N m−2 d−1) (Yousavich et al., 2024) was higher than that in other nearby borderland basins such as the San Nicolas Basin (0.38±0.03 mmol N m−2 d−1), the San Pedro Basin (0.78±0.11 mmol N m−2 d−1) (Berelson et al., 1987), and the Santa Monica Basin (1.10±0.31 mmol N m−2 d−1) (Jahnke, 1990). As mentioned above (Sect. 3.1), the addition of 15NO stimulated NO uptake rates by multiple folds (compared to BFC incubations without 15NO additions) and to a level (11.60±4.15 mmol N m−2 d−1) similar to a previous estimate (11.7 mmol N m−2 d−1) based on water column NO deficit (Valentine et al., 2016). Since bottom water NO during our sampling time (>12.5 µM in November 2019) was not as depleted as in October 2013 (∼2 µM NO) (Valentine et al., 2016), these results indicate that the microbial community in SBB sediments have the metabolic potential to further consume NO when SBB bottom water undergoes extended periods (months) of anoxia during autumn and winter. Assuming that NO in the lowermost 10 m of the water column is under the direct influence of benthic NO uptake, we estimate it would take between 1 to 4 months to deplete bottom water NO with a starting concentration of 30 µM, with the shortest depletion time at the depocenter and the longest at the periphery of the SBB. This timescale agrees with time-series measurements of water column NO concentrations in the SBB (Goericke et al., 2015), and it implies that bottom water NO is unlikely to become depleted at depths shallower than 500 m. Furthermore, we identified a significant negative correlation between NO uptake rates without substrate amendments and the fold change after 15NO addition (Fig. S3). This negative correlation indicates that benthic NO uptake rates at the shallow stations were the most responsive to exogenous NO supply, while on the other hand NO uptake rates at the deep and anoxic stations were closer to an upper limit that is determined by the microbial community present in the SBB sediments.
We investigated benthic nitrogen cycling processes using in situ incubations with 15NO addition and quantified the rates of total NO uptake, denitrification, anammox, N2O production, and DNRA. Our results indicate the role of the SBB sediments as a strong sink for fixed N. Denitrification was the dominant NO reduction process (38 %–76 %), while anammox contributed up to 27 %. DNRA accounted for less than half of NO reduction except at the deepest station (586 m) at the center of the SBB, where bottom water O2 concentrations were zero. The elevated relative importance of DNRA under high TOC and low NO conditions suggests that the fixed N loss in the SBB, especially during seasons of high surface primary productivity and thus high export production, could potentially be balanced by the N retention pathway. The higher N2O production measured in this study compared to nearby borderland basins may have stemmed from the use of benthic chambers instead of whole-core incubations, which highlights the advantage of in situ incubations in determining benthic N cycling processes. The high potential and relative importance of intracellular NO storage implied by our data pose a challenge to fully constrain the fixed N budget in the SBB, but it also presents an opportunity for future investigations targeting intracellular storage. Future intensification of water column anoxia may elevate the importance of fixed N retention via DNRA by keeping N in the system as NH, forming negative feedback that could overall reduce fixed N loss in the SBB.
The rate data in tabular form are available at https://doi.org/10.6084/m9.figshare.21824610.v1 (Peng, 2023).
The supplement related to this article is available online at: https://doi.org/10.5194/bg-21-3041-2024-supplement.
XP, TT, and DLV designed the study. XP, DJY, FW, FJ, TT, and DLV participated in the fieldwork. XP, DJY, AB, FW, and FJ performed the measurements. XP wrote the manuscript. All authors contributed to the writing of the manuscript and discussion of the data.
At least one of the (co-)authors is a member of the editorial board of Biogeosciences. The peer-review process was guided by an independent editor, and the authors also have no other competing interests to declare.
Publisher’s note: Copernicus Publications remains neutral with regard to jurisdictional claims made in the text, published maps, institutional affiliations, or any other geographical representation in this paper. While Copernicus Publications makes every effort to include appropriate place names, the final responsibility lies with the authors.
This article is part of the special issue “Low-oxygen environments and deoxygenation in open and coastal marine waters”. It is not associated with a conference.
We thank the captain, crew, and scientific party of the R/V Atlantis and the crew of the ROV Jason for their technical and logistical support during the research expedition AT42-19. We also thank De’Marcus Robinson, Sebastian Krause, Qianhui Qin, Eleanor Arrington, Megan O’Beirne, Aran Mazariegos, Xiadani Moreno, Alec Eastman, Hailie Kittner, Shey Dorji, Joshua Burgos-Ponce, Na Liu, Jonathan Tarn, and Kelsey Gosselin for assisting with shipboard analyses.
This research has been supported by the US National Science Foundation, NSF OCE-1756947 and OCE-1830033 (to David L. Valentine) and OCE- 1829981 (to Tina Treude); Danish National Research Foundation DNRF145 – HADAL (to Frank Wenzhöfer); the Simons Foundation Postdoctoral Fellowship in Marine Microbial Ecology (no. 547606 to Xuefeng Peng); and the Simons Foundation Early Career Investigator in Aquatic Microbial Ecology and Evolution Award to Xuefeng Peng.
This paper was edited by Marilaure Grégoire and reviewed by Mindaugas Zilius and one anonymous referee.
Aller, R. C., Hall, P. O. J., Rude, P. D., and Aller, J. Y.: Biogeochemical heterogeneity and suboxic diagenesis in hemipelagic sediments of the Panama Basin, Deep-Sea Res. Pt. I, 45, 133–165, https://doi.org/10.1016/S0967-0637(97)00049-6, 1998.
Becker, J. J., Sandwell, D. T., Smith, W. H. F., Braud, J., Binder, B., Depner, J., Fabre, D., Factor, J., Ingalls, S., Kim, S.-H., Ladner, R., Marks, K., Nelson, S., Pharaoh, A., Trimmer, R., Von Rosenberg, J., Wallace, G., and Weatherall, P.: Global Bathymetry and Elevation Data at 30 Arc Seconds Resolution: SRTM30_PLUS, Mar. Geodesy, 32, 355–371, https://doi.org/10.1080/01490410903297766, 2009.
Berelson, W. M., Hammond, D. E., and Johnson, K. S.: Benthic fluxes and the cycling of biogenic silica and carbon in two southern California borderland basins, Geochim. Cosmochim. Ac., 51, 1345–1363, https://doi.org/10.1016/0016-7037(87)90320-6, 1987.
Bernhard, J. M., Casciotti, K. L., McIlvin, M. R., Beaudoin, D. J., Visscher, P. T., and Edgcomb, V. P.: Potential importance of physiologically diverse benthic foraminifera in sedimentary nitrate storage and respiration, J. Geophys. Res.-Biogeo., 117, G03002, https://doi.org/10.1029/2012JG001949, 2012.
Bograd, S. J., Schwing, F. B., Castro, C. G., and Timothy, D. A.: Bottom water renewal in the Santa Barbara Basin, J. Geophys. Res.-Oceans, 107, 9-1–9-9, https://doi.org/10.1029/2001JC001291, 2002.
Bohlen, L., Dale, A. W., Sommer, S., Mosch, T., Hensen, C., Noffke, A., Scholz, F., and Wallmann, K.: Benthic nitrogen cycling traversing the Peruvian oxygen minimum zone, Geochim. Cosmochim. Ac., 75, 6094–6111, https://doi.org/10.1016/j.gca.2011.08.010, 2011.
Bonaglia, S., Hylén, A., Rattray, J. E., Kononets, M. Y., Ekeroth, N., Roos, P., Thamdrup, B., Brüchert, V., and Hall, P. O. J.: The fate of fixed nitrogen in marine sediments with low organic loading: an in situ study, Biogeosciences, 14, 285–300, https://doi.org/10.5194/bg-14-285-2017, 2017.
Brunner, B., Contreras, S., Lehmann, M. F., Matantseva, O., Rollog, M., Kalvelage, T., Klockgether, G., Lavik, G., Jetten, M. S. M., Kartal, B., and Kuypers, M. M. M.: Nitrogen isotope effects induced by anammox bacteria, P. Natl. Acad. Sci. USA, 110, 18994–18999, https://doi.org/10.1073/pnas.1310488110, 2013.
Burgin, A. J. and Hamilton, S. K.: Have we overemphasized the role of denitrification in aquatic ecosystems? A review of nitrate removal pathways, Front. Ecol. Environ., 5, 89–96, https://doi.org/10.1890/1540-9295(2007)5[89:HWOTRO]2.0.CO;2, 2007.
Caffrey, J. M., Bonaglia, S., and Conley, D. J.: Short exposure to oxygen and sulfide alter nitrification, denitrification, and DNRA activity in seasonally hypoxic estuarine sediments, FEMS Microbiol. Lett., 366, fny288, https://doi.org/10.1093/femsle/fny288, 2019.
Dalsgaard, T., Thamdrup, B., and Canfield, D. E.: Anaerobic ammonium oxidation (anammox) in the marine environment, Res. Microbiol., 156, 457–464, https://doi.org/10.1016/j.resmic.2005.01.011, 2005.
Dalsgaard, T., Stewart, F. J., Thamdrup, B., Brabandere, L. D., Revsbech, N. P., Ulloa, O., Canfield, D. E., and DeLong, E. F.: Oxygen at Nanomolar Levels Reversibly Suppresses Process Rates and Gene Expression in Anammox and Denitrification in the Oxygen Minimum Zone off Northern Chile, mBio, 5, e01966-14, https://doi.org/10.1128/mBio.01966-14, 2014.
De Brabandere, L., Bonaglia, S., Kononets, M. Y., Viktorsson, L., Stigebrandt, A., Thamdrup, B., and Hall, P. O. J.: Oxygenation of an anoxic fjord basin strongly stimulates benthic denitrification and DNRA, Biogeochemistry, 126, 131–152, https://doi.org/10.1007/s10533-015-0148-6, 2015.
Deming, W. E.: Statistical adjustment of data, Wiley, 1943.
Devol, A. H.: Denitrification, Anammox, and N2 Production in Marine Sediments, Annu. Rev. Mar. Sci., 7, 403–423, https://doi.org/10.1146/annurev-marine-010213-135040, 2015.
Elkins, J. W., Wofsy, S. C., McElroy, M. B., Kolb, C. E., and Kaplan, W. A.: Aquatic sources and sinks for nitrous oxide, Nature, 275, 602–606, https://doi.org/10.1038/275602a0, 1978.
Firestone, M. K., Firestone, R. B., and Tiedje, J. M.: Nitrous Oxide from Soil Denitrification: Factors Controlling Its Biological Production, Science, 208, 749–751, https://doi.org/10.1126/science.208.4445.749, 1980.
García-Robledo, E., Corzo, A., and Papaspyrou, S.: A fast and direct spectrophotometric method for the sequential determination of nitrate and nitrite at low concentrations in small volumes, Mar. Chem., 162, 30–36, https://doi.org/10.1016/j.marchem.2014.03.002, 2014.
Goericke, R., Bograd, S. J., and Grundle, D. S.: Denitrification and flushing of the Santa Barbara Basin bottom waters, Deep-Sea Res. Pt. II, 112, 53–60, https://doi.org/10.1016/j.dsr2.2014.07.012, 2015.
Gruber, N.: The marine nitrogen cycle: overview and challenges, Nitrogen in the Marine Environment, 2, 1–50, 2008.
Hall, P. O. J., Brunnegård, J., Hulthe, G., Martin, W. R., Stahl, H., and Tengberg, A.: Dissolved organic matter in abyssal sediments: Core recovery artifacts, Limnol. Oceanogr., 52, 19–31, https://doi.org/10.4319/lo.2007.52.1.0019, 2007.
Hall, P. O. J., Almroth Rosell, E., Bonaglia, S., Dale, A. W., Hylén, A., Kononets, M., Nilsson, M., Sommer, S., van de Velde, S., and Viktorsson, L.: Influence of Natural Oxygenation of Baltic Proper Deep Water on Benthic Recycling and Removal of Phosphorus, Nitrogen, Silicon and Carbon, Front. Mar. Sci., 4, 27, https://doi.org/10.3389/fmars.2017.00027, 2017.
Hardison, A. K., Algar, C. K., Giblin, A. E., and Rich, J. J.: Influence of organic carbon and nitrate loading on partitioning between dissimilatory nitrate reduction to ammonium (DNRA) and N2 production, Geochim. Cosmochim. Ac., 164, 146–160, https://doi.org/10.1016/j.gca.2015.04.049, 2015.
Harris, D., Horwáth, W. R., and van Kessel, C.: Acid fumigation of soils to remove carbonates prior to total organic carbon or CARBON-13 isotopic analysis, Soil Sci. Soc. Am. J., 65, 1853–1856, https://doi.org/10.2136/sssaj2001.1853, 2001.
Henrichs, S. M. and Farrington, J. W.: Peru upwelling region sediments near 15° S. 1. Remineralization and accumulation of organic matter1, Limnol. Oceanogr., 29, 1–19, https://doi.org/10.4319/lo.1984.29.1.0001, 1984.
Horak, R. E. A., Ruef, W., Ward, B. B., and Devol, A. H.: Expansion of denitrification and anoxia in the eastern tropical North Pacific from 1972 to 2012, Geophys. Res. Lett., 43, 2016GL068871, https://doi.org/10.1002/2016GL068871, 2016.
Hylén, A., Bonaglia, S., Robertson, E., Marzocchi, U., Kononets, M., and Hall, P. O. J.: Enhanced benthic nitrous oxide and ammonium production after natural oxygenation of long-term anoxic sediments, Limnol. Oceanogr., 67, 419–433, https://doi.org/10.1002/lno.12001, 2022.
Jahnke, R. A.: Early diagenesis and recycling of biogenic debris at the seafloor, Santa Monica Basin, California, J. Marine Res., 48, 413–436, 1990.
Ji, Q., Babbin, A. R., Jayakumar, A., Oleynik, S., and Ward, B. B.: Nitrous oxide production by nitrification and denitrification in the Eastern Tropical South Pacific oxygen minimum zone, Geophys. Res. Lett., 42, 10755–10764, https://doi.org/10.1002/2015GL066853, 2015.
Ji, Q., Buitenhuis, E., Suntharalingam, P., Sarmiento, J. L., and Ward, B. B.: Global Nitrous Oxide Production Determined by Oxygen Sensitivity of Nitrification and Denitrification, Global Biogeochem. Cy., 32, 1790–1802, https://doi.org/10.1029/2018GB005887, 2018.
Joye, S. B. and Hollibaugh, J. T.: Influence of Sulfide Inhibition of Nitrification on Nitrogen Regeneration in Sediments, Science, 270, 623–625, https://doi.org/10.1126/science.270.5236.623, 1995.
Kamp, A., Høgslund, S., Risgaard-Petersen, N., and Stief, P.: Nitrate Storage and Dissimilatory Nitrate Reduction by Eukaryotic Microbes, Front. Microbiol., 6, 1492, https://doi.org/10.3389/fmicb.2015.01492, 2015.
Kartal, B., Kuypers, M. M. M., Lavik, G., Schalk, J., Op den Camp, H. J. M., Jetten, M. S. M., and Strous, M.: Anammox bacteria disguised as denitrifiers: nitrate reduction to dinitrogen gas via nitrite and ammonium, Environ. Microbiol., 9, 635–642, https://doi.org/10.1111/j.1462-2920.2006.01183.x, 2007.
Kessler, A. J., Roberts, K. L., Bissett, A., and Cook, P. L. M.: Biogeochemical Controls on the Relative Importance of Denitrification and Dissimilatory Nitrate Reduction to Ammonium in Estuaries, Global Biogeochem. Cy., 32, 1045–1057, https://doi.org/10.1029/2018GB005908, 2018.
Kononets, M., Tengberg, A., Nilsson, M., Ekeroth, N., Hylén, A., Robertson, E. K., van de Velde, S., Bonaglia, S., Rütting, T., Blomqvist, S., and Hall, P. O. J.: In situ incubations with the Gothenburg benthic chamber landers: Applications and quality control, J. Marine Syst., 214, 103475, https://doi.org/10.1016/j.jmarsys.2020.103475, 2021.
Koslow, J., Goericke, R., McClatchie, S., Vetter, R., and Rogers-Bennett, L.: The California Cooperative Oceanic Fisheries Investigations (CalCOFI): the continuing evolution and contributions of a 60-year ocean observation program, 2010.
Kraft, B., Tegetmeyer, H. E., Sharma, R., Klotz, M. G., Ferdelman, T. G., Hettich, R. L., Geelhoed, J. S., and Strous, M.: The environmental controls that govern the end product of bacterial nitrate respiration, Science, 345, 676–679, https://doi.org/10.1126/science.1254070, 2014.
Laima, M. C. J.: Is KCl a reliable extractant of 15NH added to coastal marine sediments?, Biogeochemistry, 27, 83–95, https://doi.org/10.1007/BF00002812, 1994.
McHatton, S. C., Barry, J. P., Jannasch, H. W., and Nelson, D. C.: High Nitrate Concentrations in Vacuolate, Autotrophic Marine Beggiatoa spp, Appl. Environ. Microbiol., 62, 954–958, https://doi.org/10.1128/aem.62.3.954-958.1996, 1996.
McIlvin, M. R. and Altabet, M. A.: Chemical Conversion of Nitrate and Nitrite to Nitrous Oxide for Nitrogen and Oxygen Isotopic Analysis in Freshwater and Seawater, Anal. Chem., 77, 5589–5595, https://doi.org/10.1021/ac050528s, 2005.
McIlvin, M. R. and Casciotti, K. L.: Technical Updates to the Bacterial Method for Nitrate Isotopic Analyses, Anal. Chem., 83, 1850–1856, https://doi.org/10.1021/ac1028984, 2011.
Middelburg, J. J., Soetaert, K., Herman, P. M. J., and Heip, C. H. R.: Denitrification in marine sediments: A model study, Global Biogeochem. Cy., 10, 661–673, https://doi.org/10.1029/96GB02562, 1996.
Myhre, S. E., Pak, D., Borreggine, M., Kennett, J. P., Nicholson, C., Hill, T. M., and Deutsch, C.: Oxygen minimum zone biotic baseline transects for paleoceanographic reconstructions in Santa Barbara Basin, CA, Deep-Sea Res. Pt. II, 150, 118–131, https://doi.org/10.1016/j.dsr2.2017.12.009, 2018.
Nielsen, L. P. and Glud, R. N.: Denitrification in a coastal sediment measured in situ by the nitrogen isotope pairing technique applied to a benthic flux chamber, Mar. Ecol. Prog. Ser., 137, 181–186, https://doi.org/10.3354/meps137181, 1996.
Oschlies, A., Duteil, O., Getzlaff, J., Koeve, W., Landolfi, A., and Schmidtko, S.: Patterns of deoxygenation: sensitivity to natural and anthropogenic drivers, Philos. T. Roy. Soc. A, 375, 20160325, https://doi.org/10.1098/rsta.2016.0325, 2017.
Paulmier, A. and Ruiz-Pino, D.: Oxygen minimum zones (OMZs) in the modern ocean, Prog. Oceanogr., 80, 113–128, https://doi.org/10.1016/j.pocean.2008.08.001, 2009.
Peng, X.: Peng et al. 2023.xlsx, figshare [data set], https://doi.org/10.6084/m9.figshare.21824610.v1, 2023.
Peng, X., Ji, Q., Angell, J. H., Kearns, P. J., Yang, H. J., Bowen, J. L., and Ward, B. B.: Long-term fertilization alters the relative importance of nitrate reduction pathways in salt marsh sediments, J. Geophys. Res.-Biogeo., 121, 2082–2095, https://doi.org/10.1002/2016JG003484, 2016.
Peng, X., Ji, Q., Angell, J. H., Kearns, P. J., Bowen, J. L., and Ward, B. B.: Long-Term Fertilization Alters Nitrous Oxide Cycling Dynamics in Salt Marsh Sediments, Environ. Sci. Technol., 55, 10832–10842, https://doi.org/10.1021/acs.est.1c01542, 2021.
Piña-Ochoa, E., Høgslund, S., Geslin, E., Cedhagen, T., Revsbech, N. P., Nielsen, L. P., Schweizer, M., Jorissen, F., Rysgaard, S., and Risgaard-Petersen, N.: Widespread occurrence of nitrate storage and denitrification among Foraminifera and Gromiida, P. Natl. Acad. Sci. USA, 107, 1148–1153, https://doi.org/10.1073/pnas.0908440107, 2010.
Prokopenko, M. G., Hammond, D. E., Berelson, W. M., Bernhard, J. M., Stott, L., and Douglas, R.: Nitrogen cycling in the sediments of Santa Barbara basin and Eastern Subtropical North Pacific: Nitrogen isotopes, diagenesis and possible chemosymbiosis between two lithotrophs (Thioploca and Anammox) – “riding on a glider”, Earth Planet. Sc. Lett., 242, 186–204, https://doi.org/10.1016/j.epsl.2005.11.044, 2006.
Qin, Q., Kinnaman, F. S., Gosselin, K. M., Liu, N., Treude, T., and Valentine, D. L.: Seasonality of water column methane oxidation and deoxygenation in a dynamic marine environment, Geochim. Cosmochim. Ac., 336, 219–230, https://doi.org/10.1016/j.gca.2022.09.017, 2022.
Reimers, C. E., Lange, C. B., Tabak, M., and Bernhard, J. M.: Seasonal spillover and varve formation in the Santa Barbara Basin, California, Limnol. Oceanogr., 35, 1577–1585, https://doi.org/10.4319/lo.1990.35.7.1577, 1990.
Robertson, E. K., Bartoli, M., Brüchert, V., Dalsgaard, T., Hall, P. O. J., Hellemann, D., Hietanen, S., Zilius, M., and Conley, D. J.: Application of the isotope pairing technique in sediments: Use, challenges, and new directions, Limnol. Oceanogr.-Methods, 17, 112–136, https://doi.org/10.1002/lom3.10303, 2019.
Schlitzer, R.: Interactive analysis and visualization of geoscience data with Ocean Data View, Comput. Geosci., 28, 1211–1218, https://doi.org/10.1016/S0098-3004(02)00040-7, 2002.
Schulz, H. N., Brinkhoff, T., Ferdelman, T. G., Mariné, M. H., Teske, A., and Jørgensen, B. B.: Dense Populations of a Giant Sulfur Bacterium in Namibian Shelf Sediments, Science, 284, 493–495, https://doi.org/10.1126/science.284.5413.493, 1999.
Shao, M.-F., Zhang, T., Fang, H. H.-P., and Li, X.: The effect of nitrate concentration on sulfide-driven autotrophic denitrification in marine sediment, Chemosphere, 83, 1–6, https://doi.org/10.1016/j.chemosphere.2011.01.042, 2011.
Sholkovitz, E. R. and Gieskes, J. M.: A Physical-Chemical Study of the Flushing of the Santa Barbara Basin1, Limnol. Oceanogr., 16, 479–489, https://doi.org/10.4319/lo.1971.16.3.0479, 1971.
Sigman, D. M., Robinson, R., Knapp, A. N., van Geen, A., McCorkle, D. C., Brandes, J. A., and Thunell, R. C.: Distinguishing between water column and sedimentary denitrification in the Santa Barbara Basin using the stable isotopes of nitrate, Geochem. Geophy. Geosy., 4, 1040, https://doi.org/10.1029/2002GC000384, 2003.
Sommer, S., Gier, J., Treude, T., Lomnitz, U., Dengler, M., Cardich, J., and Dale, A. W.: Depletion of oxygen, nitrate and nitrite in the Peruvian oxygen minimum zone cause an imbalance of benthic nitrogen fluxes, Deep-Sea Res. Pt. I, 112, 113–122, https://doi.org/10.1016/j.dsr.2016.03.001, 2016.
Stevens, E. D.: Use of plastic materials in oxygen-measuring systems, J. Appl. Physiol., 72, 801–804, https://doi.org/10.1152/jappl.1992.72.2.801, 1992.
Stramma, L., Johnson, G. C., Sprintall, J., and Mohrholz, V.: Expanding Oxygen-Minimum Zones in the Tropical Oceans, Science, 320, 655–658, https://doi.org/10.1126/science.1153847, 2008.
Strohm, T. O., Griffin, B., Zumft, W. G., and Schink, B.: Growth Yields in Bacterial Denitrification and Nitrate Ammonification, Appl. Environ. Microb., 73, 1420–1424, https://doi.org/10.1128/AEM.02508-06, 2007.
Thamdrup, B. and Dalsgaard, T.: Production of N2 through Anaerobic Ammonium Oxidation Coupled to Nitrate Reduction in Marine Sediments, Appl. Environ. Microb., 68, 1312–1318, https://doi.org/10.1128/AEM.68.3.1312-1318.2002, 2002.
Thunell, R. C.: Particle fluxes in a coastal upwelling zone: sediment trap results from Santa Barbara Basin, California, Deep-Sea Res. Pt. II, 45, 1863–1884, https://doi.org/10.1016/S0967-0645(98)80020-9, 1998.
Tiedje, J. M., Sexstone, A. J., Myrold, D. D., and Robinson, J. A.: Denitrification: ecological niches, competition and survival, Antonie van Leeuwenhoek, 48, 569–583, https://doi.org/10.1007/BF00399542, 1983.
Townsend-Small, A., Prokopenko, M. G., and Berelson, W. M.: Nitrous oxide cycling in the water column and sediments of the oxygen minimum zone, eastern subtropical North Pacific, Southern California, and Northern Mexico (23° N–34° N), J. Geophys. Res.-Oceans, 119, 3158–3170, https://doi.org/10.1002/2013JC009580, 2014.
Valentine, D. L., Fisher, G. B., Pizarro, O., Kaiser, C. L., Yoerger, D., Breier, J. A., and Tarn, J.: Autonomous Marine Robotic Technology Reveals an Expansive Benthic Bacterial Community Relevant to Regional Nitrogen Biogeochemistry, Environ. Sci. Technol., 50, 11057–11065, https://doi.org/10.1021/acs.est.6b03584, 2016.
van den Berg, E. M., van Dongen, U., Abbas, B., and van Loosdrecht, M. C.: Enrichment of DNRA bacteria in a continuous culture, ISME J., 9, 2153–2161, https://doi.org/10.1038/ismej.2015.26, 2015.
van Helmond, N. A. G. M., Robertson, E. K., Conley, D. J., Hermans, M., Humborg, C., Kubeneck, L. J., Lenstra, W. K., and Slomp, C. P.: Removal of phosphorus and nitrogen in sediments of the eutrophic Stockholm archipelago, Baltic Sea, Biogeosciences, 17, 2745–2766, https://doi.org/10.5194/bg-17-2745-2020, 2020.
Vonnahme, T. R., Molari, M., Janssen, F., Wenzhöfer, F., Haeckel, M., Titschack, J., and Boetius, A.: Effects of a deep-sea mining experiment on seafloor microbial communities and functions after 26 years, Sci. Adv., 6, eaaz5922, https://doi.org/10.1126/sciadv.aaz5922, 2020.
Yousavich, D. J., Robinson, D., Peng, X., Krause, S. J. E., Wenzhöfer, F., Janssen, F., Liu, N., Tarn, J., Kinnaman, F., Valentine, D. L., and Treude, T.: Marine anoxia initiates giant sulfur-oxidizing bacterial mat proliferation and associated changes in benthic nitrogen, sulfur, and iron cycling in the Santa Barbara Basin, California Borderland, Biogeosciences, 21, 789–809, https://doi.org/10.5194/bg-21-789-2024, 2024.
Zehr, J. P.: New twist on nitrogen cycling in oceanic oxygen minimum zones, P. Natl. Acad. Sci. USA, 106, 4575–4576, https://doi.org/10.1073/pnas.0901266106, 2009.
Zhang, L., Altabet, M. A., Wu, T., and Hadas, O.: Sensitive Measurement of NH 15N/14N (δ15NH) at Natural Abundance Levels in Fresh and Saltwaters, Anal. Chem., 79, 5297–5303, https://doi.org/10.1021/ac070106d, 2007.
Zopfi, J., Kjær, T., Nielsen, L. P., and Jørgensen, B. B.: Ecology of Thioploca spp.: Nitrate and Sulfur Storage in Relation to Chemical Microgradients and Influence ofThioploca spp. on the Sedimentary Nitrogen Cycle, Appl. Environ. Microb., 67, 5530–5537, https://doi.org/10.1128/AEM.67.12.5530-5537.2001, 2001.
Zumft, W. G.: Cell biology and molecular basis of denitrification, Microbiol. Mol. Biol. Rev., 61, 533–616, 1997.