the Creative Commons Attribution 4.0 License.
the Creative Commons Attribution 4.0 License.
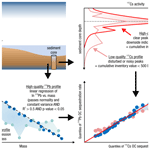
Technical note: Comparison of radiometric techniques for estimating recent organic carbon sequestration rates in inland wetland soils
Purbasha Mistry
Charles G. Trick
Eric Enanga
David A. Lobb
For wetlands to serve as natural climate solutions, accurate estimates of organic carbon (OC) sequestration rates in wetland sediments are needed. Dating using cesium-137 (137Cs) and lead-210 (210Pb) radioisotopes is commonly used for measuring OC sequestration rates in wetland sediments. 137Cs radioisotope dating is relatively simple, with calculations based on a single point representing the onset (1954) or peak (1963) of the 137Cs fallout. 210Pb radioisotope dating is more complex, as the calculations are based on multiple points. Here, we show that reliable dating of sediment cores collected from wetlands can be achieved using either 137Cs or 210Pb dating or their combination. However, 137Cs and 210Pb profiles along the depth of sediment cores need to be screened, analyzed, and interpreted carefully to estimate OC sequestration rates with high precision. To this end, we propose a decision framework for screening 137Cs and 210Pb profiles into high- and low-quality sediment profiles, and we compare dating using the 1954 and 1963 time markers, i.e., the rates of sedimentation and, consequently, OC sequestration over the past ∼ 60 years. Our findings suggest that 137Cs- and 210Pb-based OC sequestration rates are comparable, especially when using the 1963 (vs. 1954) time marker.
- Article
(3834 KB) - Full-text XML
-
Supplement
(1904 KB) - BibTeX
- EndNote
Wetlands in agricultural landscapes serve a crucial role in providing habitat for wildlife, regulating climate, improving water quality, and reducing floods. Moreover, these wetlands have the potential to sequester organic carbon (OC) (Bridgham et al., 2006; Nahlik and Fennessy, 2016; Bansal et al., 2023). Accounting for the balance between the sequestration and emission of carbon can help establish wetlands as essential candidates for natural climate solutions by offsetting carbon emissions (Hambäck et al., 2023). These wetlands embedded in agricultural landscapes are recognized as temperate inland wetland soils. The global carbon stock of temperate inland wetland soils is estimated to be 46 Pg C to 2 m depth, and Canada's temperate inland wetland soils are estimated to contain 4.6 Pg C (Bridgham et al., 2006). Compared to peatlands, the rapid rate of OC sequestration and the more considerable spatial extent of temperate inland wetland soils can help contribute significantly to regional or national carbon sequestration (Bridgham et al., 2006; Nahlik and Fennessy, 2016).
Table 1Characteristics of 137Cs and unsupported 210Pb (210Pbex) dating to estimate sedimentation rates in wetlands.
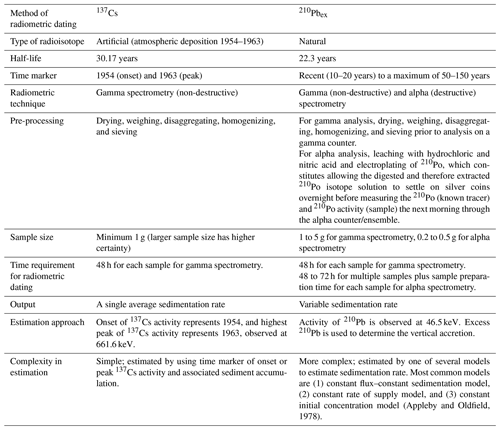
Canada encompasses around 25 % of the world's wetlands, with an area of approximately 1.29 million km2, which accounts for 13 % of the country's terrestrial area (Environment and Climate Change Canada, 2016), highlighting the global importance of these wetlands. Unfortunately, there are minimal data on the OC sequestration rates in these wetlands. To estimate the OC sequestration potential of these wetlands, it is essential to establish precise measurements to quantify wetland OC sequestration, develop strategies to promote conservation and restoration efforts, incorporate carbon credits in the carbon markets, and validate the wetland-based ecosystem services.
There are several ways to estimate the potential of wetlands to store OC (Bansal et al., 2023). One of these methods is radiometric dating, which can calculate the OC storage rates of wetlands over periods of 10 to ≥ 1000 years. Frequently used radioisotopes for radiometric dating are cesium-137 (137Cs) and lead-210 (210Pb), which can be used to estimate relatively recent (up to the last 100 years) OC sequestration rates (Villa and Bernal, 2018). Estimating OC sequestration rates involves building an age–depth profile or model of 137Cs and 210Pb from sediment cores that demonstrates the relationship between the depth of sediment layers and their corresponding age. Since the inorganic radioisotopes (137Cs and 210Pb) strongly bind with the soil particles once in contact, the radioisotopes can act as an efficient tracer for investigating OC sequestration rates (Ritchie and McHenry, 1990; Craft and Casey, 2000). These characteristics allow the accurate tracking of carbon movement within ecosystems, thereby enabling the extraction of detailed information about carbon sequestration dynamics in wetlands.
The characteristics of 137Cs and 210Pb to estimate wetland OC sequestration rates are presented in Table 1. 137Cs is an artificial radioisotope that was produced during thermonuclear bomb testing in the 1950s and 1960s, with the onset of atmospheric deposition in 1954 and a peak in 1963 (Ritchie and McHenry, 1990). The testing caused radioactive uranium to decay, and, as a result, the 137Cs isotope was released into the atmosphere and was then deposited around the globe. Although there may be challenges in applying our study to some parts of the world, the information is generally applicable and valuable for consideration in all regions. We encourage others to customize this approach further for use in other regions where 137Cs deposition histories vary.
137Cs has a half-life of 30.17 years, which can be used to estimate the last ∼ 50–70 years of OC sequestration rates in wetlands (e.g., Bernal and Mitsch, 2012). 137Cs dating assumes constant sedimentation rates measured since 1954 or 1963. In using the two time markers for 137Cs, we do not expect the sedimentation rates to be equal, but we do expect them to be similar. The onset and the peak of 137Cs activity at 661.6 keV can be used to mark 1954 and 1963, respectively. These time markers (1954 and 1963) can date sediment layers (Pennington et al., 1973; Ritchie and McHenry, 1990; DeLaune et al., 2003) and consequently the OC sequestration rates. 137Cs has an additional time marker for Europe in 1986 due to the Chernobyl nuclear accident and for Japan in 2011 due to the Fukushima Daiichi nuclear accident (Foucher et al., 2021), indicating that OC sequestration estimates can be derived for different timescales. In the Americas, we do not see evidence of the 1986 or 2011 137Cs peaks, which are observed in Europe and Japan, respectively, so we did not need to use other radioisotope techniques (e.g., 239+240Pu) to distinguish the 1986 or 2011 137Cs peak from the 1963 137Cs peak. 137Cs dating requires a gamma spectrometer to estimate OC sequestration rates. Sample preparation for gamma analysis involves drying, weighing, disaggregating, homogenizing, and sieving (Bansal et al., 2023). Samples vary from 1 to 1500 g, with smaller samples associated with higher uncertainties and therefore requiring longer times to analyze. Gamma analysis counting times range from 4 to 48 h for each sample (e.g., 4 to 12 h in Li et al., 2007; 12 to 24 h in Zarrinabadi et al., 2023; and 24 to 48 h in Kamula et al., 2017). 137Cs dating provides a simple result (an average sedimentation rate), while 210Pb dating provides a more complex result (using a supply rate model to reveal trends in sedimentation rates). Plutonium (Pu) may replace 137Cs in the future due to concerns of half-life and persistence as a dating tool. In essence, 239+240Pu has the same source and deposition mechanism as 137Cs. Its longer half-life will make its peak measurable when 137Cs is no longer measurable.
Unlike 137Cs, 210Pb is a naturally occurring radionuclide derived from uranium-238 (238U) and deposits atmospherically from the decay of radium-226 (226Ra) (Walling and He, 1999). 210Pb has a half-life of 22.3 years and is used to estimate the last 10–150 years of OC sequestration rates in wetlands (Craft and Richardson, 1998; Craft and Casey, 2000; Craft et al., 2018; Creed et al., 2022). 210Pb activity can be measured using gamma (observed at 46.5 keV) and alpha (destructive) spectrometry (Walling and He, 1999; Bellucci et al., 2007). Traditional alpha analysis requires 0.2–0.5 g of sample and additional sample preparation involving leaching with hydrochloric and nitric acid and electroplating (up to 24 h for sample preparation) (Bansal et al., 2023). Alpha analysis can be considered an indirect method for 210Pb dating where polonium-210 (210Po) activity is measured, assuming both 210Pb and 210Po are in a secular equilibrium. 210Pb activity is calculated by comparing 210Po activity against the known activity of 209Po (isotope tracer). In alpha analysis, the additional time required for sample preparation is compensated by running multiple samples simultaneously (Bansal et al., 2023). Gamma and alpha spectrometry of 210Pb provides the total 210Pb activity, which incorporates unsupported (or excess) 210Pb (210Pbex) and supported 210Pb. 210Pbex is used to determine the mass or sediment accumulation rate. Supported 210Pb is derived from the natural decay of radium-226 (226Ra) in the sediment, while unsupported 210Pb comes from the decay of atmospheric radon-222 (222Rn), which deposits 210Pb onto the sediment surface from the air. Unsupported 210Pb activity decreases over time due to radioactive decay, unlike supported 210Pb (Appleby and Oldfield, 1983). The choice of model used in 210Pb dating can reflect constant and variable sedimentation rates (Sanchez-Cabeza and Ruiz-Fernandez, 2012) and, consequently, OC sequestration rates in wetlands. Some models used for 210Pb dating are (1) the constant flux–constant sedimentation (CFCS) model, (2) the constant rate of supply (CRS) model, and (3) the constant initial concentration (CIC) model (Appleby and Oldfield, 1978). Both 137Cs and 210Pb provide suitable time markers and a longer time horizon compared to direct measurements using the time marker of horizons (2–10 years) to study sediment accretion and, subsequently, OC sequestration rates in wetlands (Bernal and Mitsch, 2013; Villa and Bernal, 2018). In this study, we compared the average OC sequestration rate derived from 137Cs time markers with the progressive OC sequestration rates derived using a constant rate of supply model applied to 210Pb.
The combined use of 137Cs and 210Pb may improve the accuracy of the dating estimation (Drexler et al., 2018; Creed et al., 2022). The more detailed assessment accrues a higher cost and time requirement, and the need for specialized equipment and technical expertise to conduct laboratory and data analyses may constrain the research efforts (Bansal et al., 2023). Furthermore, factors such as timescales, analytical complexity in interpreting radioisotope profiles (e.g., 137Cs peak clarity), variability in atmospheric deposition, and mobilization of radioisotopes can contribute to uncertainty (Drexler et al., 2018; Loder and Finkelstein, 2020; Zhang et al., 2021; Bansal et al., 2023) and limit the applicability of one radioisotope over the other. Therefore, it is essential to consider the advantages and potential challenges of using radioisotopes before designing research studies.
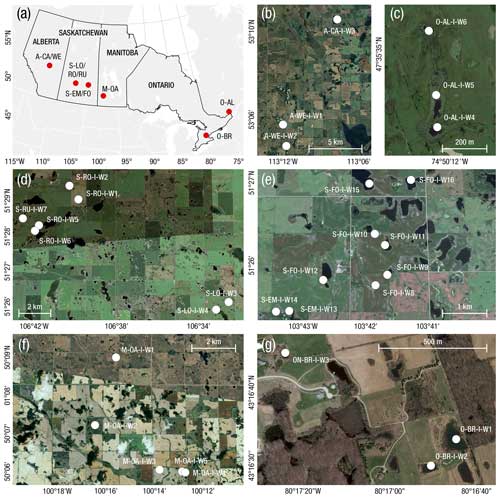
Figure 1(a) Study area situated in four provinces of Canada, (b) three wetland sites in Alberta (AB), (c) three wetland sites in Ontario (ON), (d) seven wetland sites in Saskatchewan (SK), (e) nine wetland sites in Saskatchewan (SK), (f) five wetland sites in Manitoba (MB), and (g) three wetland sites in Ontario (ON). Panels (b–g) are based on the sampling locations of wetlands used in this study reproduced using Google Earth Images: (b) and (c) ©2024 Airbus; (d), (e), and (g) ©2024 Maxar Technologies; (f) ©2024 Airbus and Maxar Technologies.
The main objective of this research paper is to explore the use of 137Cs and 210Pb to estimate recent OC sequestration rates in undisturbed (i.e., not directly impaired by human activities) temperate inland wetland soils located on agricultural landscapes. Here, we aim to (1) categorize 137Cs or 210Pb profiles into high- and low-quality via a decision framework, (2) apply the decision framework to estimate OC sequestration rates, (3) use 1963 and 1954 time markers to compare the 137Cs- and 210Pb-based OC sequestration rates to get a better understanding of the sediment history, and (4) select the best approach for 137Cs and 210Pb to estimate the OC sequestration rates with highest precision. This study helps reduce uncertainty in studies that rely on 137Cs or 210Pb radioisotope dating.
2.1 Sediment core collection
Triplicate sediment cores were collected from 30 undisturbed temperate inland wetland soils in agricultural landscapes across southern Canada (Fig. 1). A summary of the physical characteristics of these wetlands can be found in Table S1 in the Supplement. These wetlands were undisturbed, with no known history of cultivation. The sediment cores were extracted from the center of the wetland, constituting the open-water area. A Watermark Universal Core (inner diameter of 6.8 cm) or Vibecore Mini with Polycore tubes (inner diameter of 7.6 cm) was used to collect most of the sediment cores. A JMP Backsaver Soil Sampler (inner diameter of 3.2 cm) was used for compacted sediment cores. Shallow (16 to 90 cm) sediment cores were sectioned into 1 or 2 cm increments. Deeper (> 90 cm) sediment cores were sectioned into 5 cm increments. The sediment cores were stored at −5 °C for further processing at the laboratory.
2.2 Generation of 137Cs and 210Pb profiles
Sediment core increments were weighed (wet mass), dried, weighed again (dry mass), disaggregated, homogenized, and sieved. The increments were sieved to remove gravel (> 2 mm): radioisotopes do not bind on the gravel, and gravel does not contain OC; therefore, eliminating gravel improves the estimate of radioisotopes and OC. The increments were counted at 661.6 keV for 137Cs activity and 46.5 keV for 210Pb activity. 137Cs analysis was performed using a gamma spectrometer, and 210Pb analysis was performed using both gamma and alpha spectrometers to increase throughput rates. The gamma analysis was conducted using the high-purity germanium detectors; e.g., Broad Energy Germanium detectors (BE6530) and Small Anode Germanium well Detectors (GSW275L) (Mirion Technologies, Inc., Atlanta, GA, USA). The alpha analysis was conducted using an ORTEC® alpha spectrometer (AMETEK® Advanced Measurement Technology, TN, USA). Both radioisotope analyses were performed at the Landscape Dynamics Laboratory, University of Manitoba, Winnipeg, Canada. Although the underlying principles of gamma and alpha analysis differ, each analysis focuses on quantifying the decay of 210Pb, generating comparable results (Zaborska et al., 2007). Measurement accuracy of gamma detectors is ensured by assessing the counting errors with reference materials within the same geometry as the sample (e.g., Petri dish). The detection error was < 10 % with a counting time of up to 24 h. Furthermore, Landscape Dynamics Laboratory undergoes regular proficiency testing through the International Atomic Reference Material Agency (IARMA), and previously through the International Atomic Energy Agency (IAEA), to ensure acceptable accuracy and precision of analytical results using gamma spectroscopy.
2.3 Screening of 137Cs and 210Pb profiles
Sediment cores were screened to remove profiles with evidence of vertical mixing, then the remaining profiles were used to estimate OC sequestration rates using 137Cs or 210Pb radioisotope dating. The actual 137Cs peak can vary from the expected peak, increasing uncertainty in 137Cs dating (Drexler et al., 2018). 137Cs peaks can be “noisy” or “disturbed” (i.e., flattened, broadened, truncated, mixed, fluctuating; Drexler et al., 2018) or one-sided, where the 137Cs peaks appear at the surface of the sediment core (indicating no or little sedimentation since 1963). The magnitude and shape of the 137Cs peaks observed in the sediments can be affected by the atmospheric deposition rate of 137Cs, which is obviously affected by the number and magnitude of emission events and the weather conditions following these events (UNSCEAR, 2000). The magnitude and shape of these peaks are also impacted by the movement of water and sediment within each wetland's catchment during the peaks' development (Milan et al., 1995; Zarrinabadi et al., 2023). Here, changes in the shape of the peaks are caused by the upward and downward movement of the sediment within the sediment profile (the movement of 137Cs through diffusion (Klaminder et al., 2012) is presumed negligible). Bioturbation can cause an upward and downward mixing of the 137Cs in the profile, resulting in peak attenuation (Robbins et al., 1977). Even wave action during the period of atmospheric deposition will have a similar attenuation effect (Andersen et al., 2000; Zarrinabadi et al., 2023). Following peak atmospheric deposition, soil erosion and the accumulation of sediment will deliver sediments to the top of the profile, and those sediments may be higher or lower in concentration depending on the degree of preferential sediment transport and the associated enrichment or depletion of 137Cs in the added sediment (Zarrinabadi et al., 2023). Such noise in 137Cs peaks needs careful interpretation to avoid over- or underestimating the OC sequestration rates.
Selecting suitable cores: Of the 90 sediment cores (30 wetlands × 3 replicates = 90), 79 were suitable (complete and datable) for 137Cs dating and 47 were suitable for 210Pb dating. Only some replicates from the same wetland were ideal for interpretation or further screening. The suitability of 137Cs profiles for dating was assessed by zero activity before the onset and peak of 137Cs activity. The suitability of 210Pb profiles for dating was evaluated by determining the exponential decline in 210Pb activity with depth until background levels were reached.
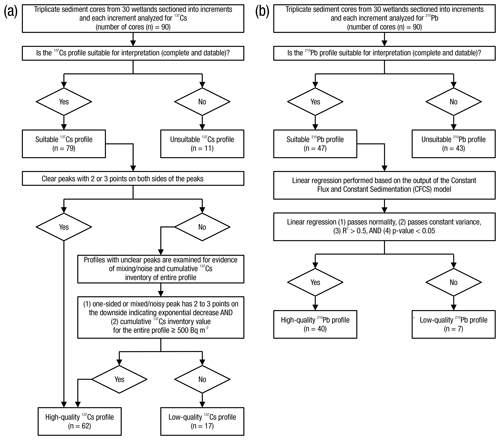
Figure 2Classification of high- and low-quality 137Cs and 210Pb profiles outlining the decision frameworks for screening (a) 137Cs and (b) 210Pb profiles.
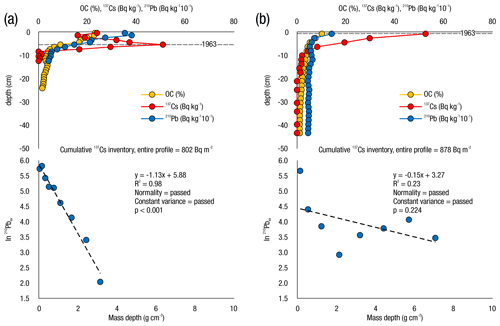
Figure 3Examples of 137Cs and 210Pb classifications showing OC (%), 137Cs (Bq kg−1), and 210Pb (Bq kg−1) depth profiles and plots of log-transformed 210Pbex against mass depth (g cm−2): (a) high-quality 137Cs and high-quality 210Pb (S-LO-I-W3-T1-CW-R1) and (b) high-quality 137Cs and low-quality 210Pb (M-OA-I-W4-T3-CW-R3).
Classification of the selected 137Cs profiles: The 79 suitable 137Cs profiles were then classified into high- and low-quality using the following steps (Fig. 2a):
- 1.
The 137C depth profile and the shape of the peak were assessed. A clear and distinct peak associated with several points on both sides of the peak verified the 137Cs depth profile as high-quality (e.g., Fig. 3a).
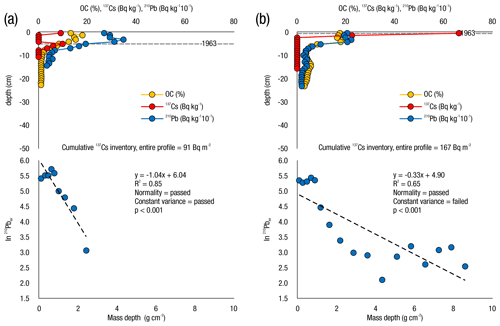
Figure 4Examples of 137Cs and 210Pb classifications showing OC (%), 137Cs (Bq kg−1), and 210Pb (Bq kg−1) depth profiles and plots of log-transformed 210Pbex against mass depth (g cm−2): (a) low-quality 137Cs and high-quality 210Pb (S-RO-I-W1-T3-CW-R3) and (b) low-quality 137Cs and low-quality 210Pb (S-RO-I-W6-T1-CW-R1).
- 2.
When analyzing sediment samples, a clear peak in 137Cs activity did not always exist (e.g., Fig. 4a). If a peak was absent, which could have resulted from sediment influxes with very high or very low 137Cs activity levels, the total 137Cs activity of the entire profile was examined. If the cumulative 137Cs inventory value for the entire profile was ≥ 500 Bq m−2, then the 137Cs profile was considered high-quality. Conversely, if the cumulative 137Cs inventory value for the entire profile was < 500 Bq m−2, the 137Cs profile was considered low-quality. The cut-off cumulative 137Cs inventory value of 500 Bq m−2 was established by assessing the 137Cs reference inventory value, the value of 137Cs present in a non-eroded system with an undisturbed profile. The 137Cs reference inventory value differs from region to region (Owens and Walling, 1996), and the most proximal regional value was used to select the cut-off 137Cs inventory value (Sutherland, 1991; Kachanoski and Von Bertoldi, 1996; Zarrinabadi et al., 2023). The 137Cs reference inventory is a catchment-wide reference value and not specific to the wetland center; thus, the cumulative 137Cs inventory value of 500 Bq m−2 was viewed as a conservative indicator of the suitability of the 137Cs profiles. Ideally, reference sites are large, open, level, non-eroded areas, usually in forage or grassland since the 1950s and within 10 km of the site of interest. In this study, it was impossible to identify a suitable reference site near every wetland; it is usually difficult to find reference sites in agricultural landscapes. However, we could locate reference sites used in other studies within 50 km except from nine wetlands in SK (51° N, 104° W), which were ∼ 150 km from the reference site. Although this was not considered ideal, it was considered acceptable.
Classification of the selected 210Pb profiles: The 47 suitable 210Pb profiles were classified into high- and low-quality profiles based on the following steps (Fig. 2b):
-
210Pb activity was plotted with a log-transformed 210Pbex against mass depth (g cm−2).
-
A linear regression analysis was performed (where the slope is used to derive the mass or sediment accumulation rate in ).
-
If the linear regression passed both normality and constant variance tests and had an R2 > 0.5 and a p value < 0.05, then the 210Pb profile was classified as high-quality (e.g., Fig. 3a).
-
If either normality or constant variance tests were not passed, with an R2 ≤ 0.5 or a p value ≥ 0.05, then the 210Pb profile was considered low-quality (e.g., Fig. 3b).
The 210Pb profiles were also classified using a two-step piecewise linear regression model to capture recent shifts in OC sequestration rates. However, no significant improvement was observed. Consequently, 210Pb-based OC sequestration rates were derived from the linear regression line. An R2 > 0.5 was selected as the cut-off for selecting high-quality over low-quality profiles. Increasing the cut-off R2 value may produce better profiles to be chosen for the study. Still, it can reduce the number of available sediment cores and potentially ignore the natural variability and significant events occurring in the real environment.
2.4 Organic carbon stocks and sequestration rates
Radioisotope activity measurements were utilized to assign two time markers, one for 1954 and the other for 1963, in the sediment cores. Sediment radioisotope dating was used to calculate the rates of sediment or mass accumulation and OC sequestration.
For 137Cs dating, sediment accumulation and OC sequestration rates () were estimated using the cumulative sum of sediment or OC (Mg ha−1) from the surface to the depth corresponding to the time markers of 137Cs of each core and dividing by the number of years from the time marker to the years the samples were collected. Unit conversion is applied to report the OC sequestration rate estimates in from for easy standardization and comparability with other studies. For 137Cs profiles with noisy peaks and comparatively larger cumulative 137Cs inventory values, the first elongated peak with a sharp rise after the onset of the 137Cs peak was considered the 1963 peak instead of the peak with the highest activity in the profile.
For 210Pb dating, mass or sediment accumulation and OC sequestration rates were estimated using the constant flux–constant sedimentation (CFCS) model (Sanchez-Cabeza and Ruiz-Fernandez, 2012; Kamula et al., 2017). Here, 210Pbex was estimated by subtracting 226Ra activity (186 keV) from the total 210Pb activity. The CFCS model uses the log-linear relationship of 210Pbex with mass depth and converts 210Pbex to the mass or sediment accumulation rate and, consequently, the OC sequestration rate. The OC stock was estimated by taking the cumulative sum of OC (Mg ha−1) from the surface of each sediment core to the depth increments represented by the time marker (e.g., 1963).
OC stocks for the 1954 and 1963 time markers were calculated by multiplying the OC content per unit mass of soil (g). Here, OC content was calculated from OC concentration (%) measured by the loss-on-ignition (LOI) method (Kolthoff and Sandell, 1952; Dean, 1974) by the mass of sediment for each section interval and specific depth interval per unit area (g cm−2) down the profile to the respective time marker. OC (%) was calculated by multiplying organic matter (%) by LOI with 0.58, assuming 58 % of the organic matter is carbon. Despite the broad applicability, simplicity in measurement techniques, and cost-effectiveness, the LOI approach is associated with some limitations, such as the ignition of non-organic particles at high temperatures or the use of a conventional conversion factor (Pribyl, 2010; Hoogsteen et al., 2015), which can result in overestimation of OC content.
2.5 Statistical analysis
Statistical analyses used sediment cores with 137Cs- and 210Pb-based OC sequestration rates available (number of sediment cores (n) = 44). The 137Cs- and 210Pb-based estimates of OC sequestration rates were compared using a quantile–quantile (Q–Q) plot. Firstly, the comparison was done via assessment of the Q–Q plots. Four sample datasets were used to construct Q–Q plots to compare the distribution of 137Cs- and 210Pb-based OC sequestration against the 1:1 line.
The sample datasets included the following:
-
D1, all suitable 137Cs and 210Pb profiles with OC sequestration rates estimated since 1954 (n = 44),
-
D2, all suitable 137Cs and 210Pb profiles with OC sequestration rates estimated since 1963 (n = 44),
-
D3, high-quality 137Cs and 210Pb profiles with OC sequestration rates estimated since 1954 (n = 30),
-
D4, high-quality 137Cs and 210Pb profiles with OC sequestration rates estimated since 1963 (n = 30).
A Q–Q plot was calculated for each dataset. The x and y coordinates of a point in a Q–Q plot corresponded to the path percentiles of the two OC sequestration rate estimates being compared in the plot. Here, p = , where n is the sample size and (Jain et al., 2007). The distribution of the points on the Q–Q plot was compared against the y=x (1:1) line to assess whether the two OC sequestration rate estimates are similar. If the points were distributed in a straight line and close to a 1:1 line, then it suggested that the two estimates came from the same distribution. In contrast, if the points were not distributed in a straight line or deviated from the 1:1 line, then it suggested that the two estimates did not come from the same distribution. The Q–Q plots were generated in Microsoft Excel (Microsoft 365, Version 2402, Redmond, WA).
Since interpreting the Q–Q plot through a visual inspection can be subjective to human perception, we compared the 137Cs- and 210Pb-based OC sequestration rate estimates using a distance sampling model. A distance sampling model captures how the detectability of objects from the observer (walking along a straight line) decreases with the increase in the object-to-observer distance. If the objects are closely distributed along the observer's path (i.e., if points of the Q–Q plot were closely distributed along the 1:1 line), then the distribution of the distances is expected to be a half-normal distribution. The Cramer–von Mises test was used to estimate whether the distances () from the points to the 1:1 line were from a half-normal distribution. Given a set of distance samples () and a detection function, the Cramer–von Mises test builds a model that fits the distance sampling data to the detection function (for details on modeling, see Miller et al., 2019). A half-normal key is commonly used as a detection function, corresponding to a half-normal distribution's shape.
The Cramer–von Mises test produced a p value and Akaike's information criterion (AIC) as its test statistics. A p value larger than the significant level (p = 0.05) indicated that the likelihood of points being observed closer to the 1:1 line is high and that the probability decreases as the distances increase. This provided evidence of the points being closely distributed along the 1:1 line. The AIC was used to rank the distance sampling models, which are built by the Cramer–von Mises tests, from best to worst (e.g., Burnham and Anderson, 2003): a small AIC value indicates a good fit to the half-normal key and thus provides evidence that the points are close to the 1:1 line (Miller et al., 2019). The distance-sampling Cramer–von Mises test was computed using the “distance” package in R version 4.0.3 (Miller and Clark-Wolfe, 2023; R Core Team, 2023).
3.1 High- and low-quality 137Cs and 210Pb profiles
Of the 79 suitable 137Cs profiles, 62 (78 %) were classified as high-quality. Of the 62 high-quality 137Cs profiles, 61 % had clear and distinct peaks, with a smooth rise and decline. In contrast, the remaining 39 % had noise – either one-sided peaks or disturbed peaks (e.g., Fig. 4). Of the 62 high-quality 137Cs profiles, 4 (6.5 %) were repositioned to capture the 137Cs-enriched sediments post-1963 (e.g., 137Cs profile of S-LO-I-W4-T2-CW-R2 in Fig. S2a in the Supplement). In these profiles, which had a cumulative 137Cs inventory value > 1200 Bq m−2, the depth that corresponded to the 137Cs cumulative inventory value of ∼ 500 Bq m−2 was regarded as the 1963 time marker. The high total quantities of 137Cs profile inventories can be attributed to receiving 137Cs-enriched sediments from the surrounding landscape. Sediments that have undergone substantial preferential detachment and entrainment on their pathway into a wetland can have very high concentrations of 137Cs and, when interlayered with sediments that are not so enriched, can generate multiple 137Cs peaks in the sediment profile peaks after 1963. These observed multiple peaks are local and not regional, ruling out the association with the Chernobyl and Fukushima events. Two 137Cs profiles were considered high-quality despite a cumulative 137Cs inventory value < 500 Bq m−2 because the 1963 peak was clear, distinct, and elongated with two to three points on both sides of the peak (e.g., 137Cs profile of M-OA-I-W4-T2-CW-R2 in Fig. S7b in the Supplement). One 137Cs profile was considered high-quality despite showing marginal quality to the set criteria in the decision framework, where the peak profile had good shape with several points on the downside of the peak and one point on the other side and had a cumulative 137Cs inventory value of 499 Bq m−2. One 137Cs profile was classified as low-quality despite a cumulative 137Cs inventory value > 500 Bq m−2 because the peak was highly fluctuating and not discernible (e.g., 137Cs profile of O-AL-I-W6-T1-CW-R1 in Fig. S12b in the Supplement).
Of the 47 210Pb profiles, 40 (85 %) were classified as high-quality.
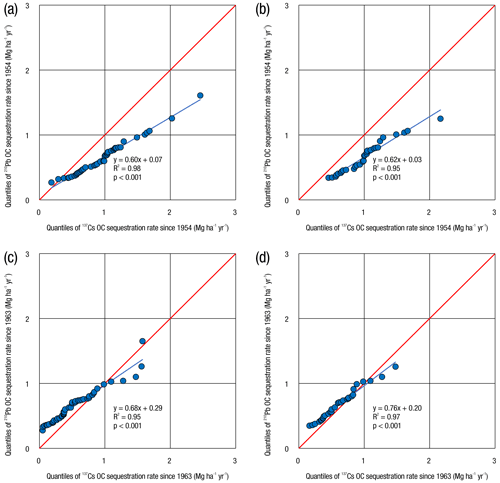
Figure 5Q–Q plot of 137Cs- vs. 210Pb-based organic carbon (OC) sequestration rates using (a) all suitable 137Cs and 210Pb profiles estimated since 1954 (D1), (b) high-quality 137Cs and 210Pb profiles estimated since 1954 (D3), (c) all suitable 137Cs and 210Pb profiles estimated since 1963 (D2), and (d) high-quality 137Cs and high-quality 210Pb profiles estimated since 1963 (D4).
There were 44 sediment cores with both 137Cs- and 210Pb-suitable profiles available. Of these, 30 were categorized as high-quality 137Cs and high-quality 210Pb (Fig. 3a), 6 were categorized as high-quality 137Cs and low-quality 210Pb (Fig. 3b), 7 were classified as low-quality 137Cs and high-quality 210Pb (Fig. 4a), and 1 was categorized as low-quality 137Cs and low-quality 210Pb (Fig. 4b); see Figs. S1 to S12 in the Supplement for 137Cs and 210Pb profiles in all study wetlands.
3.2 137Cs- vs. 210Pb-derived organic carbon sequestration rates
For each of the four datasets (D1–D4), the points on the Q–Q plot were distributed in a straight line, showing a linear relationship between the two estimates being compared (R2 > 0.95, p value < 0.001) (Fig. 5).
Visual inspection of the Q–Q plots showed that the points for D2 (i.e., all suitable 137Cs and 210Pb profiles using the 1963 time marker; Fig. 5c) and D4 (i.e., high-quality 137Cs and 210Pb profiles using the 1963 time marker; Fig. 5d) were distributed more closely along the 1:1 line compared to those of D1 (i.e., all suitable 137Cs and 210Pb profiles using the 1954 time marker; Fig. 5a) and D3 (i.e., high-quality 137Cs and 210Pb profiles using the 1954 time marker; Fig. 5b).
Table 2Sedimentation accumulation, organic carbon (OC) sequestration rates, and OC stocks of undisturbed wetlands estimated using high-quality 137Cs and high-quality 210Pb profiles.

An intercept closer to 0 and a slope closer to 1 indicated good alignment of the regression line to the 1:1 line. The slope (s) and intercept (i) of the regression lines were: s = 0.60, i = 0.07 for D1 (Fig. 5a); s = 0.62, i = 0.03 for D3 (Fig. 5b); s = 0.68, i = 0.29 for D2 (Fig. 5c); and s = 0.76, i = 0.20 for D4 (Fig. 5d). D2 and D4 had regression lines and slopes closer to the 1:1 line but intercepts further from the origin than D1 and D3.
The Cramer–von Mises test was used to build distance-sampling models using the point-to-1:1-line distances computed from the Q–Q plots. Models built with the D4 dataset produced the best-fit model (i.e., p value > 0.05, AIC = −114). Models built with the D1, D2, and D3 datasets had weaker p values (p value < 0.05) and can be ranked based on lower AIC scores (AIC = −116 for D2, AIC = −54 for D1, and AIC = −34 for D3).
3.3 Sediment accumulation, organic carbon sequestration rates, and organic carbon stocks
The 30 sediment cores (68 % of all the suitable 137Cs and 210Pb profiles) with high-quality 137Cs and 210Pb profiles were used to calculate mass or sediment accumulation rates, OC sequestration rates, and OC stocks (Table 2). OC sequestration rates based on 137Cs and 210Pb dating estimated since 1954 and 1963 of 44 suitable sediment cores (where both 137Cs and 210Pb profiles were available) are presented in Table S2 in the Supplement.
Based on the 1954 time marker, the total sediment accumulation ranged from 214–1727 Mg ha−1 using 137Cs dating and 111–1014 Mg ha−1 using 210Pb dating. In contrast, the total sediment accumulation based on the 1963 time marker was lower, ranging from 56–1272 Mg ha−1 using 137Cs dating and 95–874 Mg ha−1 using 210Pb dating.
The 137Cs-derived mean OC sequestration rate was almost 2 times larger, at 1.02 using the 1954 time marker compared to 0.63 using the 1963 time marker. The corresponding 137Cs-based mean OC stocks were 66 Mg ha−1 for 1954 and 35 Mg ha−1 for 1963 (Table 2).
The 210Pb-derived mean OC sequestration rate was similar at 0.67 using the 1954 time marker compared to 0.68 using the 1963 time marker. 210Pb-based OC sequestration rates show minimal variation, since they were calculated using the same sedimentation rate. The corresponding mean OC stocks were 43 Mg ha−1 for the 1954 time marker and 38 Mg ha−1 for the 1963 time marker, with a variable depth.
Figure 3 and Figs. S1 to S12 in the Supplement present the depth distributions of 137Cs and 210Pb activity (along with the linear plots of log-transformed 210Pbex against mass depth in g cm−2) of all suitable profiles (n = 44) where both radioisotope profiles are available.
This study compared 137Cs and 210Pb dating for OC estimates in wetlands that were undisturbed (i.e., without direct impact human activities), since the dating of both radioisotopes is known to provide reliable forecasts for recent OC sequestration rates (i.e., post-1954, which coincides with the onset of 137Cs atmospheric deposition) (Drexler et al., 2018; Creed et al., 2022).
This study highlights some advantages and disadvantages of using 137Cs vs. 210Pb dating. For example, the smaller number of suitable 210Pb profiles ( = 52 %) due to the lack of a complete decay profile (following the CFCS model as described in Sanchez-Cabeza and Ruiz-Fernandez, 2012) indicates that 210Pb dating is more prone to disturbance than 137Cs dating ( = 88 %). For 137Cs, even if the sediment core is disturbed, estimation of OC sequestration rates may be possible with careful interpretation (e.g., see Fig. 2). The larger number of sediment cores using 137Cs dating can be beneficial in accurately representing the heterogeneity of OC sequestration rates, as it provides a larger dataset (a 36 % gain compared to 210Pb).
Table 3The advantages and disadvantages of using 137Cs and unsupported 210Pb (210Pbex) to estimate wetland organic carbon (OC) sequestration rates.
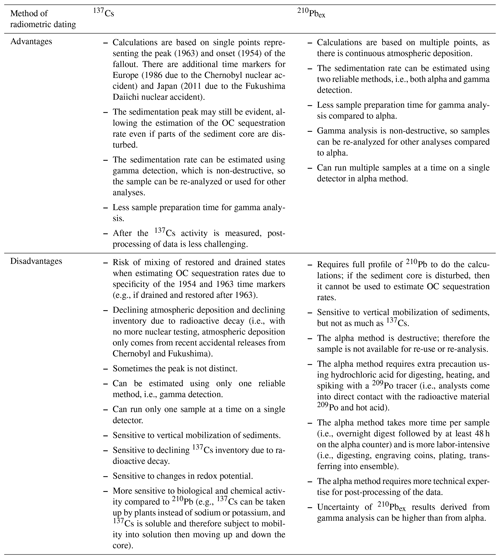
Other advantages and disadvantages of 137Cs vs. 210Pb radioisotope dating are presented in Table 3. 137Cs deposition was a pulse that occurred in 1954 and 1963. At the 1963 peak, the activity declined with time because of two factors: (1) peak natural radioactive decay, with the 137Cs 30-year half-life reducing the peak size over time, and (2) peak attenuation due to physical, chemical, or biological reasons (Drexler et al., 2018). The declining 137Cs activity limits its applicability as a radioisotope dating tool; however, recent studies have reported adequate 137Cs reference inventories for Canadian landscapes (Sutherland, 1991; Kachanoski and Von Bertoldi, 1996; Li et al., 2008; Mabit et al., 2014; Zarrinabadi et al., 2023). In addition, the use of the 137Cs inventory for dating to complement the peak has addressed the potential inadequacies that could be attributed to declining peak resolution with time. 137Cs dating is advantageous for its simplicity in pre- and post-processing of samples and the presence of additional time markers in other regions (Breithaupt et al., 2018; Foucher et al., 2021). For example, additional time markers correspond to the 1986 Chernobyl nuclear plant accident and the 2011 Fukushima accident. However, their effect has yet to be recorded in North America due to the substantial distance from the source. Recognizing that there may be regional or local variation in peaks, we used non-eroded 137Cs reference sites to deal with regional variation in deposition. We also used multiple sampling sites within wetlands to assess local variation in deposition. Furthermore, we looked for evidence from Chernobyl and Fukushima nuclear events in our data but found none (data not shown).
Furthermore, we looked for evidence from the Chernobyl and Fukushima nuclear events in our data but found none (data not shown). 137Cs dating is best suited for where the total OC is sequestered since a fixed time marker (1954 onset or 1963 peak) or where the average OC sequestration rate is needed. In contrast, the atmospheric deposition of 210Pb is continuous and therefore not limited in its applicability as a radioisotope dating tool. 210Pb dating is best suited for where variable OC sequestration rates are needed over a more extended period (earlier than 1954). 210Pb dating is advantageous because its calculations are based on multiple points associated with progressive OC sequestration rates derived using a constant rate of supply model – including the 1954 onset and 1963 peak of 137Cs activity – improving the precision of the OC sequestration rates. This precision enables the estimation of OC sequestration rates when wetlands are not undisturbed (history of drainage or at different ages since restoration) and when they are undisturbed (no history of drainage).
4.1 Challenges in interpreting the 137Cs peak
A potential weakness of 137Cs radioisotope dating arises from the challenges in interpreting the disturbed 1963 peak. The noise in the 1963 peak in wetlands on agricultural landscapes can be due to the redistribution of sediments, since wetlands are susceptible to receiving a large mass of sediments resulting from various erosional processes due to their positioning within the landscape (Lobb, 2011; Zarrinabadi et al., 2023). Soil erosion resulting from wind, water, and tillage can lead to higher or lower 137Cs levels (Li et al., 2010; Foucher et al., 2021; Zarrinabadi et al., 2023) in wetlands in agricultural landscapes. If 137Cs-enriched soil from the surrounding landscape gets deposited on top of the wetland's original soil layer, it can increase the 137Cs inventory value (Walling and Quine, 1991; Li et al., 2010). The magnitude of 137Cs enrichment depends on whether sediment comes from surface or sub-surface layers (Li et al., 2010; Lal, 2020). For example, if the wetland receives 137Cs-enriched topsoil post-1963, the 137Cs inventory would be higher than the 137Cs-depleted subsoil.
The screening of 137Cs profiles (Fig. 2a) considered the redistribution of sediments within the landscape. It demonstrated that the difficulty in disturbed 137C profile interpretation can be reduced by investigating the cumulative 137C inventory value. A cut-off cumulative 137Cs inventory value can help exclude questionable profiles. The range of 137Cs reference inventory values from previous erosion studies within the study area (e.g., Sutherland, 1991; Kachanoski and Von Bertoldi, 1996; Zarrinabadi et al., 2023) can help in establishing and setting the cut-off cumulative 137Cs inventory value. The mean 137Cs reference inventory values in the four provinces of Canada where our wetland sites are located were utilized in this instance. The mean 137Cs reference inventory value is estimated to be 1684 Bq m−2 (coefficient of variation (CV) = 49 %) for three AB wetland sites (53° N, 113° W) (Zarrinabadi et al., 2023), 989 Bq m−2 (CV = 20 %) for seven SK wetland sites (51° N, 107° W) (Sutherland, 1991), 1008 Bq m−2 (CV = 17.9 %) for nine SK wetland sites (51° N, 104° W) (Sutherland, 1991), 1430 Bq m−2 (CV = 8.6 %) for five MB wetland sites (50° N, 100° W) (Zarrinabadi et al., 2023), 1447 Bq m−2 (CV = 8.8 %) for three ON wetland sites (43.3° N, 80.3° W) (Kachanoski and Von Bertoldi, 1996), and 1534 Bq m−2 (CV = 1.7 %) for three ON wetland sites (45.6° N, 74.8° W) (Kachanoski and Von Bertoldi, 1996). The 137Cs reference inventory values were decay-corrected to 2021 for comparability. The cut-off cumulative 137Cs inventory value for this study was selected by checking the minimum 137Cs reference inventory value of the local region, i.e., 546 Bq m−2 (using values reported in Sutherland, 1991; Kachanoski and Von Bertoldi, 1996; and Zarrinabadi et al., 2023). Hence, any 137Cs inventory value less than 500 Bq m−2 was considered questionable and low-quality. Additionally, > 75 % of 137C profiles had a cumulative 137Cs inventory value of > 500 Bq m−2, indicating that the 137Cs reference inventory value of our wetland sites is most likely around 500 Bq m−2.
Variations in the 137Cs peak types (e.g., distinct, broadened, fluctuating) and in 137Cs inventory values in this study suggested that the 137Cs profiles were impacted by various regional erosional processes in the surrounding agricultural landscape. Recent evidence suggests that there may be an outward movement of sediment and 137Cs from the center of the wetlands to the riparian area (Zarrinabadi et al., 2023), suggesting that the base 137Cs inventory value observed in the center of wetlands from atmospheric deposition in the 1950s–1960s could be less than that of the non-eroded reference 137Cs values from the surrounding catchment. A 137Cs inventory of a sediment core can further help assign the 137Cs peak. For example, the 137Cs peak was repositioned in disturbed sediment cores with a higher 137Cs inventory, where the first discernable peak after the sharp rise from the onset of 137Cs activity and exceeding or around the reference value was assumed to be the original 137Cs peak. 239+240Pu isotopes, like 137Cs, are a product of nuclear testing and can be used to identify the peak of 137Cs. Future research will use 239+240Pu to replace 137Cs as 137Cs levels diminish.
4.2 Challenges in interpreting 137Cs and 210Pb profiles
Mobilization of 137Cs and 210Pb in the sediment often occurs in wetlands. Vertical mixing of 137Cs within sediments can be caused by remobilization and redistribution by wind and water, ice movement and inversion, disturbance by animals, and disturbance by humans who ditch and drain the wetlands when they are dry and let cattle access them for water, which causes disturbances to the bottom sediments (Robbins et al., 1977; Milan et al., 1995; Takahashi et al., 2015). Vertical mixing affects the profile by attenuating the peak upward and downward (which we addressed using the 137Cs inventory value and not just the peak when assessing the profile). Horizontal mixing of 137Cs dating within sediment occurs by physical movement of sediments into or out of the wetland, causing uneven distribution of the OC content, where accumulation may be high at the edges of open water of the wetland (Lobb, 2011; Zarrinabadi et al., 2023). This heterogeneity can be caused by the horizontal focusing of sediments in sub-basins within a wetland, i.e., multiple center points. Sampling multiple sediment cores from individual wetlands can help capture the heterogeneity within the wetland. Suppose the 137Cs activity of most of the sediment cores from a particular wetland is noisy with a higher inventory value (e.g., 137Cs profile of S-LO-I-W4-T2-CW-R2 in Fig. S2a in the Supplement). In that case, the impact by erosional processes can be deduced with higher certainty. The higher observed inventory value could result from the movement of enriched material via erosion/lateral flow to the center of the wetland, increasing the amount of 137Cs. In this study, the assumption of no substantial downward mixing of 137Cs was supported by (1) sampling three cores from each wetland, (2) assessing the sharpness of the rise of the peaks (a sharp rise means negligible mixing), (3) examining the cumulative 137Cs inventory value and validating against the known reference level, (4) classifying 137Cs profiles, and (5) corroborating with 210Pb dating.
4.3 137Cs- vs. 210Pb-derived organic carbon sequestration rates and stocks
137Cs radioisotope dating using the 1954 or 1963 time markers gives reasonable estimates of OC sequestration rates as compared to 210Pb radioisotope dating. The 137Cs–210Pb Q–Q plot of the 1963 OC sequestration rates is closer to the 1:1-line, suggesting compatibility between 137Cs- and 210Pb-based estimates (Fig. 5c and d). Conversely, the 137Cs–210Pb Q–Q plot of the 1954 OC sequestration rates showed more deviation from the 1:1 line; 137Cs-based OC sequestration rates were more dispersed and were higher than the 210Pb-based OC sequestration rates (Fig. 5a and b). The mean OC sequestration rates in Table 2 further verify the comparability of OC sequestration rates using the 1963 time marker (the mean 137Cs OC sequestration rate is 0.63 , while the mean 210Pb OC sequestration rate is 0.68 ). For the dispersion using the 1954 time marker, the mean 137Cs OC sequestration rate is 1.02 , while the mean 210Pb OC sequestration rate is 0.67 . Providing better sequestration rate estimates has consequences for estimating OC stocks with an improved degree of accuracy, which may provide policymakers with better tools to make informed carbon management decisions supported with data.
To put our findings into practice and into the broader OC sequestration perspective, we consider a scenario where two independent studies were performed using 137Cs and 210Pb (with the CFCS model) at the exact locations. If the cores were not selected based on the criteria we used to choose high-quality profiles, then these two studies' OC sequestration rate estimates are likely to disagree. However, we know and have demonstrated through our findings that they are linearly dependent and that the equation of our linear regression lines may be used to transform one estimate to the other. Consequently, if the cores were selected based on our selection criteria, then one can expect the OC sequestration rate estimates to have similar values, which alleviates the interpretation challenges of having two different estimates from two independent studies. This observation may help with consistency when disagreements in estimates are observed. Another practical application of our findings may be in data augmentation. For example, if we have 210Pb data for a set of locations and 137Cs data for other locations, the linear regression equation could transform 210Pb data to augment 137Cs data and vice versa. This can help data-driven modeling approaches, whereas larger datasets help achieve robust modeling tools. Similarly, because OC stocks can be derived from sequestration rates for specific years, estimates derived using one radioisotope can be used to estimate OC from a dataset derived from another estimate, further contributing to the augmentation of the corresponding OC stock data.
Based on the results of this study, we recommend (1) using high-quality 137Cs and 210Pb profiles to estimate OC sequestration rates, (2) carefully interpreting 137Cs profiles from agricultural landscapes from the perspective of redistribution of sediments, and (3) using both 137Cs and 210Pb to compare and validate estimates if logistically possible. However, in cases where one has to choose between 137Cs and 210Pb, we recommend the following: (1) for 137Cs, use 1963 time markers to estimate OC sequestration rates (compared to 1954), since it is found to be most comparable with 210Pb dating techniques (CFCS model); (2) for 210Pb (CFCS model), OC sequestration rates from 1963 to present can be estimated with the highest precision, since we corroborated the estimates with 137Cs. However, we cannot comment on the precision of 210Pb-based OC sequestration rate estimation before 1963 based on the scope of this study.
Information regarding OC sequestration rates within temperate inland wetland soils is crucial for evaluating the potential of these ecosystems to serve as natural climate solutions. Radiometric dating using 137Cs and 210Pb presents a valuable tool for estimating the recent OC sequestration potential of wetlands. Notably, a robust 1:1 linear correlation has been observed between 137Cs- and 210Pb-based OC sequestration rates in high-quality sediment profiles.
While estimations based on the onset of 137Cs in 1954 or its peak in 1963 were reasonable, estimates anchored to the 1963 peak of 137Cs exhibited closer alignment with those derived from 210Pb data (using the CFCS model). These findings suggest that estimates derived from 137Cs and 210Pb radioisotope dating methods are interchangeable and reasonably comparable when utilizing the 1963 137Cs time marker.
Combining 137Cs and 210Pb tracers provides a comprehensive assessment of sedimentation rates. While one tracer offers an average sedimentation rate over 60 years, the other provides a temporal trend over the same period. This interchangeability enables more thorough evaluations of the average sedimentation rate in wetlands, which is crucial for leveraging them as natural climate solutions.
The R code for the distance sampling modeling, along with the data to run the code, is available at https://doi.org/10.5281/zenodo.10951658 (Mistry et al., 2024). The organic carbon (OC) sequestration rate data used to check the comparability of the radioisotope profiles can be found in the Supplement. These sequestration rate data and the geographical locations, years of sampling, and additional information about the sediment cores are available at https://doi.org/10.5281/zenodo.13696300 (Creed et al., 2024). The radioisotope profiles used for screening are in the paper and Supplement. The paper and Supplement present other relevant data to support our conclusion.
The supplement related to this article is available online at: https://doi.org/10.5194/bg-21-4699-2024-supplement.
The authors' contributions are as follows. PM: methodology, field and lab analysis, statistical analysis and modeling, and writing. IFC: conceptualization, methodology, field and lab analysis, editing, and supervision. CGT: conceptualization, editing, and supervision. EE: methodology, field and lab analysis, and editing. DAL: methodology, field and lab analysis, and editing.
The contact author has declared that none of the authors has any competing interests.
Publisher's note: Copernicus Publications remains neutral with regard to jurisdictional claims made in the text, published maps, institutional affiliations, or any other geographical representation in this paper. While Copernicus Publications makes every effort to include appropriate place names, the final responsibility lies with the authors.
Purbasha Mistry was supported by a Natural Sciences and Engineering Research Council of Canada Canadian Graduate Scholarship, a Saskatchewan Innovation and Opportunity Scholarship, a PhD scholarship from the School of Environment and Sustainability at the University of Saskatchewan, and a Wanda Young Scholarship. The authors thank Jacqueline Serran, Kevin Erratt, Oscar Senar, Ehsan Zarrinabadi, and many others for assisting in field sampling.
This research has been supported by the Natural Sciences and Engineering Research Council of Canada (grant no. STPGP 506809), awarded to Irena F. Creed, David A. Lobb, and Charles G. Trick.
This paper was edited by Ji-Hyung Park and reviewed by two anonymous referees.
Andersen, T. J., Mikkelsen, O. A., Møller, A. L., and Pejrup, M.: Deposition and mixing depths on some European intertidal mudflats based on 210Pb and 137Cs activities, Cont. Shelf Res., 20, 1569–1591, https://doi.org/10.1016/S0278-4343(00)00038-8, 2000. .
Appleby, P. G. and Oldfield, F.: The calculation of lead-210 dates assuming a constant rate of supply of unsupported 210Pb to the sediment, Catena, 5, 1–8, https://doi.org/10.1016/S0341-8162(78)80002-2, 1978.
Appleby, P. G., and Oldfield, F.: The assessment of 210Pb data from sites with varying sediment accumulation rates, Hydrobiologia, 103, 29–35, https://doi.org/10.1007/BF00028424, 1983.
Bansal, S., Creed, I. F., Tangen, B. A., Bridgham, S. D., Desai, A. R., Krauss, K. W., Neubauer, S. C., Noe, G. B., Rosenberry, D. O., Trettin, C., Wickland, K. P., Allen, S. T., Arias-Ortiz, A., Armitage, A. R., Baldocchi, D., Banerjee, K., Bastviken, D., Berg, P., Bogard, M., Chow, A. T., Conner, W. H., Craft, C., Creamer, C., DelSontro, T., Duberstein, J. A., Eagle, M., Fennessy, M. S., Finkelstein, S. A., Göckede, M., Grunwald, S., Halabisky, M., Herbert, E., Jahangir, M. M. R., Johnson, O. F., Jones, M. C., Kelleway, J. J., Knox, S., Kroeger, K. D., Kuehn, K. A., Lobb, D., Loder A. L., Ma, S., Maher, D. T., McNicol, G., Meier, J., Middleton, B. A., Mills, C., Mistry, P., Mitra, A., Mobiian, C., Nahlik, A. M., Newman, S., O'Connell, J. L., Oikawa, P., Post van der Burg, M., Schutte, C. A., Song, C., Stagg, C. L., Turner, J., Vargas, R., Waldrop, M. P., Wallin, M. B., Wang, Z. A., Ward, E. J., Willard, D. A., Yarwood, S., and Zhu X.: Practical guide to measuring wetland carbon pools and fluxes, Wetlands, 43, 105, https://doi.org/10.1007/s13157-023-01722-2, 2023.
Bellucci, L. G., Frignani, M., Cochran, J. K., Albertazzi, S., Zaggia, L., Cecconi, G., and Hopkins, H.: 210Pb and 137Cs as chronometers for salt marsh accretion in the Venice Lagoon–links to flooding frequency and climate change, J. Environ. Radioactiv., 97, 85–102, https://doi.org/10.1016/j.jenvrad.2007.03.005, 2007.
Bernal, B. and Mitsch, W. J.: Comparing carbon sequestration in temperate freshwater wetland communities, Glob. Change Biol., 18, 1636–1647, https://doi.org/10.1111/j.1365-2486.2011.02619.x, 2012.
Bernal, B. and Mitsch, W. J.: Carbon sequestration in two created riverine wetlands in the Midwestern United States, J. Environ. Qual., 42, 1236–1244, https://doi.org/10.2134/jeq2012.0229, 2013.
Breithaupt, J. L., Smoak, J. M., Byrne, R. H., Waters, M. N., Moyer, R. P., and Sanders, C. J.: Avoiding timescale bias in assessments of coastal wetland vertical change, Limnol. Oceanogr., 63, S477–S495, https://doi.org/10.1002/lno.10783, 2018.
Bridgham, S. D., Megonigal, J. P., Keller, J. K., Bliss, N. B., and Trettin, C.: The carbon balance of North American wetlands, Wetlands, 26, 889–916, https://doi.org/10.1672/0277-5212(2006)26[889:TCBONA]2.0.CO;2, 2006.
Burnham K. P. and Anderson D. R. (Eds.): Model Selection and Multimodel Inference: A Practical Information-Theoretic Approach, Springer, https://doi.org/10.1007/b97636, 2003.
Craft, C. B. and Casey, W. P.: Sediment and nutrient accumulation in floodplain and depressional freshwater wetlands of Georgia, USA, Wetlands, 20, 323–332, https://doi.org/10.1672/0277-5212(2000)020[0323:SANAIF]2.0.CO;2, 2000.
Craft, C. B. and Richardson, C. J.: Recent and long-term organic soil accretion and nutrient accumulation in the Everglades, Soil Sci.Soc. Am. J., 62, 834–843, https://doi.org/10.2136/sssaj1998.03615995006200030042x, 1998.
Craft, C., Vymazal, J., and Kröpfelová, L.: Carbon sequestration and nutrient accumulation in floodplain and depressional wetlands, Ecol. Eng., 114, 137–145, https://doi.org/10.1016/j.ecoleng.2017.06.034, 2018.
Creed, I. F., Badiou, P., Enanga, E., Lobb, D. A., Pattison-Williams, J. K., Lloyd-Smith, P., and Gloutney, M.: Can restoration of freshwater mineral soil wetlands deliver nature-based climate solutions to agricultural landscapes?, Frontiers in Ecology and Evolution, 10, 932415, https://doi.org/10.3389/fevo.2022.932415, 2022.
Creed, I., Mistry, P., Enanga, E., and Erratt, K.: Wetland sediment soil organic carbon sequestration data to support radiometric technique comparisons, Zenodo [data set], https://doi.org/10.5281/zenodo.13696300, 2024.
Dean, W. E.: Determination of carbonate and organic matter in calcareous sediments and sedimentary rocks by loss on ignition; comparison with other methods, J. Sediment. Res., 44, 242–248, https://doi.org/10.1306/74D729D2-2B21-11D7-8648000102C1865D, 1974.
DeLaune, R. D., Jugsujinda, A., Peterson, G. W., and Patrick Jr, W. H.: Impact of Mississippi River freshwater reintroduction on enhancing marsh accretionary processes in a Louisiana estuary, Estuar. Coastal Shelf S., 58, 653–662, https://doi.org/10.1016/S0272-7714(03)00177-X, 2003.
Drexler, J. Z., Fuller, C. C., and Archfield, S.: The approaching obsolescence of 137Cs dating of wetland soils in North America, Quaternary Sci. Rev., 199, 83–96, https://doi.org/10.1016/j.quascirev.2018.08.028, 2018.
Environment and Climate Change Canada (ECCC): Canadian Environmental Sustainability Indicators: Extent of Canada's Wetlands, ECCC, Gatineau, Quebec, https://www.ec.gc.ca/indicateurs-indicators/default.asp?lang=en&n=69E2D25B-1 (last access: 10 July 2024), 2016.
Foucher, A., Chaboche, P.-A., Sabatier, P., and Evrard, O.: A worldwide meta-analysis (1977–2020) of sediment core dating using fallout radionuclides including 137Cs and 210Pbxs, Earth Syst. Sci. Data, 13, 4951–4966, https://doi.org/10.5194/essd-13-4951-2021, 2021.
Hambäck, P. A., Dawson, L., Geranmayeh, P., Jarsjö, J., Kačergytė, I., Peacock, M., Collentine, D., Destouni, G., Futter, M., Hugelius, G., Hedman, S., Jonsson, S., Klatt, B. K., Lindström, A., Nilsson, J. E., Pärt, T., Schneider, L. D., Strand, J. A., Urrutia-Cordero, P., Åhlén, D., Åhlén., I., and Blicharska, M.: Tradeoffs and synergies in wetland multifunctionality: A scaling issue, Sci. Total Environ., 862, 160746, https://doi.org/10.1016/j.scitotenv.2022.160746, 2023.
Hoogsteen, M. J., Lantinga, E. A., Bakker, E. J., Groot, J. C., and Tittonell, P. A.: Estimating soil organic carbon through loss on ignition: effects of ignition conditions and structural water loss, Eur. J. Soil Sci., 66, 320–328, https://doi.org/10.1111/ejss.12224, 2015.
Jain, J. L., Mohanty, S. G., and Böhm, W.: A Course on Queueing Models, Chapman and Hall/CRC, https://doi.org/10.1201/b15892, 2007.
Kachanoski, R. G. and Von Bertoldi, P.: Monitoring soil loss and redistribution using 137Cs, COESA Report No. RES/MON-008/96, Green Plan Research Sub-program, Agriculture and Agri-Food Canada, London, Ontario, 1996.
Klaminder, J., Appleby, P., Crook, P., and Renberg, I.: Post-deposition diffusion of 137Cs in lake sediment: Implications for radiocaesium dating, Sedimentology, 59, 2259–2267, https://doi.org/10.1111/j.1365-3091.2012.01343.x, 2012.
Kamula, C. M., Kuzyk, Z. Z. A., Lobb, D. A., and Macdonald, R. W.: Sources and accumulation of sediment and particulate organic carbon in a subarctic fjord estuary: 210Pb, 137Cs, and δ13C records from Lake Melville, Labrador, Can. J. Earth Sci., 54, 993–1006, https://doi.org/10.1139/cjes-2016-0167, 2017.
Kolthoff, I. M. and Sandell, E. B.: Textbook of quantitative inorganic analysis, 3rd edn., The Macmillan Company, https://doi.org/10.1002/sce.3730380480, 1952.
Lal, R.: Soil erosion and gaseous emissions, Appl. Sci., 10, 2784, https://doi.org/10.3390/app10082784, 2020.
Li, S., Lobb, D. A., Lindstrom, M. J., and Farenhorst, A.: Tillage and water erosion on different landscapes in the northern North American Great Plains evaluated using 137Cs technique and soil erosion models, Catena, 70, 493–505, https://doi.org/10.1016/j.catena.2006.12.003, 2007.
Li, S., Lobb, D. A., Lindstrom, M. J., and Farenhorst, A.: Patterns of water and tillage erosion on topographically complex landscapes in the North American Great Plains, J. Soil Water Conserv., 63, 37–46, https://doi.org/10.2489/jswc.63.1.37, 2008.
Li, S., Lobb, D. A., Tiessen, K. H., and McConkey, B. G.: Selecting and applying cesium-137 conversion models to estimate soil erosion rates in cultivated fields, J. Environ. Qual., 39, 204–219, 2010. https://doi.org/10.2134/jeq2009.0144, 2010.
Lobb, D. A.: Understanding and managing the causes of soil variability, J. Soil Water Conserv., 66, 175A–179A, https://doi.org/10.2489/jswc.66.6.175A, 2011.
Loder, A. L., and Finkelstein, S. A.: Carbon accumulation in freshwater marsh soils: A synthesis for temperate North America, Wetlands, 40, 1173–1187, https://doi.org/10.1007/s13157-019-01264-6, 2020.
Mabit L., Kieth, S. C., Dornhofer, P., Toloza, A., Benmansour, M., Bernard, C., Fulajtar, E., and Walling D. E.: 137Cs: A widely used and validated medium term soil tracer, Guidelines for Using Fallout Radionuclides to Assess Erosion and Effectiveness of Soil Conservation Strategies, IAEA-TECDOC-1741, International Atomic Energy Agency, Vienna, https://doi.org/10.13140/2.1.2586.1122, 2014.
Milan, C. S., Swenson, E. M., Turner, R. E., and Lee, J. M.: Assessment of the method for estimating sediment accumulation rates: Louisiana salt marshes, J. Coastal Res., 11, 296–307, https://www.jstor.org/stable/4298341 (last access: 7 March 2024), 1995.
Miller, D. L. and Clark-Wolfe, T. J.: Package “Distance”, Foundation for Statistical Computing, Vienna, https://cran.r-project.org/web/packages/Distance/Distance.pdf (last access: 13 April 2024), 2023.
Miller, D. L., Rexstad, E., Thomas, L., Marshall, L., and Laake, J. L.: Distance sampling in R, J. Stat. Softw., 89, 1–28, https://doi.org/10.18637/jss.v089.i01, 2019.
Mistry, P., Creed, I. F., Trick, C. G., Enanga, E., and Lobb, D. A.: R script for “Technical Note: Comparison of radiometric techniques for estimating recent organic carbon sequestration rates in inland wetland soils”, Zenodo [code], https://doi.org/10.5281/zenodo.10951658, 2024.
Nahlik, A. M. and Fennessy, M. S.: Carbon storage in US wetlands, Nat. Commun., 7, 1–9, https://doi.org/10.1038/ncomms13835, 2016.
Owens, P. N. and Walling, D. E.: Spatial variability of caesium-137 inventories at reference sites: an example from two contrasting sites in England and Zimbabwe, Appl. Radiat. Isotopes, 47, 699–707, https://doi.org/10.1016/0969-8043(96)00015-2, 1996.
Pennington, W., Tutin, T. G., Cambray, R. S., and Fisher, E. M.: Observations on lake sediments using fallout 137Cs as a tracer, Nature, 242, 324–326, https://doi.org/10.1038/242324a0, 1973.
Pribyl, D. W.: A critical review of the conventional SOC to SOM conversion factor, Geoderma, 156, 75–83, https://doi.org/10.1016/j.geoderma.2010.02.003, 2010.
R Core Team: R: A language and environment for statistical computing, R Foundation for Statistical Computing, Vienna, Austria, https://www.R-project.org/ (last access: 13 April 2024), 2023.
Ritchie, J. C. and McHenry, J. R.: Application of radioactive fallout cesium-137 for measuring soil erosion and sediment accumulation rates and patterns: A review, J. Environ. Qual., 19, 215–233, https://doi.org/10.2134/jeq1990.00472425001900020006x, 1990.
Robbins, J. A., Krezoski, J. R., and Mozley, S. C.: Radioactivity in sediments of the Great Lakes: post-depositional redistribution by deposit-feeding organisms, Earth Planet. Sc. Lett., 36, 325–333, https://doi.org/10.1016/0012-821X(77)90217-5, 1977.
Sanchez-Cabeza, J. A. and Ruiz-Fernández, A. C.: 210Pb sediment radiochronology: an integrated formulation and classification of dating models, Geochim. Cosmochim. Ac., 82, 183–200, https://doi.org/10.1016/j.gca.2010.12.024, 2012.
Sutherland, R. A.: Examination of caesium-137 areal activities in control (uneroded) locations, Soil Technol., 4, 33–50, https://doi.org/10.1016/0933-3630(91)90038-O, 1991.
Takahashi, J., Tamura, K., Suda, T., Matsumura, R., and Onda, Y.: Vertical distribution and temporal changes of 137Cs in soil profiles under various land uses after the Fukushima Dai-ichi Nuclear Power Plant accident, J. Environ. Radioactiv., 139, 351–361, https://doi.org/10.1016/j.jenvrad.2014.07.004, 2015.
UNSCEAR: Sources and Effects of Ionizing Radiation, V1, United Nations Scientific Committee on the Effects of Atomic Radiation, United Nations, New York, https://doi.org/10.18356/49c437f9-en, 2000.
Villa, J. A. and Bernal, B.: Carbon sequestration in wetlands, from science to practice: An overview of the biogeochemical process, measurement methods, and policy framework, Ecol. Eng., 114, 115–128, https://doi.org/10.1016/j.ecoleng.2017.06.037, 2018.
Walling, D. E. and He, Q.: Using fallout lead-210 measurements to estimate soil erosion on cultivated land. Soil Sci.Soc. Am. J., 63, 1404–1412, https://doi.org/10.2136/sssaj1999.6351404x, 1999.
Walling, D. E. and Quine, T. A.: Use of 137Cs measurements to investigate soil erosion on arable fields in the UK: potential applications and limitations, J. Soil Sci., 42, 147–165, https://doi.org/10.1111/j.1365-2389.1991.tb00099.x, 1991.
Zaborska, A., Carroll, J., Papucci, C., and Pempkowiak, J.: Intercomparison of alpha and gamma spectrometry techniques used in 210Pb geochronology, J. Environ. Radioactiv., 93, 38–50, https://doi.org/10.1016/j.jenvrad.2006.11.007, 2007.
Zarrinabadi, E., Lobb, D. A., Enanga, E., Badiou, P., and Creed, I. F.: Agricultural activities lead to sediment infilling of wetlandscapes in the Canadian Prairies: Assessment of soil erosion and sedimentation fluxes, Geoderma, 436, 116525, https://doi.org/10.1016/j.geoderma.2023.116525, 2023.
Zhang, F., Wang, J., Baskaran, M., Zhong, Q., Wang, Y., Paatero, J., and Du, J.: A global dataset of atmospheric 7Be and 120Pb measurements: annual air concentration and depositional flux, Earth Syst. Sci. Data, 13, 2963–2994, https://doi.org/10.5194/essd-13-2963-2021, 2021.