the Creative Commons Attribution 4.0 License.
the Creative Commons Attribution 4.0 License.
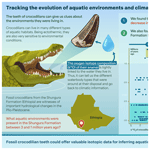
Stable oxygen isotopes of crocodilian tooth enamel allow tracking Plio-Pleistocene evolution of freshwater environments and climate in the Shungura Formation (Turkana Depression, Ethiopia)
Axelle Gardin
Emmanuelle Pucéat
Géraldine Garcia
Jean-Renaud Boisserie
Adélaïde Euriat
Michael M. Joachimski
Alexis Nutz
Mathieu Schuster
Olga Otero
This study adopts a new approach describing palaeohydrology and palaeoclimates based on the interpretation of stable oxygen isotopes (δ18Op) recorded in fossil crocodilian teeth. They represent an archive of prime interest for tracking freshwater palaeoenvironmental change, applicable to many palaeontological localities in the world: crocodilian teeth are abundant in continental basins and have been widely distributed since their diversification during the Mesozoic; the enamel phosphate is resistant to diagenesis and retains its original isotopic composition over geological timescales; and their δ18Op mainly relies on that of the crocodilian's home waterbody (δ18Ow), which in turn reflects waterbody types, regional climate, and evaporation conditions. This study presents the first application of this theoretical interpretative model to the Shungura Formation (Lower Omo Valley, Ethiopia), a key witness of the important environmental change in eastern Africa during the Plio-Pleistocene that impacted the evolution of regional faunas, including humans. In this complex and variable environmental context, the δ18Op of coexisting crocodilians allows for the fingerprinting of the diversity of aquatic environments they had access to at a local scale. This study sheds light on two important results: the δ18Op of crocodilian teeth (1) indicates stable aquatic environments in the northern Turkana Depression from 2.97 to ca. 2.57 Ma but a decline in local waterbodies diversity after 2.32 Ma, suggesting increasing aridity, and (2) shows, like previous geochemical studies on palaeosols and bivalves in the area, a significant increase in δ18Ow from 2.97 to ca. 1.14 Ma, likely due to the shifting air stream convergence zones between the West African and Indian Summer Monsoons and/or reduced rainfall over the Ethiopian Highlands.
- Article
(5680 KB) - Full-text XML
- BibTeX
- EndNote
Water availability is the main factor constraining the distribution of continental flora and fauna, in close connection to regional and local hydrography (climate, physiography of watersheds, geodynamics) (e.g. Bauder, 2005; Otero et al., 2011; Mushet et al., 2019; Smith and Boers, 2023). Freshwater environments, therefore, constitute vital components of regions that have yielded fossil hominid remains, but they are often overlooked and described in a more simplified way compared to terrestrial ones.
Over the last million years, in eastern Africa, the Turkana Depression experienced significant environmental change, particularly in terms of hydrology (e.g. aridification, landscape opening, lake level fluctuation); however new studies have recently challenged the previous models (e.g. level fluctuations in the palaeolake that occupied the Turkana Depression in Nutz et al., 2017, 2020). This region may have hosted a great diversity of types of freshwater environments (hereafter also called waterbodies, including channels, rivers, deltas, lakes, swamps, ponds, floodplains; Fig. 1), whose spatial distribution and evolution across time remain to be studied (Haesaerts et al., 1983; Nutz et al., 2017, 2020). The Shungura Formation (Fm) in south-western Ethiopia, located upstream of the delta of the Omo River flowing in Lake Turkana, indicates the continuous presence of water during the Plio-Pleistocene and is thus interpreted as a refuge area for wildlife (Bobe, 2006) (Fig. 1). Freshwater environments of the Shungura Fm have primarily been explored through sedimentological studies and the analysis of invertebrate assemblages (e.g. Van Damme and Gautier, 1972; Peypouquet et al., 1979; Peypouquet and Carbonel, 1980; de Heinzelin et al., 1983; Van Boxclaer and Van Damme, 2009). However, to date, only about 15 % of studies related to the Shungura Fm have addressed the aquatic component of its ecosystems. This might be mainly due to the terrestrial ecology of humans, which has led to a concentration on the terrestrial components of ecosystems. Nevertheless, it is essential to recognise the significance of aquatic environments in understanding the intricate interactions among climate, geodynamics, and aquatic communities. Therefore, there is a lack of studies, tools, and methodologies suitable for describing aquatic environments with the same precision as terrestrial environments. This scarcity of focus on aquatic environments in these contexts makes it difficult to obtain comprehensive palaeoenvironmental reconstructions.
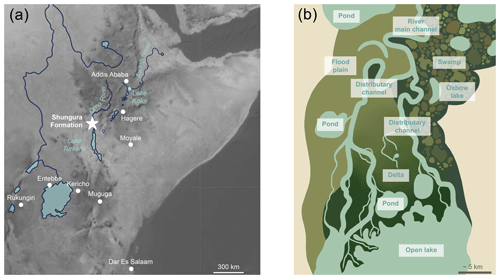
Figure 1Map showing location and schematic representation of freshwater environments expected in a lacustrine delta: (a) map displaying the geographical position of the Shungura Formation and Lake Turkana in the Turkana Depression in eastern Africa and of GNIP stations used in the study; (b) diagram illustrating the theoretical diversity of freshwater environments and types of waterbodies that may be present at a local scale in a lacustrine delta context, such as in the Turkana Depression.
In this context, the study of freshwater ectotherm vertebrates can provide original and valuable insights into the associated environmental conditions, such as hydrographic network connections, drainage characteristics, waterbody depth, salinity levels, temperatures, and seasonal climatic shifts (e.g. Otero et al., 2011). Such features differ from the environmental information gained through the study of terrestrial fauna, which are highly dependent on ecology.
The present study focuses on the oxygen isotopic composition of crocodilian teeth enamel phosphate (δ18Op) to track hydrological change over time. Crocodilian enamel apatite is an ideal palaeoenvironmental archive that can be used in multiple sites, as it is highly resistant to diagenetic and dissolution–precipitation processes (Kolodny et al., 1983, 1996; Shemesh et al., 1983; Lécuyer et al., 1999) and is found in abundance in continental sediments dating back to the expansion of this clade in the Jurassic, especially in African continental series (e.g. Arambourg et al., 1967; Tchernov, 1976, 1986; Markwick, 1998; Brochu et al., 2022). As described hereafter, crocodilians have a low metabolism and are aquatic; this means that the interpretation of their δ18Op is much more constrained because it mainly reflects δ18O of the waterbody (δ18Ow) they occupied during tooth formation (over several months). The linear relationship between δ18Op of crocodilian apatite and δ18Ow (Amiot et al., 2007) enables estimation of δ18Ow from δ18Op, thus providing valuable insights into aquatic environmental conditions. Previous analyses of δ18Op on crocodilians were systematically interpreted together with isotopic data from other sympatric vertebrates (fish, turtles, dinosaurs, mammals). This approach can help to infer the thermophysiology and ecology of taxa of interest (e.g. Amiot et al., 2010a), to estimate palaeotemperatures (e.g. Amiot et al., 2008) and aridity (Amiot et al., 2010b), to identify terrestrial or aquatic, freshwater or marine lifestyles (e.g. Goedert et al., 2020), or to differentiate among the water sources used by different co-existing taxa (Suarez et al., 2012).
This paper proposes a new theoretical interpretative model of (1) the range of δ18Op values recorded in crocodilian teeth to describe the diversity of waterbodies accessible to crocodilians at a local scale and (2) the evolution of minimum δ18O values over time, by using the comprehensive knowledge of the physiology and ecology of crocodilians. This approach is adapted and applied to fossil teeth from the Shungura Fm, representing an ideal framework for the first application, due to the continuous sedimentary record from ca. 3.75 to ca. 1.09 Ma (Boisserie et al., 2008; Table S1 in Gardin et al., 2023). The Shungura Fm reveals diverse aquatic environments related to lake level fluctuations, mainly fed by two monsoons, despite a complex, highly variable, and increasingly arid context (Haesaerts et al., 1983; Kebede and Travi, 2012; Nutz et al., 2020). The growing knowledge of the evolution of its environments, the continuity of the series over several million years, and its precise dating allow for discussion of the new isotopic data in a well-constrained framework. Because of disparate results across the various proxies, uncertainties remain about the timing and factors that induced major environmental changes and how they affected the evolution of fauna, including humans in the Plio-Pleistocene in the Shungura Fm and more widely in eastern Africa (e.g. Trauth et al., 2021, for a recent review).
By determining to what extent the δ18Op of crocodilians would allow tracking complex environmental changes in this region, this paper discusses (1) the diversity of aquatic environments occupied by crocodilians by interpreting the range of δ18Op values and (2) the variation in the δ18Op values as an indicator of local evaporation and δ18O values of meteoric waters. Building up on this discussion, guidelines are provided for further applications to other palaeontological and archaeological sites.
2.1 Study area and main information on the aquatic environments
The Omo Group, including the Shungura Fm, became a major reference for the Plio-Pleistocene biotic and environmental evolution thanks to the early work of the International Omo Research Expedition (IORE) from 1967 to 1976 (e.g. Howell, 1968; Coppens and Howell, 1976; de Heinzelin, 1983). The present study takes place within the framework of renewed fieldwork in the Shungura Fm and Usno Fm, enabling new palaeontological, archaeological, geological, and palaeoenvironmental analyses developed by the Omo Group Research Expedition (OGRE) on both IORE and new data. This research programme investigates biological evolution and its interactions with environmental factors at the local scale of the Lower Omo Valley (Boisserie et al., 2008). One approach favoured by the OGRE is to exploit palaeoenvironmental proxies directly linked to the continental palaeobiological record (e.g. Souron et al., 2012; the present study).
The Shungura Fm is exposed in the Lower Omo Valley (Fig. 2). With 800 m of alternating volcanic tuffs and fluvial, deltaic, and lacustrine fossil-bearing sediments (more than 57 000 specimens to date), this continental sedimentary succession is one of the most extensive and best-dated, documenting the evolution of ecosystems of the Lower Omo between ca. 3.75 and ca. 1.09 Ma (Fig. 2). The stratigraphic division into members and sub-members of the Shungura Formation defined by de Heinzelin (1983) is used in this paper, while keeping in mind that it has its limits at the scale of the formation. A revised age model including the latest absolute ages is provided in Table S1 in Gardin et al. (2023). For the last 5 million years, the Omo River, which also feeds Lake Turkana, mainly received sediments and water from the Ethiopian Highlands. The Shungura Fm recorded hydrological change over time: although it was dominated by a river system most of the time, the level of the palaeolake occupying the Turkana Depression rose until covering the site in the middle of Member G (2.06–1.95 Ma, Lorenyang highstand) and again at the top of Member L (1.19–1.09 Ma) (Haesaerts et al., 1983; Nutz et al., 2020). New fieldwork observations by the OGRE suggest that the Shungura Fm was under lacustrine influence from the Basal Member to unit B-8, maybe until B-10 (3.75–2.98 Ma, Lokochot highstand). Currently, the course of the Omo River is more incised and constrained due to the rift narrowing that occurred after 1.2 Ma (Nutz et al., 2022). Before, the Omo River migrated onto the area occupied at present-day by the Shungura Fm.
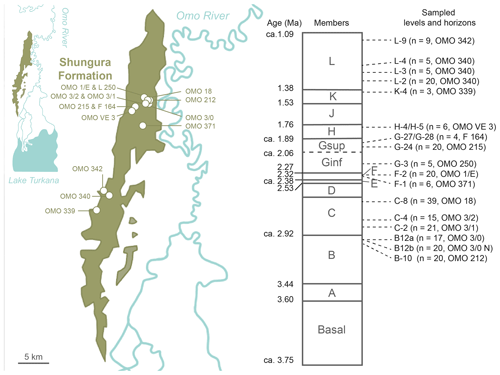
Figure 2Geographic location and chronostratigraphic column of the Shungura Formation, Lower Omo Valley, Ethiopia, and distribution in the map and the log of horizons where crocodilian teeth were sampled for this study. Geographic location of geochemical data from eastern African GNIP stations and water samples of Ethiopian rivers and lakes (map ESRI 2022).
This area underwent a general trend towards aridification in the Plio-Pleistocene, with the Lower Omo Valley becoming increasingly dominated by grasslands (e.g. Levin et al., 2011; Negash et al., 2015, 2020; Blondel et al., 2018, 2022). This trend was nevertheless locally buffered in the Lower Omo Valley, which maintained a more wooded environment than along the lake shores (Nachukui Fm and Koobi Fora Fm), at least until the end of the Lorenyang highstand (Levin et al., 2011). This was punctuated by periods of increased humidity and precipitation in the Ethiopian Highlands, which temporarily increased the Omo woody cover (Nutz et al., 2020). This may have made the Omo Valley a refuge for forest and woodland flora and fauna during this period of high climate variability (e.g. Bobe, 2006).
2.2 Plio-Pleistocene eastern African crocodilian diversity
The Shungura Fm yielded two distinct crocodilian teeth morphologies: elongated and anteroposteriorly curved teeth (hereafter referred to as pointed) and shorter and stouter, conical teeth (hereafter referred to as stout) (Fig. 3). This indicates the persistent presence of several crocodilian taxa over time, despite the local environmental changes. Three main genera of crocodilians have been identified in the Plio-Pleistocene outcrops of the Turkana Depression: the longirostrine and piscivorous Euthecodon and Mecistops and the generalist to brevirostrine Crocodylus (C. niloticus preys on all kind of prey and C. thorbjarnarsoni is the largest known species in this genus and probably fed on large mammals) (Arambourg et al., 1967; Tchernov 1976, 1986; Storrs, 2003; Brochu et al., 2010; Brochu and Storrs, 2012; Brochu, 2020). The pointed crocodilian teeth correspond to a piscivorous crocodilian such as Euthecodon and Mecistops, whereas the stout teeth could belong to Crocodylus and possibly Mecistops, too (molariform teeth at the back of the tooth rows). The different diets reflected by these two dental morphologies could induce different ecologies and therefore lead to a different oxygen isotopic signal as well, which was also tested here.
2.3 Sample collection
Two hundred thirty-eight (238) crocodilian teeth were collected from 16 units of the Shungura Fm dated to between ca. 2.97 and ca. 1.14 Ma (Tables 1 and S2, Fig. 2). The specimens were purposely collected for destructive analysis during the OGRE field missions in 2013 and 2016. To determine the minimum number of teeth required for a reliable representation of isotopic range of values, an approach based on data from level C-8 was employed chosen for its larger sample and its large range of the values. By applying the slope break criterion, a threshold of six teeth was established as optimal to stabilise the range measurement. However, for five levels, fewer teeth were available, and it is acknowledged that this requires increased caution in interpreting these results. All teeth with a minimum size of 1 cm in height were exhaustively collected (stout and pointed) from a large part of the Shungura series, each sample corresponding to a single locality (or part of a locality). This sampling strategy allows for exploration of the signal yielded by crocodilian teeth in units documenting major environmental transitions. Units K-4 and L-3 did not yield stout teeth, and no pointed teeth were present in unit L-9. The analyses presented were preferentially conducted on pointed teeth to build a comparable dataset along the series as they are most likely to be from piscivorous crocodilians and are particularly linked to aquatic environments. Teeth with bright enamel and without white alteration marks were selected for isotopic analyses. B-12a and B-12b are two spots in the same locality in lateral continuity, OMO 3/0 (ca. 2.94 Ma), separated by about 200 m, with B-12a being siltier and more stratified and B-12b coarser and with less structure. Some units are sometimes difficult to differentiate, and the elements collected on a surface can thus group two units (Tables 2 and S2, Fig. 2). On surface collections of this type, a little temporal mixing is therefore possible. The average height of “stout” teeth is approximately 1.8 cm, while “pointed” teeth have an average height of around 3.0 cm. A crocodile is typically considered large when it reaches a mass of over 100 kg and an estimated length of at least 2.8 m (Smith, 1979). Using approximate calculations based on photographs of skulls and live specimens, the minimum size of the crocodilians represented in the sample is approximately 2 m, but the majority likely exceed 2.8 m in length, classifying them as large individuals.
2.4 Sample preparation and analysis
For each tooth, enamel was sampled from base to apex of the crown to average potential seasonal variation in δ18Op using a micro-milling Dremel device and was then crushed in an agate mortar and pestle.
To isolate the phosphate group of enamel apatite (O'Neil et al., 1994; Wenzel et al., 2000), dissolved apatite was converted in Ag3PO4 following a method adapted from Joachimski et al. (2009). About 1 mg of enamel powder was weighed into small polyethylene beakers and dissolved overnight in 33 µL 2 M HNO3. The solution was neutralised with 33 µL 2 M KOH, and Ca2+ precipitated as CaF2 by adding 33 µL of 2 M HF. After centrifugation, the supernatant was transferred to a clean beaker and 0.5 ml of a silver amine solution was added in order to precipitate Ag3PO4 at 60 ∘C for 8 h. Silver phosphate crystals were rinsed several times with distilled water and dried at 40 ∘C for 30–60 min, and ground into powder in an agate mortar and pestle.
Between 0.2 and 0.3 mg of Ag3PO4 were weighed, placed in silver foil, and part of the sample set was analysed at the GeoZentrum Nordbayern of the Friedrich-Alexander University Erlangen-Nürnberg using a TC-EA (high-temperature elemental analyser) coupled online to a Thermo Scientific Delta V IRMS and the other part was analysed at the University of Burgundy (Dijon, France) using a high-temperature pyrolysis analyser (Elementar Pyrocube) connected online to an Elementar isoprime mass spectrometer. Samples were all combusted at 1450 ∘C and the generated CO gas was transferred in a helium stream to the mass spectrometer. Samples as well as standards measured over the course of analyses were generally measured in triplicate. All values are reported in per mille relative to V-SMOW. The average oxygen isotopic composition of NBS 120c standard was measured as 21.7 ‰ VSMOW. Accuracy was monitored by replicate analyses of NBS120c. Reproducibility was calculated based on triplicate sample and standard analyses and was generally < ±0.3 ‰ (based on 1 SD statistics).
Oxygen isotope analyses were additionally performed on the carbonate group of some powdered enamel samples (δ18Oc) to check for a possible effect of diagenesis on the sample isotopic composition. To this end, 0.10–0.13 mg of enamel powder were loaded in glass vials and reacted with a 102 % H3PO4 solution at 70 ∘C for 20 min in a Thermo Scientific™ Kiel VI carbonate preparation device coupled with a Thermo Scientific™ Delta V Plus™ IRMS at the Biogéosciences Laboratory of the University of Burgundy. δ18Oc data were reported in per mille relative to V-PDB (Vienna Pee Dee Belemnite) using certified international standard NBS19 (δ18O 2.20 ‰). External reproducibility was assessed by replicate analyses of NBS19 over the course of the analyses and was better than ±0.07 ‰ (2σ). Calculation of δ18Ow values from δ18Op of Shungura fossil crocodilian enamel was based on the fractionation equation of Amiot et al. (2007).
2.5 Statistical analyses
Means are presented with ±1 SD. Significance is evaluated at α= 0.05. Analyses were performed in R Version 4.2.2 (R Core Team, 2023). The δ18Op difference between stratigraphical units was investigated with a two-way ANOVA and pairwise Wilcoxon rank sum tests with Holm correction (non-parametric post hoc tests). The trend over time was identified using least-squares linear regression and reported as adjusted R2.
The isotopic composition of crocodilian enamel phosphate and the resulting estimated δ18Ow are summarised in Table 1 (full dataset in Table S2 in Gardin et al., 2023) and displayed in Figs. 4 and 8. δ18Op values range between 15.1 ‰ and 22.9 ‰, while δ18Oc values range between −8.2 ‰ and 1.2 ‰. The δ18Op is not statistically different between round and pointed teeth (ANOVA: F= 1.136, df= 8, p= 0.342) (Fig. 4). From B-10 to C-8, F-2, G-27/G-28, H-4/H-5, and K-4, the range of δ18Op values is greater than 2.3 ‰, while in the other units F-1, G-3 to G-24, and L-2 to L-9, the range of δ18Op values does not or barely exceeds this variation. Minimal δ18Op values increase from 15.3 ‰ in B-10 (2.97 Ma) to 18.6 ‰ in L-9 (ca. 1.14 Ma) (R2 = 0.49), corresponding to an increase in δ18Ow values from −6.6 ‰ to −3.5 ‰. However, this trend is nuanced when studying the isotopic signal between the stratigraphic units. The δ18Op values are particularly high in units F-2, G-24, L-2, and L-9, while units C-2 and C-8 yielded low δ18Op values (Table S3 in Gardin et al., 2023). The δ18Op values are statistically homogenous in the two sub-localities of unit B-12 (spatially close but with slightly different sedimentary facies, p= 0.12). After unit H-4/H-5 (1.84 Ma), the δ18Op values strongly increase and remain high.
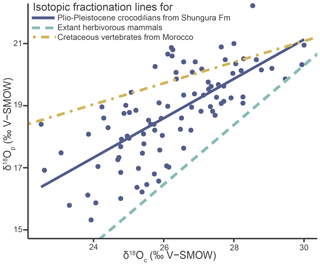
Figure 4Oxygen isotopic composition (mean ±1 SD) of the crocodilian teeth of the Shungura Formation, relative to the tooth morphotypes.
4.1 Preservation of the original oxygen isotopic composition
Tooth enamel has densely packed large apatite crystallites, which should best retain the primary δ18Op (Kolodny et al., 1983; Shemesh et al., 1983; Pucéat et al., 2004). However, alterations due to secondary apatite precipitation and diagenesis can still occur and the preservation of the original isotopic signal must be ensured before proceeding to palaeoenvironmental interpretations (Blake et al., 1997; Zazzo et al., 2004). Although no dual δ18O data exist for modern crocodilians or other sauropsids, which could differ from mammals, the data in this article are compared to those obtained from well-preserved modern mammals and Mesozoic vertebrates from Morocco (Iacumin et al., 1996; Amiot et al., 2010b). The fossil data from Amiot et al. (2010b) exhibit a lack of homogenisation in δ18O values and follow an expected linear relationship for at least partially preserved isotopic compositions. Applying the same approach to the crocodilians of the Shungura Formation demonstrates at least partial preservation of the original signal by exhibiting a linear relationship. While achieving an exact match can be challenging due to various factors, including differences in the clades' evolutionary history, the new dataset falls within the anticipated range for values considered “at least partially preserved” (adjusted R2 = 0.50; Spearman's correlation coefficient = 0.74) (Fig. 5) and thus supports reasonable interpretability. In addition, the lack of homogeneity (< 1 ‰ range) of δ18Op values within each unit further supports this view, reflecting the diversity of the life history of individuals and the good match of values to other geochemical studies dealing with modern mammals and ancient crocodilians (Iacumin et al., 1996; Amiot et al., 2010b). The δ18Op values of the teeth analysed in this work can be interpreted as a function of the evolution of the local aquatic environments and regional climate during the Plio-Pleistocene in the Turkana Depression.
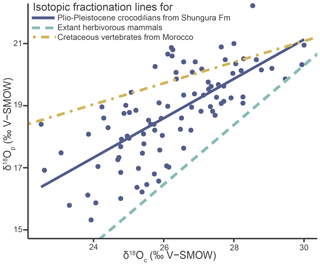
Figure 5Oxygen isotope compositions of enamel phosphate (δ18Op) from the studied crocodilians from the Shungura Formation plotted against their corresponding oxygen isotope composition of carbonate (δ18Oc), along with the phosphate–carbonate isotopic fractionation line established for Cretaceous vertebrates from Morocco (Amiot et al., 2010b) and extant herbivorous mammals (Iacumin et al., 1996).
4.2 Construction of the interpretation model of the δ18Op of a fossil crocodilian enamel in the Shungura Fm
4.2.1 How representative is the δ18Op of a fossil crocodilian tooth of the waterbody it used to live in?
To use the δ18Op of crocodilian enamel as a palaeoenvironmental proxy, it is first necessary to understand how the isotopic signal is constructed. The δ18Op of crocodilian enamel depends on the body temperature in the jaw where the enamel mineralises, which controls the amplitude of the isotopic fractionation between phosphate and body water, and on the isotopic composition of the body water, which is dependent on the balance between oxygen inputs and output fluxes (Kohn, 1996; Bryant and Froelich, 1995; Amiot et al., 2007) (Fig. 6).
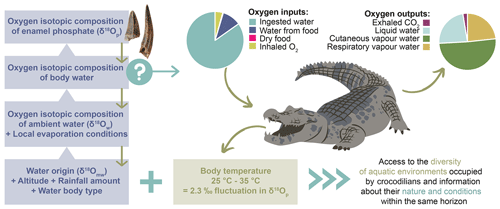
Figure 6Summary of the extrinsic (water origin, altitude, rainfall amount, evaporation) and intrinsic (oxygen outputs, body temperature) factors influencing the oxygen isotopic composition of the crocodilian enamel, including the main oxygen fluxes controlling the oxygen isotope composition of crocodilian body water.
Crocodilians have various sources of oxygen, including drinking water, water from food, oxygen from dry food, and inhaled O2. Oxygen outputs include liquid water losses (urine) and vapour water losses (cutaneous, oral, and nasal) as well as exhaled CO2 (Bryant and Froelich, 1995; Kohn, 1996). Rough estimates of the relative contribution of these oxygen fluxes can be made based on the existing literature on crocodilian physiology and ecology (Cott, 1960; Cloudsley-Thompson, 1968; Altman and Dittmer, 1972; Grigg, 1978; Glass and Johansen, 1979; Seymour et al., 1985, 2013; Davis et al., 1980; Mazzotti and Duson, 1989; Christian et al., 1996). Based on these studies, at 25 ∘C, 85 % of oxygen input comes from environmental water and most of the water output is vapour water losses (up to 75 %) (Fig. 6). Hence, the crocodilian body water δ18O is mainly determined by their drinking water, corresponding to the water in which crocodilians live (δ18Ow), and by body water evaporation (Fig. 6).
Crocodilians are ectothermic vertebrates, meaning their body temperature fluctuates with environmental conditions, which has an impact on the amplitude of the oxygen isotopic fractionation between body water and enamel apatite. However, they can somewhat regulate their body temperature through heat-seeking or -avoidance land–water movement and physiological adjustments (Smith, 1979; Lang, 1987a, b). The activity range for many extant species is 25–35 ∘C, but they tend to prefer a thermal gradient near the upper limit (35–37 ∘C) in tropical regions (Lang, 1987b; Markwick, 1998). Existing studies suggest that crocodilians have a high degree of thermoregulation, and their body temperature is relatively independent of water temperature, especially for large individuals (≥ 100 kg) (Smith, 1979; Lang, 1987b; Grigg et al., 1998; Seebacher et al., 1999). This is due to the convective transfer of heat decreasing and the metabolic production of heat increasing with body size. In this study, most of the sampled material consists of large fossil teeth, allowing us to assume that they may have belonged to large individuals with relatively stable and high body temperatures. Given the thermoregulation abilities of crocodilians, depending on the individuals, their behaviour, and their environment, the body temperature can be slightly different between individuals occupying the same waterbody and possibly greater between two populations and species occupying different aquatic environments (Amiot et al., 2007). The body temperature of crocodilians varies mainly within 10 ∘C, which approximately corresponds to a difference of ∼ 2.3 ‰ in δ18Op (Longinelli and Nuti, 1973; Kolodny et al., 1983; Pucéat et al., 2010) (Fig. 6). Amiot et al. (2007) did not identify any interspecific variation in δ18Op in captive crocodilians reared in the same water, but it may be due to the artificial environment they were in, which may not have allowed them to display the same diversity of behaviour as in the wild.
Dental development can also influence the construction of the isotopic signal, in particular the duration of enamel mineralisation, and time of year can influence the duration of recording of seasonal isotopic fluctuations in water. According to previous studies, tooth replacement in alligators occurs approximately once a year, with a range of 8 to 16 months, depending on the tooth's position in the dental row (Edmund, 1962). Other studies, such as those by Erickson (1992, 1996), based on dentin increment counting, estimate tooth formation time in both modern and fossil crocodilians to be between 83 and 285 d and it seems to increase with age and body size. Therefore, it is difficult to definitively state whether tooth formation occurs during a particular season, and it is important to note that crocodilians may estivate or hibernate, which can affect somatic and maybe dental growth (Chabreck and Joanen, 1979; Hutton, 1987; Taplin, 1988).
Crocodilians are relatively sedentary, they only migrate over short distances seasonally or to mate (a few tens of kilometres) (Lang, 1987a; Tucker et al., 1997; Swanepoel, 1999; Campbell et al., 2010; Beauchamp et al., 2018). Their fast-growing teeth thus record the oxygen isotopic composition of the waterbody or watershed they occupy. Despite the significant Plio-Pleistocene hydrological changes, several crocodilian species have persisted for millions of years in the Shungura Fm, likely due to their ability to adapt to a wide range of aquatic habitats. These include rivers, swamps, lakes, estuaries, and freshwater and saline waters (e.g. Isberg et al., 2019). The diversity of waterbodies occupied by past crocodilians thus likely reflects a significant part of the types of aquatic environments available at a local scale (Figs. 1 and 6).
4.2.2 Determinants of δ18O of Ethiopian waters
Rainfall amount, topography, distance from coastlines, temperature, and moisture source are usually invoked to explain the isotopic composition of meteoric waters (δ18Omw) in intertropical regions (Dansgaard, 1964; Rozanski et al., 1993; Levin et al., 2009). Moisture supply in the Ethiopian Highlands is determined by the seasonal migration of the Intertropical Convergence Zone (ITCZ) and the Congo Air Boundary (CAB) and comes from the Atlantic and Indian oceans and recycled continental moisture from the Congo Basin as well as possibly from southern lakes (Levin et al., 2009; Kebede and Travi, 2012). Table 2 shows that the oxygen isotopic composition of rainfall in eastern Africa at similar altitudes is not correlated to the quantity of precipitation. This indicates that other factors, such as the source of the moisture, are dominant.
Table 2Present-day geographic, meteorological, and geochemical data recorded from eastern African GNIP stations and surface water samples of Ethiopian rivers and lakes, placed on the map in Fig. 1a. Additional data from the Goma station are provided in Sect. 4.
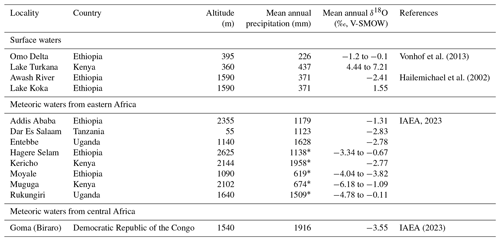
* Data are from Merkel (2023).
Currently, the Turkana Depression is a region with a hot and semi-arid climate, with less than 500 mm of annual precipitation and an annual average temperature of 29–30 ∘C (Merkel, 2023), a climate that does not explain the relatively large water availability in the basin. Lake Turkana is mainly fed by the Omo River, which originates in the Ethiopian Highlands, south-west of Addis Ababa (Schuster et al., 2022). The δ18Ow of the Omo River is influenced by the climate of this region, where moisture is maintained at 75 % by the Western African Monsoon, coming from the Atlantic Ocean and crossing the Congo basin (June–September, δ18Omw of −2.59 ‰ to −1.99 ‰ at Entebbe, west to Addis Ababa), and the rest by the Indian Summer Monsoon from the equatorial Indian Ocean (δ18Omw of −4.65 to −3.81 ‰ at Muguga, east to Addis Ababa) (Camberlin, 1997; Kebede and Travi, 2012). In regions with high levels of evapotranspiration, the δ18Omw values are generally similar or greater than the δ18O values of ocean source water due to the wholesale return of transpired water consumed by plants to the atmosphere (Levin et al., 2009). Therefore, the water vapour in the Congo basin results in rainfall with δ18Omw equal to or greater than that of the Atlantic Ocean. This 18O-enriched rainfall is the main contributing factor to the higher δ18Omw values recorded in Addis Ababa compared to other parts of eastern Africa (Table 1, Rozanski et al., 1996; Levin et al., 2009; Kebede and Travi, 2012). The Ethiopian and eastern African Dome form channels or obstacles for these low-level air streams, preventing the Indian Summer Monsoon from moving further west in the Ethiopian Highlands and the Atlantic and Congo moisture from moving further north and east (Levin et al., 2009). The elevation of the Ethiopian Dome, up to 4500 m, came to be well before the Plio-Pleistocene, so a change in topography seems unlikely to result in significant variation in δ18Omw in the context of the Shungura series (Abbate et al., 2015). Moreover, Passey et al. (2010) concluded that air temperature has not changed or only slightly decreased in the Turkana Depression over the past 3 million years and thus cannot explain the δ18Omw variation observed in this region.
Today, the average δ18Ow value at the Omo River Delta is between −1.2 ‰ and −0.1 ‰, while the value at Lake Turkana (endorheic) is between 4.4 ‰ and 7.2 ‰, with an 18O enrichment between 4.3 ‰ and 8.4 ‰ (Table 1) (Kebede and Travi, 2012; Vonhof et al., 2013). Lake Koka is an artificial and exorheic lake (δ18Ow= 1.55 ‰) with a minor 18O enrichment of 4.0 ‰ compared to its tributary, the Awash River (δ18Ow= −2.4 ‰) (Table 1) (Hailemichael et al., 2002). Surface area, evaporation, and drainage are potential determining factors of the isotopic composition of lakes (Kebede et al., 2009; Kebede and Travi, 2012). From the data of Lake Koka and the Awash River, one could expect an exorheic lake fed by the Omo River to have a δ18Ow on the order of 2 ‰ to 4 ‰. These values will be compared to the Plio-Pleistocene δ18Ow values estimated from the δ18Op of Shungura crocodilian enamel. Calculation of δ18Ow values from δ18Op of Shungura fossil crocodilian enamel is based on the fractionation equation of Amiot et al. (2007). Note that this fractionation equation mainly relies on isotopic data from extant modern captive crocodilians, which form their teeth in humid greenhouse contexts, potentially overestimating the inferred values. In their natural environment, large cutaneous and respiratory evaporation may lead to an 18O enrichment of crocodilians' body water relative to their environmental water, which is not considered in the equation (Amiot et al., 2007).
4.2.3 Interpretative model of the δ18Op of crocodilian enamel
The interpretative model proposed in this paper is adapted to the context of the Shungura Fm (Fig. 7). The first part of the model is based on the idea that the teeth discovered in the Shungura Formation may have originated from two scenarios: (1) some teeth belonged to individuals that developed their teeth within the local waterbody, reflecting the depositional environment of the Shungura Formation or (2) other teeth might have come from individuals growing their teeth in another nearby waterbody, and these teeth were later shed when the crocodilian came to the waterbody recorded in the Shungura Fm.
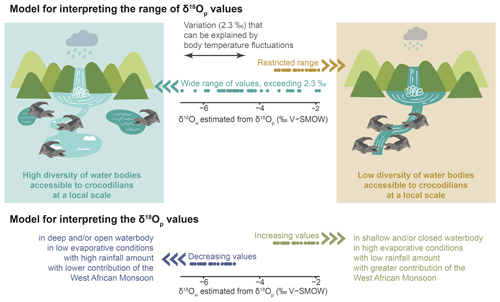
Figure 7Interpretative model of the δ18Ow estimated from δ18Op of crocodilian teeth in the context of the Shungura Fm.
Considering a ∼ 2.3 ‰ range in δ18Op will allow us to take precaution for possible body temperature differences between individuals (behaviour, size, age), populations, and species. Once this variation in δ18Op due to the fluctuating body temperature of crocodilians is excluded, the remaining range of δ18Op values within the same unit should reflect the local variety of waterbody types (with different δ18Ow) occupied by these animals. In other words, only one type of aquatic environment was available for crocodilians in a unit where the data distribution does not exceed ∼ 2.3 ‰. Conversely, if the distribution exceeds ∼ 2.3 ‰, then different aquatic environments nearby were occupied by these animals, characterised by different evaporation conditions and/or different water sources. This part of the model opens a window into the hydrological dynamics of the basin. It is noteworthy that this variability in the palaeohydrology, revealed by a single stratigraphic unit-based study, corresponding to or within a given locality, could not have been highlighted by a study based on mean values performed at the stratigraphic member-based scale of the Shungura Formation. As mentioned in Sect. 4.2.1, the season and duration of tooth mineralisation cannot be definitively determined but might affect the interpretation of the resulting isotopic signal. Hence, the diversity of freshwater environment observed with the range of δ18Ow could be on an annual or seasonal scale within each unit. Moreover, the interannual variability in the isotopic signal in the Turkana Depression can be influenced by different types of aquatic environments and water mixing, as evidenced by mollusc shells in the Omo Delta (range of 6 ‰), Omo River (range of 2.5 ‰), and Turkana Lake (negligible fluctuations) (Vonhof et al., 2013).
Concerning the second part of the model, interpreting past δ18Ow in the Omo Valley context should mainly consider the variability in the evaporative state of water (aridity, drainage, and depth of waterbodies) in rainfall (especially the water supply on the Ethiopian Dome), and in low-level air streams, as well as the migration of the convergence zone. In other words, decreasing δ18Ow values might be mainly linked to an increased rainfall supply on the Ethiopian Dome, a northward penetration of the Indian Summer Monsoon (consistent with low relief and a decrease in its intensity), and/or a more open/deep waterbody (Otero et al., 2011; Kebede and Travi, 2012). Conversely, increasing δ18Ow values are expected to result from drier regional conditions, an increase in the intensity of the Indian Summer Monsoon, and a relatively greater contribution of the recycled moisture from the Congo Basin to the water supply and/or to a close and/or shallow water system (Otero et al., 2011; Kebbede and Travi, 2012).
4.3 Interpretation of the δ18Op of fossil crocodilian enamel
4.3.1 Restriction of local diversity of aquatic environments
The large range of δ18Op values in units B-10 to C-8 indicates that crocodilians occupied different waterbody types. Low δ18Op values suggest waterbodies with low evaporation state (probably linked to short water residence time, through flowing, exorheic lake basin, river setting) or an 18O-depleted water source (e.g. unevaporated meteoric water from rivers or underground water), while the high values reflect shallow and/or isolated waterbodies subject to strong evaporation (e.g. enclosed endorheic lake basin, pond, shallow small lake, swamp) or an 18O-enriched water source. If the Shungura Fm was under lacustrine influence in unit B-10, it could explain the great diversity of waterbodies present in the area (Fig. 8). In Member C, the environment is predominantly fluvial, but the present isotopic data suggest that it was probably connected to other nearby waterbodies, making them accessible to local crocodilians. These δ18Op values do not indicate aridification affecting freshwater environments between 2.8 and 2.5 Ma (Members B and C). This contrasts with changes observed in the aquatic and terrestrial ecosystems of the eastern African Rift such as lake level drop, landscape opening, and aridification (e.g. Maslin and Trauth, 2009; Trauth et al., 2009, 2021; Levin et al., 2011; Negash et al., 2015, 2020; Blondel et al., 2018, 2022; Nutz et al., 2020) (Fig. 8). Interestingly, the δ13C of soil carbonates increases, thus indicating aridification in the Shungura Fm but not in the Nachukui Fm in the West Turkana area (Levin et al., 2011; Levin, 2013; Nutz et al., 2020) (Fig. 8). Nutz et al. (2020) explain that the δ13C record in the Shungura Fm reflects climatic conditions upstream on the Ethiopian Dome and that of Nachukui Fm reflects more local and regional climatic conditions. The δ13C of the palaeosols would therefore indicate a less significant supply of water from the Ethiopian Dome towards the Lower Omo Valley. On the other hand, the hydrological conditions remain stable in the western part of the Turkana Depression between 2.8 and 2.5 Ma, thus possibly preserving a certain diversity of aquatic environments in the surroundings. Instead of increasing and showing a restricted range of δ18Op values, as expected for an aridification context, the distribution of δ18Op values in Members B to C remains relatively stable, suggesting that crocodilians had access to the same range of waterbodies, which remained nearby or interconnected. This implies that the δ18O of the water sources remained relatively the same; or less likely, if evaporation increased, the δ18O of the source decreased in balance (resulting then from a greater contribution of the Indian Summer Monsoon) (Kebede and Travi, 2012) (Fig. 8).
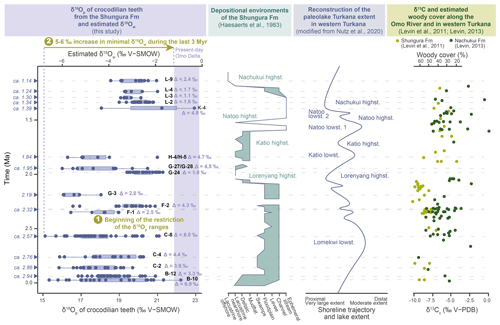
Figure 8Comparison of oxygen isotopic composition of crocodilian teeth (δ18Op) and estimated for surface water (δ18Ow) fluctuations with data on the evolution of sedimentary systems of the Shungura Formation, Lake Turkana level, and woody cover in the Shungura Fm.
The δ18O values are more homogeneous in unit F-1, indicating that crocodilians probably had access to only one type of waterbody at the local scale (Fig. 8). The minimal values are higher than in the older units, while the maximal values are lower, indicating that if the most evaporated waterbodies present in Members B and C still existed, they were no longer accessible to crocodilians. In unit F-2, the values are mostly higher than those of F-1 except for two teeth. This range would reflect at least two distinct waterbody types (Fig. 8). This is coherent with the formation of this unit during a period of lake transgression and increased water supply over the Ethiopian Dome (Levin et al., 2011; Nutz et al., 2020) (Fig. 8).
By contrast, in unit G-3 (with few teeth analysed), crocodilian teeth yielded a restricted range of low δ18Op values, reflecting a single accessible environment, probably the river according to sedimentological studies and to the increasingly humid conditions (Haesaerts et al., 1983; Levin et al., 2011; Nutz et al., 2020) (Fig. 8).
During the Lorenyang lake's highstand, the lake reached its maximum extent between approximately 2.10 and 1.90 Ma, coinciding with the presence of lacustrine facies in the Shungura Formation. According to Nutz et al. (2020), the regression phase commenced around 2 Ma (with G-24 dated at approximately 1.98 Ma), marked by a shoreline migration towards the basin and the gradual reappearance of deltaic facies within the G-sup Member. This suggests a transition towards more segmented aquatic environments, including distributary channels, abandoned channels, shallow satellite lakes, and ponds, rather than a uniform open lake. Given that the Shungura Formation is located upstream of the system (on the northern lake coast, near the primary tributary), there may have been an early emergence in this region. In contrast, the basin area likely retained a relatively clear lacustrine environment, as indicated on the map in Fig. 2. The G-sup Member records the initial declines in lake level and the subsequent development of a delta, particularly from G-27 (Haesaerts et al., 1983). In Fig. 8, lacustrine facies are briefly documented in the Shungura Formation, primarily marking the end of the lake's transgression and the beginning of its regression during the Lorenyang highstand. Specifically, G-24 appears as an exceptional event within the sequence: sedimentological and palaeontological data from this level exhibit distinct characteristics, potentially indicating secondary evaporitic facies and mass fish mortality events (Van Damme and Gautier, 1972; Haesaerts et al., 1983). The inclusion of G-24 within lacustrine layers supports that it may carry climatic significance, possibly signifying an abrupt arid event. Thus, this could correspond to a particular short event where the water supply is particularly low within a trend of aridification of the Ethiopian Dome. The isotopic data of crocodilians align with this interpretation, as reflected in the strongly positive d δ18Ow, signifying a highly evaporated waterbody.
The G-27/G-28 and H-4/H-5 units exhibit similar isotopic composition pattern, featuring a wide range of δ18O values, including both more negative and even some positive values akin to those observed in G-24 (Fig. 8). This suggests that crocodilians had access to at least two distinct types of waterbodies during this period: an evaporated lake and its tributary source.
The observed 4 ‰ difference between the isotopic compositions aligns with expectations for a contemporary Ethiopian exorheic lake and its tributary, as documented by Hailemichael et al. (2002, Table 2). This shift in isotopic patterns may illustrate the ongoing regression of the lake during the Katio lowstand, marking the establishment of a deltaic environment. This transition is consistent with previous sedimentological studies (Nutz et al., 2020) (Fig. 8). Notably, the present-day extensive Omo Delta exhibits a diverse range of sub-environments, including active or abandoned distributary channels and interior lakes that are not directly connected to the open lake. This modern landscape provides valuable insights into the Pleistocene environment (Schuster et al., 2022). With the Shungura Formation situated at the location of the primary tributary to Lake Turkana, specifically the Omo and its delta, the shift from shallow, evaporated lake facies in G-24 towards a deltaic environment was gradual. The crocodilians in the G-27/G-28 and H-4/H-5 units inhabited the interface between the tributary (less evaporated) and the lake (highly evaporated, resembling the conditions in G-24). This regression appears to be linked to climatic factors, potentially induced by a decrease in precipitation over the Ethiopian Dome, as suggested by Nutz et al. (2020).
The three teeth analysed in unit K-4 show divergent values, reflecting the presence of at least two different waterbodies accessible to crocodilians, but this interpretation must be viewed with caution because the number of teeth available for this unit was low.
Units L-2 to L-4 show particularly high minimal δ18Op values, indicating that the crocodilians only had access to one type of waterbody, evaporated and/or that the δ18O of precipitation increased. The depositional environments in these units are described as ephemeral streams by Haesaerts et al. (1983); therefore at least seasonally evaporating waterbodies could have existed (Fig. 8). The L-9 unit does not appear to be different from the other units analysed in Member L in terms of the oxygen isotopic composition of crocodilian enamel. Nevertheless, sedimentary studies demonstrate that it was deposited in a lacustrine context (Haesaerts et al., 1983). The distinction in δ18Ow between ephemeral streams and the lake may not be pronounced when the evaporation conditions of the waterbodies, including the ephemeral stream and offshore lake waters, are similar. Alternatively, this similarity might be balanced by a shift in the δ18O of precipitation, although this is less likely. It is important to note that the geochemical approach used in this study cannot provide a definitive answer to this question.
4.3.2 Long palaeoclimatic trend, the increase in δ18Ow during the last 3 Myr
The minimum estimated values of δ18Ow display evolution through time, increasing from −6.5 ± 2 ‰ at 2.97 Ma to −3.9 ± 2 ‰ at ca. 1.14 Ma. In general, estimated δ18Ow values do not, or barely, reach (considering the 2 ‰ error in the estimate) the modern δ18Ow values of Omo River waters at the delta (between −1.2 ‰ and −0.1 ‰) and of the Ethiopian lakes (between 1.5 ‰ and 7.2 ‰) (Table 2). Considering minimum δ18Ow value at 2.97 Ma and comparing it to present-day values, our reconstructed δ18Ow data show an increase between 5.3 ± 2 ‰ and 6.4 ± 2 ‰ (compared to the Omo River) (Table 2). Again, it should be kept in mind that these values represent a maximum estimate of the ancient δ18Ow values. Therefore, the difference between the ancient and the present values may be greater because the rainwater probably suffered from evaporation before its uptake crocodilian body water. This increase in δ18Ow is similar to that evidenced by the oxygen isotopic composition of palaeosols and fossil molluscs in other Plio-Pleistocene eastern African deposits (Hailemichael et al., 2002; Levin et al., 2004) and thus requires a regional explanation.
The increase in δ18Ow cannot be explained by major changes in the topography in the Ethiopian and Kenyan domes and the eastern African Rift (Abbate et al., 2005; Nutz et al., 2017; Ragon et al., 2019) but could be due to a rarefaction of rainfall. The extent of this remains difficult to estimate, as the oxygen isotopic composition of meteoric water (δ18Omw) in eastern Africa is currently not highly correlated with rainfall intensity. Levin et al. (2004) suggest that a 1 ‰ increase in δ18Omw would reflect a 32 mm decrease in precipitation, based on GNIP stations in east central Africa. The data from Table 2 show a relatively weak correlation between δ18Omw and the quantity of precipitation (R2 = 0.21), suggesting that a 1 ‰ increase in δ18Omw could be associated with a 160 mm decrease in precipitation. In the case of the Ethiopian Dome, with δ18Omw values at least 5.3 ‰ lower than today's, Addis Ababa would then have received 870 mm more precipitation in the late Pliocene, thus reaching about 2050 mm yr−1. This number is close to that recorded now in the equatorial forest of Congo (e.g. Goma GNIP station, IAEA, 2023, Table 2). If the Pliocene climatic regime were similar to the present day's, then higher sea surface temperatures in the Indian Ocean could have led to increased precipitation in eastern Africa at that time. This hypothesis is consistent with the progressive global cooling observed in the last million years (Zachos et al., 2001). Although there is no direct evidence for the existence of a rainforest on the Ethiopian Dome during the Pliocene, palaeobotanical studies reveal a period of drastic rainforest retreat between 3.5 and 2.5 Ma, probably before the first hominins in the region (Bonnefille, 2010), and the installation of an assemblage of mountain forest plants on the Ethiopian Dome, during global cooling (Bonnefille, 1983).
On the other hand, the sources and trajectories of precipitation play a major role in the variation in δ18Omw in eastern Africa. Changes in the location of the Intertropical Convergence Zone (ITCZ) or Congo Air Boundary (CAB) are more likely to strongly impact the variation in the isotopic composition of the eastern African meteoric water, potentially exceeding 3 ‰ (Kebede and Travi, 2012). With a warmer Indian Ocean, the ITCZ would have been expected to move northward, and the Ethiopian Dome would have received a larger proportion of Indian Summer Monsoon rains, which have lower δ18Omw values than the West African Monsoon. Alternatively, a more westerly location of the Congo Air Boundary in Ethiopia during the Plio-Pleistocene could have lowered the δ18Omw values up to the extent recorded 2.97 Ma in the Lower Omo Valley (Kebede and Travi, 2012; Levin et al., 2009).
Given their low metabolism and aquatic ecology, crocodilians stand out as excellent recorders of environmental conditions, with their δ18Op values closely linked to that of the water they live in during teeth formation. Combining knowledge of the physiology, ecology and development of crocodilians is of crucial importance to further interpret their oxygen isotopic signal: it allows indirectly gaining insights into the local diversity of aquatic environments, local evaporation, and δ18O values of meteoric waters. This approach opens a new understanding of past freshwater environments and is complementary to sedimentological studies and terrestrial proxies. Given the uncertainty surrounding the season and duration of tooth mineralisation and their potential impact on isotopic interpretations, further investigation on this issue could lead to a refined model. It could elucidate whether the observed diversity of freshwater environments within a stratigraphic unit represents year-round availability, season-specific occurrences, or variations across different seasons. The study of palaeohydrological variability should be conducted at the stratigraphic unit scale to fully capture its potential, and potentially within units based on lateral facies variations. A broader-scale study, such as that of the Shungura Formation members, would not provide such detailed insights.
The new dataset suggests that between 2.97 and ca. 2.57 Ma, the Lower Omo Valley hosted a great diversity of waterbody types, with different water sources or evaporative states. From ca. 2.32 Ma, the diversity of freshwater environments decreased drastically to only one or two types of waterbodies at each stratigraphic unit, probably due to aridification that caused some waterbodies to either disappear or to become further separated and isolated, making them inaccessible to local crocodilians. This freshwater environment restriction prevailed in the rest of the Shungura Fm except at ca. 1.95 and 1.84 Ma: the lake level decreased giving way to a deltaic environment in the Shungura Fm with a high diversity of waterbodies.
The δ18Op of crocodilian teeth also carries valuable information about regional palaeoenvironments but cannot help in identifying the exact cause between different potential factors (origin of water, rainfall amount, topography, temperature, etc.). This new dataset highlights a large increase in δ18Ow values, between 5.3 ‰ and 6.4 ‰, from the Pliocene to the present, consistent with other studies in eastern Africa (Hailemichael et al., 2002; Levin et al., 2004). This increase is likely to have resulted from the migration of convergence zones of low air streams affecting the distribution of the monsoons affecting the δ18Ow in addition to the resulting scarcity of rainfall over the Ethiopian Dome. The data point to a main shift in δ18Ow between 1.84 and ca. 1.34 Ma, slightly later than suggested by studies on palaeosols (Levin et al., 2011). While several terrestrial and aquatic proxies indicate aridification in the Turkana Depression between 2.8 and 2.5 Ma, the δ18Op of crocodilian teeth points to the stability of the local diversity of aquatic environments during this period. Crocodilian teeth are also abundant in continental series and widely distributed across the globe, notably in the Neogene strata of Africa. Although adapted in the context of the Shungura Fm, the proposed interpretation model can be generalised to comparable palaeontological and archaeological sites, especially in other sites in the Turkana Depression but will require sampling as detailed as that done by OGRE in the Shungura Fm. For optimal use of this approach, particularly along another stratigraphic sequence in the intertropical zone, it is necessary to ensure that temperature changes are limited and do not significantly affect the body temperature of crocodilians. For the same purpose, it is necessary to compare the same taxa throughout the sequence to avoid interspecific variation. A study on the systematics and temporal distribution of the different crocodilian taxa in the Shungura Fm would lead to better control of the potential phylogeny-dependent variation in δ18Op in the present dataset. Once the preservation of the original isotopic signal of the crocodilian fossil tooth enamel is ensured and the physiological, ecological, and environmental parts of the signal identified, then the δ18Op could be used to characterise the aquatic environments and their diversity over time in many other continental intertropical contexts.
The data related to this article is available online at https://doi.org/10.5281/zenodo.10084380 (Gardin et al., 2023).
AG, EP, GG, JRB, and OO designed the study. EP, GG, and OO provided supervision. JRB supervised fieldwork and specimen sampling, involving AN, MS, and OO. AG and EP performed enamel sampling and preparation in the lab. EP and MMJ performed the stable isotope analysis and data treatment. AE, AG, and JRB built the age model for the Shungura Fm. AG was responsible for statistical analysis, data curation, and visualisation, and wrote the paper with contributions from all the co-authors.
The contact author has declared that none of the authors has any competing interests.
Publisher's note: Copernicus Publications remains neutral with regard to jurisdictional claims made in the text, published maps, institutional affiliations, or any other geographical representation in this paper. While Copernicus Publications makes every effort to include appropriate place names, the final responsibility lies with the authors.
The authors are indebted to all the people who contributed to the fieldwork in the Shungura Formation as part of the Omo Group Research Expedition (OGRE, PI: Jean-Renaud Boisserie). Corresponding OGRE fieldwork was logistically supported by the UAR 3137 CFEE (Centre National de la Recherche Scientifique and Ministry of Europe and Foreign Affairs). Fieldwork, sampling material exportation, and destructive analyses of fossil remains were authorised by the Ethiopian Heritage Authority (previously ARCCH) within the framework of the OGRE research permit and greatly facilitated by its staff members. The authors also thank Sophie Hemry and Guillaume Di Bez, who prepared 80 % of the samples for phosphates for their master's theses. The authors are grateful to Ivan Jovovic (UMR 5561 Biogéosciences, Centre National de la Recherche Scientifique and University of Burgundy), who analysed part of the enamel carbonates sample. The authors also thank the reviewers for their insightful comments which allowed the article to be improved.
Corresponding OGRE fieldwork seasons were funded notably by the UMR 7262 PALEVOPRIM (Centre National de la Recherche Scientifique and University of Poitiers), the French Ministry of Europe and Foreign Affairs, the CNRS (grant SEEG Shungura), the French National Agency for Research, and the Fyssen Foundation. This work forms part of the PhD thesis of Axelle Gardin, who received financial assistance from the UMR 7262 (PALEVOPRIM, Centre National de la Recherche Scientifique and University of Poitiers), the ASAP project (grant no. 210724, Nouvelle-Aquitaine region, France, PI: Olga Otero), and the French Ministry of Higher Education, Research and Innovation.
This paper was edited by Steven Bouillon and reviewed by two anonymous referees.
Abbate, E., Bruni, P., and Sagri, M.: Geology of Ethiopia: A Review and Geomorphological Perspectives, in: Landscapes and Landforms of Ethiopia, edited by: Billi, P., Springer Netherlands, Dordrecht, 33–64, https://doi.org/10.1007/978-94-017-8026-1_2, 2015.
Altman, P. L. and Dittmer, D. S.: Biology Data Book, Federation of American Societies for Experimental Biology, Bethesda, MD, 2123 pp., ISBN 0-913822-08-6, 1972.
Amiot, R., Lécuyer, C., Escarguel, G., Billon-Bruyat, J.-P., Buffetaut, E., Langlois, C., Martin, S., Martineau, F., and Mazin, J.-M.: Oxygen isotope fractionation between crocodilian phosphate and water, Palaeogeogr. Palaeocl., 243, 412–420, https://doi.org/10.1016/j.palaeo.2006.08.013, 2007.
Amiot, R., Göhlich, U. B., Lécuyer, C., de Muizon, C., Cappetta, H., Fourel, F., Héran, M.-A., and Martineau, F.: Oxygen isotope compositions of phosphate from Middle Miocene–Early Pliocene marine vertebrates of Peru, Palaeogeogr. Palaeocl., 264, 85–92, https://doi.org/10.1016/j.palaeo.2008.04.001, 2008.
Amiot, R., Buffetaut, E., Lécuyer, C., Wang, X., Boudad, L., Ding, Z., Fourel, F., Hutt, S., Martineau, F., Medeiros, M. A., Mo, J., Simon, L., Suteethorn, V., Sweetman, S., Tong, H., Zhang, F., and Zhou, Z.: Oxygen isotope evidence for semi-aquatic habits among spinosaurid theropods, Geology, 38, 139–142, https://doi.org/10.1130/G30402.1, 2010a.
Amiot, R., Wang, X., Lécuyer, C., Buffetaut, E., Boudad, L., Cavin, L., Ding, Z., Fluteau, F., Kellner, A. W. A., Tong, H., and Zhang, F.: Oxygen and carbon isotope compositions of middle Cretaceous vertebrates from North Africa and Brazil: Ecological and environmental significance, Palaeogeogr. Palaeocl., 297, 439–451, https://doi.org/10.1016/j.palaeo.2010.08.027, 2010b.
Arambourg, C., Chavaillon, J., and Coppens, Y.: Premiers résultats de la nouvelle mission de l’Omo, C. R. Acad. Sci., 265, 1891–1896, 1967.
Bauder, E. T.: The effects of an unpredictable precipitation regime on vernal pool hydrology, Freshwater Biol., 50, 2129–2135, https://doi.org/10.1111/j.1365-2427.2005.01471.x, 2005.
Beauchamp, J. S., Hart, K. M., Cherkiss, M. S., and Mazzotti, F. J.: Variation in home range size and patterns in adult female American crocodiles Crocodylus acutus, Endanger. Species Res., 36, 161–171, https://doi.org/10.3354/esr00900, 2018.
Blake, R. E., O'Neil, J. R., and Garcia, G. A.: Oxygen isotope systematics of biologically mediated reactions of phosphate: I. Microbial degradation of organophosphorus compounds, Geochim. Cosmochim. Ac., 61, 4411–4422, https://doi.org/10.1016/S0016-7037(97)00272-X, 1997.
Blondel, C., Rowan, J., Merceron, G., Bibi, F., Negash, E., Barr, W. A., and Boisserie, J.-R.: Feeding ecology of Tragelaphini (Bovidae) from the Shungura Formation, Omo Valley, Ethiopia: Contribution of dental wear analyses, Palaeogeogr. Palaeocl., 496, 103–120, https://doi.org/10.1016/j.palaeo.2018.01.027, 2018.
Blondel, C., Merceron, G., Rowan, J., Surault, J., and Boisserie, J.-R.: Dietary ecology of Reduncini (Bovidae) from the Shungura Formation, Lower Omo Valley, Ethiopia, Palaeogeogr. Palaeocl., 587, 110789, https://doi.org/10.1016/j.palaeo.2021.110789, 2022.
Bobe, R.: The evolution of arid ecosystems in eastern Africa, J. Arid Environ., 66, 564–584, https://doi.org/10.1016/j.jaridenv.2006.01.010, 2006.
Boisserie, J.-R., Guy, F., Delagnes, A., Hlukso, L. J., Bibi, F., Beyene, Y., and Guillemot, C.: New palaeoanthropological research in the Plio-Pleistocene Omo Group, Lower Omo Valley, SNNPR (Southern Nations, Nationalities and People Regions), Ethiopia, C. R. Palevol, 7, 429–439, https://doi.org/10.1016/j.crpv.2008.07.010, 2008.
Bonnefille, R.: Evidence for a cooler and drier climate in the Ethiopian uplands towards 2.5 Myr ago, Nature, 303, 487–491, https://doi.org/10.1038/303487a0, 1983.
Bonnefille, R.: Cenozoic vegetation, climate changes and hominid evolution in tropical Africa, Global Planet. Change, 72, 390–411, https://doi.org/10.1016/j.gloplacha.2010.01.015, 2010.
Brochu, C. A.: Pliocene crocodiles from Kanapoi, Turkana Basin, Kenya, J. Hum. Evol., 140, 102410, https://doi.org/10.1016/j.jhevol.2017.10.003, 2020.
Brochu, C. A. and Storrs, G. W.: A giant crocodile from the Plio-Pleistocene of Kenya, the phylogenetic relationships of Neogene African crocodylines, and the antiquity of Crocodylus in Africa, J. Vertebr. Paleontol., 32, 587–602, https://doi.org/10.1080/02724634.2012.652324, 2012.
Brochu, C. A., Njau, J., Blumenschine, R. J., and Densmore, L. D.: A New Horned Crocodile from the Plio-Pleistocene Hominid Sites at Olduvai Gorge, Tanzania, PLoS One, 5, e9333, https://doi.org/10.1371/journal.pone.0009333, 2010.
Brochu, C. A., de Celis, A., Adams, A. J., Drumheller, S. K., Nestler, J. H., Benefit, B. R., Grossman, A., Kirera, F., Lehmann, T., and Liutkus-Pierce, C.: Giant dwarf crocodiles from the Miocene of Kenya and crocodylid faunal dynamics in the late Cenozoic of East Africa, Anat. Rec., 305, 2729–2765, https://doi.org/10.1002/ar.25005, 2022.
Bryant, D. J. and Froelich, P. N.: A model of oxygen isotope fractionation in body water of large mammals, Geochim. Cosmochim. Ac., 59, 4523–4537, https://doi.org/10.1016/0016-7037(95)00250-4, 1995.
Camberlin, P.: Rainfall anomalies in the source region of the Nile and their connection with the Indian summer monsoon, J. Climate, 10, 1380–1392, 1997.
Campbell, H. A., Watts, M. E., Sullivan, S., Read, M. A., Choukroun, S., Irwin, S. R., and Franklin, C. E.: Estuarine crocodiles ride surface currents to facilitate long-distance travel, J. Anim. Ecol., 79, 955–964, https://doi.org/10.1111/j.1365-2656.2010.01709.x, 2010.
Chabreck, R. H. and Joanen, T.: Growth rates of American alligators in Louisiana, Herpetologica, 14, 51–57, 1979.
Christian, K., Green, B., and Kennett, R.: Some Physiological Consequences of Estivation by Freshwater Crocodiles, Crocodylus johnstoni, J. Herpetol., 30, 1–9, https://doi.org/10.2307/1564699, 1996.
Cloudsley-Thompson, J. L.: Water Relations of Crocodiles, Nature, 220, 708–708, https://doi.org/10.1038/220708a0, 1968.
Coppens, Y. and Howell, F. C.: Mammalian faunas of the Omo Group: distributional and biostratigraphic aspects, in: Earliest Man and Environments in the Lake Rudolf Basin, edited by: Coppens, Y., Howell, F. C., Isaac, G. L., and Leakey, R. E. F., Chicago, 177–192, ISBN 0-226-11577-1, 1976.
Cott, H. B.: Scientific results of an inquiry into the ecology and economic status of the Nile Crocodile (Crocodilus niloticus) in Uganda and Northern Rhodesia, J. Zool., 29, 211–356, 1960.
Dansgaard, W.: Stable isotopes in precipitation, Tellus, 16, 436–468, https://doi.org/10.1111/j.2153-3490.1964.tb00181.x, 1964.
Davis, J. E., Spotila, J. R., and Schefler, W. C.: Evaporative water loss from the American alligator, Alligator mississippiensis: The relative importance of respiratory and cutaneous components and the regulatory role of the skin, Comp. Biochem. Phys. A, 67, 439–446, https://doi.org/10.1016/S0300-9629(80)80020-X, 1980.
de Heinzelin, J.: The Omo Group. Archives of the International Omo Research Expedition, Musée Royal de l'Afrique Centrale, Tervuren, 365 pp., 1983.
de Heinzelin, J. and Haesaerts, P.: The Shungura Formation, in: The Omo Group. Archives of the International Omo Research Expedition, edited by: de Heinzelin, J. , Tervuren, 25–127, 1983.
Edmund, A. G.: Sequence and rate of tooth replacement in the Crocodilia, R. Ont. Mus. Life Sci. Div. Cont., 56, 1–42, 1962.
Erickson, G. M.: Verification of von Ebner incremental lines in extant and fossil archosaur dentine and tooth replacement rate assessment using counts of von Ebner lines, PhD Thesis, Montana State University-Bozeman, College of Letters & Science, 1992.
Erickson, G. M.: Incremental lines of von Ebner in dinosaurs and the assessment of tooth replacement rates using growth line counts, P. Natl. Acad. Sci. USA, 93, 14623–14627, https://doi.org/10.1073/pnas.93.25.14623, 1996.
Gardin, A., Pucéat, E., Géraldine, G., Boisserie, J.-R., Adélaïde, E., Joachimski, M. M., Nutz, A., Schuster, M., and Otero, O.: Data from: Stable oxygen isotopes of crocodilian tooth enamel allow tracking Plio-Pleistocene evolution of freshwater environments and climate in the Shungura Formation (Turkana Depression, Ethiopia), Zenodo [data set], https://doi.org/10.5281/zenodo.10084380, 2023.
Glass, M. L. and Johansen, K.: Periodic breathing in the crocodile, Crocodylus niloticus: consequences for the gas exchange ratio and control of breathing, J. Exp. Zool., 208, 319–325, https://doi.org/10.1002/jez.1402080308, 1979.
Goedert, J., Amiot, R., Berthet, D., Fourel, F., Simon, L., and Lécuyer, C.: Combined oxygen and sulphur isotope analysis – a new tool to unravel vertebrate (paleo)-ecology, The Science of Nature, 107, 10, https://doi.org/10.1007/s00114-019-1664-3, 2020.
Grigg, G. C.: Metabolic Rate, Q 10 and Respiratory Quotient (RQ) in Crocodylus porosus, and Some Generalizations about Low RQ in Reptiles, Physiol. Zool., 51, 354–360, https://doi.org/10.1086/physzool.51.4.30160960, 1978.
Haesaerts, P., Stoops, G., and Van Vliet-Lanoe, B.: Data on sediments and fossil soils, in: The Omo Group. Archives of the International Omo Research Expedition, edited by: de Heinzelin, J., Tervuren, 149–185, 1983.
Hailemichael, M., Aronson, J. L., Savin, S., Tevesz, M. J. S., and Carter, J. G.: δ18O in mollusk shells from Pliocene Lake Hadar and modern Ethiopian lakes: implications for history of the Ethiopian monsoon, Palaeogeogr. Palaeocl., 186, 81–99, https://doi.org/10.1016/S0031-0182(02)00445-5, 2002.
Howell, C.: Omo research expedition, Nature, 219, 5154, 567–572, 1968.
Howell, F. C., Haesaerts, P., and de Heinzelin, J.: Depositional environments, archeological occurrences and hominids from Members E and F of the Shungura Formation (Omo basin, Ethiopia), J. Hum. Evol., 16, 665–700, https://doi.org/10.1016/0047-2484(87)90019-4, 1987.
Hutton, J. M.: Growth and Feeding Ecology of the Nile Crocodile Crocodylus niloticus at Ngezi, Zimbabwe, J. Anim. Ecol., 56, 25–38, https://doi.org/10.2307/4797, 1987.
Iacumin, P., Bocherens, H., Mariotti, A., and Longinelli, A.: Oxygen isotope analyses of co-existing carbonate and phosphate in biogenic apatite: a way to monitor diagenetic alteration of bone phosphate?, Earth Planet. Sc. Lett., 142, 1–6, https://doi.org/10.1016/0012-821X(96)00093-3, 1996.
IAEA: Global Network of Isotopes in Precipitation. The GNIP Database: https://www.iaea.org/services/networks/gnip, last access: 2 May 2023.
Isberg, S., Combrink, X., Lippai, C., and Balaguera-Reina, S. A.: Crocodylus niloticus, The IUCN Red List of Threatened Species, e-T45433088A3010181, https://doi.org/10.2305/IUCN.UK.2019-1.RLTS.T45433088A3010181.en, 2019.
Joachimski, M. M., Breisig, S., Buggisch, W., Talent, J. A., Mawson, R., Gereke, M., Morrow, J. R., Day, J., and Weddige K.: Devonian climate and reef evolution: Insights from oxygen isotopes in apatite, Earth Planet. Sc. Lett., 284, 3–4, https://doi.org/10.1016/j.epsl.2009.05.028, 2009.
Kebede, S. and Travi, Y.: Origin of the δ18O and δ2H composition of meteoric waters in Ethiopia, Quatern. Int., 257, 4–12, https://doi.org/10.1016/j.quaint.2011.09.032, 2012.
Kebede, S., Travi, Y., and Rozanski, K.: The δ18O and δ2H enrichment of Ethiopian lakes, J. Hydrol., 365, 173–182, https://doi.org/10.1016/j.jhydrol.2008.11.027, 2009.
Kohn, M. J.: Predicting animal δ18O: Accounting for diet and physiological adaptation, Geochim. Cosmochim. Ac., 60, 4811–4829, https://doi.org/10.1016/S0016-7037(96)00240-2, 1996.
Kolodny, Y., Luz, B., and Navon, O.: Oxygen isotope variations in phosphate of biogenic apatites, I. Fish bone apatite – rechecking the rules of the game, Earth Planet. Sc. Lett., 64, 398–404, https://doi.org/10.1016/0012-821X(83)90100-0, 1983.
Kolodny, Y., Luz, B., Sander, M., and Clemens, W. A.: Dinosaur bones: fossils or pseudomorphs? The pitfalls of physiology reconstruction from apatitic fossils, Palaeogeogr. Palaeocl., 126, 161–171, https://doi.org/10.1016/S0031-0182(96)00112-5, 1996.
Lang, J. W.: Crocodilian thermal selection, in: Widlife Management: Crocodiles and Alligators, edited by: Grahame, J. W., Webb, S., Manolis, C., and Whitehead, P. J., Surrey Beatty, Sydney, 273–294, ISBN 0-949324-09-4, 1987a.
Lang, J. W.: Crocodilian thermal selection, in: Widlife Management: Crocodiles and Alligators, edited by: Grahame, J. W., Webb, S., Manolis, C., and Whitehead, P. J., Surrey Beatty, Sydney, 301–317, ISBN 0-949324-09-4, 1987b.
Lecuyer, C., Grandjean, P., and Sheppard, S. M. F.: Oxygen isotope exchange between dissolved phosphate and water at temperatures ≤ 135 ∘C: inorganic versus biological fractionations, Geochim. Cosmochim. Ac., 63, 855–862, https://doi.org/10.1016/S0016-7037(99)00096-4, 1999.
Levin, N. E.: Compilation of East Africa Soil Carbonate Stable Isotope Data, Version 1.0, EarthChem [data set], https://doi.org/10.1594/IEDA/100231, last access: 10 May 2023.
Levin, N. E., Quade, J., Simpson, S. W., Semaw, S., and Rogers, M.: Isotopic evidence for Plio–Pleistocene environmental change at Gona, Ethiopia, Earth Planet. Sc. Lett., 219, 93–110, https://doi.org/10.1016/S0012-821X(03)00707-6, 2004.
Levin, N. E., Zipser, E. J., and Cerling, T. E.: Isotopic composition of waters from Ethiopia and Kenya: Insights into moisture sources for eastern Africa, J. Geophys. Res., 114, D23306, https://doi.org/10.1029/2009JD012166, 2009.
Levin, N. E., Brown, F. H., Behrensmeyer, A. K., Bobe, R., and Cerling, T. E.: Paleosol carbonates from the Omo Group: Isotopic records of local and regional environmental change in East Africa, Palaeogeogr. Palaeocl., 307, 75–89, https://doi.org/10.1016/j.palaeo.2011.04.026, 2011.
Longinelli, A. and Nuti, S.: Revised phosphate-water isotopic temperature scale, Earth Planet. Sc. Lett., 19, 373–376, https://doi.org/10.1016/0012-821X(73)90088-5, 1973.
Markwick, P. J.: Fossil crocodilians as indicators of Late Cretaceous and Cenozoic climates: implications for using palaeontological data in reconstructing palaeoclimate, Palaeogeogr. Palaeocl., 137, 205–271, https://doi.org/10.1016/S0031-0182(97)00108-9, 1998.
Maslin, M. A. and Trauth, M. H.: Plio-Pleistocene East African pulsed climate variability and its influence on early human evolution, in: The First Humans – Origin and Early Evolution of the Genus Homo, edited by: Grine, F. E., Fleagle, J. G., and Leakey, R. E., Springer, Dordrecht, 151–158, https://doi.org/10.1007/978-1-4020-9980-9, 2009.
Mazzotti, F. J. and Duson: Osmoregulation in Crocodilians, Am. Zool., 29, 903–920, 1989.
Merkel, A.: Climate-Data.org, http://fr.climate-data.org, last access: 2 May 2023.
Mushet, D. M., Alexander, L. C., Bennett, M., Schofield, K., Christensen, J. R., Ali, G., Pollard, A., Fritz, K., and Lang, M. W.: Differing modes of biotic connectivity within freshwater ecosystem mosaics, J. Am. Water Resour. As., 55, 307–317, 2019.
Negash, E. W., Alemseged, Z., Wynn, J. G., and Bedaso, Z. K.: Paleodietary reconstruction using stable isotopes and abundance analysis of bovids from the Shungura Formation of South Omo, Ethiopia, J. Hum. Evol., 88, 127–136, https://doi.org/10.1016/j.jhevol.2015.07.009, 2015.
Negash, E. W., Alemseged, Z., Bobe, R., Grine, F., Sponheimer, M., and Wynn, J. G.: Dietary trends in herbivores from the Shungura Formation, southwestern Ethiopia, P. Natl. Acad. Sci. USA, 117, 21921–21927, https://doi.org/10.1073/pnas.2006982117, 2020.
Nutz, A., Schuster, M., Boës, X., and Rubino, J.-L.: Orbitally-driven evolution of Lake Turkana (Turkana Depression, Kenya, EARS) between 1.95 and 1.72 Ma: A sequence stratigraphy perspective, J. Afr. Earth Sci., 125, 230–243, https://doi.org/10.1016/j.jafrearsci.2016.10.016, 2017.
Nutz, A., Schuster, M., Barboni, D., Gassier, G., Van Bocxlaer, B., Robin, C., Ragon, T., Ghienne, J.-F., and Rubino, J.-L.: Plio-Pleistocene sedimentation in West Turkana (Turkana Depression, Kenya, East African Rift System): Paleolake fluctuations, paleolandscapes and controlling factors, Earth Sci. Rev., 211, 103415, https://doi.org/10.1016/j.earscirev.2020.103415, 2020.
Nutz, A., Ragon, T., and Schuster, M.: Cenozoic tectono-sedimentary evolution of the northern Turkana Depression (East African Rift System) and its significance for continental rifts, Earth Planet. Sc. Lett., 578, 117285, https://doi.org/10.1016/j.epsl.2021.117285, 2022.
O'Neil, J. R., Roe, L. J., Reinhard, E., and Blake, R. E.: A rapid and precise method of oxygen isotope analysis of biogenic phosphate, Isr. J. Earth Sci., 43, 203–212, 1994.
Otero, O., Lécuyer, C., Fourel, F., Martineau, F., Mackaye, H. T., Vignaud, P., and Brunet, M.: Freshwater fish δ18O indicates a Messinian change of the precipitation regime in Central Africa, Geology, 39, 435–438, https://doi.org/10.1130/G31212.1, 2011.
Passey, B. H., Levin, N. E., Cerling, T. E., Brown, F. H., and Eiler, J. M.: High-temperature environments of human evolution in East Africa based on bond ordering in paleosol carbonates, P. Natl. Acad. Sci. USA, 107, 11245–11249, https://doi.org/10.1073/pnas.1001824107, 2010.
Peypouquet, J. P. and Carbonel, P.: Les ostracodes indicateurs des paléoenvironnements lacustres des rifts de l'Afrique roeintale au Plio-Pléistocène, CR Acad. Sci., 290, 563–566, 1980.
Peypouquet, J. P., Carbonel, P., and Heinzelin, J. de: Les ostracodes indicateurs de l'évolution des environnements lacustres de la fin du Cénozoïque dans la branche orientale du Rift africain, Bulletin Institute de Geologie du Basin d'Aquitarine, Bordeaux, 25, 201–219, 1979.
Pucéat, E., Reynard, B., and Lécuyer, C.: Can crystallinity be used to determine the degree of chemical alteration of biogenic apatites?, Chem. Geol., 205, 83–97, https://doi.org/10.1016/j.chemgeo.2003.12.014, 2004.
Pucéat, E., Joachimski, M. M., Bouilloux, A., Monna, F., Bonin, A., Motreuil, S., Morinière, P., Hénard, S., Mourin, J., Dera, G., and Quesne, D.: Revised phosphate–water fractionation equation reassessing paleotemperatures derived from biogenic apatite, Earth Planet. Sc. Lett., 298, 135–142, https://doi.org/10.1016/j.epsl.2010.07.034, 2010.
R Core Team: R: A language and environment for statistical computing, R Foundation for Statistical Computing, Vienna, Austria, 2023.
Ragon, T., Nutz, A., Schuster, M., Ghienne, J.-F., Ruffet, G., and Rubino, J.-L.: Evolution of the northern Turkana Depression (East African Rift System, Kenya) during the Cenozoic rifting: New insights from the Ekitale Basin (28–25.5 Ma), Geol. J., 54, 3468–3488, https://doi.org/10.1002/gj.3339, 2019.
Rozanski, K., Araguás-Araguás, L., and Gonfiantini, R.: Isotopic patterns in modern global precipitation, in: Climate Change in Continental Isotopic Records, Vol. 78, edited by: Swart, P. K., Lohmann, K. C., Mckenzie, J., and Savin, S., American Geophysical Union, Washington, D. C., 1–36, https://doi.org/10.1029/GM078p0001, 1993.
Schuster, M., Zainescu, F., Nutz, A., Storms, J. E. A., van der Vegt, H., Bozetti, G., May, J. H., May, S. M., Boisserie, J.-R., Ragon, T., Bouchette, F., and Ghienne, J.-F.: Le delta de l'Omo: Un delta à dominante rivière en contexte lacustre, Géochronique, 162, 35–39, 2022.
Seebacher, F., Grigg, G. C., and Beard, L. A.: Crocodiles as dinosaurs: behavioural thermoregulation in very large ectotherms leads to high and stable body temperatures, J. Exp. Biol., 202, 77–86, https://doi.org/10.1242/jeb.202.1.77, 1999.
Seymour, R., Bennett, A. F., and Bradford, D. F.: Blood gas tensions and acid-base regulation in the salt-water crocodile, Crocodylus porosus, at rest and after exhaustive exercise, J. Exp. Biol., 118, 143–159, https://doi.org/10.1242/jeb.118.1.143, 1985.
Seymour, R. S., Gienger, C. M., Brien, M. L., Tracy, C. R., Manolis, C. S., Webb, G. J. W., and Christian, K. A.: Scaling of standard metabolic rate in estuarine crocodiles Crocodylus porosus, J. Comp. Physiol. B, 183, 491–500, https://doi.org/10.1007/s00360-012-0732-1, 2013.
Shemesh, A., Kolodny, Y., and Luz, B.: Oxygen isotope variations in phosphate of biogenic apatites, II. Phosphorite rocks, Earth Planet. Sc. Lett., 64, 405–416, https://doi.org/10.1016/0012-821X(83)90101-2, 1983.
Smith, E. N.: Behavioral and Physiological Thermoregulation of Crocodilians, Am. Zool., 19, 239–247, https://doi.org/10.1093/icb/19.1.239, 1979.
Smith, T. and Boers, N.: Global vegetation resilience linked to water availability and variability, Nat. Commun., 14, 498, https://doi.org/10.1038/s41467-023-36207-7, 2023.
Souron, A., Balasse, M., and Boisserie, J.-R.: Intra-tooth isotopic profiles of canines from extant Hippopotamus amphibius and late Pliocene hippopotamids (Shungura Formation, Ethiopia): Insights into the seasonality of diet and climate, Palaeogeogr. Palaeocl., 342–343, 97–110, https://doi.org/10.1016/j.palaeo.2012.05.007, 2012.
Storrs, G. W.: Late Miocene–Early Pliocene Crocodilian Fauna of Lothagam, Southwest Turkana Basin, Kenya, in: Lothagam. The Dawn of Humanity in Eastern Africa, edited by: Leakey, M. and Harris, J., Columbia University Press, New York, 137–160, ISBN 0-231-11870-8, 2003.
Suarez, C. A., González, L. A., Ludvigson, G. A., Cifelli, R. L., and Tremain, E.: Water utilization of the Cretaceous Mussentuchit Member local vertebrate fauna, Cedar Mountain Formation, Utah, USA: Using oxygen isotopic composition of phosphate, Palaeogeogr. Palaeocl., 313–314, 78–92, https://doi.org/10.1016/j.palaeo.2011.10.011, 2012.
Swanepoel, D. G. J., Ferguson, N. S., and Perrin, M. R.: Nesting ecology of Nile crocodiles (Crocodylus niloticus) in the Olifants River, Kruger National Park, Koedoe, 43, 35–46, https://doi.org/10.4102/koedoe.v43i2.197, 2000.
Taplin, L. E.: Osmoregulation in the estuarine crocodile, Crocodilus porosus, PhD Thesis, University of Sydney, 1988.
Tchernov, E.: Crocodilians from the Late Cenozoic of the Rudolf Basin, in: Earliest Man and Environments in Lake Rudolf Basin. Stratigraphy, Paleoecology, and Evolution, edited by: Coppens, Y., Howell, F. C., Isaac, G. L., and Leakey, R. E., Chicago and London, 370–378, https://doi.org/10.1016/0033-5894(78)90078-9, 1976.
Tchernov, E.: Évolution des Crocodiles en Afrique du Nord et de l'Est, Cahiers de Paléontologie, Centre National de la Recherche Scientifique, Paris, ISBN 2-222-03822-7, 1986.
Trauth, M. H., Larrasoaña, J. C., and Mudelsee, M.: Trends, rhythms and events in Plio-Pleistocene African climate, Quaternary Sci. Rev., 28, 399–411, 2009.
Trauth, M. H., Asrat, A., Berner, N., Bibi, F., Foerster, V., Grove, M., Kaboth-Bahr, S., Maslin, M. A., Mudelsee, M., and Schäbitz, F.: Northern Hemisphere Glaciation, African climate and human evolution, Quaternary Sci. Rev., 268, 107095, https://doi.org/10.1016/j.quascirev.2021.107095, 2021.
Tucker, A. D., Limpus, C. J., McCallum, H. I., and McDonald, K. R.: Movements and Home Ranges of Crocodylus johnstoni in the Lynd River, Queensland, Wildl. Res., 24, 379–396, https://doi.org/10.1071/wr95073, 1997.
Van Bocxlaer, B. and Van Damme, D.: Palaeobiology and evolution of the late Cenozoic freshwater Molluscs of the Turkana basin: Iridinidae Swainson, 1840 and Etheriidae Deshayes, 1830 (Bivalvia: Etherioidea), J. Syst. Palaeontol., 7, 129–161, https://doi.org/10.1017/S1477201908002587, 2009.
Van Damme, D. and Gautier, A.: Molluscan assemblages from the late Cenozoic of the Lower Omo Basin, Ethiopia, Quatern. Res., 2, 25–37, https://doi.org/10.1016/0033-5894(72)90003-8, 1972.
Vonhof, H. B., Joordens, J. C. A., Noback, M. L., van der Lubbe, J. H. J. L., Feibel, C. S., and Kroon, D.: Environmental and climatic control on seasonal stable isotope variation of freshwater molluscan bivalves in the Turkana Basin (Kenya), Palaeogeogr. Palaeocl., 383–384, 16–26, https://doi.org/10.1016/j.palaeo.2013.04.022, 2013.
Wenzel, B., Lécuyer, C., and Joachimski, M. M.: Comparing oxygen isotope records of Silurian calcite and phosphate – δ18O compositions of brachiopods and conodonts, Geochim. Cosmochim. Ac., 64, 1859–1872, https://doi.org/10.1016/S0016-7037(00)00337-9, 2000.
Zachos, J., Pagani, M., Sloan, L., Thomas, E., and Billups, K.: Trends, rhythms, and aberrations in global climate 65 Ma to present, Science, 292, 686–693, 2001.
Zazzo, A., Lécuyer, C., and Mariotti, A.: Experimentally-controlled carbon and oxygen isotope exchange between bioapatites and water under inorganic and microbially-mediated conditions, Geochim. Cosmochim. Ac., 68, 1–12, https://doi.org/10.1016/S0016-7037(03)00278-3, 2004.