the Creative Commons Attribution 4.0 License.
the Creative Commons Attribution 4.0 License.
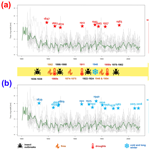
Assessing the impact of forest management and climate on a peatland under Scots pine monoculture using a multidisciplinary approach
Mariusz Lamentowicz
Piotr Kołaczek
Daria Wochal
Paweł Matulewski
Dominik Kopeć
Martyna Wietecha
Dominika Jaster
Katarzyna Marcisz
Assessing the scale, rate and consequences of climate change, manifested primarily by rising average air temperatures and altered precipitation regimes, is a critical challenge in contemporary scientific research. These changes are accompanied by various anomalies and extreme events that negatively impact ecosystems worldwide. Monoculture forests, including Scots pine (Pinus sylvestris L.) monocultures, are particularly vulnerable to these changes due to their homogeneous structure and simplified ecosystem linkages compared to mixed forests, making them more sensitive to extreme events such as insect outbreaks, droughts, fires and strong winds. In the context of global warming, forest fires are becoming extremely dangerous, and the risk of their occurrence increases as average temperatures rise. The situation becomes even more dramatic when fire enters areas of peatlands, as these ecosystems effectively withdraw carbon from the rapid carbon cycle and store it for up to thousands of years. Consequently, peatlands become emitters of carbon dioxide into the atmosphere.
In this study, we aim to trace the last 300 years of historical development of a peatland situated in a Scots pine monoculture. Our focus is on the Okoniny (Jezierzba) peatland located within Tuchola Forest in northern Poland, one of the country's largest forest complexes. We delved into the phase when the peatland's surroundings transitioned from a mixed forest to a pine monoculture and investigated the impact of changes in forest management on the peatland vegetation and hydrology. Our reconstructions are based on a multi-proxy approach using pollen, plant macrofossils, micro- and macro-charcoal, and testate amoebae. We combine the peatland palaeoecological record with the dendrochronology of Pinus sylvestris to compare the response of these two archives. Our results show that a change in forest management and progressive climate warming affected the development of the peatland. We note an increase in acidity over the analysed period and a decrease in the water table over the last few decades that led to the lake–peatland transition. These changes progressed along with the strongest agricultural activity in the area in the 19th century. However, the 20th century was a period of continuous decline in agriculture and an increase in the dominance of Scots pine in the landscape as the result of afforestation. Dendroclimatic data indicate a negative effect of temperature on Scots pine and pressure from summer rainfall deficiency. Additional remote sensing analysis, using hyperspectral, lidar and thermal airborne data, provided information about the current condition of the peatland vegetation. With the application of spectral indices and the analysis of land surface temperature, spatial variations in peatland drying have been identified. Considering the context of forest management and the protection of valuable ecosystems in monocultural forests, the conclusions are relevant for peatland and forest ecology, palaeoecology, and forestry.
- Article
(11018 KB) - Full-text XML
-
Supplement
(362 KB) - BibTeX
- EndNote
Peatlands, which play an important role in the global carbon cycle and whose destabilization can create positive feedback for climate warming, are vulnerable to various types of change (Gallego-Sala et al., 2018; Wilson et al., 2016). Peatlands, although they only cover about 3 % of the Earth's total land area (Parish et al., 2008; Rydin and Jeglum, 2013), store more than 30 % of the organic carbon (C) (Freeman et al., 2004; Gorham, 1991; Harenda et al., 2018), which is far more carbon than the entire biomass of the world's forests (Beaulne et al., 2021b). Their advantage over forests is due to not only their ability to accumulate C but also the fact that they do not emit decomposed carbon from the so-called rapid carbon cycle for up to thousands of years (Blodau, 2002; Gorham, 1991). Estimation of the C content accumulated in peatlands is challenging (Sanderson et al., 2023), although some studies indicate ca. 600 Gt C in the Northern Hemisphere alone (Yu et al., 2010). It has recently been shown that even the smallest kettle-hole peatlands effectively accumulate C and serve as important C hot spots (Karpińska-Kołaczek et al., 2024).
Insufficient awareness of the ecological importance of peatlands has led to them being treated as wastelands and drained for hundreds of years, to obtain land for agriculture and forestry or to exploit commercially as an energy resource (Joosten et al., 2012; Łuców et al., 2022; Paavilainen and Päivänen, 1995). Many of these areas have also had to adapt to a changing environment resulting from the use of various forest management techniques, e.g. the replacement of mixed forests with more easily managed monoculture forests (plantations; Lee et al., 2023; Łuców et al., 2021; Słowiński et al., 2019). Mixed forests, through greater biodiversity, are more resilient and better able to adapt to environmental change (Bauhus et al., 2017; Messier et al., 2022), providing a more comprehensive range of ecosystem services (Felton et al., 2016; Huuskonen et al., 2021).
Despite being more straightforward to manage, forest monocultures are characterized by simplified ecosystem linkages (Chapin et al., 2012). As a result, they are more susceptible to various extreme events and disturbances both natural and anthropogenic, including droughts, fires, strong winds and pest infestations (Grondin et al., 2014). This is particularly important as disturbances of these types of forests are becoming more common (Seidl et al., 2014; Westerling, 2016). Natural disturbance regimes in forests are mainly a response to climate change (Hanson and Weltzin, 2000; Pureswaran et al., 2015; Seidl et al., 2017; Trumbore et al., 2015); therefore, they are expected to increase in frequency and severity in the coming years (Gregow et al., 2017; Moritz et al., 2012; Wotton et al., 2010). Moreover, the problem applies to all kinds of monoculture forests regardless of the dominant species and climate zones (Booth, 2013; Guariguata et al., 2008; McNulty et al., 2013; Spiecker, 2000), including pine plantations in the temperate climate zone of Central and Eastern Europe (Łuców et al., 2021; Schüle et al., 2023). Thus, peatlands located in areas of forest monocultures are crucial in terms of their impact on global climate change and are even more vulnerable to extreme phenomena and disturbance, despite the already high climatic and anthropogenic pressure.
The history of peatlands' development can be traced using palaeoecological analyses, which allow numerous reconstructions of past environmental conditions including climate change (Lamentowicz et al., 2015a; Mauquoy and Yeloff, 2008). These include reconstructions of vegetation changes in the peatland and its surroundings, changes in the water table, and reconstructions of past fire activity (Gałka et al., 2022; Kołaczek et al., 2018; Marcisz et al., 2017, 2020b; Mroczkowska et al., 2021). Peat archive records contain a wide range of preserved micro- and macrofossils, for example, pollen, spores, microbial remains and charcoal are deposited in situ and brought in by wind or water (Godwin, 1981). While palaeoenvironmental reconstructions based on peat records have become common, few studies integrate palaeoecological data with other methods. For example, studies that combine palaeoecological and dendrochronological records, including dendroclimatic reconstructions based on analysis of the annual growth of tree rings, are still relatively rare (Ballesteros-Cánovas et al., 2022; Beaulne et al., 2021a; Dinella et al., 2021; Edvardsson et al., 2016, 2019, 2022; González de Andrés et al., 2022; Kuosmanen et al., 2020; Lamentowicz et al., 2009b). Yet combining peat records with dendrochronological data can benefit interpretations of trees, forest resilience and resistance to disturbances compared to local environmental changes recorded in peat. Such a view of past environmental changes through several proxies and other archive types is fundamental and will be helpful for forest management and nature conservation in the future. To assess the current state of the peatland, we also included remote sensing data in the analysis. Remote sensing methods have been applied to study wetland conditions for over 50 years and are currently regarded as some of the most useful methods in this research area (FAO, 2020; Guo et al., 2017). Remote sensing technologies enable the remote and non-invasive acquisition of information about the research object using specialized sensors, typically mounted aboard satellites or aircraft. In this study, data obtained from a multi-sensor aerial platform were used to assess the extent of peatland, to identify drainage ditches and to monitor the condition of the current vegetation.
Our study aims to assess the impact of forest management (the introduction of pine monoculture) and changing climate on the vegetation as well as on the hydrological and trophic conditions of a peatland in Central and Eastern Europe by integrating various data sources – palaeoecology, dendrochronology, remote sensing and historical information. We assumed that the introduction of pine monoculture led to changes in the species composition of peatlands in favour of Sphagnum mosses, as well as to the stabilization of the water table. We also undertook to confirm whether peatlands register and respond to extreme events, both in situ and in the immediate environment. We assumed that the disturbances that occurred in the monoculture forest would be recorded in the tree-ring (annual growth) record of Scots pine (Pinus sylvestris L.) and would confirm and complement the palaeoecological reconstruction of the peatland. Thus, we have identified peat layers corresponding to the occurrence of extremes gathered from historical sources and compared dendrochronological (dendroclimatic) data to them.
2.1 Study site
The Okoniny (Jezierzba) peatland (– N – E according to the WGS 84) is located in northern Poland, about 60 km north of Bydgoszcz and about 20 km northeast of Tuchola (Fig. 1). The study area is located within the Tuchola Forest mesoregion (Kondracki, 2001), close to the Pomeranian ice margin of the Vistulian glaciation dated to ca. 17 000–16 000 cal BP (Marks, 2012). The entire area of Tuchola Forest is a young glacial landscape covered by glacial till, sand, and numerous depressions and other forms originating from melting dead ice (Błaszkiewicz et al., 2015). Based on the analysis of remote sensing data, it was determined that the surface area of the peatland is 27.08 ha, with approximately 7.00 ha designated as non-forested area. The direct catchment area of the peatland covers a surface of 33.23 ha. The current elevation of the peatland is around 119 m a.s.l., with the highest-elevated area within the direct catchment reaching around 128 m a.s.l. It is part of a protected area (regulation no. 64/97, 1997) included within the boundaries of Tuchola Landscape Park (created in 1985). Moreover, since 2008 the entire complex of Tuchola Forest has been included on the Natura 2000 list as a special protection area. Since 2010, it has been listed as a UNESCO biosphere reserve.
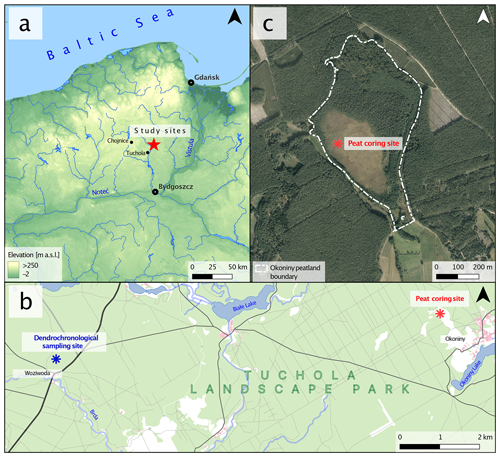
Figure 1Location of the study area. (a) Location on a map of northwestern Poland. (b) Location of the two study sites – dendrochronological sampling site and peat coring site. (c) Okoniny (Jezierzba) peatland sampling site with current peatland boundaries.
The Okoniny (Jezierzba) peatland is located in a temperate latitude zone, with a transitional climate influenced by continental air masses from eastern Europe and oceanic air masses from the Atlantic Ocean (Beck et al., 2018). According to climate data obtained from the Institute of Meteorology and Water Management from the meteorological station in Chojnice (35 km west of the study area) for the period between 1991 and 2020, the coldest month is January, with an average temperature of −1.5 °C, and the warmest month is July, with an average temperature of 18.0 °C. Between 1961 and 1990, both January and July were cooler by 1.6 °C compared to 1991–2020. The average annual temperature increased from 6.9 °C in 1951–1990 to 8.1 °C in 1991–2020. In terms of precipitation, February has the least amount, with an average of 31.1 mm for the period of 1991–2020, and July has the most, with an average of 80.7 mm for the period of 1991–2020. Compared to 1951–1990, the average precipitation for February increased by 7.7 mm, and for July it decreased by 4.1 mm. Mean annual rainfall increased from 558.1 mm for 1951–1990 to 612.4 mm for 1991–2020.
Samples for dendroclimatic analysis were taken from forest division no. 91 in Woziwoda Forest, Woziwoda forest district, about 9.5 km west of the study site (Fig. 1). The oldest pine trees in the forest district were selected for the study according to the indications of the forest survey and taxonomic descriptions.
2.2 Peat and tree core sampling
A peat core was taken from the northwestern part of the peatland in February 2022 using a Wardenaar corer (chamber dimension: ; Wardenaar, 1987). The entire length of the sampled peat core – 96 cm long monolith – was analysed. The core was sampled continuously every 1 cm, except for the top 10 cm, which contained a living Sphagnum layer. The first sample covered 4 cm of the surface layer (0–4 cm), and the following three samples were taken every 2 cm (4–6, 6–8, and 8–10 cm). We obtained 90 samples and analysed them for bulk density, ash content, peat and carbon accumulation rates, plant macrofossils, testate amoebae, macroscopic and microscopic charcoal, and pollen.
The research tree stem material was taken in April 2023 from 23 living and healthy trees at the Woziwoda site, ca. 9.5 km west of the Okoniny (Jezierzba) peatland. From each tree, a minimum of two cores was taken (from the eastern and western sides) at chest height (1.3 m) using a Pressler increment corer. In total, 50 cores were acquired from the Scots pine tree stems.
2.3 Radiocarbon dating and chronology
We used 10 samples containing Sphagnum stems and leaves for accelerator mass spectroscopy (AMS) 14C dating of the entire length of the profile. The survey was conducted at the Poznan Radiocarbon Laboratory in Poland (laboratory code marked Poz; Table 1). The IntCal20 (Reimer et al., 2020) and Bomb21NH1 (Hua et al., 2021) atmospheric curves were used to calibrate the dates.
Table 1The list of radiocarbon dates with calibration from Okoniny (Jezierzba) peatland in the OxCal v4.4.4 software using the IntCal20 calibration curve for the atmospheric data and Bomb21NH1 curve for bomb series.
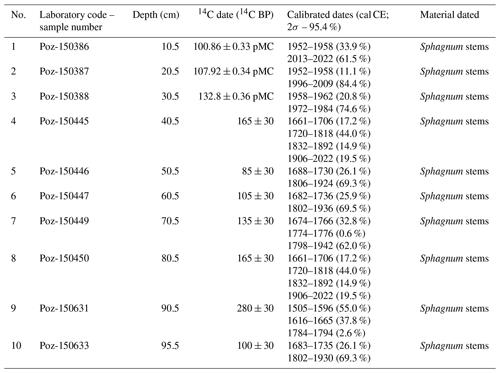
The absolute chronology of the entire core was based on a Bayesian age–depth model using OxCal v4.4.4 (OxCal v4.4.4, 2023). The P_Sequence
command with a parameter k of 0.1 cm−1 was used to calculate the model, assuming and an interpolation of 1 cm. The most pronounced change in peat composition, as manifested by changes in pollen concentration, testate amoeba species composition and species composition of plant macrofossils (which may signal changes in peat accumulation rates), was input using the Boundary
command at a depth of 66 cm. For better readability of the age–depth model, mean values (μ) were introduced and used to illustrate the modelled age.
2.4 Peat properties and carbon accumulation rate
Analyses of bulk density (BD), loss on ignition and peat carbon accumulation rate (PCAR) were carried out for each of the 90 samples. Each sample's volume [cm3] was carefully measured beforehand using calipers to avoid compressing the material. Each sample was then placed in a separate crucible and dried to determine the percentage of water content. The weighed and dried samples were incinerated at 550 °C for 12 h and reweighed according to the protocol of Heiri et al. (2001) to determine the ash mass [g]. Bulk density [g cm−3] was obtained by dividing the dry sample mass by the volume of the fresh sample according to Chambers et al. (2010). Loss on ignition [g] was obtained by subtracting the ash mass from the dry sample mass. Accumulation rates obtained from the peat core chronologies were multiplied by the bulk density without ash and by 50 % to obtain the PCAR, following the protocol of Loisel et al. (2014). The top 11 cm of the core (0–11 cm) was discarded for PCAR assessment due to the unrepresentative nature of the results obtained, as increased values of carbon accumulation in near-surface peat cannot be used for inference (Young et al., 2019).
2.5 Plant macrofossil analysis
The analysis of plant macrofossils was carried out using the modified protocol of Mauquoy et al. (2010). Each sample of approximately 5 cm3 was wet sieved (mesh diameter: 200 µm). The generalized content of the sample was estimated in percentage using a binocular microscope. Fruits, seeds, caryopses, achenes, perigynia, bud scales, catkin scales, whole preserved leaves, whole preserved needles, cones, anthers, sporangia, opercula, fungi sclerotia and wood pieces were counted as total numbers in each sample. The tissues of monocotyledon species and moss leaves (brown and Sphagnum mosses) were identified on slides using magnifications of ×200 and ×400. The material was compared with the guides (Anderberg, 1994; Berggren, 1969; Bojňanský and Fargašová, 2007; Mauquoy and van Geel, 2007). The diagram for the analysed proxy was plotted using the riojaPlot package for R (Rioja, 2023).
2.6 Testate amoebae analysis
Samples for testate amoeba analysis (volume of ca. 5 cm3) were washed under 300 µm sieves following the method described by Booth et al. (2010). Testate amoebae were analysed under a light microscope with ×200 and ×400 magnifications until the sum of 100 tests per sample was reached (Payne and Mitchell, 2009). Several keys and taxonomic monographs (Clarke, 2003; Mazei and Tsyganov, 2006; Meisterfeld, 2001; Ogden and Hedley, 1980) as well as online resources (Microworld, world of amoeboid organisms, 2023, last access: 26 April 2024) were used to achieve the highest-possible taxonomic resolution. The results of the testate amoebae analysis were used for the quantitative depth-to-water table (DWT) and pH reconstructions. Both the full diagram and the reconstructions were performed in the C2 software (Juggins, 2007) using the European training set (Amesbury et al., 2016).
2.7 Pollen and non-pollen palynomorphs
Samples for palynological analysis (volume of 2 cm3) were prepared using standard laboratory procedures (Berglund and Ralska-Jasiewiczowa, 1986). To remove the carbonates, samples were treated with 10 % hydrochloric acid. This step was followed by digestion in hot 10 % potassium hydroxide (to remove humic compounds) and soaking in 40 % hydrofluoric acid for 24 h (to remove the mineral fraction). Next, acetolysis was carried out. Three Lycopodium tablets (batch 280521291, containing 18 407 spores per tablet, produced by Lund University) were added to each sample during the laboratory procedures for the calculation of microfossil concentration (Stockmarr, 1971). Pollen, spores and selected non-pollen palynomorphs (NPPs) were counted under an upright microscope (Zeiss Axio SCOPE A1) until the number of total pollen sum (TPS) grains in each sample reached at least 500, apart from 23 samples in which pollen concentrations were very low. Sporomorphs were identified with the assistance of atlases, keys (Beug, 2004; Moore et al., 1991), various publications and the image database in the case of NPPs, for which there are no atlases (Miola, 2012; Shumilovskikh et al., 2022; Shumilovskikh and van Geel, 2020). The results of the palynological analysis were expressed as percentages; calculations are based on the ratio of an individual taxon to the TPS, i.e. the sum of AP (arboreal pollen) and NAP (non-arboreal pollen) excluding aquatic and wetland plants (together with Cyperaceae and Ericaceae), cryptogams, and fungi. The pollen diagram for the analysed proxy was plotted using Tilia graphing software (Grimm, 1991, 1992).
2.8 Macro- and micro-charcoal analysis
Microscopic charcoal particles (size of >10 µm) were counted from the same slides as pollen until the number of charcoal particles and Lycopodium spores, counted together, exceeded 200 (Finsinger and Tinner, 2005; Tinner and Hu, 2003). Microscopic charcoal influx or accumulation rates (MIC) were calculated by multiplying charcoal concentrations by peat accumulation rates (PAR; Davis and Deevey, 1964; Tinner and Hu, 2003).
For macroscopic charcoal analysis, samples (volume of 2 cm3) were prepared by bleaching to create a more visible contrast between the charcoal and the remaining organic matter following the method described by Whitlock and Larsen (2001). Samples were sieved through a 500 µm mesh and only large charcoal fragments >600 µm were analysed to obtain a local fire signal (Adolf et al., 2018). Samples were analysed with a binocular microscope using 60× magnification. Macroscopic charcoal influx or accumulation rates (MAC; ) were calculated using the charcoal concentrations and PAR.
2.9 Tree core chronology construction
Tree cores underwent a standardized dendrochronological procedure (Zielski and Krąpiec, 2004). Polished cores were scanned between 1200 and 2400 DPI using an Epson Perfection V700 photo scanner. Annual growth rings were measured from digital images with an accuracy of 0.01 mm using CooRecorder. This facilitated the selection of individual growth sequences, which were utilized to form a chronology for each plot. Visual comparisons were made between individual sequences, and the significance of correlations was assessed using Student's t test (Baillie and Pilcher, 1973). Subsequently, cross-dating was conducted using the COFECHA software (Grissino-Mayer, 2001), which evaluates each data series according to the reference chronology created and compares the correlation coefficients obtained. Raw chronologies were derived by employing an arithmetic mean. For climate–growth analysis, standardized chronologies were used, obtained by fitting a spline function (i.e. the n-year spline was set to of the wavelength of n years of single growth series) using the dplR
package (Bunn, 2008), package version 1.7.6 (2023), in the software R, version 4.3.0 (R, 2023). Using this standardization method, random variation in the radial growth was removed (Cook et al., 1990). For the chronologies obtained, i.e. raw (TRW) and standardized (RWI), values for the following descriptive statistics were computed: the mean correlation between series (inter-series correlation or Rbar), the GLK index (Gleichläufigkeit; Eckstein and Bauch, 1969) and EPS (express population signal; McCarroll and Loader, 2004).
2.10 Dendroclimatological and pointer year analysis
The chron
function from the dplR
package allowed for the making of a residual chronology, which was used for climate–growth analysis. The dcc
function and its moving response (25-year window) function method were used to determine the effects of climate conditions on the growth of Scots pine using the treeclim
package (Zang and Biondi, 2015) version 2.0.6.0 in R (R, 2023). This package allows the use of the bootstrap procedure to test the significance and stability of the coefficients of determination (r2) over a set period (Guiot, 1991). Monthly mean air temperature (Temp) and total monthly precipitation (Prec) were used to analyse climate–growth for the period of 1920–2022 (Klein Tank et al., 2002). Climate data were acquired via Climate Explorer (Trouet and van Oldenborgh, 2013) and calculated from the monthly gridded observational dataset E OBS v. 25.0e (Haylock et al., 2008) obtained for the 53.50–53.75° N, 17.75–18.00° E grid.
The Becker algorithm (Becker et al., 1994) was used to determine the pointer years in the Woziwoda chronology. Calculations were made using the “dplR” package in R and the “pointer” function (Bunn, 2008). Pointer years were calculated using adjustable thresholds of relative variation in radial growth set to a 10-year time window and the number of series exhibiting a similar incremental growth pattern. The main criterion for determining pointer years was the occurrence of unidirectional changes (i.e. a decrease or increase in the number of annual rings) in a minimum of 85 % of the tested sequences of annual increments observed in a group of trees at the Woziwoda site.
2.11 Acquisition and post-processing of remote sensing data
The analysis of the current state of Okoniny (Jezierzba) peatland was conducted using airborne remote sensing data. The data were acquired from a multi-sensor aerial platform by the MGGP Aero company on 25 March 2022 (leaf-off collection) and 20 July 2022, one of the warmest days of the year, which was particularly important for acquiring thermal data (leaf-on collection). Multispectral images (acquired with the IXM-100 camera) and airborne laser scanning data (ALS; acquired with the Riegl VQ780-II scanner) were obtained in the leaf-off season. Subsequently, during the vegetation season, the dataset was enhanced by acquiring hyperspectral data (collected using the HySpex VS-725 scanner) and thermal data (obtained with the InfraTEC 9400 camera). Based on the multispectral images, an orthophotomap was generated with a ground sampling distance (GSD) of 10 cm. Hyperspectral data were used to create a mosaic consisting of 430 bands (in the range from 400 to 2500 nm), ALS data were applied for the development of a digital terrain model (DTM) and thermal data were used to produce a land surface temperature (LST) mosaic. Thermal and hyperspectral mosaics and DTM were prepared with GSD=1 m.
Photo interpretation was carried out to assess the extent of peatlands and the course of drainage ditches using orthophotos and the DTM as a base map. The DTM was also used to delineate the catchment area of the peatland. Hydrological modelling methods based on watershed analyses were employed for this purpose. A hyperspectral mosaic was used to calculate spectral indices such as the Normalized Difference Vegetation Index (NDVI; Rouse et al., 1974) and Moisture Stress Index (MSI; Hunt and Rock, 1989). Spectral indices are mathematical formulae that enable the simultaneous analysis of reflectance across multiple spectral ranges. The NDVI is a measure of healthy, green vegetation ranging from −1 to 1. Vegetation values typically range from 0.2 to 0.8, with higher values indicating healthier and denser vegetation. The MSI is sensitive to increasing leaf water content. Its values range from 0 to more than 3, but the common values for vegetation are from 0.4 to 2. Higher values indicate greater water stress and less water content in this case. Thermal data were used to calculate land surface temperature (LST), measured in °C.
2.12 Historical and cartographic information
Several historical cartographic studies were used to assess changes to the peatland and its surroundings. The oldest of the materials used is the Schrötter–Engelhardt map of 1803. Work on creating the map began in 1796 under the leadership of the Prussian government minister Friedrich Leopold von Schrötter (1743–1815) and topographer Friedrich Bernhard Engelhardt (1768–1854). The first version was produced at a scale of 1:50 000. Still, due to the concerns of the Prussian army command about the map being too detailed and capable of being used by enemy armies, a generalized version was eventually published at a scale of 1:150 000. A larger-scale version of the map was not available until the 1920s (Jäger, 1981, 1982). In this article, the generalized version of the map is used.
The Prussian topographic map Messtischblatt of 1874 (on a scale of 1:25 000, sheet no. 982, Zalesie section) was also analysed, along a detailed map of Poland issued by the Military Geographical Institute in 1933 at a scale of 1:25 000 (PAN map sheet 34 – SLUP 26 – B (Linsk)). In addition, a geological–agricultural map compiled between 1899 and 1900 using the topographic Messtischblatt of 1874 was considered. The Prussian Geological Survey produced the map (Königlich-Preußische Geologische Landesanstalt), which provides information on alluvial and diluvial deposits covering the area under study. The maps show the changes in the peatland and its surroundings from the early 19th century to the 1930s. Aerial images from 1964, 1984 and 1997 obtained from the Head Office of Geodesy and Cartography were also used for the same purpose (license no. DIO.7211.457.2023_PL_N).
Insect outbreak data are based on the literature (Orłowicz, 1924; Schütte, 1893; Wilson, 2012).
3.1 Age–depth model and peat accumulation rate
The age–depth model showed an agreement index (Amodel) of 60 % (Fig. 2), precisely at the limit of the recommended minimum for its reliability (60 % according to Bronk Ramsey, 2008). The model spanned a period of ca. 282 years, with a maximum uncertainty of ca. 30 years (mostly in the time period of ca. 1883–1783 cal CE). Most of the core consisted of well-preserved Sphagnum peat, while the lower part consisted of sedge peat. The peat accumulation rate averaged 3.6 mm yr−1, with the highest values associated with the un-decomposed acrotelm zone. The upper layers located between 0 and 11 cm were excluded from the analysis of peat accumulation rates. The fastest rate was 0.71 cm yr−1 (at 11.5 cm), and the slowest was 0.1 cm yr−1 (at 91.5 cm). The mean BD value across the core was 0.07 g cm−3. It was highest in the lower part of the core, with 0.10 g cm−3 between 96 and 70 cm, and lowest in the middle part – 0.05 g cm−3 between 69 and 30 cm. In the upper part between 29 and 0 cm, it was 0.06 g cm−3. Similarly, this upper, un-decomposed layer was excluded from the peat carbon accumulation rate (PCAR) analysis. For the rest of the core (11–96 cm), PCAR averaged 112 . The mean water content of the wet sample was 93.8 %, and the mean organic matter content of the dry sample was 95.5 %.
3.2 Palaeoecological analyses
3.2.1 Phase 1 (∼1726–1838, 96–74 cm): wet conditions and low human impact
The plant macrofossil and pollen analyses point to the presence of a shallow water body during this time interval. Plant macrofossil analysis (Fig. 3) showed that the peatland vegetation in this phase was strongly dominated by vascular vegetation, mainly Carex spp. monocotyledons. Shallow water and edges of the water body were overgrown by sedge communities (Cyperaceae pollen, 2.8 %–14.5 %; Fig. 5). Additionally, this was indicated by the presence of macrophytes represented by pollen of Potamogeton subgen. Eupotamogeton (0 %–0.9 %), Nymphaea (0 %–0.4 %) and Utricularia (0 %–0.3 %) (Fig. 5). The high percentage of aquatic non-pollen palynomorphs (NPPs) such as cyanobacteria and the algae Tetraëdron minimum, Scenedesmus, Botryoccocus and Pediastrum (Fig. 5) confirms the results of plant macrofossil and pollen analyses.
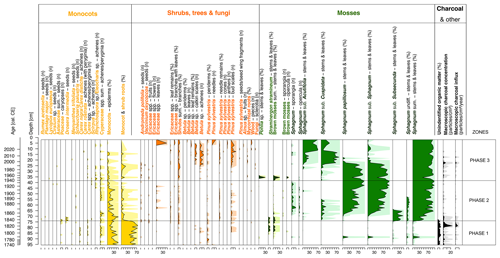
Figure 3Diagram showing macrofossil percentages, macroscopic charcoal concentrations and influx as a local fire proxy. An exaggeration of 10 times is marked using lighter-coloured shading behind the main curves in the lower part of the figure.
This phase was also characterized by the presence of the brown moss Straminergon stramineum (max 9 % of the subsample content; Fig. 3). This species occurs in a wide range of habitats (Hedenäs, 1993) but is most common in wet, moderately acidic habitats (Blockeel, 2010). Straminergon stramineum is usually found as scattered stems or small patches among other mosses but occasionally forms scattered mats – sometimes partially submerged in water, next to lakes, on the edges of peatland or in lakeside marshes (Hill and Blockeel, 2014).
This phase of peatland development was characterized by a very low concentration of testate amoebae in the samples. Centropyxis aculeata was the most abundant species (Fig. 4). The dominance of plagiostomic species from the genus Centropyxis may point to the presence of mineral input into the peatland (Lamentowicz et al., 2009a; Marcisz et al., 2020a). The water level in the peatland was quite unstable and fluctuated between 4.3 and 16.5 cm below the ground; and the pH value ranged between 4.5 and 5.2, but due to the low number of identified tests, these reconstructions should be viewed with caution (Fig. 4).
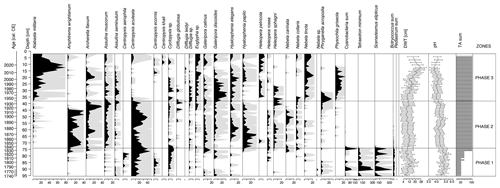
Figure 4Testate amoebae and selected aquatic non-pollen palynomorphs (Cyanobacteria, Tetraedron minimum, Scenedesmus, Botryococcus and Pediastrum). Percentages are shown in black, and the 10 times exaggeration is marked using light-grey shading behind the main curves. The testate amoeba-based depth-to-water table (DWT) and pH reconstructions as well as the sum of testate amoeba shells counted in each sample (TA sum) are presented.
The surrounding vegetation was characterized by the dominance of forests, as evidenced by the high proportion of arboreal pollen (AP; 83.6 %–91.1 %) in the total pollen (TP) content (Fig. 5). The main species recorded were Pinus sylvestris (62.6 %–81.3 % AP) and Betula (6.8 %–16.0 % AP), with admixtures of Alnus (2.5 %–7.7 % AP), Quercus (1.8 %–8.1 % AP), Corylus avellana (0.6 %–3.8 % AP), Carpinus betulus (0 %–3.4 % AP) and Fagus sylvatica (0.4 %–3.3 % AP). Cerealia pollen (0 %–7.8 % TP) along with Centaurea cyanus, a crop weed, indicated a stable presence of cultivated fields.
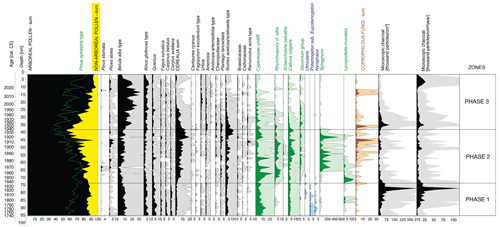
Figure 5Pollen diagram with selected taxa presented (list of taxa presented in the associated open dataset). Pollen percentages are shown in black, and the 10 times exaggeration is marked using lighter-coloured shading behind the main curves in the lower part of the figure. Microscopic charcoal concentrations and influx as a proxy for non-local fires are also presented.
This phase also had the highest influx of macroscopic charcoal (MAC) of all three of the phases distinguished (Fig. 3). Towards the end of the phase at depths of 79.5 and 78.5 cm (first half of the 1820s according to the calibrated dates), the influx reached the highest values throughout the core and equaled 24.5 and 11.5 , respectively. The highest influx of MAC in both subsamples corresponded with the influx of microscopic charcoal (MIC), reaching over 53 200 for the 79.5 cm subsample and over 125 000 for the 78.5 cm subsample (Fig. 5). This distinct fire event was followed by a slight decrease in pH, an appearance of wet-indicator mixotrophic testate amoeba species (Amphitrema wrightianum, Archerella flavum, Hyalosphenia papilio), and the disappearance of cyanobacteria and algae (Fig. 4).
3.2.2 Phase 2 (∼1838–1945, 74–37 cm): stabilization of the water table and increase in acidity, a transition from mixed forest to pine monoculture and agricultural development
The local vegetation (Fig. 3) in this phase was dominated by Sphagnum, first by the subgenus Subsecunda, then for most of this period by Sphagnum papillosum. S. papillosum occupies the more oligotrophic lawns with a preference for open spaces (Clymo and Hayward, 1982; Laine et al., 2018). Along with the appearance of Sphagnum from the subgenus Subsecunda, Drosera intermedia was also recorded. Currently, in Poland, it is a very rare species, found in dispersed peatlands (Mirek et al., 2006). Individuals often stand in the water throughout the season. Andromeda polifolia also appeared in this phase. Initially, the presence of Sphagnum was accompanied by Straminergon stramineum (max 10 %), but later it disappeared completely. By the beginning of the 20th century, a relatively high proportion of monocotyledonous plants was also observed, represented in the samples by their epidermis, averaging about 20 % in a sample, with a much higher proportion in the early stages. All these taxa indicate an intermediate environment between a shallow lake and a moss peatland.
After an initial decline (from 9.2 cm at 73.5 cm, 1838 cal CE, to 13.0 cm at 66.5 cm, 1862 cal CE), the water table level increased and stabilized at a high level, reaching a maximum of 6.8 cm at 47.5 cm, 1907 cal CE (Fig. 4). The abundance of individual testate amoeba species also increased. Initially, C. aculeata dominated, but later Amphitrema wrightianum and Hyalosphenia papilio, mixotrophic taxa that contain endosymbiotic photosynthetic algae, begin to prevail (Lamentowicz and Mitchell, 2005a; Marcisz et al., 2020a; Fig. 4). Subsequently, the proportion of A. wrightianum and H. papilio begun to decline in favour of Archerella flavum and Hyalosphenia elegans (Fig. 4). All four species are associated with the presence of Sphagnum, with A. flavum and A. wrightianum tolerating very wet or even submerged Sphagnum habitats, which correspond to a stably high water table. Then, from the mid-1880s for another ca. 20 years, C. aculeata again became dominant. After this period, species associated with Sphagnum – A. wrightianum, A. flavum and Heleopera sphagni – began to dominate again. During this phase, further acidification of the site was noted through a drop in the pH value from the initial 4.8 to 4.1 (Fig. 4).
The forests surrounding the peatland (55.1 %–92.7 % TP) were still dominated by pine (64.5 %–92.8 % AP), although the percentage has decreased in comparison to phase 1, especially during the 1920s and 1930s (Fig. 5). Deciduous taxa such as Quercus, Corylus avellana, Carpinus betulus and Fagus sylvatica retreated. The percentage of Cerealia in the TP increased significantly, from 0 %–7.8 % TP in the first phase to 2.8 %–19.8 % in the second phase, with a peak in the late 1910s and early 1920s, indicating the development of agriculture in the vicinity of the peatland (Fig. 5). Around the same time, the proportion of Rumex also increased significantly (0 %–11.5 %). The low values of MAC (Fig. 3) and MIC (Fig. 5) indicated low fire activity in the area studied.
3.2.3 Phase 3 (∼1945–present, 37–0 cm): lowering of the groundwater table, further afforestation with Pinus sylvestris, a succession of Betula
The local vegetation (Fig. 3) underwent several changes during this phase. Although Sphagnum dominated for the entire time, the subgenus Sphagnum receded in favour of first the subgenus Cuspidata and then the subgenus Acutifolia. The beginning of the phase was marked by Pohlia nutans, which can win the competition in unstable habitat conditions such as during the dry season (Boulc'h et al., 2020). Its occurrence correlated with the presence of Phryganella acropodia among testate amoebae (Fig. 4), which is an indicator of low water levels in Sphagnum peatland (Diaconu et al., 2017; Lamentowicz and Mitchell, 2005b).
This was followed by Alabasta militaris (average of 25.5 %), Galeripora discoides (average of 10.5 %) and Nebela tincta (average of 8.2 %) beginning to dominate (Fig. 4). G. discoides is typically present in acidic sites with unstable hydrological conditions (Lamentowicz and Mitchell, 2005b; Sullivan and Booth, 2011). N. tincta tolerates dry, highly acidic conditions with a mineral matter supply (Booth, 2002; Koenig et al., 2018; Lamentowicz et al., 2011). A. militaris, dominant in recent years, is indicative of dry and markedly acidic conditions (Amesbury et al., 2016; Booth, 2002; Lamentowicz et al., 2011; Marcisz et al., 2020a; Sullivan and Booth, 2011). Based on testate amoebae, this phase was distinguished by a significant drop in the groundwater table, from an average level of 9.6 cm below the ground surface in the second phase to 15.7 cm. In the last decade, the most significant decline was observed, with an average level of 21.9 cm and with a maximum of 27.5 cm, 1983 cal CE. The pH continued to decrease, from 4.4 to 4.0 (Fig. 4).
On a regional scale, there is an increase in the relative abundance of Pinus pollen in the TP, from about 46 % at the beginning of the phase to about 85 % today, as an effect of afforestation (Fig. 5). The Betula pollen percentage has an apparent increase, from 0.7 %–11.3 % in the second phase to 5.6 %–32.5 %. The increased percentage of Betula pollen, combined with macroscopic remains in the form of achenes and catkin scales, indicates the intensive succession of this species on the peatland surface. The ruderal species Urtica and Artemisia were also more strongly present. The average proportion of Urtica pollen in the TPS increased distinctly (from 0 %–0.7 % to 0 %–2.9 %). The percentage of Cerealia in TP has decreased significantly, from nearly 20 % in the early 1920s to just over 1 % today.
Local (Fig. 3) and regional (Fig. 5) fire activity continued to be low, although two slightly more intensive periods of regional fires were marked – ca. 1945–1963 and in the early 2020s.
3.3 Dendrochronological and pointer year analysis
A total of 50 tree-ring series of 23 Pinus sylvestris L. trees from the Woziwoda site were successfully cross-dated. Based on the TRW (Fig. 6) and RWI, a well-synchronized tree-ring series spanning 222 years (1801–2022) was developed. The statistical characteristics of the ring-width series and the statistical parameters indicating the signal strength of the regional RWI chronology are shown in Table 2. The mean EPS was 0.93, which is well above the threshold value (EPS=0.85) required to produce a statistically robust RWI chronology. Mean series inter-correlation, MS, SNR and other statistical parameters indicating the strength of chronology signals were also high, indicating the suitability of chronology for climate–growth analysis.
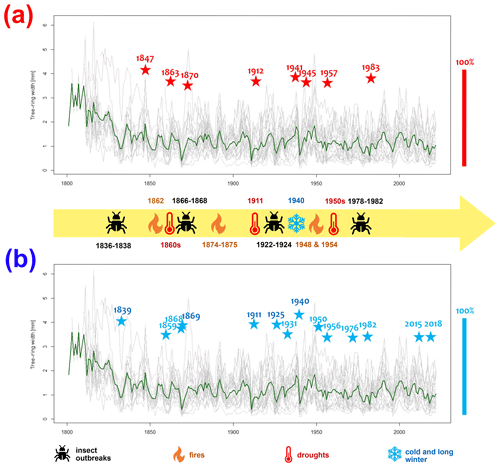
Figure 6The grey lines depict the individual tree-ring series of each tree, while the green line represents the average raw chronology of Pinus sylvestris L. at the Woziwoda site. Identified within the Scots pine chronology from Woziwoda are pointer years, categorized as negative (NEG) (a) and positive (POS) (b). These pointer years are highlighted with coloured asterisks: red for positive pointer years and blue for negative pointer years. The position of the asterisks refers to a scale of 0 %–100 %. Information on extreme phenomena is based on Orłowicz (1924), Schütte (1893), Broda (2000) and Wilson (2012).
Table 2Descriptive statistics of standardized Pinus sylvestris L. (RWI) chronology for the Woziwoda site.
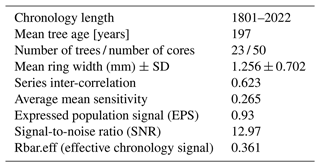
Across the study period (1920–2022) a significant positive relationship between growth and February mean temperature was identified (Fig. 7). The moving correlation analysis showed an increasing trend in the sensitivity of tree growth to climatic factors (Fig. 8). The positive response of tree growth to February mean temperature remained constant throughout the study period (1920–2022; Fig. 8). However, the sensitivity of tree growth to summer temperature increased. The relationship between annual growth and summer temperature was not stable during the period of 1920–2022. Nevertheless, in the last 30 years, a significant negative relationship between annual growth and June mean temperature was observed.
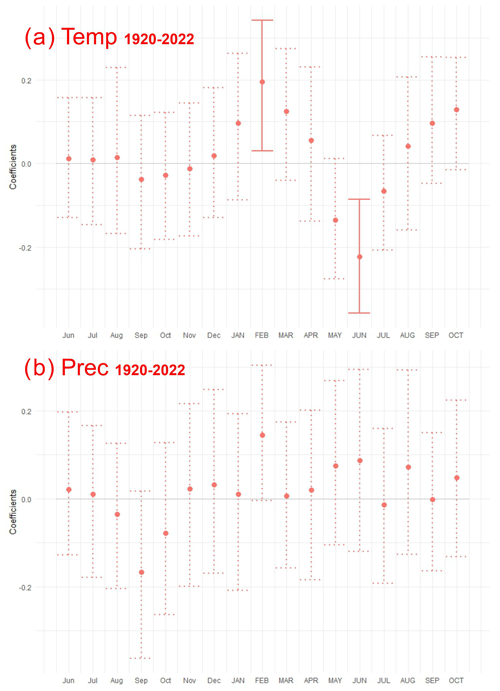
Figure 7Response function coefficients between residual Pinus sylvestris L. chronology and climate variables: (a) mean air temperature (Temp) and (b) precipitation (Prec) for the period of 1920–2022. Months in upper case are the current year, whereas the standard abbreviations indicate months from the previous year. Solid lines represent significant coefficients at p<0.05.
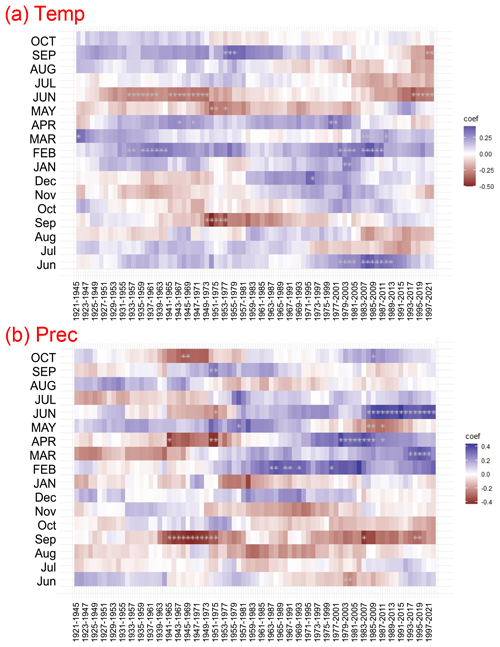
Figure 8Moving response correlations (25-year window) between residual Pinus sylvestris L. chronology and climate variables: (a) mean air temperature (Temp) and (b) precipitation (Prec) for the period of 1920–2022. The colour code represents the response function coefficients. Significant correlations are indicated by white asterisks.
Climate–growth analysis for monthly data did not show a statistically significant relationship between growth and precipitation (Fig. 7). However, the moving response analysis revealed significant short-term relationships between tree growth and precipitation. Furthermore, we demonstrated that the influence of precipitation in the months of the current year on tree growth, calculated for the years 1960–2022, was more significant than the relationships calculated for the years 1921–1959. In recent years, a particularly positive relationship between tree growth and early-year (February–April) precipitation, as well as June precipitation, has become apparent.
For the Woziwoda site, 8 positive and 13 negative pointer years were identified for the period of 1814–2022 (with a minimum sample depth of 10 trees; Fig. 6). The most-pronounced positive pointer years with more than 90 % tree response were as follows: 1847, 1863, 1912, 1941, 1945, 1957 and 1983. The most-pronounced negative pointer years were 1839, 1868, 1869, 1911, 1925, 1940 and 1950. Figure 6 provides indicators of pointer years together with meteorological and ecological characteristics.
3.4 The current state of the peatland based on remote sensing data analysis
Presently, the non-forested part of the peatland is drained by two parallel ditches. One is located in the northern area, and the other is in the southern, non-forested part of the peatland. The analysis of thermal data obtained on a midsummer day indicates that the average LST for the non-forested part of the peatland is approximately 34.29 °C, with a temperature range extending from 19.22 to 46.37 °C. There is a distinct internal variability in LST values within the studied area. Higher values, indicative of more significant dehydration, were identified in the eastern part of the peatland, while lower values were observed in the western part. A repeating spatial pattern of values was observed in the analysis of vegetation indices (NDVI and MSI). High NDVI values and low MSI values, indicative of good vegetation condition and low water stress, were observed in the western and southwestern parts of the peatland (Fig. 9). The average NDVI value in these areas is 0.71, and MSI is 0.6. Conversely, low NDVI values and high MSI values, indicative of significant dehydration of the peatland and low vegetation vigour, were observed in the eastern part of the area (Fig. 9), where NDVI averages 0.63, and MSI is around 0.69. The overall average NDVI for the area was 0.65, and for MSI, it was 0.68.
3.5 Historical maps and airborne images as confirmation of changes shown in palaeoecological data
Analysis of historical materials (Fig. 10), including maps and airborne images, confirms the results of the palaeoecological analysis. Both the Schrötter–Engelhardt map of 1802 and the Messtischblatt of 1874 indicate the existence of a small lake in the coring area. Again, however, it should be noted that the Schrötter–Engelhardt map is a highly generalized study and does not give much information about the surroundings of today's peatland, other than that we are dealing with an area with the character of a dense forest complex with wetlands in isolated places. The Messtischblatt allows us to better interpret the surroundings of the modern peatland at the time in which the map was prepared. A small lake named Kolze See is observed in an advanced stage of development, i.e. progressive overgrowth. This lake is surrounded by wetlands (Bruch in German), somewhat distant heathland (Heide in German) and wasteland (Ödland in German) (the original nomenclature of the map legend was adopted). This lake and two other lakes close by are enclosed within a single catchment area. To the south, the area of the current peatland was adjacent to an open, extensive meadow.
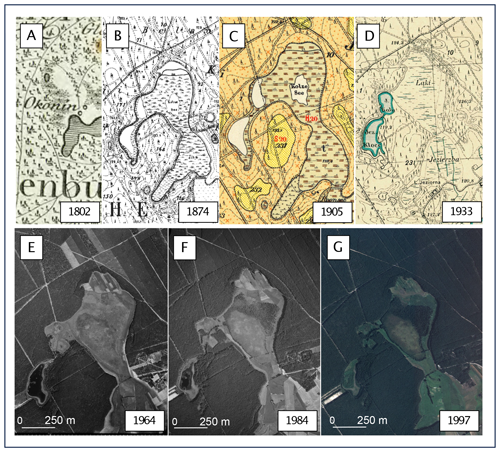
Figure 10Changes in the peatland and its surroundings since the beginning of the 19th century based on historical maps and aerial images. (a) Schrötter–Engelhardt map, 1:150 000 (1802); (b) Messtischblatt map no. 982, 1:25 000 (1874); (c) Prussian geological and agricultural map no. 2374, 1:25 000 (1905); (d) detailed map of Poland, 1:25 000 (1933); (e) aerial photograph from 1964; (f) aerial photograph from 1984; and (g) aerial photograph from 1997. The maps in (a), (b), (c) and (d) are in the public domain. Aerial photographs are © Head Office of Geodesy and Cartography in Poland, license no. DIO.7211.457.2023_PL_N.
Even more information is provided by a 1905 geological–agricultural map prepared on the topographic base of the Messtischblatt map of 1874. In addition to land use, it shows the type and thickness of alluvial and diluvial deposits. According to this map, the area around the lake was covered by alluvial sediments – humus with peat subsoil and shallow groundwater (German original – Humus (Peat) mit Torf-Untergrund und nahem Grundwasser). The thickness of the peat was marked at 2 m. However, it should be noted that drilling surveys at that time only covered a maximum depth of 2 m, so the maps do not provide information on the total thickness of the sediments (Jasnowski, 1962). Places that were used as heathland and wasteland on the topographic map are covered by sandy humus on a sandy substrate with shallow groundwater (German original – sandiger Humus mit Sand-Untergrund und nahem Grundwasser) and by humic sands on a substrate of permeable sands with shallow groundwater (German original – humoser Sand mit durchlässigen Sand-Untergrund und nahem Grundwasser).
A detailed map of Poland from 1933 documents the change in the ecosystem from lake to land. The area, which on Prussian topographic maps was a lake with a surrounding bog, is described as a meadow on this map. Moreover, the adjacent meadows to the south were marked with drainage ditches, which were not marked on the Prussian maps. The area's surroundings, as before, were dominated by coniferous forests.
Aerial photos document subsequent changes in the ecosystem. The 1964 photo shows the northern part of the peatland's agricultural use today (regular surface layout). Lake Kały, located nearby, became completely overgrown, and its area was later drained by several ditches brought to the peatland. The surrounding area of the peatland is dominated by dense forest with occasional open clear-cutting areas. A photo from 1984 documents the succession of trees in the north–central part of the peatland. In the surrounding area, open forest areas have entirely disappeared. A photo from 1997 clearly shows the development of trees on the peatland, which have formed a dense block in its north–central part. A distinct area of Sphagnum-dominated peatland with a well-marked edge has also emerged. Currently, the northernmost part of the peatland is overgrown by pine; it is almost impossible to identify the maximum extent of the peatland surface in the field (Fig. 1).
4.1 Exceptionally high peat accumulation rate
In the Okoniny (Jezierzba) peatland, a rapid rate of peat accumulation is observed, averaging 3.56 mm yr−1, with a maximum value of 7.1 mm yr−1 at a depth between 11 and 12 cm. This accumulation rate is not commonly observed. There are only a few peatlands in Poland for which higher accumulation rates were reported. In Tuchola Forest, these were Dury – 10 mm yr−1 (Pawlyta and Lamentowicz, 2010); Mukrza – 4.6 mm yr−1 (Lamentowicz and Obremska, 2010); Jelenia Wyspa mire, where the accumulation rates reached 0.4 mm yr−1 for the first 3000 years but accelerated to 3 mm yr−1 in the last 150 years (Lamentowicz et al., 2007); and the Tuchola kettle-hole bog – 1.2 mm yr−1, and after ca. 1320 cal BP the accumulation rate dropped to 0.4 mm yr−1 (Lamentowicz et al., 2008a). In other pine monocultures, such as Noteć Forest, the Rzecin peatland stands out for its high accumulation rate – an average of 6.8 mm yr−1 in one profile and 7.5 mm yr−1 in the other one (Milecka et al., 2017). Peatlands in Tuchola Forest, including Okoniny (Jezierzba) peatland, generally have a faster accumulation rate than peatlands located in other parts of Pomerania, especially small kettle-hole peatlands that accumulate carbon the fastest of all peatland types (Karpińska-Kołaczek et al., 2024). In Pomeranian peatlands, the highest accumulation rates were reported for the period between ca. 150 and 1230 cal. CE and reached 2.2 mm yr−1 in Stążki (Lamentowicz et al., 2008b) and 1.38 mm between 1830 and 2006, although the highest accumulation rate was 5 mm yr−1 (during 840–860 cal. CE) in the Słowińskie Błota raised bog (Lamentowicz et al., 2009b). At the Gołębiewo sites, the maximum accumulation rates were 1.85 and 0.36 mm yr−1 (Pędziszewska and Latałowa, 2016). For many Sphagnum-dominated peatlands in other parts of Poland, the average PAR varied between 1.4 and 2.5 mm yr−1 (Gałka et al., 2015; Lamentowicz et al., 2015a, 2020; Marcisz et al., 2020b). Such high accumulation rate values are also rare in other parts of the temperate climate zone of Europe. Teiči bog (Latvia) showed similar accumulation rates – 3.5 mm yr−1 – from 1835 to 1965 cal. CE and 10 mm yr−1 after 2000 (Stivrins et al., 2018). Okoniny (Jezierzba) peatland after 2000 (between 21.5 and 11.5 cm) recorded an accumulation of 5.7 mm yr−1. Saxnäs Mosse in Sweden showed an almost linear peat accumulation rate of 2–2.5 mm yr−1 (van der Linden et al., 2014). The maximum accumulation was recorded at around 2310–2250 cal BP in the Estonian Hara bog (31–15 cm), reaching 2.4 mm yr−1 (Łuców et al., 2022). A comparison with other regions of Poland and Europe shows that the exceptionally high accumulation rates at the analysed site are worth highlighting.
4.2 Relationships between forest management and pollen analysis
4.2.1 The complex history of Tuchola Forest and its influence on the forest
The results of the pollen analysis of the collected cores enabled us to illustrate how the forest was managed over the past 300 years. Due to political changes and several administrative decisions, the management strategies of Tuchola Forest underwent vital changes. The consequences of the implementation of forest management techniques were visible in the palaeoecological record.
With the first partition of Poland by Prussia in 1772, regulations for planned forest management began to be introduced. The main planting species was Scots pine, which over time began to dominate the forest, replacing deciduous admixture species (Broda, 1993). The region's forest cover and forest composition were also affected by later political and administrative developments. For more information on the history of forest management in the late 18th and early 19th centuries; see File 1 in the Supplement.
Our data confirm an increase in the proportion of pine pollen in the forest composition and a decrease in the proportion of pollen of other species. From the 1730s to the mid-1860s, the share of pine pollen in the pollen of all trees increased from about 60 % to about 90 %. Our pollen diagram shows the rapid increase in the Pinus sylvestris pollen percentage after 1850. It can, therefore, be assumed that this resulted from the Pinus sylvestris introduced by mass monoculture plantings in the early 1830s reaching reproductive capacity. Pine usually reaches sexual maturity between 10 and 15 years of age (Sullivan, 1993), although the threshold age has been set at 25 years (Matthias and Giesecke, 2014). The decline in the share of deciduous species and the increase in the share of Scots pine in the landscape began in Poland with the formation of the state. However, at that time, it was associated with the expansion of agriculture and the harvesting of preferred species such as Carpinus betulus (Czerwiński et al., 2021). Nevertheless, in the Prussian partition, planned forest management permanently changed the composition of Poland's largest forest complexes, which were dominated by easy-to-grow pine (Broda, 1993; see File 1 in the Supplement). A dynamic increase in the share of pine pollen until the 1860s in Tuchola Forest was also recorded at Czechowskie Lake (Słowiński et al., 2019). An increase in pine pollen percentage since the 19th century was also shown in pollen diagrams from other sites in Pomerania – Stążki (Lamentowicz et al., 2008b) and Słowińskie Błota (Lamentowicz et al., 2009b) – and in other monoculture plantation complexes from the Prussian partitioning area – i.e. the Rzecin peatland in Noteć Forest (Milecka et al., 2017).
Although attempts were undertaken to correct earlier mistakes, this did not stop the massive deforestation (among other consequences of war events and administrative regulations for settlement, more in File 1 in the Supplement). Until the 1870s, the feudal system was still mixed with capitalist components, but from the 1870s onward, under monopoly capitalism, timber trade and processing began to reach a significant level (Broda, 2000). However, it has been noted that forests regulate air temperature, store water in the soil more efficiently and reduce wind speed, preventing soil erosion, which can help local agriculture facing difficult environmental conditions (Wilson, 2012). For this reason, as early as the 1870s, the state administration encouraged landowners to protect forest stands on their lands and establish forestry cooperatives. The government also guaranteed funds for the reforestation of private and municipal lands. In the mid-1870s, the Landtag set aside a budget for the purchase and reforestation of wasteland by the state. However, these funds were only used to a small extent, although this somewhat reduced the share of forested private property (Broda, 2000; Wilson, 2012). In 1886, the Royal Settlement Commission (German original – Königliche Ansiedlungskommission) was established to buy up the estates of impoverished Polish nobility to acquire agricultural land for German settlers (Wilson, 2012).
At the end of the 19th century, Tuchola Forest became the largest timber production hub in the Prussian partition. The Bydgoszcz timber industry region also played a major role in wood processing. The first steam sawmill in the Bydgoszcz region was built in 1873, and by 1913, there were 20 of them, processing some 500 000 m3 of wood and employing more than 1600 people (Broda, 2000). All this resulted in a significant decline in the share of tree pollen in the total pollen share in our diagram, to less than 60 % by the late 1920s and early 1930s. At the same time, we have seen intensive agricultural development. At Okoniny (Jezierzba), the proportion of Cerealia pollen doubled between ca. 1900 and 1920. This trend is also confirmed by pollen data from the site in Okoniny Nadjeziorne on the other side of Okonińskie Lake (Tipton, 2023), as well as from Czechowskie Lake, about 25 km northeast of our site (Słowiński et al., 2019). Despite intensive deforestation in general, further afforestation with pine was also progressing. In 1893, pine forests accounted for 99 % of all forests in Tuchola county (Szwankowski, 2005). Intense changes in forest management (pine dominance) and agricultural development (high percentage of Cerealia pollen) in the 19th century are also evident in records of profiles outside large, dense forest complexes – i.e. Kusowskie Bagno (Gałka et al., 2014) and Linje mire (Marcisz et al., 2015).
4.2.2 Impact of forest management on peatland vegetation
As a result of changes related to forest management, the lake-to-peatland transition occurred rapidly. We assume that this was primarily the result of drainage, which was undertaken in the area at the end of the 19th century (see drainage ditches on the southern side and a dike in the middle part of the site on maps in Fig. 6), and secondly, to a lesser extent, the result of the transition from mixed forests to pine monoculture. These activities contributed to an increase in the acidity of the peatland. Forest drainage is often associated with the acidification of surface waters (Miller et al., 1990). The introduction of forest drainage in or near peatlands to improve tree growth has become quite common in northern and northeastern Europe (Westman and Laiho, 2003). The oxidation of organic sediments and the detachment of H+ ions increase acidity (Ulrich, 1980). In addition, the supply of alkaline cations to the peat is impeded by drainage ditches (Minkkinen et al., 2008). However, the long-term consequences of drainage are devastating to peatlands, as they initiate vegetation succession, in which species typical of peatlands are replaced by forest vegetation (Laine et al., 1995). In the example of our palaeoecological data, the dynamic succession of pine and birch in the Okoniny (Jezierzba) peatland is evident, which is also supported by aerial imaging. As already mentioned, the successive decline in pH is also the result of the impact of pine plantations growing in catchments. A drop in pH in Okoniny (Jezierzba) has likely enabled the rapid growth and expansion of Sphagnum and the peatland initiation. The crowns of forests, especially the needles, can increase the uptake of atmospheric pollutants such as sulfur and nitrogen components, contributing to the acidification of surface waters (Nisbet, 2001; Reynolds et al., 1994). Conifers also can capture ions of marine origin – Na and Mg cations. These in turn, displace hydrogen and aluminium cations from the soil, leading to acid runoff from the forests along with surface runoff, which is known as the sea-salt effect (Drinan et al., 2013; Harriman et al., 2003; Reynolds et al., 1994). We observed the presence of Pinus needles at the beginning of phase 2 (from 1838 cal CE), at the transition from pond to peatland ecosystem. Moreover, Pinus stomata were also present in palynological samples at that time, pointing to more frequent needle falls. The presence of Pinus stomata has been suggested as a possibly important proxy for insect outbreaks in palaeoecological records in previously published studies from another pine monoculture forest in Poland, Noteć Forest (Barabach, 2015), where this phenomenon has been observed (Słowiński et al., 2019). More pine trees in Tuchola Forest resulted in much higher quantities of needles and other pine fragments accumulating on the forest floor, leading to soil acidification. This, together with drier conditions, could quickly lead to acidification around the pond, forming perfect conditions for Sphagnum to encroach – as a floating mat that successively grows over the pond. We sampled the peat core close to the edge of the peatland, in the place where moss encroachment on the open water body began; therefore, we were able to track this succession in our record. This succession and the disappearance of Lake Kolze are also clearly visible on historical maps (Fig. 10). Other examples of quick encroachment of floating mats on the surface of the lake have been observed and mapped in other open water bodies in Tuchola Forest (Kowalewski, 2003; Kowalewski and Milecka, 2003) and in other regions (Warner, 1993).
4.3 Anomalies and extreme events
4.3.1 The impact of droughts and fires on the forest and peatland
Historical sources indicate that in the 18th and 19th centuries, Tuchola Forest was relatively often affected by droughts resulting in fires (Wilson, 2012). In 1781, there was a fire in Tuchola (ca. 16 km to the southwest), during which a large part of the city including the church and town hall burned down, and in 1792, Starogard Gdański (ca. 42 km to the northeast) burned almost to the ground (Orłowicz, 1924). Major fires also occurred in 1794 and 1807, when more than 34 000 ha of forest burned (Orłowicz, 1924; Schütte, 1893). Fires in 1809, 1810, 1812, 1813 and 1828 in the Świt forest district about 15 km from the study site were also recorded (Cyzman, 2008). Palaeoecological data, especially MIC, confirm high fire activity in the first decades of the 19th century (a rapid increase). Słowiński et al. (2019) emphasized that data on fires before the 1830s, especially regarding their area, should be treated with caution due to the lack of accurate measurement techniques. In the Woziwoda forest district, within which the Okoniny (Jezierzba) peatland is located, the forests of the Biała and Barłogi forest districts also burned in 1842 (Cyzman, 2008). Intense fires also appeared in Tuchola Forest between 1846 and 1848 (Orłowicz, 1924; Schütte, 1893).
Later, numerous fires were also reported in the Woziwoda forest district. Between 1860 and 1889, 310 fires were observed, destroying 4206 ha of the forest (Orłowicz, 1924; Schütte, 1893). The highest number of fires in this period was registered in 1862–1864 and 1874–1875 when 3565 ha of forest burned; altogether, nearly 85 % of the area burned in 1860–1889 (Schütte, 1893). The largest area burned in 1863 equaled 2333 ha, including more than 1250 ha in the Woziwoda forest district; altogether, 25 % of the whole forest burned during 1860–1889 (Orłowicz, 1924; Schütte, 1893). Meteorological data confirm dry years in the period from 1862 to 1865. In 1862 and 1863, the annual precipitation in Bydgoszcz was only a little over 450 mm (Kirschenstein, 2005), and it was then that the largest number of hectares of forest in the known history of Tuchola Forest burned (Dietze et al., 2019).
The number of fires can also be linked to political events (Orłowicz, 1924; Schütte, 1893; Wilson, 2012). In 1901, in the nearby Trzebciny and Gołąbek forest districts, a fire consumed 663 ha of forest (there was a parallel children's strike in Września province; Orłowicz, 1924; Wilson, 2012). Fires could also be caused by agricultural activities and land preparation for crops (Poraj-Górska et al., 2017). By the 1830s, charcoal production was widespread (McGrath et al., 2015), and forest burning was used to create heathlands for beekeeping (Bienias, 2009).
Fires in the 1860s provide a regional signal at another site in Tuchola Forest – Czechowskie Lake (Dietze et al., 2019). Increased fire activity in the mid-19th century was also observed at the Lake Jaczno site (Poraj-Górska et al., 2017). At the Okoniny (Jezierzba) peatland, MIC and MAC values decreased after 1850, but at the same time, the water level stabilized and remained high. Fire activity remained low in areas where wet conditions prevailed, such as southern Finland (Väliranta et al., 2007) and eastern Estonia (Sillasoo et al., 2011).
In 1948, about 450 ha of forest burned near Osieczna, and in 1954, 80 ha burned near Ocypel (Cherek, 2007). Palaeoecological data record an increased MIC supply during this period. The first of these fires was also recorded in the sediments of Czechowskie Lake (Słowiński et al., 2019). The summer drought of 1921 occurred over a larger area of Europe, from Poland and Czechia to the UK (van der Schrier et al., 2021). Summer droughts also affected Tuchola Forest in 1951 and 1959. In 1959, Bydgoszcz received only 37 mm of precipitation from August to October (Mitosek, 1960), and from 1950 to 1958 Bydgoszcz received less than 500 mm of rain per year (Kirschenstein, 2005). Our palaeoecological data confirm droughts in the 1950s. There is a sharp increase in the proportion of Phryganella acropodia among the testate amoebae, an indicator of dry conditions (Diaconu et al., 2017), and an expansion of brown mosses in the form of Pohlia nutans (up to 30 % of the peat sample composition) is also marked. Dendroclimatic data recorded the negative impact of climatic conditions on pine, especially strong in 1950 and 1956.
Studies show that particle size illustrates the distance of the fire from the site: the heavier the particles, the shorter distances they travel (Clark, 1988; Peters and Higuera, 2007). However, many factors determine the particles' transport – the intensity of the fire, the burning areas and the wind direction. Adolf et al. (2018) point out that the charcoal source area of both MIC and MAC can reach a radius of 40 km. However, it is often assumed that MAC indicates fires that occurred up to 1–3 km away (Clark, 1990; Higuera et al., 2007; Oris et al., 2014). The distances which particles move are also determined by terrain and vegetation. They move longer distances over flat terrain covered with grasses (Woodward and Haines, 2020), while they move shorter distances in dense forests (Kelly et al., 2013; Oris et al., 2014). In this context, we must conclude that the local fire activity in the peatland was low, with an average of 0.36 , although fires are known to have occurred nearby, according to historical sources.
4.3.2 Insect outbreaks and their impact on pine monoculture
Palaeoecological studies based on the presence of insect head capsules and/or faeces, as well as other insect remains, could be helpful, but these methods are rarely used (Bhiry and Filion, 1996; Lavoie et al., 2009; Simard et al., 2006; Waller, 2013). Often the main obstacle to performing this method is bad preservation of insect remains in peat. In the Okoniny (Jezierzba) peatland, we found no insect remains, even though quite a large sample volume was analysed for the plant macrofossil analysis. Therefore, we can interpret the effect of insect outbreaks using other sources of evidence.
The earliest information on insect outbreaks from the region of Tuchola Forest under planned forest management dates back to 1836–1838. An infestation of Panolis flammea occurred at that time (Schütte, 1893). The insects also attacked between 1866 and 1868. As a result of this infestation, 1380 ha of forest were destroyed in the Woziwoda forest district alone (Schütte, 1893). The pollen diagram from the Okoniny (Jezierzba) peatland documents the phenomenon in the 1860s, with a decrease in Pinus sylvestris pollen and an increased presence of Pinus stomata that may be indicators of the insect outbreak (Barabach, 2015). The needles that fell were partially decomposed and carried downwind to the peatland, where they were preserved (Słowiński et al., 2019). The same effect was noted in another nearby peatland in Okoniny Nadjeziorne, where the 1866–1868 infestation also corresponds with increased numbers of Pinus stomata (Tipton, 2023). In 1855, Lymantria monacha appeared in large numbers but damaged only some of the younger stands (Schütte, 1893).
A serious incidence of Panolis flammea infestation also occurred in 1922–1924 (Kiełczewski, 1947; Mokrzecki, 1928). Between 1978 and 1985, with a peak in 1982, the forests in the northern part of the country were overrun by Lymantria monacha, and this was the largest infestation since the establishment of the national forests in 1924, with salvage treatments covering more than 6.3×106 ha of forest over 7 years (Broda, 2000; Jabłoński, 2015; Śliwa, 1987, 1989). Both major infestations are reflected in palynological data, manifested by declines in the pollen percentage from trees, primarily Pinus and Picea. A decrease in conifer pollen during the infestation period has also been shown by studies of other sites in Tuchola Forest (Łuców et al., 2021; Tipton, 2023). Another pine monoculture area in Poland, Noteć Forest, was also affected by infestation in 1922–1924, and this event manifested itself in palaeoecological data (Barabach, 2015; Lamentowicz et al., 2015b; Milecka et al., 2017). Among other things, Barabach (2015) noted an increase in Glomeromycota fungal spores, which according to this author may indicate intense soil erosion caused by the felling of dead trees and a marked increase in Calluna and Poaceae, indicating an increase in the openness of the landscape. Lamentowicz et al. (2015b) noted an increase in mineral content in the sediment as indicated by Centropyxis platystoma, which was confirmed by X-ray microcomputed tomography (XMT) analysis of the peat. Milecka et al. (2017) described higher ash and charcoal content in the sediments. Although Tuchola Forest and Noteć Forest are in the region with the highest risk of outbreaks, other areas in Poland were also affected, such as the Kampinos Forest in 1972 (Śliwa, 1974) or over the last decade, the Białowieża primeval forest (Grodzki, 2016; Kamińska et al., 2021).
It is difficult to assess unequivocally whether the infestations affected the immediate vicinity of the peatland or whether this is a regional signal. Historic maps could be helpful, but these usually do not show the difference between old and new plantings (Barabach, 2012). However, dendrochronological data obtained from pine trees could help to reconstruct the extent of the outbreak. The main problem in monoculture forests, however, is that the forest is successively cut and new trees are planted regularly. However, for our dendrochronological record, we were able to obtain samples from the oldest pine trees in the area. The oldest trees in the region analysed in this study were planted over 200 years ago in the close vicinity of the Woziwoda forest district after the introduction of the Prussian forest management strategies and have been kept there by foresters to obtain tree saplings and for monitoring. The influence of insect outbreaks has been recorded in these pine trees, and we were able to track all the outbreak events in the wood. The first years after the infestations – 1839, 1869, 1925 and 1982 – manifested very strongly in the dendrochronological data as negative indicator years.
4.4 The current condition of the peatland vs. remote sensing and dendroclimatic data
The assessed growth reactions of pine trees to climate factors at the Woziwoda site may be considered typical. The effect of February air temperatures on Scots pine growth in northern Poland was previously noted (Cedro, 2001; Cedro and Lamentowicz, 2011; Feliksik and Wilczyński, 2009; Koprowski et al., 2011, 2012; Matulewski et al., 2019; Zielski, 1996; Zielski et al., 2010; Zielski and Sygit, 1998). Although the pines from Woziwoda showed a similar growth response to climate as other pines from northern Poland, their climate sensitivity was greater. The highest negative correlation for pine radial growth from the Woziwoda site was found with July's mean air temperature.
Another factor commonly affecting the radial growth of Scots pine according to the literature is pluvial conditions in February. This linkage was identified by Cedro (2001), Feliksik and Wilczyński (2009), and Koprowski et al. (2011) in the Pomeranian region (northern Poland). The present study confirmed a short-term relationship between pine radial growth and precipitation totals in February (Fig. 7). Late February and early March are when additional water is required due to the initiation of biochemical processes in trees (Przybylski, 1993). Additionally, in our study, a stronger dependence of pine radial growth on precipitation was demonstrated in June. A similar result for pine from northern Poland was obtained by Matulewski et al. (2019) and Zielski and Barankiewicz (2000), where pine growth was threatened by a water deficit in the summer season. Increased pine demand for water occurs in June and July, the months of the most intense growth (Obmiński, 1970). At the same time, these are the months when droughts have become more frequent in recent years (Łabędzki, 2004; Spinoni et al., 2018). Our results confirm that within the temperature and monthly precipitation values typically observed in central Europe, the primary environmental factor influencing the diversity of species growth in the near future will be the availability of water (Boczoń et al., 2017; Taeger et al., 2013). This availability is determined by the precipitation level and losses caused by evapotranspiration (Boczoń and Wróbel, 2015; Zajączkowski et al., 2013).
The higher climatic sensitivity of pines at the Woziwoda site was manifested also by a higher number of pointer years. The pointer years identified in this study are confirmed by earlier studies performed on pine trees in northern Poland for 1910–2014 (Matulewski et al., 2019; Zielski et al., 1998; Zielski and Barankiewicz, 2000). The years 1911, 1940, 1950 and 1982 attract particular attention. These are years in which dry and hot summers were recorded (Matulewski et al., 2019; Zielski, 1996).
Our data show that Pinus sylvestris has been under critical climatic pressure and is responding negatively to a warming climate and changing precipitation regime. Models predict a severe decline in coniferous species in the next 50 years, including Pinus sylvestris in the temperate zone of Europe (Dyderski et al., 2018; Hanewinkel et al., 2013; Schueler et al., 2014). The disappearance of the currently dominant species in the forests of central and eastern Europe will result in the profound disruption or disappearance of ecosystems functionally related to them, such as peatlands (Dyderski et al., 2018).
Peatlands are also affected by accelerating climate change, and on top of that they are at risk of losing their favourable environment, especially in Pinus sylvestris monoculture forests, which are particularly vulnerable to increasing extreme events. Studies conducted by various researchers confirm that remote sensing data provide a valuable source of information about peatlands and help in monitoring their condition (Czapiewski and Szumińska, 2021; Kaplan et al., 2019; Lees et al., 2021; Rapinel et al., 2023). The analyses conducted in this study have demonstrated that multi-sensor airborne data can be successfully utilized to assess the current state of peatland vegetation. The application of simple remote sensing indices enabled the detection of spatial differences in the condition and water stress of vegetation in the Okoniny (Jezierzba) peatland. According to Rastogi et al. (2019), NDVI values for peatland vegetation may decrease in areas affected by stress factors such as warming and reduced precipitation. Moreover, NDVI values for healthy Sphagnum moss in peatland usually range from 0.8 to 0.9 during the summer, but they are also species-dependent (Harris, 2008; Letendre et al., 2008; Péli et al., 2015). Consequently, the values of NDVI observed in this study (averaging 0.65) may indicate a prevailing drought situation in certain areas of the Okoniny (Jezierzba) peatland. Comparable findings can be drawn from the spatial variation in MSI values presented in this study. Harris et al. (2006, 2005) demonstrated that MSI is significantly correlated with the near-surface moisture condition of Sphagnum moss. Despite the wide application of optical data and spectral indices in assessing peatland conditions, Gerhards (2018) found that spectral indices may only be useful under conditions of severe or prolonged water stress. For the pre-visual detection of initial vegetation water stress symptoms, temperature-based indices are most suitable, exemplified by the LST index used in this study. Although aerial thermal data have been previously applied in peatland research (Kopeć et al., 2016), further research into the potential use of airborne thermal data in assessing peatland vegetation conditions is recommended. To date, there has been little research in Poland using spectral data in peatland monitoring (Bandopadhyay et al., 2019, 2021), and none of this research has attempted to collate palaeoecological, dendrochronological and remote sensing data.
Our data show that peatlands are highly sensitive to the progressive rise in Earth's temperatures and changing precipitation regimes. Groundwater levels have dropped dramatically in recent years, causing intense heating of the peatland surface in summer and stressing peat-forming vegetation due to water scarcity. The pine monocultures surrounding the peatlands are also sensitive to climate change. They are currently responding very strongly to summer precipitation deficiency, and these data fit into dendrological predictive models. Planned forest management has permanently changed the composition of the forest. Deciduous tree species such as Quercus, Fagus, Carpinus and Corylus avellana have almost disappeared. Forest management has also contributed to increased acidity in the peatland and thus to the rapid development of Sphagnum specialized for life in acidic conditions. After the expansion of Sphagnum, the water level in the peatland stabilized. Peatlands are also valuable archives of past climatic anomalies and catastrophic events. Pest infestations are recorded, among other things, by the presence of Pinus stomata and periods of drought by an increase in the values of coprophilous fungi. These events correspond with dendrochronological records. There is a strong correlation between the first years after hailstorms and smaller increments of tree rings. Our study shows that the combination of different data (palaeoecological, dendrochronological, remote sensing and historical) can be complementary, can create a more complete picture of past environmental changes, and can expand knowledge of best practices for local (Konczal et al., 2024) and global (Joosten, 2021) recommendations for peatland conservation in forests. Healthy wetlands could be key to protecting forests and slowing the transformation of forests caused by climate change (Marcisz et al., 2024). The results are essential for peatland conservation in planned forest management.
All data associated with this article are openly available on Mendeley Data at https://doi.org/10.17632/prdgmjcg69.3 (Bąk et al., 2024).
The supplement related to this article is available online at: https://doi.org/10.5194/bg-21-5143-2024-supplement.
MB – fieldwork, laboratory analyses (bulk density, carbon accumulation, plant macrofossils, selection of plant macrofossils for AMS radiocarbon dating), age–depth modelling, data interpretation, visualization and writing (original draft). ML – fieldwork, support in plant macrofossil analysis, data interpretation and writing (commenting and editing). PK – fieldwork, laboratory analyses (pollen and spores), age–depth modelling, data interpretation, visualization and writing (commenting and editing). DW – laboratory analyses (testate amoebae), testate amoeba-based reconstructions and data interpretation. PM – fieldwork, laboratory analyses (dendrochronology), data interpretation, visualization and writing (commenting and editing). DK and MW – fieldwork, remote sensing analyses and interpretation, and writing (commenting and editing). DJ – laboratory analyses (dendrochronology) and data interpretation. KM – funding acquisition, conceptualization, fieldwork, laboratory analyses (charcoal), testate amoeba-based reconstructions, data interpretation, visualization and writing (commenting and editing).
The contact author has declared that none of the authors has any competing interests.
Publisher's note: Copernicus Publications remains neutral with regard to jurisdictional claims made in the text, published maps, institutional affiliations, or any other geographical representation in this paper. While Copernicus Publications makes every effort to include appropriate place names, the final responsibility lies with the authors.
The study was funded by the National Science Centre, Poland, grant no. 2020/39/D/ST10/00641. Remote sensing data collection and visualization were done within the scope of the project “Protection of Valuable Ecosystems of Tuchola Forest” funded by the European Economic Area Financial Mechanism 2014–2021 within the framework of the Environment, Energy and Climate Change Programme MF EEA 2014–2021 “Implementation of Ecosystem Management Plans”.
We want to thank Stefan Konczal and other foresters from the Woziwoda forestry unit for their cooperation and help in the field, for providing us with historical maps, and for sharing knowledge of the forest's history and management. We thank Małgorzata Suchorska (Adam Mickiewicz University, Poznań) for her help in the field.
This research has been supported by the Narodowe Centrum Nauki (grant no. 2020/39/D/ST10/00641) and the EEA Grants/Norway Grants (grant no. MFEOG.07.02.01-50-0028/21-00).
This paper was edited by Petr Kuneš and reviewed by Dmitri Mauquoy and one anonymous referee.
Adolf, C., Wunderle, S., Colombaroli, D., Weber, H., Gobet, E., Heiri, O., van Leeuwen, J. F. N., Bigler, C., Connor, S. E., Gałka, M., La Mantia, T., Makhortykh, S., Svitavská-Svobodová, H., Vannière, B., and Tinner, W.: The sedimentary and remote-sensing reflection of biomass burning in Europe, Global Ecol. Biogeogr., 27, 199–212, https://doi.org/10.1111/geb.12682, 2018.
Amesbury, M. J., Swindles, G. T., Bobrov, A., Charman, D. J., Lamentowicz, M., Mallon, G., Mazei, Y., Mitchell, E. A. D., Payne, R. J., Roland, T. P., Turner, E. T., and Warner, B. G.: Development of a new pan-European testate amoeba transfer function for reconstructing peatland palaeohydrology, Quaternary Sci. Rev., 152, 132–151, https://doi.org/10.1016/j.quascirev.2016.09.024, 2016.
Anderberg, A.-L.: Atlas of seeds and small fruits of Northwest-European plant species with morphological descriptions. Part 4: Resedaceae – Umbelliferae, Risbergs Tryckeri AB, Uddevalla, 1994.
Anon: Regulation no. 64/97 of the Bydgoszcz Voivode of October 30, 1997, on the recognition as ecological sites of natural objects in the Bydgoszcz Voivodeship, 1997.
Baillie, M. G. L. and Pilcher, J.: A simple cross-dating program for tree-ring research, Tree-Ring Bull., 33, 7–14, 1973.
Bąk, M., Lamentowicz, M., Kołaczek, P., Wochal, D., Matulewski, P., Kopec, D., Wietecha, M., Jaster, D., and Marcisz, K.: Dataset for the paper: Assessing the impact of forest management and climate on a peatland under Scots pine monoculture using a multidisciplinary approach, Mendeley Data [data set], https://doi.org/10.17632/prdgmjcg69.3, 2024.
Ballesteros-Cánovas, J. A., Edvardsson, J., Corona, C., Mažeika, J., and Stoffel, M.: Estimation of recent peat accumulation with tree saplings, Progress in Physical Geography: Earth and Environment, 46, 515–529, https://doi.org/10.1177/03091333211073786, 2022.
Bandopadhyay, S., Rastogi, A., Rascher, U., Rademske, P., Schickling, A., Cogliati, S., Julitta, T., Mac Arthur, A., Hueni, A., Tomelleri, E., Celesti, M., Burkart, A., Stróżecki, M., Sakowska, K., Gąbka, M., Rosadziński, S., Sojka, M., Iordache, M.-D., Reusen, I., Van Der Tol, C., Damm, A., Schuettemeyer, D., and Juszczak, R.: Hyplant-Derived Sun-Induced Fluorescence – A New Opportunity to Disentangle Complex Vegetation Signals from Diverse Vegetation Types, Remote Sens.-Basel, 11, 1691, https://doi.org/10.3390/rs11141691, 2019.
Bandopadhyay, S., Rastogi, A., Cogliati, S., Rascher, U., Gąbka, M., and Juszczak, R.: Can Vegetation Indices Serve as Proxies for Potential Sun-Induced Fluorescence (SIF)? A Fuzzy Simulation Approach on Airborne Imaging Spectroscopy Data, Remote Sens.-Basel, 13, 2545, https://doi.org/10.3390/rs13132545, 2021.
Barabach, J.: The history of Lake Rzecin and its surroundings drawn on maps as a background to palaeoecological reconstruction, Limnological Review, 12, 103–114, https://www.mdpi.com/2300-7575/12/3/103, 2012.
Barabach, J.: Zapis zdarzeń katastrofalnych na obszarze Puszczy Noteckiej w osadach Torfowiska Rzecin, 2015.
Bauhus, J., Forrester, D. I., Gardiner, B., Jactel, H., Vallejo, R., and Pretzsch, H.: Ecological Stability of Mixed-Species Forests, in: Mixed-Species Forests, Springer, Berlin, Heidelberg, 337–382, https://doi.org/10.1007/978-3-662-54553-9_7, 2017.
Beaulne, J., Boucher, É., Garneau, M., and Magnan, G.: Paludification reduces black spruce growth rate but does not alter tree water use efficiency in Canadian boreal forested peatlands, For. Ecosyst., 8, 28, https://doi.org/10.1186/s40663-021-00307-x, 2021a.
Beaulne, J., Garneau, M., Magnan, G., and Boucher, É.: Peat deposits store more carbon than trees in forested peatlands of the boreal biome, Sci. Rep.-UK, 11, 2657, https://doi.org/10.1038/s41598-021-82004-x, 2021b.
Beck, H. E., Zimmermann, N. E., McVicar, T. R., Vergopolan, N., Berg, A., and Wood, E. F.: Present and future Köppen-Geiger climate classification maps at 1 km resolution, Sci. Data, 5, 180214, https://doi.org/10.1038/sdata.2018.214, 2018.
Becker, M., Nieminen, T., and Gérémia, F.: Short-term variations and long-term changes in oak productivity in northeastern France. The role of climate and atmospheric CO2, Ann. Sci. Forest., 51, 477–492, https://doi.org/10.1051/forest:19940504, 1994.
Berggren, G.: Atlas of seeds and small fruits of Northwest-European plant species (Sweden, Norway, Denmark, East Fennoscandia and Iceland) with morphological descriptions. Part 2: Cyperaceae, Berlingska Boktryckeriet, Lund, 1969.
Berglund, B. E. and Ralska-Jasiewiczowa, M.: Pollen analysis and pollen diagrams, in: Handbook of Holocene Palaeoecology and Palaeohydrology, edited by: Berglund, B. E., John Wiley & Sons, Chichester, 455–484, 1986.
Beug, H.-J.: Leitfaden der Pollenbestimmung für Mitteleuropa und angrenzende Gebiete, Verlag Dr. Friedrich Pfeil, München, 2004.
Bhiry, N. and Filion, L.: Mid-Holocene Hemlock Decline in Eastern North America Linked with Phytophagous Insect Activity, Quaternary Res., 45, 312–320, https://doi.org/10.1006/qres.1996.0032, 1996.
Bienias, D.: Las i człowiek w Borach Tucholskich uwagi o bartnictwie i smolarstwie w Borach Tucholskich, in: Dziedzictwo techniczne Boro' w Tucholskich, edited by: Woźny, J., Przedsiębiorstwo Marketingowe LOGO, Bydgoszcz, 43–51, 2009.
Błaszkiewicz, M., Piotrowski, J. A., Brauer, A., Gierszewski, P., Kordowski, J., Kramkowski, M., Lamparski, P., Lorenz, S., Noryśkiewicz, A. M., Ott, F., Słowiński, M., and Tyszkowski, S.: Climatic and morphological controls on diachronous postglacial lake and river valley evolution in the area of Last Glaciation, northern Poland, Quaternary Sci. Rev., 109, 13–27, https://doi.org/10.1016/j.quascirev.2014.11.023, 2015.
Blockeel, T.: Straminergon stramineum, in: Mosses and Liverworts of Britain and Ireland a field guide, edited by: Atherton, I., Bosanquet, S., and Lawley, M., British Bryological Society, Plymouth, 720, 2010.
Blodau, C.: Carbon cycling in peatlands – A review of processes and controls, Environ. Rev., 10, 111–134, https://doi.org/10.1139/a02-004, 2002.
Boczoń, A. and Wróbel, M.: Wpływ suszy na pobór wody przez sosnę zwyczajną (Pinus sylvestris L.) o różnej pozycji w drzewostanie, Leśne Prace Badawcze, 76, 370–376, 2015.
Boczoń, A., Kowalska, A., and Gawryś, R.: Glebowo-wodne uwarunkowania prowadzenia gospodarki leśnej w perspektywie zmian klimatu, Sylwan, 161, 763–771, 2017.
Bojňanský, V. and Fargašová, A.: Atlas of seeds and fruits of central and east-european flora. The Carpathian Mountains Region, Springer, Dordrecht, 2007.
Booth, R. K.: Testate amoebae as paleoindicators of surface-moisture changes on Michigan peatlands: modern ecology and hydrological calibration, J. Paleolimnol., 28, 329–348, 2002.
Booth, R. K., Lamentowicz, M., and Charman, D. J.: Preparation and analysis of testate amoebae in peatland paleoenvironmental studies, Mires Peat, 7, 1–7, 2010.
Booth, T. H.: Eucalypt plantations and climate change, Forest Ecol. Manag., 301, 28–34, https://doi.org/10.1016/j.foreco.2012.04.004, 2013.
Boulc'h, P.-N., Caullireau, E., Faucher, E., Gouerou, M., Guérin, A., Miray, R., and Couée, I.: Abiotic stress signalling in extremophile land plants, J. Exp. Bot., 71, 5771–5785, https://doi.org/10.1093/jxb/eraa336, 2020.
Broda, J.: Sosna w czasach historycznych, in: Biologia sosny zwyczajnej, edited by: Białobok, S., Boratyński, A., and Bugała, W., Instytut Dendrologii PAN, Poznań-Kórnik, 17–31, 1993.
Broda, J.: Historia leśnictwa w Polsce, Wydawnictwo Akademii Rolniczej im. Augusta Cieszkowskiego w Poznaniu, Poznań, 20–70, 2000.
Bronk Ramsey, C.: Deposition models for chronological records, Quaternary Sci. Rev., 27, 42–60, 2008.
Bunn, A. G.: A dendrochronology program library in R (dplR), Dendrochronologia, 26, 115–124, https://doi.org/10.1016/j.dendro.2008.01.002, 2008.
Cedro, A.: Próba oceny oddziaływania temperatury powietrza i opadów atmosferycznych na przyrost radialny sosny zwyczajnej (Pinus sylvestris) na Pomorzu Zachodnim, Annales Universitatis Mariae Curie-Skłodowska. Sectio B, Geographia, Geologia, Mineralogia et Petrographia, 55/56, 105–112, 2001.
Cedro, A. and Lamentowicz, M.: Contrasting responses to environmental changes by pine (Pinus sylvestris L.) growing on peat and mineral soil: An example from a Polish Baltic bog, Dendrochronologia, 29, 211–217, https://doi.org/10.1016/j.dendro.2010.12.004, 2011.
Chambers, F. M., Beilman, D. W., and Yu, Z.: Methods for determining peat humification and for quantifying peat bulk density, organic matter and carbon content for palaeostudies of climate and peatland carbon dynamics, Mires Peat, 7, 1–10, 2010.
Chapin, F. S., Matson, P. A., and Vitousek, P.: Managing and Sustaining Ecosystems, in: Principles of Terrestrial Ecosystem Ecology, edited by: Chapin, F. S., Springer, 447, 2012.
Cherek, E.: Ochotnicza Straż Pożarna w Kasparusie 1932–2007, Kasparus, 2007.
Clark, J. S.: Particle Motion and the Theory of Charcoal Analysis: Source Area, Transport, Deposition, and Sampling, Quaternary Res., 30, 67–80, https://doi.org/10.1016/0033-5894(88)90088-9, 1988.
Clark, J. S.: Fire and climate change during the last 750 yr in northwestern Minnesota, Ecol. Monogr., 60, 135–159, https://doi.org/10.2307/1943042, 1990.
Clarke, K. J.: Guide to Identification of Soil Protozoa – Testate Amoebae, edited by: Sutcliffe, D. W., Freshwater Biological Association, Ambleside, U. K., 1–40, 2003.
Clymo, R. S. and Hayward, P. M.: The Ecology of Sphagnum, in: Bryophyte Ecology, edited by: Smith, A. J. E., Chapman & Hall, London, New York, 229–289, 1982.
Cook, E. R., Briffa, K., Shiyatov, S., Mazepa, A., and Jones, P. D.: Data analysis, in: Methods of Dendrochronology: Applications in the Environmental Sciences, edited by: Cook, E. R. and Kairiukstis, L. A., Kluwer Academic Publ., Dordrecht, 97–162, 1990.
Cyzman, W.: Jednolity Program Gospodarczo-Ochronny dla Leśnego Kompleksu Promocyjnego “Bory Tucholskie”, Regionalna Dyrekcja Lasów Państwowych w Toruniu, Toruń, 122–124, 2008.
Czapiewski, S. and Szumińska, D.: An Overview of Remote Sensing Data Applications in Peatland Research Based on Works from the Period 2010–2021, Land-Basel, 11, 24, https://doi.org/10.3390/land11010024, 2021.
Czerwiński, S., Guzowski, P., Lamentowicz, M., Gałka, M., Karpińska-Kołaczek, M., Poniat, R., Łokas, E., Diaconu, A.-C., Schwarzer, J., Miecznik, M., and Kołaczek, P.: Environmental implications of past socioeconomic events in Greater Poland during the last 1200 years. Synthesis of paleoecological and historical data, Quaternary Sci. Rev., 259, 106902, https://doi.org/10.1016/j.quascirev.2021.106902, 2021.
Davis, M. B. and Deevey, E. S.: Pollen Accumulation Rates: Estimates from Late-Glacial Sediment of Rogers Lake, Science, 145, 1293–1295, https://doi.org/10.1126/science.145.3638.1293, 1964.
Diaconu, A.-C., Tóth, M., Lamentowicz, M., Heiri, O., Kuske, E., Tanţău, I., Panait, A.-M., Braun, M., and Feurdean, A.: How warm? How wet? Hydroclimate reconstruction of the past 7500 years in northern Carpathians, Romania, Palaeogeogr Palaeocl., 482, 1–12, https://doi.org/10.1016/j.palaeo.2017.05.007, 2017.
Dietze, E., Brykała, D., Schreuder, L. T., Jażdżewski, K., Blarquez, O., Brauer, A., Dietze, M., Obremska, M., Ott, F., Pieńczewska, A., Schouten, S., Hopmans, E. C., and Słowiński, M.: Human-induced fire regime shifts during 19th century industrialization: A robust fire regime reconstruction using northern Polish lake sediments, PLoS One, 14, e0222011, https://doi.org/10.1371/journal.pone.0222011, 2019.
Dinella, A., Giammarchi, F., Prendin, A. L., Carrer, M., and Tonon, G.: Xylem traits of peatland Scots pines reveal a complex climatic signal: A study in the Eastern Italian Alps, Dendrochronologia, 67, 125824, https://doi.org/10.1016/j.dendro.2021.125824, 2021.
Drinan, T. J., Graham, C. T., O'Halloran, J., and Harrison, S. S. C.: The impact of catchment conifer plantation forestry on the hydrochemistry of peatland lakes, Sci. Total Environ., 443, 608–620, https://doi.org/10.1016/j.scitotenv.2012.10.112, 2013.
Dyderski, M. K., Paź, S., Frelich, L. E., and Jagodziński, A. M.: How much does climate change threaten European forest tree species distributions?, Global Change Biol., 24, 1150–1163, https://doi.org/10.1111/gcb.13925, 2018.
Eckstein, D. and Bauch, J.: Beitrag zur Rationalisierung eines dendrochronologischen Verfahrens und zur Analyse seiner Aussagesicherheit, Forstwiss. Centralbl., 88, 230–250, https://doi.org/10.1007/BF02741777, 1969.
Edvardsson, J., Corona, C., Mažeika, J., Pukienė, R., and Stoffel, M.: Recent advances in long-term climate and moisture reconstructions from the Baltic region: Exploring the potential for a new multi-millennial tree-ring chronology, Quaternary Sci. Rev., 131, Part A, 118–126, https://doi.org/10.1016/j.quascirev.2015.11.005, 2016.
Edvardsson, J., Baužienė, I., Lamentowicz, M., Šimanauskienė, R., Tamkevičiūtė, M., Taminskas, J., Linkevičienė, R., Skuratovič, Ž., Corona, C., and Stoffel, M.: A multi-proxy reconstruction of moisture dynamics in a peatland ecosystem: A case study from Čepkeliai, Lithuania, Ecol. Indic., 106, 105484, https://doi.org/10.1016/j.ecolind.2019.105484, 2019.
Edvardsson, J., Helama, S., Rundgren, M., and Nielsen, A. B.: The Integrated Use of Dendrochronological Data and Paleoecological Records From Northwest European Peatlands and Lakes for Understanding Long-Term Ecological and Climatic Changes – A Review, Front. Ecol. Evol., 10, 781882, https://doi.org/10.3389/fevo.2022.781882, 2022.
FAO: Peatlands mapping and monitoring. Recommendations and technical overview, Rome, https://doi.org/10.4060/ca8200en, 2020.
Feliksik, E. and Wilczyński, S.: The Effect of Climate on Tree-Ring Chronologies of Native and Nonnative Tree Species Growing Under Homogenous Site Conditions, Geochronometria, 33, 49–57, https://doi.org/10.2478/v10003-009-0006-4, 2009.
Felton, A., Gustafsson, L., Roberge, J.-M., Ranius, T., Hjältén, J., Rudolphi, J., Lindbladh, M., Weslien, J., Rist, L., Brunet, J., and Felton, A. M.: How climate change adaptation and mitigation strategies can threaten or enhance the biodiversity of production forests: Insights from Sweden, Biol. Conserv., 194, 11–20, https://doi.org/10.1016/j.biocon.2015.11.030, 2016.
Finsinger, W. and Tinner, W.: Minimum count sums for charcoal-concentration estimates in pollen slides: accuracy and potential errors, Holocene, 15, 293–297, 2005.
Freeman, C., Fenner, N., Ostle, N., Kang, H., Dorwick, D. J., Reynolds, B., Lock, M. A., Sleep, D., Hughes, S., and Hudson, J.: Export of dissolved organic carbon from peatlands under elevated carbon dioxide levels, Nature, 430, 195–198, 2004.
Gałka, M., Tobolski, K., Górska, A., Milecka, K., Fiałkiewicz-Kozieł, B., and Lamentowicz, M.: Disentangling the drivers for the development of a Baltic bog during the Little Ice Age in northern Poland, Quatern. Int., 328–329, 323–337, https://doi.org/10.1016/j.quaint.2013.02.026, 2014.
Gałka, M., Miotk-Szpiganowicz, G., Marczewska, M., Barabach, J., van der Knaap, W. O., and Lamentowicz, M.: Palaeoenvironmental changes in Central Europe (NE Poland) during the last 6200 years reconstructed from a high-resolution multi-proxy peat archive, Holocene, 25, 421–434, https://doi.org/10.1177/0959683614561887, 2015.
Gałka, M., Knorr, K.-H., Tobolski, K., Gallego-Sala, A., Kołaczek, P., Lamentowicz, M., Kajukało-Drygalska, K., and Marcisz, K.: How far from a pristine state are the peatlands in the Białowieża Primeval Forest (CE Europe) – Palaeoecological insights on peatland and forest development from multi-proxy studies, Ecol. Indic., 143, 109421, https://doi.org/10.1016/j.ecolind.2022.109421, 2022.
Gallego-Sala, A. V, Charman, D. J., Brewer, S., Page, S. E., Prentice, I. C., Friedlingstein, P., Moreton, S., Amesbury, M. J., Beilman, D. W., Björck, S., Blyakharchuk, T., Bochicchio, C., Booth, R. K., Bunbury, J., Camill, P., Carless, D., Chimner, R. A., Clifford, M., Cressey, E., Courtney-Mustaphi, C., De Vleeschouwer, F., de Jong, R., Fialkiewicz-Koziel, B., Finkelstein, S. A., Garneau, M., Githumbi, E., Hribjlan, J., Holmquist, J., Hughes, P. D. M., Jones, C., Jones, M. C., Karofeld, E., Klein, E. S., Kokfelt, U., Korhola, A., Lacourse, T., Le Roux, G., Lamentowicz, M., Large, D., Lavoie, M., Loisel, J., Mackay, H., MacDonald, G. M., Makila, M., Magnan, G., Marchant, R., Marcisz, K., Martínez Cortizas, A., Massa, C., Mathijssen, P., Mauquoy, D., Mighall, T., Mitchell, F. J. G., Moss, P., Nichols, J., Oksanen, P. O., Orme, L., Packalen, M. S., Robinson, S., Roland, T. P., Sanderson, N. K., Sannel, A. B. K., Silva-Sánchez, N., Steinberg, N., Swindles, G. T., Turner, T. E., Uglow, J., Väliranta, M., van Bellen, S., van der Linden, M., van Geel, B., Wang, G., Yu, Z., Zaragoza-Castells, J., and Zhao, Y.: Latitudinal limits to the predicted increase of the peatland carbon sink with warming, Nat. Clim. Change, 8, 907–913, 2018.
Gerhards, M.: Advanced Thermal Remote Sensing for Water Stress Detection of Agricultural Crops, PhD thesis, Universität Trier, Trier, 1–140, 2018.
Godwin, H.: Archives of the Peat Bogs, Cambridge University Press, Cambridge, 1981.
González de Andrés, E., Shestakova, T. A., Scholten, R. C., Delcourt, C. J. F., Gorina, N. V, and Camarero, J. J.: Changes in tree growth synchrony and resilience in Siberian Pinus sylvestris forests are modulated by fire dynamics and ecohydrological conditions, Agr. Forest Meteorol., 312, 108712, https://doi.org/10.1016/j.agrformet.2021.108712, 2022.
Gorham, E.: Northern Peatlands: Role in the Carbon Cycle and Probable Responses to Climatic Warming, Ecol. Appl., 1, 182–195, https://doi.org/10.2307/1941811, 1991.
Gregow, H., Laaksonen, A., and Alper, M. E.: Increasing large scale windstorm damage in Western, Central and Northern European forests, 1951–2010, Sci. Rep.-UK, 7, 46397, https://doi.org/10.1038/srep46397, 2017.
Grimm, E. C.: Tilia and Tilia Graph, Illinois State Museum, 1991.
Grimm, E. C.: Tilia and Tilia-Graph. Pollen Spreadsheet and Graphics Programs, 8th International Palynological Congress (President: A. Pons), Aix-en-Provence, 6–12 September 1992, Program and Abstracts, 56, 1992.
Grissino-Mayer, H. D.: Evaluating crossdating accuracy: A manual and tutorial for the computer program COFECHA, Tree-Ring Res., 57, 205–221, 2001.
Grodzki, W.: Mass outbreaks of the spruce bark beetle Ips typographus in the context of the controversies around the Białowieża Primeval Forest, Forest Research Papers, 77, 324–331, https://open.icm.edu.pl/handle/123456789/12042 (last access: 26 April 2024), 2016.
Grondin, P., Gauthier, S., Borcard, D., Bergeron, Y., and Noël, J.: A new approach to ecological land classification for the Canadian boreal forest that integrates disturbances, Landscape Ecol., 29, 1–16, https://doi.org/10.1007/s10980-013-9961-2, 2014.
Guariguata, M. R., Cornelius, J. P., Locatelli, B., Forner, C., and Sánchez-Azofeifa, G. A.: Mitigation needs adaptation: Tropical forestry and climate change, Mitig. Adapt. Strat. Gl., 13, 793–808, https://doi.org/10.1007/s11027-007-9141-2, 2008.
Guiot, J.: The bootstrapped response function, Tree-Ring Bulletin, 51, 39–41, 1991.
Guo, M., Li, J., Sheng, C., Xu, J., and Wu, L.: A Review of Wetland Remote Sensing, Sensors, 17, 777, https://doi.org/10.3390/s17040777, 2017.
Hanewinkel, M., Cullmann, D. A., Schelhaas, M.-J., Nabuurs, G.-J., and Zimmermann, N. E.: Climate change may cause severe loss in the economic value of European forest land, Nat. Clim. Change, 3, 203–207, https://doi.org/10.1038/nclimate1687, 2013.
Hanson, P. J. and Weltzin, J. F.: Drought disturbance from climate change: response of United States forests, Sci. Total Environ., 262, 205–220, https://doi.org/10.1016/S0048-9697(00)00523-4, 2000.
Harenda, K. M., Lamentowicz, M., Samson, M., and Chojnicki, B. H.: The Role of Peatlands and Their Carbon Storage Function in the Context of Climate Change, in: Interdisciplinary Approaches for Sustainable Development Goals, edited by: Zieliński, T., Sagan, I., and Surosz, W., Springer International Publishing, Gdańsk, 169–187, https://doi.org/10.1007/978-3-319-71788-3_12, 2018.
Harriman, R., Watt, A. W., Christie, A. E. G., Moore, D. W., McCartney, A. G., and Taylor, E. M.: Quantifying the effects of forestry practices on the recovery of upland streams and lochs from acidification, Sci. Total Environ., 310, 101–111, https://doi.org/10.1016/S0048-9697(02)00626-5, 2003.
Harris, A.: Spectral reflectance and photosynthetic properties of Sphagnum mosses exposed to progressive drought, Ecohydrology, 1, 35–42, https://doi.org/10.1002/eco.5, 2008.
Harris, A., Bryant, R., and Baird, A.: Detecting near-surface moisture stress in spp., Remote Sens. Environ., 97, 371–381, https://doi.org/10.1016/j.rse.2005.05.001, 2005.
Harris, A., Bryant, R. G., and Baird, A. J.: Mapping the effects of water stress on Sphagnum: Preliminary observations using airborne remote sensing, Remote Sens. Environ., 100, 363–378, https://doi.org/10.1016/j.rse.2005.10.024, 2006.
Haylock, M. R., Hofstra, N., Klein Tank, A. M. G., Klok, E. J., Jones, P. D., and New, M.: A European daily high-resolution gridded data set of surface temperature and precipitation for 1950–2006, J. Geophys. Res.-Atmos., 113, D20119, https://doi.org/10.1029/2008JD010201, 2008.
Hedenäs, L.: A generic revision of the Warnstorfia-Calliergon group, J. Bryol., 17, 447–479, https://doi.org/10.1179/jbr.1993.17.3.447, 1993.
Heiri, O., Lotter, A. F., and Lemcke, G.: Loss on ignition as a method for estimating organic and carbonate content in sediments: Reproducibility and comparability of results, J. Paleolimnol., 25, 101–110, https://doi.org/10.1023/A:1008119611481, 2001.
Higuera, P., Peters, M., Brubaker, L., and Gavin, D.: Understanding the origin and analysis of sediment-charcoal records with a simulation model, Quaternary Sci. Rev., 26, 1790–1809, https://doi.org/10.1016/j.quascirev.2007.03.010, 2007.
Hill, M. O. and Blockeel, T. L.: Straminergon stramineum, in: Atlas of British and Irish Bryophytes, edited by: Blockeel, T. L., Bosanquet, S. D. S., Hill, M. O., and Preston, C. D., British Bryological Society, Newbury, Berkshire, 464, 2014.
Hua, Q., Turnbull, J. C., Santos, G. M., Rakowski, A. Z., Ancapichún, S., De Pol-Holz, R., Hammer, S., Lehman, S. J., Levin, I., Miller, J. B., Palmer, J. G., and Turney, C. S. M.: Atmospheric Radiocarbon for the Period 1950–2019, Radiocarbon, 64, 1–23, https://doi.org/10.1017/RDC.2021.95, 2021.
Hunt, E. and Rock, B.: Detection of changes in leaf water content using Near- and Middle-Infrared reflectances?, Remote Sens. Environ., 30, 43–54, https://doi.org/10.1016/0034-4257(89)90046-1, 1989.
Huuskonen, S., Domisch, T., Finér, L., Hantula, J., Hynynen, J., Matala, J., Miina, J., Neuvonen, S., Nevalainen, S., Niemistö, P., Nikula, A., Piri, T., Siitonen, J., Smolander, A., Tonteri, T., Uotila, K., and Viiri, H.: What is the potential for replacing monocultures with mixed-species stands to enhance ecosystem services in boreal forests in Fennoscandia?, Forest Ecol. Manag., 479, 118558, https://doi.org/10.1016/j.foreco.2020.118558, 2021.
Jabłoński, T.: Występowanie i zwalczanie leśnych foliofagów – trendy i prognozy, Postępy Techniki w Leśnictwie, 132, 13–19, 2015.
Jäger, E.: Die Schroettersche Landesaufnahme von Ost- und Westpreußen (1796–1802). Entstehungsgeschichte, Herstellung und Vertrieb der Karte, Z. Ostforsch., 30, 359–389, 1981.
Jäger, E.: Prussia-Karten 1542–1810: Geschichte der kartographischen Darstellung Ostpreussens vom 16. bis zum 19. Jahrhundert. Entstehung der Karten – Kosten – Vertrieb: bibliographischer Katalog, Anton H. Konrad Verlag, Weißenhorn, 1982.
Jasnowski, M.: Budowa i roślinność torfowisk Pomorza Szczecińskiego, Societas Scientarium Stetinensis, Szczecin, 1962.
Joosten, H.: Global guidelines for peatland rewetting and restoration, Ramsar Technical Report no. 11, Gland, Switzerland, 2021.
Joosten, H., Tapio-Biström, M.-L., and Tol, S.: Peatlands – guidance for climate change mitigation through conservation, rehabilitation and sustainable use, 2nd edn., Food and Agriculture Organization of the United Nations, Rome, 2012.
Juggins, S.: C2 Version 1.5 User guide. Software for ecological and palaeoecological data analysis and visualisation, Newcastle University, Newcastle upon Tyne, UK, 73, 2007.
Kamińska, A., Lisiewicz, M., Kraszewski, B., and Stereńczak, K.: Mass outbreaks and factors related to the spatial dynamics of spruce bark beetle (Ips typographus) dieback considering diverse management regimes in the Białowieża forest, Forest Ecol. Manag., 498, 119530, https://doi.org/10.1016/j.foreco.2021.119530, 2021.
Kaplan, G., Yigit Avdan, Z., and Avdan, U.: Mapping and Monitoring Wetland Dynamics Using Thermal, Optical, and SAR Remote Sensing Data, in: Wetlands Management – Assessing Risk and Sustainable Solutions, IntechOpen, https://doi.org/10.5772/intechopen.80264, 2019.
Karpińska-Kołaczek, M., Kołaczek, P., Marcisz, K., Gałka, M., Kajukało-Drygalska, K., Mauquoy, D., and Lamentowicz, M.: Kettle-hole peatlands as carbon hot spots: Unveiling controls of carbon accumulation rates during the last two millennia, Catena, 237, 107764, https://doi.org/10.1016/j.catena.2023.107764, 2024.
Kelly, R., Chipman, M. L., Higuera, P. E., Stefanova, I., Brubaker, L. B., and Hu, F. S.: Recent burning of boreal forests exceeds fire regime limits of the past 10 000 years, P. Natl. Acad. Sci. USA, 110, 13055–13060, https://doi.org/10.1073/pnas.1305069110, 2013.
Kiełczewski, B.: Klęska sówki chojnówki jako zagadnienie biocenotyczne, Prace Komisji Matematyczno-Przyrodniczej, 10, 167–171, 1947.
Kirschenstein, M.: Wieloletnie zmiany sum opadów atmosferycznych na wybranych stacjach północno-zachodniej Polski, Słupskie Prace Geograficzne, 2, 199–214, 2005.
Klein Tank, A. M. G., Wijngaard, J. B., Können, G. P., Böhm, R., Demarée, G., Gocheva, A., Mileta, M., Pashiardis, S., Hejkrlik, L., Kern-Hansen, C., Heino, R., Bessemoulin, P., Müller-Westermeier, G., Tzanakou, M., Szalai, S., Pálsdóttir, T., Fitzgerald, D., Rubin, S., Capaldo, M., Maugeri, M., Leitass, A., Bukantis, A., Aberfeld, R., van Engelen, A. F. V., Forland, E., Mietus, M., Coelho, F., Mares, C., Razuvaev, V., Nieplova, E., Cegnar, T., Antonio López, J., Dahlström, B., Moberg, A., Kirchhofer, W., Ceylan, A., Pachaliuk, O., Alexander, L. V., and Petrovic, P.: Daily dataset of 20th-century surface air temperature and precipitation series for the European Climate Assessment, Int. J. Climatol., 22, 1441–1453, https://doi.org/10.1002/joc.773, 2002.
Koenig, I., Mulot, M., and Mitchell, E. A. D.: Taxonomic and functional traits responses of Sphagnum peatland testate amoebae to experimentally manipulated water table, Ecol. Indic., 85, 342–351, https://doi.org/10.1016/j.ecolind.2017.10.017, 2018.
Kołaczek, P., Karpińska-Kołaczek, M., Marcisz, K., Gałka, M., and Lamentowicz, M.: Palaeohydrology and the human impact on one of the largest raised bogs complex in the Western Carpathians (Central Europe) during the last two millennia, Holocene, 28, 595–608, https://doi.org/10.1177/0959683617735587, 2018.
Konczal, S., Lamentowicz M, Bąk, M., Czerwiński, S., Kołaczek, P., Wochal, D., Marcisz, M., Chojnicki, B., Harenda, K., Poczta, P., Gąbka, M., Jaster, D., Matulewski, P., Jedliński, J., Niedzielko, J., Wylazłowska, J., Żmuda, M., Żmuda, D., Kopeć, D., Rosadziński, S., Wietecha, M., Landowska, J., and Landowski, J.: Rekomendacje dla ochrony mokradeł w lasach, in: Jak chronić torfowiska w lasach?, edited by: Lamentowicz, M. and Konczal, S., ArchaeGraph, Łódź, 161–165, 2024.
Kondracki, J.: Geografia regionalna Polski, Wydawnictwo Naukowe PWN, Warszawa, 2001.
Kopeć, D., Michalska-Hejduk, D., Sławik, Ł., Berezowski, T., Borowski, M., Rosadziński, S., and Chormański, J.: Application of multisensoral remote sensing data in the mapping of alkaline fens Natura 2000 habitat, Ecol. Indic., 70, 196–208, https://doi.org/10.1016/j.ecolind.2016.06.001, 2016.
Koprowski, M., Zielski, A., and Skowronek, T.: Analiza przyrostów rocznych dwóch sosen (Pinus sylvestris) o nietypowej budowie strzały na terenie Nadleśnictwa Borne Sulinowo, Sylwan, 155, 555–562, 2011.
Koprowski, M., Przybylak, R., Zielski, A., and Pospieszyńska, A.: Tree rings of Scots pine (Pinus sylvestris L.) as a source of information about past climate in northern Poland, Int. J. Biometeorol., 56, 1–10, https://doi.org/10.1007/s00484-010-0390-5, 2012.
Kowalewski, G.: Shoreline changes of basins in the mire-lake reserves in s Tuchola Pinewoods, Limnological Review, 3, 119–126, 2003.
Kowalewski, G. and Milecka, K.: Palaeoecology of basins of organic sediment accumulation in the Reserve Dury, Studia Quaternaria, 20, 73–82, 2003.
Kuosmanen, N., Čada, V., Halsall, K., Chiverrell, R. C., Schafstall, N., Kuneš, P., Boyle, J. F., Knížek, M., Appleby, P. G., Svoboda, M., and Clear, J. L.: Integration of dendrochronological and palaeoecological disturbance reconstructions in temperate mountain forests, Forest Ecol. Manag., 475, 118413, https://doi.org/10.1016/j.foreco.2020.118413, 2020.
Łabędzki, L.: Problematyka susz w Polsce, Woda-Środowisko-Obszary Wiejskie, 4, 47–66, 2004.
Laine, J., Vasander, H., and Laiho, R.: Long-Term Effects of Water Level Drawdown on the Vegetation of Drained Pine Mires in Southern Finland, J. Appl. Ecol., 32, 785–802, https://doi.org/10.2307/2404818, 1995.
Laine, J., Flatberg, K. I., Harju, P., Timonen, T., Minkinen, K., Laine, A., Tuittila, E.-S., and Vasander, H.: Sphagnum mosses. The Stars of European Mires, Department of Forest Sciences, University of Helsinki, Sphagna Ky, Helsinki, 58–62, 2018.
Lamentowicz, Ł., Gąbka, M., Rusińska, A., Sobczyński, T., Owsianny, P. M., and Lamentowicz, M.: Testate amoeba (Arcellinida, Euglyphida) ecology along a poor-rich gradient in fens of western Poland, Int. Rev. Hydrobiol., 96, 256–380, 2011.
Lamentowicz, M. and Mitchell, E. A. D.: Testate amoebae (Protists) as palaeoenvironmental indicators in peatlands, Polish Geological Institute Special Papers, 16, 58–64, 2005a.
Lamentowicz, M. and Mitchell, E. A. D.: The ecology of testate amoebae (Protists) in Sphagnum in north-western Poland in relation to peatland ecology, Microb. Ecol., 50, 48–63, 2005b.
Lamentowicz, M. and Obremska, M.: A rapid response of testate amoebae and vegetation to inundation of a kettle hole mire, J. Paleolimnol., 43, 499–511, https://doi.org/10.1007/s10933-009-9347-2, 2010.
Lamentowicz, M., Tobolski, K., and Mitchell, E. A. D.: Palaeoecological evidence for anthropogenic acidification of a kettle-hole peatland in northern Poland, Holocene, 17, 1185–1196, 2007.
Lamentowicz, M., Obremska, M., and Mitchell, E. A. D.: Autogenic succession, land-use change, and climatic influences on the Holocene development of a kettle hole mire in Northern Poland, Rev. Palaeobot. Palyno., 151, 21–40, https://doi.org/10.1016/j.revpalbo.2008.01.009, 2008a.
Lamentowicz, M., Cedro, A., Gałka, M., Goslar, T., Miotk-Szpiganowicz, G., Mitchell, E. A. D., and Pawlyta, J.: Last millennium palaeoenvironmental changes from a Baltic bog (Poland) inferred from stable isotopes, pollen, plant macrofossils and testate amoebae, Palaeogeogr Palaeocl., 265, 93–106, 2008b.
Lamentowicz, M., Balwierz, Z., Forysiak, J., Płóciennik, M., Kittel, P., Kloss, M., Twardy, J., Żurek, S., and Pawlyta, J.: Multiproxy study of anthropogenic and climatic changes in the last two millennia from a small mire in central Poland, Hydrobiologia, 631, 213–230, https://doi.org/10.1007/s10750-009-9812-y, 2009a.
Lamentowicz, M., Milecka, K., Gałka, M., Cedro, A., Pawlyta, J., Piotrowska, N., Lamentowicz, Ł., and van der Knaap, W. O.: Climate and human induced hydrological change since AD 800 in an ombrotrophic mire in Pomerania (N Poland) tracked by testate amoebae, macro-fossilis, pollen tree-rings of pine, Boreas, 38, 214–229, 2009b.
Lamentowicz, M., Gałka, M., Lamentowicz, Ł., Obremska, M., Kühl, N., Lücke, A., and Jassey, V. E. J.: Reconstructing climate change and ombrotrophic bog development during the last 4000 years in northern Poland using biotic proxies, stable isotopes and trait-based approach, Palaeogeogr Palaeocl., 418, 261–277, https://doi.org/10.1016/j.palaeo.2014.11.015, 2015a.
Lamentowicz, M., Mueller, M., Gałka, M., Barabach, J., Milecka, K., Goslar, T., and Binkowski, M.: Reconstructing human impact on peatland development during the past 200 years in CE Europethrough biotic proxies and X-ray tomography, Quatern. Int., 357, 282–294, https://doi.org/10.1016/j.quaint.2014.07.045, 2015b.
Lamentowicz, M., Marcisz, K., Guzowski, P., Gałka, M., Diaconu, A.-C., and Kołaczek, P.: How Joannites' economy eradicated primeval forest and created anthroecosystems in medieval Central Europe, Sci. Rep.-UK, 10, 18775, https://doi.org/10.1038/s41598-020-75692-4, 2020.
Lavoie, M., Filion, L., and Robert, É. C.: Boreal peatland margins as repository sites of long-term natural disturbances of balsam fir/spruce forests, Quaternary Res., 71, 295–306, https://doi.org/10.1016/j.yqres.2009.01.005, 2009.
Lee, D., Holmström, E., Hynynen, J., Nilsson, U., Korhonen, K. T., Westerlund, B., Bianchi, S., Aldea, J., and Huuskonen, S.: Current state of mixed forests available for wood supply in Finland and Sweden, Scand. J. Forest Res., 38, 442–452, https://doi.org/10.1080/02827581.2023.2259797, 2023.
Lees, K. J., Artz, R. R. E., Chandler, D., Aspinall, T., Boulton, C. A., Buxton, J., Cowie, N. R., and Lenton, T. M.: Using remote sensing to assess peatland resilience by estimating soil surface moisture and drought recovery, Sci. Total Environ., 761, 143312, https://doi.org/10.1016/j.scitotenv.2020.143312, 2021.
Letendre, J., Poulin, M., and Rochefort, L.: Sensitivity of spectral indices to CO2 fluxes for several plant communities in a Sphagnum-dominated peatland, Can. J. Remote Sens., 34, S414–S425, https://doi.org/10.5589/m08-053, 2008.
Loisel, J., Yu, Z., Beilman, D. W., Camill, P., Alm, J., Amesbury, M. J., Anderson, D., Andersson, S., Bochicchio, C., Barber, K., Belyea, L. R., Bunbury, J., Chambers, F. M., Charman, D. J., De Vleeschouwer, F., Fiałkiewicz-Kozieł, B., Finkelstein, S. A., Gałka, M., Garneau, M., Hammarlund, D., Hinchcliffe, W., Holmquist, J., Hughes, P., Jones, M. C., Klein, E. S., Kokfelt, U., Korhola, A., Kuhry, P., Lamarre, A., Lamentowicz, M., Large, D., Lavoie, M., MacDonald, G., Magnan, G., Mäkilä, M., Mallon, G., Mathijssen, P., Mauquoy, D., McCarroll, J., Moore, T. R., Nichols, J., O'Reilly, B., Oksanen, P., Packalen, M., Peteet, D., Richard, P. J. H., Robinson, S., Ronkainen, T., Rundgren, M., Sannel, A. B. K., Tarnocai, C., Thom, T., Tuittila, E.-S., Turetsky, M., Väliranta, M., van der Linden, M., van Geel, B., van Bellen, S., Vitt, D., Zhao, Y., and Zhou, W.: A database and synthesis of northern peatland soil properties and Holocene carbon and nitrogen accumulation, Holocene, 24, 1028–1042, https://doi.org/10.1177/0959683614538073, 2014.
Łuców, D., Lamentowicz, M., Kołaczek, P., Łokas, E., Marcisz, K., Obremska, M., Theuerkauf, M., Tyszkowski, S., and Słowiński, M.: Pine Forest Management and Disturbance in Northern Poland: Combining High-Resolution 100-Year-Old Paleoecological and Remote Sensing Data, Front. Ecol. Evol., 9, 747976, https://doi.org/10.3389/fevo.2021.747976, 2021.
Łuców, D., Küttim, M., Słowiński, M., Kołaczek, P., Karpińska-Kołaczek, M., Küttim, L., Salme, M., and Lamentowicz, M.: Searching for an ecological baseline: Long-term ecology of a post-extraction restored bog in Northern Estonia, Quatern. Int., 607, 65–78, https://doi.org/10.1016/j.quaint.2021.08.017, 2022.
Marcisz, K., Tinner, W., Colombaroli, D., Kołaczek, P., Słowiński, M., Fiałkiewicz-Kozieł, B., Łokas, E., and Lamentowicz, M.: Long-term hydrological dynamics and fire history over the last 2000 years in CE Europe reconstructed from a high-resolution peat archive, Quaternary Sci. Rev., 112, 138–152, https://doi.org/10.1016/j.quascirev.2015.01.019, 2015.
Marcisz, K., Gałka, M., Pietrala, P., Miotk-Szpiganowicz, G., Obremska, M., Tobolski, K., and Lamentowicz, M.: Fire activity and hydrological dynamics in the past 5700 years reconstructed from Sphagnum peatlands along the oceanic–continental climatic gradient in northern Poland, Quaternary Sci. Rev., 177, 145–157, https://doi.org/10.1016/j.quascirev.2017.10.018, 2017.
Marcisz, K., Jassey, V. E. J., Kosakyan, A., Krashevska, V., Lahr, D. J. G., Lara, E., Lamentowicz, Ł., Lamentowicz, M., Macumber, A., Mazei, Y., Mitchell, E. A. D., Nasser, N. A., Patterson, R. T., Roe, H. M., Singer, D., Tsyganov, A. N., and Fournier, B.: Testate Amoeba Functional Traits and Their Use in Paleoecology, Front. Ecol. Evol., 8, 340, https://doi.org/10.3389/fevo.2020.575966, 2020a.
Marcisz, K., Kołaczek, P., Gałka, M., Diaconu, A.-C., and Lamentowicz, M.: Exceptional hydrological stability of a Sphagnum-dominated peatland over the late Holocene, Quaternary Sci. Rev., 231, 106180, https://doi.org/10.1016/j.quascirev.2020.106180, 2020b.
Marcisz, K., Bąk, M., Kołaczek, P., Lamentowicz, M., and Wochal, D.: Historia lasu i mokradełzapisana w torfowiskach, in: Jak chronić torfowiska w lasach?, edited by: Lamentowicz, M. and Konczal, S., ArchaeGraph, Łódź, 29–45, 2024.
Marks, L.: Timing of the Late Vistulian (Weichselian) glacial phases in Poland, Quaternary Sci. Rev., 44, 81–88, https://doi.org/10.1016/j.quascirev.2010.08.008, 2012.
Matthias, I. and Giesecke, T.: Insights into pollen source area, transport and deposition from modern pollen accumulation rates in lake sediments, Quaternary Sci. Rev., 87, 12–23, https://doi.org/10.1016/j.quascirev.2013.12.015, 2014.
Matulewski, P., Buchwal, A., and Makohonienko, M.: Higher climatic sensitivity of Scots pine (Pinus sylvestris L.) subjected to tourist pressure on a hiking trail in the Brodnica Lakeland, NE Poland, Dendrochronologia, 54, 78–86, https://doi.org/10.1016/j.dendro.2019.02.008, 2019.
Mauquoy, D. and van Geel, B.: Mire and peat macros, in: Encyclopedia of Quaternary Science, vol. 3, Elsevier, Heidelberg, 2315–2336, 2007.
Mauquoy, D. and Yeloff, D.: Raised peat bog development and possible responses to environmental changes during the mid- to late-Holocene. Can the palaeoecological record be used to predict the nature and response of raised peat bogs to future climate change?, Biodivers. Conserv., 17, 2139–2151, https://doi.org/10.1007/s10531-007-9222-2, 2008.
Mauquoy, D., Hughes, P. D. M., and van Geel, B.: A protocol for plant macrofossil analysis of peat deposits, Mires Peat, 7, 1–5, 2010.
Mazei, Y. and Tsyganov, A. N.: Freshwater testate amoebae, KMK, Moscow, 2006.
McCarroll, D. and Loader, N. J.: Stable isotopes in tree rings, Quaternary Sci. Rev., 23, 771–801, https://doi.org/10.1016/j.quascirev.2003.06.017, 2004.
McGrath, M. J., Luyssaert, S., Meyfroidt, P., Kaplan, J. O., Bürgi, M., Chen, Y., Erb, K., Gimmi, U., McInerney, D., Naudts, K., Otto, J., Pasztor, F., Ryder, J., Schelhaas, M.-J., and Valade, A.: Reconstructing European forest management from 1600 to 2010, Biogeosciences, 12, 4291–4316, https://doi.org/10.5194/bg-12-4291-2015, 2015.
McNulty, S., Caldwell, P., Doyle, T. W., Johnsen, K., Liu, Y., Mohan, J., Prestemon, J., and Sun, G.: Forests and Climate Change in the Southeast USA, in: Climate of the Southeast United States, edited by: Ingram, K. T., Dow, K., Carter, L., and Anderson, J., Island Press, Washington, 165–189, 2013.
Meisterfeld, R.: Testate amoebae, in: Patrimoines Naturels, vol. 50, edited by: Costello, M. J., Emblow, C. S., and White, R., Muséum National d'Histoire Naturelle – Institut d'Ecologie et de Gestion de la Biodiversité (I. E. G. B.) – Service du Patrimoine Naturel (S. P. N.), Paris, 54–57, 2001.
Messier, C., Bauhus, J., Sousa-Silva, R., Auge, H., Baeten, L., Barsoum, N., Bruelheide, H., Caldwell, B., Cavender-Bares, J., Dhiedt, E., Eisenhauer, N., Ganade, G., Gravel, D., Guillemot, J., Hall, J. S., Hector, A., Hérault, B., Jactel, H., Koricheva, J., Kreft, H., Mereu, S., Muys, B., Nock, C. A., Paquette, A., Parker, J. D., Perring, M. P., Ponette, Q., Potvin, C., Reich, P. B., Scherer-Lorenzen, M., Schnabel, F., Verheyen, K., Weih, M., Wollni, M., and Zemp, D. C.: For the sake of resilience and multifunctionality, let's diversify planted forests!, Conserv. Lett., 15, e12829, https://doi.org/10.1111/conl.12829, 2022.
Microworld, world of amoeboid organisms: https://arcella.nl/, last access: 23 November 2023.
Milecka, K., Kowalewski, G., Fiałkiewicz-Kozieł, B., Gałka, M., Lamentowicz, M., Chojnicki, B. H., Goslar, T., and Barabach, J.: Hydrological changes in the Rzecin peatland (Puszcza Notecka, Poland) induced by anthropogenic factors: Implications for mire development and carbon sequestration, Holocene, 27, 651–664, https://doi.org/10.1177/0959683616670468, 2017.
Miller, J. D., Anderson, H. A., Ferrier, R. C., and Walker, T. A. B.: Hydrochemical Fluxes and their Effects on Stream Acidity in Two Forested Catchments in Central Scotland, Forestry, 63, 311–331, https://doi.org/10.1093/forestry/63.4.311, 1990.
Minkkinen, K., Byrne, K. A., and Trettin, C.: Climate impacts of peatland forestry, in: Peatlands and climate change, edited by: Strack, M., International Peat Society, Saarijärvi, 98–122, 2008.
Miola, A.: Tools for Non-Pollen Palynomorphs (NPPs) analysis: A list of Quaternary NPP types and reference literature in English language (1972–2011), Rev. Palaeobot. Palyno., 186, 142–161, https://doi.org/10.1016/j.revpalbo.2012.06.010, 2012.
Mirek, Z., Zarzycki, K., Wojewoda, W., and Szeląg, Z.: Red list of plants and fungi in Poland. Czerwona lista roślin i grzybów Polski, Instytut Botaniki im. W. Szafera, Polska Akademia Nauk, Kraków, 2006.
Mitosek, H.: Letnio-jesienna susza 1959 r., Postępy Nauk Rolniczych, 7, 53–64, 1960.
Mokrzecki, Z.: Strzygonia choinówka, Związek Zawodowy Leśników w Rzeczypospolitej Polskiej, Warszawa, 1928.
Moore, P. D., Webb, J. A., and Collinson, M. E.: Pollen Analysis, Blackwell Scientific Publications, Oxford, 1991.
Moritz, M. A., Parisien, M.-A., Batllori, E., Krawchuk, M. A., Van Dorn, J., Ganz, D. J., and Hayhoe, K.: Climate change and disruptions to global fire activity, Ecosphere, 3, 1–22, https://doi.org/10.1890/ES11-00345.1, 2012.
Mroczkowska, A., Kittel, P., Marcisz, K., Dolbunova, E., Gauthier, E., Lamentowicz, M., Mazurkevich, A., Obremska, M., Płóciennik, M., Kramkowski, M., Łuców, D., Kublitskiy, Y., and Słowiński, M.: Small peatland with a big story: 600 year paleoecological and historical data from a kettle-hole peatland in Western Russia, Holocene, 31, 1761–1776, https://doi.org/10.1177/09596836211033224, 2021.
Nisbet, T. R.: The role of forest management in controlling diffuse pollution in UK forestry, Forest Ecol. Manag., 143, 215–226, https://doi.org/10.1016/S0378-1127(00)00519-3, 2001.
Obmiński, Z.: Zarys ekologii, in: Sosna zwyczajna. Nasze drzewa leśne, edited by: Białobok, S., Warszawa-Poznań, 152–231, 1970.
Ogden, C. G. and Hedley, R. H.: An Atlas of Freshwater Testate Amoebae, 1st Edn., Oxford University Press, London, 1–228, 1980.
Oris, F., Ali, A. A., Asselin, H., Paradis, L., Bergeron, Y., and Finsinger, W.: Charcoal dispersion and deposition in boreal lakes from 3 years of monitoring: Differences between local and regional fires, Geophys. Res. Lett., 41, 6743–6752, https://doi.org/10.1002/2014GL060984, 2014.
Orłowicz, M.: Ilustrowany przewodnik po województwie pomorskiem, Książnica Polska, Lwów, 360–361, 1924.
OxCal v4.4.4: https://c14.arch.ox.ac.uk/oxcal.html, last access: 21 November 2023.
Paavilainen, E. and Päivänen, J.: Peatland Forestry: Ecology and Principles, Springer, Berlin, 1995.
Parish, F., Sirin, A., Charman, D. J., Joosten, H., Minayeva, T., Silvius, M., and Stringer, L.: Assessment on peatlands, biodiversity and climate change: main report, 2008.
Pawlyta, J. and Lamentowicz, M.: Age-depth model for modern peat core. Methodological approach, methods of absolute chronology., in: Methods of absolute chronology: 10th International Conference, GADAM Centre of Excellence. Department of Radioisotopes, Institute of Physics, Silesian University of Technology, Gliwice, Poland, 22–25 April 2010, p. 109, 2010.
Payne, R. J. and Mitchell, E. A. D.: How many is enough? Determining optimal count totals for ecological and palaeoecological studies of testate amoebae, J. Paleolimnol., 42, 483–495, https://doi.org/10.1007/s10933-008-9299-y, 2009.
Pędziszewska, A. and Latałowa, M.: Stand-scale reconstruction of late Holocene forest succession on the Gdańsk Upland (N. Poland) based on integrated palynological and macrofossil data from paired sites, Veg. Hist. Archaeobot., 25, 239–254, https://doi.org/10.1007/s00334-015-0546-7, 2016.
Péli, E. R., Nagy, J. G., and Cserhalmi, D.: In situ measurements of seasonal productivity dynamics in two sphagnum dominated mires in Hungary, Carpath. J. Earth Env., 10, 231–240, 2015.
Peters, M. E. and Higuera, P. E.: Quantifying the source area of macroscopic charcoal with a particle dispersal model, Quaternary Res., 67, 304–310, https://doi.org/10.1016/j.yqres.2006.10.004, 2007.
Poraj-Górska, A. I., Żarczyński, M. J., Ahrens, A., Enters, D., Weisbrodt, D., and Tylmann, W.: Impact of historical land use changes on lacustrine sedimentation recorded in varved sediments of Lake Jaczno, northeastern Poland, Catena, 153, 182–193, https://doi.org/10.1016/j.catena.2017.02.007, 2017.
Przybylski, T.: Ekologia, in: Biologia sosny zwyczajnej, edited by: Białobok, S., Boratyński, A., and Bugała, W., Sorus, Poznań-Kórnik, 255–300, 1993.
Pureswaran, D. S., De Grandpré, L., Paré, D., Taylor, A., Barrette, M., Morin, H., Régnière, J., and Kneeshaw, D. D.: Climate-induced changes in host tree–insect phenology may drive ecological state-shift in boreal forests, Ecology, 96, 1480–1491, https://doi.org/10.1890/13-2366.1, 2015.
R: A Language and Environment for Statistical Computing, R Foundation for Statistical Computing, https://www.R-project.org, last access: 4 December 2023.
Rapinel, S., Panhelleux, L., Gayet, G., Vanacker, R., Lemercier, B., Laroche, B., Chambaud, F., Guelmami, A., and Hubert-Moy, L.: National wetland mapping using remote-sensing-derived environmental variables, archive field data, and artificial intelligence, Heliyon, 9, e13482, https://doi.org/10.1016/j.heliyon.2023.e13482, 2023.
Rastogi, A., Stróżecki, M., Kalaji, H. M., Łuców, D., Lamentowicz, M., and Juszczak, R.: Impact of warming and reduced precipitation on photosynthetic and remote sensing properties of peatland vegetation, Environ. Exp. Bot., 160, 71–80, https://doi.org/10.1016/j.envexpbot.2019.01.005, 2019.
Reimer, P. J., Austin, W. E. N., Bard, E., Bayliss, A., Blackwell, P. G., Bronk Ramsey, C., Butzin, M., Cheng, H., Edwards, R. L., Friedrich, M., Grootes, P. M., Guilderson, T. P., Hajdas, I., Heaton, T. J., Hogg, A. G., Hughen, K. A., Kromer, B., Manning, S. W., Muscheler, R., Palmer, J. G., Pearson, C., van der Plicht, J., Reimer, R. W., Richards, D. A., Scott, E. M., Southon, J. R., Turney, C. S. M., Wacker, L., Adolphi, F., Büntgen, U., Capano, M., Fahrni, S. M., Fogtmann-Schulz, A., Friedrich, R., Köhler, P., Kudsk, S., Miyake, F., Olsen, J., Reinig, F., Sakamoto, M., Sookdeo, A., and Talamo, S.: The IntCal20 Northern Hemisphere Radiocarbon Age Calibration Curve (0–55 cal kBP), Radiocarbon, 62, 725–757, https://doi.org/10.1017/RDC.2020.41, 2020.
Reynolds, B., Ormerod, S. J., and Gee, A. S.: Spatial patterns concentrations in upland Wales in relation to catchment forest cover and forest age, Environ. Pollut., 84, 27–33, https://doi.org/10.1016/0269-7491(94)90067-1, 1994.
Rioja: Analysis of Quaternary Science Data, https://cran.r-project.org/web/packages/rioja/index.html, last access: 4 December 2023.
Rouse, J. W., Haas, R. H., Schell, J. A., and Deering, D. W.: Monitoring vegetation systems in the great plains with ERTS, in: Third Earth Resources Technology Satellite-l Symposium, Volume I: Technical Presentations Section A. Paper A-20. Goddard Space Flight Center: Washington, D.C., December 10–14, 1973, National Aeronautics and Space Administration: Washington, D.C., 309–317, 1974.
Rydin, H. and Jeglum, J. K.: The biology of peatlands, 2nd Edn., Oxford University Press, 2013.
Sanderson, N., Loisel, J., Gallego-Sala, A., Anshari, G., Novita, N., Marcisz, K., Lamentowicz, M., Bąk, M., and Wochal, D.: Setting a new research agenda for tropical peatlands, recent carbon accumulation and ecosystem services, Past Global Changes Magazine, 31, 121–121, https://doi.org/10.22498/pages.31.2.121, 2023.
Schueler, S., Falk, W., Koskela, J., Lefèvre, F., Bozzano, M., Hubert, J., Kraigher, H., Longauer, R., and Olrik, D. C.: Vulnerability of dynamic genetic conservation units of forest trees in Europe to climate change, Global Change Biol., 20, 1498–1511, https://doi.org/10.1111/gcb.12476, 2014.
Schüle, M., Domes, G., Schwanitz, C., and Heinken, T.: Early natural tree regeneration after wildfire in a Central European Scots pine forest: Forest management, fire severity and distance matters, Forest Ecol. Manag., 539, 120999, https://doi.org/10.1016/j.foreco.2023.120999, 2023.
Schütte, R.: Die Tucheler Haide vornehmlich in forstlicher Beziehung, Bertling, Danzig, 1893.
Seidl, R., Schelhaas, M.-J., Rammer, W., and Verkerk, P. J.: Increasing forest disturbances in Europe and their impact on carbon storage, Nat. Clim. Change, 4, 806, https://doi.org/10.1038/nclimate2318, 2014.
Seidl, R., Thom, D., Kautz, M., Martin-Benito, D., Peltoniemi, M., Vacchiano, G., Wild, J., Ascoli, D., Petr, M., Honkaniemi, J., Lexer, M. J., Trotsiuk, V., Mairota, P., Svoboda, M., Fabrika, M., Nagel, T. A., and Reyer, C. P. O.: Forest disturbances under climate change, Nat. Clim. Change, 7, 395, https://doi.org/10.1038/nclimate3303, 2017.
Shumilovskikh, L. S. and van Geel, B.: Non-Pollen Palynomorphs, in: Handbook for the Analysis of Micro-Particles in Archaeological Samples, edited by: Henry, E. G., Springer Cham, 65–94, 2020.
Shumilovskikh, L. S., Shumilovskikh, E. S., Schlütz, F., and van Geel, B.: NPP-ID: Non-Pollen Palynomorph Image Database as a research and educational platform, Veg. Hist. Archaeobot., 31, 323–328, https://doi.org/10.1007/s00334-021-00849-8, 2022.
Sillasoo, U., Väliranta, M., and Tuittila, E. S.: Fire history and vegetation recovery in two raised bogs at the Baltic Sea, J. Veg. Sci., 22, 1084–1093, 2011.
Simard, I., Morin, H., and Lavoie, C.: A millennial-scale reconstruction of spruce budworm abundance in Saguenay, Quéebec, Canada, Holocene, 16, 31–37, https://doi.org/10.1191/0959683606hl904rp, 2006.
Śliwa, E.: Gradacja strzygoni choinówki (Panolis flammea Schiff.) w Kampinowskim Parku Narodowym, Sylwan, 11, 61–66, 1974.
Śliwa, E.: Występowanie i zwalczanie ważniejszych folio fagów w drzewostanach sosnowych w latach 1946–1985, Sylwan, 13, 49–59, 1987.
Śliwa, E.: Przebieg masowego pojawu brudnicy mniszki (Lymantria monacha L.) i jej zwalczania w Polsce w latach 1978–1985 oraz regeneracja aparatu asymilacyjnego w uszkodzonych drzewostanach, Prace Instytutu Badawczego Leśnictwa, 710, 3–120, 1989.
Słowiński, M., Lamentowicz, M., Łuców, D., Barabach, J., Brykała, D., Tyszkowski, S., Pieńczewska, A., Śnieszko, Z., Dietze, E., Jażdżewski, K., Obremska, M., Ott, F., Brauer, A., and Marcisz, K.: Paleoecological and historical data as an important tool in ecosystem management, J. Environ. Manage., 236, 755–768, https://doi.org/10.1016/j.jenvman.2019.02.002, 2019.
Spiecker, H.: Growth of Norway Spruce (Picea abies [L.] Karst.) under Changing Environmental Conditions in Europe, in: Spruce Monocultures in Central Europe – Problems and Prospects, edited by: Klimo, E., Hager, H., and Kulhavý, J., European Forest Institute, Joensuu, 11–26, 2000.
Spinoni, J., Vogt, J. V., Naumann, G., Barbosa, P., and Dosio, A.: Will drought events become more frequent and severe in Europe?, Int. J. Climatol., 38, 1718–1736, https://doi.org/10.1002/joc.5291, 2018.
Stivrins, N., Liiv, M., Ozola, I., and Reitalu, T.: Carbon accumulation rate in a raised bog in Latvia, NE Europe, in relation to climate warming, Est. J. Earth Sci., 67, 247, https://doi.org/10.3176/earth.2018.20, 2018.
Stockmarr, J.: Tablets with spores used in absolute pollen analysis, Pollen et Spores, 13, 615–621, 1971.
Sullivan, J.: Pinus sylvestris, Fire Effects Information System,https://www.fs.usda.gov/database/feis/plants/tree/pinsyl/all.html (last access: 30 April 2024), 1993.
Sullivan, M. E. and Booth, R. K.: The Potential Influence of Short-term Environmental Variability on the Composition of Testate Amoeba Communities in Sphagnum Peatlands, Microb. Ecol., 62, 80–93, https://doi.org/10.1007/s00248-011-9875-y, 2011.
Szwankowski, J.: Powiat tucholski w latach 1875–1920, administracja, ludność, gospodarka, kultura, Logo, Tuchola, 2005.
Taeger, S., Zang, C., Liesebach, M., Schneck, V., and Menzel, A.: Impact of climate and drought events on the growth of Scots pine (Pinus sylvestris L.) provenances, Forest Ecol. Manag., 307, 30–42, https://doi.org/10.1016/j.foreco.2013.06.053, 2013.
Tinner, W. and Hu, F. S.: Size parameters, size-class distribution and area-number relationship of microscopic charcoal: relevance for fire reconstruction, Holocene, 13, 499–505, 2003.
Tipton, J.: Past anthropogenic impacts on peatland through the forest management practices in the Polish Tuchola Forest, Master thesis, Adam Mickiewicz University, Poznań, 2023.
Trouet, V. and Van Oldenborgh, G. J.: KNMI Climate Explorer: A Web-Based Research Tool for High-Resolution Paleoclimatology, Tree-Ring Res., 69, 3–13, https://doi.org/10.3959/1536-1098-69.1.3, 2013.
Trumbore, S., Brando, P., and Hartmann, H.: Forest health and global change, Science, 349, 814–818, https://doi.org/10.1126/science.aac6759, 2015.
Ulrich, B.: Production and consumption of Hydrogen ions in the ecosphere, in: Effects of acid precipitation on terrestrial ecosystems, edited by: Hutchinson, T. and Havas, M., Plenum Press, New York, London, 255–282, 1980.
Väliranta, M., Korhola, A., Seppä, H., Tuittila, E. S., Sarmaja-Korjonen, K., Laine, J., and Alm, J.: High-resolution reconstruction of wetness dynamics in a southern boreal raised bog, Finland, during the late Holocene: a quantitative approach, Holocene, 17, 1093–1107, https://doi.org/10.1177/0959683607082550, 2007.
van der Linden, M., Heijmans, M. M., and van Geel, B.: Carbon accumulation in peat deposits from northern Sweden to northern Germany during the last millennium, Holocene, 24, 1117–1125, https://doi.org/10.1177/0959683614538071, 2014.
van der Schrier, G., Allan, R. P., Ossó, A., Sousa, P. M., Van de Vyver, H., Van Schaeybroeck, B., Coscarelli, R., Pasqua, A. A., Petrucci, O., Curley, M., Mietus, M., Filipiak, J., Štěpánek, P., Zahradníček, P., Brázdil, R., Řezníčková, L., van den Besselaar, E. J. M., Trigo, R., and Aguilar, E.: The 1921 European drought: impacts, reconstruction and drivers, Clim. Past, 17, 2201–2221, https://doi.org/10.5194/cp-17-2201-2021, 2021.
Waller, M.: Drought, disease, defoliation and death: forest pathogens as agents of past vegetation change, J. Quaternary Sci., 28, 336–342, https://doi.org/10.1002/jqs.2631, 2013.
Wardenaar, E. C. P.: A new hand tool for cutting peat profiles, Can. J. Botany, 65, 1772–1773, https://doi.org/10.1139/b87-243, 1987.
Warner, B. G.: Palaeoecology of floating bogs and landscape change in the Great Lakes drainage basin of North America, in: Climate change and human impact on the landscape, edited by: Chambers, F. M., Chapman & Hall, 237–248, 1993.
Westerling, A. L.: Increasing western US forest wildfire activity: sensitivity to changes in the timing of spring, Philos. T. Roy. Soc. B, 371, 20150178, https://doi.org/10.1098/rstb.2015.0178, 2016.
Westman, C. J. and Laiho, R.: Nutrient dynamics of drained peatland forests, Biogeochemistry, 63, 269–298, https://doi.org/10.1023/A:1023348806857, 2003.
Whitlock, C. and Larsen, C.: Charcoal as a fire proxy., in: Tracking environmental change using lake sediments. Terrestrial, algal, and siliceous indicators, vol. 3, edited by: Smol, J. P., Birks, H. J. B., and Last, W. M., Kluwer, Dordrecht, 75–97, 2001.
Wilson, J. K.: The German Forest. Nature, Identity, and the Contestation of a National Symbol, 1871–1914, University of Toronto Press, 132–174, 2012.
Wilson, R. M., Hopple, A. M., Tfaily, M. M., Sebestyen, S. D., Schadt, C. W., Pfeifer-Meister, L., Medvedeff, C., McFarlane, K. J., Kostka, J. E., Kolton, M., Kolka, R. K., Kluber, L. A., Keller, J. K., Guilderson, T. P., Griffiths, N. A., Chanton, J. P., Bridgham, S. D., and Hanson, P. J.: Stability of peatland carbon to rising temperatures, Nat. Commun., 7, 13723, https://doi.org/10.1038/ncomms13723, 2016.
Woodward, C. and Haines, H. A.: Unprecedented long-distance transport of macroscopic charcoal from a large, intense forest fire in eastern Australia: Implications for fire history reconstruction, Holocene, 30, 947–952, https://doi.org/10.1177/0959683620908664, 2020.
Wotton, B. M., Nock, C. A., and Flannigan, M. D.: Forest fire occurrence and climate change in Canada, Int. J. Wildland Fire, 19, 253, https://doi.org/10.1071/WF09002, 2010.
Young, D. M., Baird, A. J., Charman, D. J., Evans, C. D., Gallego-Sala, A. V., Gill, P. J., Hughes, P. D. M., Morris, P. J., and Swindles, G. T.: Misinterpreting carbon accumulation rates in records from near-surface peat, Sci. Rep.-UK, 9, 17939, https://doi.org/10.1038/s41598-019-53879-8, 2019.
Yu, Z., Loisel, J., Brosseau, D. P., Beilman, D. W., and Hunt, S. J.: Global peatland dynamics since the Last Glacial Maximum, Geophys. Res. Lett., 37, L13402, https://doi.org/10.1029/2010GL043584, 2010.
Zajączkowski, J., Brzeziecki, B., and Kozak I.: Wpływ potencjalnych zmian klimatycznych na zdolność konkurencyjną głównych gatunków drzew w Polsce, Sylwan, 157, 253–261, 2013.
Zang, C. and Biondi, F.: treeclim: an R package for the numerical calibration of proxy-climate relationships, Ecography, 38, 431–436, https://doi.org/10.1111/ecog.01335, 2015.
Zielski, A.: Wpływ temperatury i opadów na szerokość słojów rocznych drewna u sosny zwyczajnej (Pinus sylvestris L. w rejonie Torunia), Sylwan, 2, 71–80, 1996.
Zielski, A. and Barankiewicz, A.: Dendrochronologiczna analiza przyrostów radialnych sosny zwyczajnej na terenie leśnictwa Dębie, Nadleśnictwa Włocławek, Sylwan, 2000, 69–74, 2000.
Zielski, A. and Krąpiec, M.: Dendrochronologia, PWN, 2004.
Zielski, A. and Sygit, W.: Wpływ klimatu na przyrost radialny sosny zwyczajnej (Pinus sylvestris L.), badania wzdłuż równoleżnika 52° N i transekcie Śląsk-Białowieża, in: Reakcja borów sosnowych na zmianę klimatu wzdłuż równoleżnika 52° N (12–32° E) oraz na zmiany w depozycji zanieczyszczeń chemicznych na transekcie Śląsk-Białowieża, edited by: Breymeyer, A. and Roo-Zielińska, E., Dokumentacja Geograficzna IG PAN Warszawa, 13, 161–185, 1998.
Zielski, A., Błaszkowski, A., and Barankiewicz, A.: Dynamika przyrostu radialnego sosny zwyczajnej (Pinus sylvestris L.) na obszarze leśnym eksploatowanym turystycznie nad jeziorem Wielkie Partęczyny (Nadleśnictwo Brodnica), Sylwan, 3, 69–78, 1998.
Zielski, A., Krąpiec, M., and Koprowski, M.: Dendrochronological data, in: The Polish Climate in the European Context: An Historical Overview, edited by: Przybylak, R., Majorowicz, J., Brázdil, R., and Kejna, M., 191–217, 2010.