the Creative Commons Attribution 4.0 License.
the Creative Commons Attribution 4.0 License.
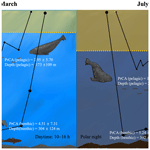
Seasonal foraging behavior of Weddell seals in relation to oceanographic environmental conditions in the Ross Sea, Antarctica
Hyunjae Chung
Jikang Park
Mijin Park
Yejin Kim
Unyoung Chun
Sukyoung Yun
Won Sang Lee
Hyun A. Choi
Ji Sung Na
Won Young Lee
Understanding the foraging behavior of marine animals in Antarctica is crucial for assessing their ecological significance and responses to environmental changes, such as seasonal changes in seawater or light hours. However, studying their responses to these seasonal changes remains challenging due to the difficult logistics of conducting observations, particularly during the harsh austral winter months. In this study, we investigated the influence of changes in seawater properties and light conditions on the seasonal foraging behavior of Weddell seals (Leptonychotes weddellii) in the Ross Sea, Antarctica. We affixed 64 Weddell seals with conductivity–temperature–depth tags for 3 consecutive years from 2021 to 2023 to record their locations and dive profiles, including depth, head acceleration, temperature, and salinity. We found that seals foraged more frequently in Modified Shelf Water and Ice Shelf Water than in Antarctic Surface Water. This preference could be connected to greater food availability. Seals also dove to greater depths and displayed increased activity in capturing prey during daylight hours. This behavior may correspond to the diel vertical migration of pelagic prey in response to varying light conditions. Consequently, marine fauna were confronted with distinct seasonal changes in the Antarctic environment and adjusted their foraging behaviors to respond to them. This highlights the importance of extrinsic factors in estimating their seasonal foraging behavior.
- Article
(5757 KB) - Full-text XML
-
Supplement
(1363 KB) - BibTeX
- EndNote
Marine animals must adapt to environmental changes in the Antarctic ecosystem, such as seawater and light availability fluctuations. Extrinsic factors play a vital role in their foraging success and food availability, specifically under challenging conditions such as oceanic warming, complex bathymetry, and changing sea ice cover (Speakman et al., 2020; Harcourt et al., 2021; Arce et al., 2022). Therefore, understanding how marine animals adapt to spatial and temporal shifts in oceanographic conditions is paramount. Antarctic animals are currently experiencing rapid environmental change (Schofield et al., 2010; Doney et al., 2011). Glacier melting and the associated oceanic changes pose significant challenges for these animals (Huang et al., 2011; Ainley et al., 2015; Sahade et al., 2015; Hückstädt et al., 2020). As top and mesopredators, marine animals serve as indicators for drastic changes. For example, Adélie penguins (Pygoscelis adeliae) explored a newly exposed sea after calving of ice shelf for potentially high prey availability (Park et al., 2021), while southern elephant seals (Mirounga leonine) and Weddell seals (Leptonychotes weddellii) were reported to shift their foraging locations and depths with the sea ice extent and oceanographic conditions (Bailleul et al., 2007; Labrousse et al., 2021).
The Ross Sea is the largest (2.09×106 km2) marine protected area (MPA) worldwide owing to its ecological significance (Brooks et al., 2021). It also stands as the largest continental shelf region in Antarctica. The Ross Sea has been preserved as a primary habitat for predatory animals, maintaining a pristine ecosystem because of its limited human accessibility (Smith et al., 2012). Notably, 40 % of Weddell seals, 38 % of Adélie penguins, and 26 % of emperor penguins (Aptenodytes forsteri) worldwide, along with a majority of South Polar skuas (Stercorarius maccormicki) in the Pacific sector, reside in the Ross Sea (LaRue et al., 2021; Smith et al., 2012). In the coastal polynyas of the Ross Sea, dense shelf water, a parent water mass of the Antarctic Bottom Water (AABW), is formed by strong polynyal activity (Rusciano et al., 2013; Yoon et al., 2020). This water mass contributes approximately a quarter to the total AABW production in Antarctica (Orsi et al., 1999; Orsi and Wiederwohl, 2009; Jendersie et al., 2018; Silvano et al., 2023). Hydrographic observations have been actively conducted in the Ross Sea since the 1950s, revealing changes in its marine environment due to recent climate shifts (Jacobs et al., 2002; Castagno et al., 2019; Silvano et al., 2020; Thomas et al., 2020; Yoon et al., 2020). According to these observations, hydrographic variations in the Ross Sea, including changes in the properties of shelf water, respond sensitively to air–sea interactions driven by katabatic winds and the advection of meltwater or sea ice from the Amundsen Sea (Rusciano et al., 2013; Castagno et al., 2019; Piñones et al., 2019; Silvano et al., 2020; Yoon et al., 2020). Climate-induced variations in the marine environment of the Ross Sea are anticipated to significantly impact the behavior of marine mammals. However, our understanding of their responses remains limited due to logistical and technological challenges.
Recent technological advancements employing miniaturized CTD (conductivity–temperature–depth) devices have enabled researchers to monitor seawater temperature and salinity (Kokubun et al., 2021; McMahon et al., 2021; Zheng et al., 2021). Deep-diving seals have mainly been used in oceanographic observation studies, with seal-tagging datasets shared among researchers, particularly within polar ocean studies (Treasure et al., 2017). In addition to physical oceanographic data, behavioral data, such as diving patterns and acceleration, serve as valuable indicators for estimating underwater foraging. Detailed feeding indices can be estimated from foraging diving depths and prey capture movements (Viviant et al., 2010; Volpov et al., 2015; Heerah et al., 2019; Nachtsheim et al., 2019; Photopoulou et al., 2020; Aubone et al., 2021).
Weddell seals are resident and primarily forage within the continental shelf of the Ross Sea (Harcourt et al., 2021; Goetz et al., 2023). Within this region, their primary diet consists of fish (notothenioids), supplemented by minor dietary components such as cephalopods and invertebrates (Dearborn, 1965; Plötz et al., 1991; Burns et al., 1998; Goetz et al., 2017). They are ranked as the deepest-diving phocid species except for the southern (Mirounga leonine) and northern elephant seals (Mirounga angustirostris). Hence, Weddell seals have been used to collect oceanographic and behavioral data at depths exceeding 600 m (Heerah et al., 2013; Zheng et al., 2021). These seals endure energetically demanding periods during the austral spring and autumn (October–February) seasons, marked by colony formation for pup birthing, rearing pups, breeding, and molting, often leading to considerable weight loss (Wheatley et al., 2006, 2008; Harcourt et al., 2007). Although both male and female Weddell seals sporadically forage during the reproductive season, they are classified as capital breeders that rely on energy reserves accumulated before breeding (Harcourt et al., 2007; Wheatley et al., 2008; Goetz et al., 2017). Consequently, the overwintering period (February–September) may be critical for seals to replenish their body mass and condition.
In this study, we aimed to examine the foraging behavior of Weddell seals in association with the seasonal changes during Antarctic summer to winter seasons (March to July) using acceleration-combined CTD data from seal-tagging observations in the Ross Sea. By categorizing different water masses, we examined seasonal preferences of the seals for specific water masses. In addition, we estimated foraging behavior in response to daylight conditions.
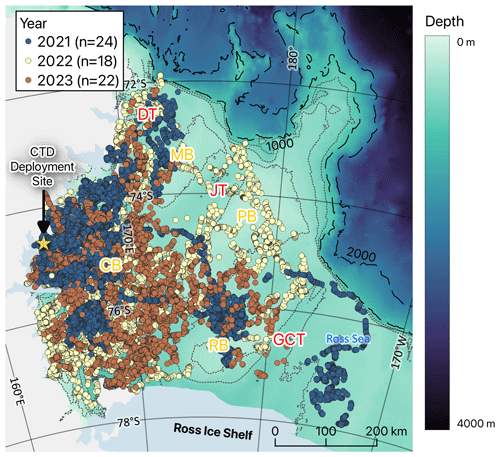
Figure 1Dive locations of seals tagged at Terra Nova Bay in the Ross Sea (blue, yellow, and brown dots indicate seal ARGOS locations in 2021, 2022, and 2023, respectively). The abbreviations CB, MB, PB, RB, DB, DT, JT, and GCT mean Crary Bank, Mawson Bank, Pannell Bank, Ross Bank, Drygalski Trough, Joides Trough, and Glomar Challenger Trough, respectively. The dashed line represents the shelf break (at depths of 1000 and 2000 m), while the dotted line represents bathymetry at 200 m intervals (200–800 m).
2.1 Study area and CTD deployment
We conducted seal tagging in January or early February of 2021, 2022, and 2023 along the shores of Jang Bogo Station (74°37′26′′ S, 164° 13′44′′ E) and Gondwana Station (74°38′7′′ S, 164°13′18′′ E) situated in Terra Nova Bay, Ross Sea, Antarctica (Fig. 1). We approached Weddell seals on the shore to deploy 57 CTD–satellite relay data loggers (CTD-SRDLs) or 7 CTD-SRDLs with GPS (weight: 545 g; size: mm; SMRU, UK). Among the 57 CTD-SRDLs affixed to individuals (19, 16, and 22 in 2021, 2022, and 2023, respectively), 55 were attached to their head, and 2 (ID 329 and 330, approached in 2021) were secured to their backs. Additionally, seven CTD-SRDLs (five in 2021 and two in 2022) with GPS technology were attached to their backs. Among the 64 seals, 27 were identified as females, and 35 were males based on their morphological feature. Two were not clearly distinguished in the field; hence, these were excluded from the model analysis for comparing the sexes (see Table S1 in the Supplement). These devices, with temperature, conductivity, and pressure sensors, collected hydrographic data from 48 individuals (17, 16, and 15 in 2021, 2022, and 2023, respectively), excluding 3 individuals in 2023 due to satellite communication issues and 7, 2, and 4 in 2021, 2022, and 2023, respectively, with data ending before March. According to the specifications of the sensors of CTD-SRDLs, the accuracy values of temperature, pressure, and conductivity are ±0.005°, 2 dBar, and ±0.01 mS cm−1, respectively (SMRU Instrumentation, 2024). However, low-resolution vertical profiles used in this study have a relatively low accuracy for temperature (±0.04°) and salinity (± 0.03 g kg−1) (Siegelman et al., 2019). All data obtained from CTD-SRDLs were received via Argos satellites, and no instruments were recovered. Detailed information on the tagged individuals is provided in Table S1.
Before deployment, we used an anesthetic (Zoletil® 50, Virbac Laboratoires, Carros, France; a combination of 125 mg tiletamine and 125 mg zolazepam in a 50 mL solution) administered through a blowpipe. Before anesthetization, the body size was roughly estimated by the field researchers. Following this, an appropriate dosage of anesthetic was administered using the proportional relationship between the body length and mass of the Weddell seals (Noren et al., 2008). The dosage administered to each individual (2 to 5 mL) is included in Table S1. We note that two individuals (ID 329 and 330) in February 2021 were approached with a canvas bag (McMahon et al., 2000) and that no anesthesia was treated in those cases. Following the injection, we waited for over 10 min until the seals were sufficiently sedated. Once the seals exhibited no response to the researcher's approach, we proceeded to affix a CTD device to the seal's head using Loctite glue (Loctite 401 was used in 2021, Loctite 422 in 2022 and 2023) or Araldite epoxy resin (Araldite® 2012 adhesive).
Prey capture attempts were estimated from the transmitted head acceleration data obtained from the accelerometer embedded in the CTD tags (referred to as “accelerometer processing”, as detailed in the SMRU Instrumentation manual 2023). The accelerometer mounted on this tag was initially configured to measure the three-axis acceleration at 25 Hz. However, due to network bandwidth limitations, summarized information was transmitted in lieu of complete acceleration data. To summarize prey capture behavior, the total jerk (m s−3), the time derivative of acceleration, was calculated using the method outlined by Ydesen et al. (2014). For each second, the tag compared the maximum value of the root-mean-square (rms) jerk to a threshold of 250 m s−3 to ascertain the occurrence of a prey capture attempt (PrCA) within that specific second. If the rms jerk exceeded the threshold for several consecutive seconds, it was considered a single PrCA event. Due to bandwidth limitations, summarized information was transmitted by dividing dives into three phases (descent, bottom, and ascent) and indicating the phase in which PrCAs occurred, instead of transmitting the exact time and depth. Each dive was fitted to 12 broken-stick points (i.e., the depth at the first point below the dive threshold (6 m), 10 internal points, and a final point before the dive threshold (6 m)). Dive descents were defined as the start of the dive until the first internal point that exceeded 75 % of the maximum dive depth. Similarly, the ascent phase began at the first internal point, where depths exceeded 75 % of the maximum dive depth, and ended after the dive. The tags computed the number of PrCA events for each phase and subsequently transmitted through a satellite network system.
2.2 Hydrological data
2.2.1 Quality control for hydrographic data
Temperature and salinity profiles obtained from seal-tagging observations were quality-controlled by standard procedures widely used for the low-resolution ascent profiles of instrumented seals (Fig. S1a in the Supplement; Boehme et al., 2009; Roquet et al., 2011; Siegelman et al., 2019). The procedure comprises three steps: tag-by-tag visualization, pressure effect correction, and delayed-mode calibration.
In step 1, we checked reasonable ranges of temperature and salinity in Terra Nova Bay, Ross Sea, using historical (2014–2018) ship-based CTD data and ocean moorings (sourced from Yoon et al., 2020) and removed outliers from 2021, 2022, and 2023 seal-tagging data (Fig. S1a). Subsequently, we applied the density removal algorithm regarding the minimum N2 (N is the Brunt–Väisälä frequency) threshold as s−2. Vertical profiles of N2 show that the density removal algorithm was successfully applied to the 3 years of seal data (Fig. S1b, c, and d). We found 500, 1630, and 3333 irregular profiles out of the 3315, 7552, and 7654 seal-tagging profiles recorded in 2021, 2022, and 2023 respectively, through step 1. Therefore, we used 2815, 5922, and 4321 profiles from step 2.
In step 2, we corrected the pressure effect for the temperature and salinity profiles using at-sea experimental data (Fig. S2a; Roquet et al., 2011). The in situ calibration constituted a ship-based calibration cast for CTD-SRDL sensors, attaching CTD-SRDL sensors to the CTD frame of the ship. Using at-sea experimental data, we derived linear relationships between temperature and salinity differences for each CTD-SRDL sensor and ship-based CTD data according to pressure (Roquet et al., 2011). Temperature and salinity biases were subsequently removed from the entire profile of each tag according to the pressure calculated from each relationship (Roquet et al., 2011). The calibration cast was conducted only before the 2022 deployment; therefore, step 2 was conducted only for the 2022 seal-tagging data.
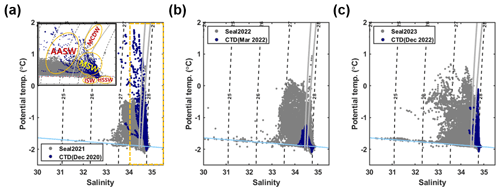
Figure 2(a) A θ–S diagram for seal-tagging data obtained during 2021 (gray) and ship-based CTD data recorded from 6 to 25 December 2020 (blue). The dashed black lines indicate isopycnals (kg m−3), while solid gray lines represent 28 and 28.27 kg m−3 neutral density surfaces. The solid sky-blue line indicates the surface freezing point depending on the salinity. The inset indicates a zoomed-in plot for the θ–S diagram (dashed yellow box) and shows the approximate temperature and salinity range of each water mass. The abbreviations AASW, MCDW, MSW, ISW, and HSSW correspond to Antarctic Surface Water, Modified Circumpolar Deep Water, Ice Shelf Water, and High-Salinity Shelf Water, respectively. (b) A θ–S diagram for seal-tagging data obtained during 2022 and ship-based CTD data recorded from 15 to 19 March 2022 and (c) for seal-tagging data obtained during 2023 and ship-based CTD data recorded from 3 to 17 December 2022.
Finally, in step 3, we implemented a delayed-mode calibration approach to correct the offsets in the temperature and salinity profiles. Here, we used the High-Salinity Shelf Water (HSSW) method (Fig. S1), as an alternative to the Lower Circumpolar Deep Water (LCDW) method generally used for correcting seal data in the Southern Ocean (Roquet et al., 2011) because LCDW is rarely found in the continental shelf region of the Ross Sea (Budillon et al., 2011). HSSW, characterized by a homogeneous layer (Yoon et al., 2020), offers a highly stable absolute reference for estimating offsets of seal-tagging data in Terra Nova Bay (TNB). Approximately 1 month after the 2021, 2022, and 2023 deployments, we conducted full-depth CTD casts at 56, 43, and 69 stations within Terra Nova Bay, Ross Sea, from 6 to 25 December 2020, 15–19 March 2022, and 3–17 December 2023, respectively, aboard the ice-breaking research vessel ARAON (Figs. 2 and S2). Absolute values from ship-based CTD can be regarded as actual values because all CTD sensors of RV ARAON were sent to Sea-Bird Electronics (SBE; manufacturer) for sensor calibration 1 year before the observation period. We adjusted offsets of the seal-tagging data by comparing the salinity and temperature of HSSW within the TNB observed from ship-based CTD profiles with those from seal-tagging profiles. Potential density over 28 kg m−3 and potential temperature below −1.9° were used as criteria for HSSW (Yoon et al., 2020).
The salinity offset range for the 2021 seal data was from −0.16 to −0.03, and the temperature was not adjusted because the temperature of HSSW from the 2021 seal data was consistent with those from the ship-based CTD data. Temperature and salinity offsets for 2022 seal data were estimated as −0.03–0.23° and −0.38–0.01, respectively. Temperature and salinity offsets for 2023 seal data were estimated as −0.01–0.27° and −0.41–0.01, respectively. As depicted in Figs. 2 and S2, quality-controlled seal data show consistent features from ship-based CTD data in Terra Nova Bay, Ross Sea.
Furthermore, we classified these water masses based on potential temperature and potential density to investigate the spatial and temporal variations in water masses within the continental shelf region of the Ross Sea (Yoon et al., 2020). Potential temperature and potential density criteria for HSSW are defined as below −1.9° and over 28 kg m−3, respectively. Potential temperature and potential density for Ice Shelf Water (ISW) are defined as below −1.9° and below 28 kg m−3, respectively. Modified Shelf Water (MSW) is defined as colder (warmer) than −0.5° (−1.9°) and denser than 27.74 kg m−3. For Modified Circumpolar Deep Water (MCDW), the potential temperature is over −0.5°, while the potential density ranges between 27.74–27.88 kg m−3. Antarctic Surface Water (AASW) is defined by temperatures colder than −0.5° and densities lighter than 27.74 kg m−3 (Fig. 2a).
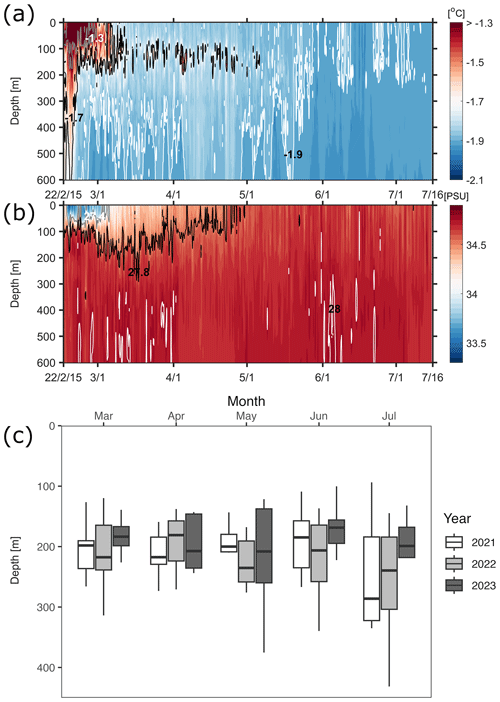
Figure 3Temporal variation in dive depths of Weddell seals for 2021, 2022, and 2023 with a Hovmöller diagram of seawater properties around Terra Nova Bay in 2022. (a) Hovmöller diagram of potential temperature around Terra Nova Bay. Solid gray, black, and white lines represent −1.3, −1.7, and −1.9° isotherms, respectively. (b) Hovmöller diagram of salinity around Terra Nova Bay. Solid gray, black, and white lines represent 27.4, 27.8, and 28 kg m−3 isopycnals (σθ), respectively. (c) White, gray, and black boxes indicate diving behaviors in 2021, 2022, and 2023, respectively, showing a tendency for deeper dives as austral winter approaches.
2.2.2 Kriging
A total of 13 058 profiles were observed (2815, 5922, and 4321 in 2021, 2022, and 2023, respectively) and filtered through quality control procedures. To investigate the relationship between foraging behavior and the oceanographic environment, we calculated the physical characteristics of the water column at the maximum depth of each dive. We employed the kriging method, a commonly used technique for interpolating autocorrelated data, to calculate salinity and temperature because the oceanographic and behavioral data obtained from the CTDs did not temporally match (Oliver and Webster, 1990). Kriging was performed using the gstat package (Pebesma, 2004) in R, and the salinity and temperature at the maximum depth of each dive were obtained by calculating the two-dimensional space of depth and time. Water masses were classified based on these values. To account for the spatiotemporal anisotropy, we scaled the values between 0 and 1 based on the maximum and minimum values and multiplied the time values by 50. Separate kriging processes were conducted for the 2021, 2022, and 2023 datasets, and the reliability of the results was confirmed via 5-fold cross-validation. The mean, root-mean-square error, and mean absolute error for the kriging estimates are summarized in Table S2. To create the Hovmöller diagram (Fig. 3a and b), salinity and temperature from 1 to 600 m depth between 15 February and 15 July 2022 were also calculated using kriging with seal-tagging profiles around Terra Nova Bay within the longitude range between 160 and 170° E and latitude range between 76 and 74° S.
2.3 Dive data classification and filtration
We distinguished between benthic and pelagic seal dives. The bathymetric depth corresponding to each dive location was assigned using bathymetry data from IBCSO (http://IBCSO.org, last access: 20 May 2022, Dorschel et al., 2022). Dives characterized by a submergence depth of 80 % or more of the assigned depth were classified as benthic dives (Kokubun et al., 2021). The Python package pvlib (Holmgren et al., 2018) determined the solar altitude at each dive location and time, with altitudes above 0 categorized as daytime and below 0 as nighttime. Dives with bathymetric values greater than 0 were excluded to eliminate inaccurately recorded dives. When seal diving was deeper than the bathymetric values, the dives were regarded as benthic dives. Furthermore, dives with durations that were too short or long and had depths that were too great (dive duration 0 s; dive duration >5760 s; dive depth >906 m; Heerah et al., 2013) and those characterized by vertical travel speeds exceeding 5.1 m s−1 were excluded (Davis et al., 2003).
2.4 Statistics
To investigate the factors influencing the feeding behavior of Weddell seals, we set the response variable as log-transformed prey capture attempts (log(PCA_BTM +1)) and used dive type (benthic or pelagic), season (month), sex, water mass, and year as explanatory variables to determine the minimal model through backward elimination. For the analysis, we excluded the data obtained from seals with CTD devices attached to their backs to catch the head movements. First, we compared the full model containing all explanatory variables against the models with each variable systematically removed using a likelihood test; through this process, we eliminated variables deemed non-contributory. After repeating this process, we obtained a parsimonious model containing only the important variables. Additionally, we compared all possible models created using different combinations of explanatory variables by comparing their Akaike information criterion (AIC) and Bayesian information criterion (BIC) values. We subsequently obtained the best model with the smallest AIC and BIC values (Tables S3 and S4). The explanatory variables of the best model obtained using the three methods (backward elimination, AIC, and BIC) were consistent. After finding the minimal model, we conducted post hoc tests using the multcomp R package (Hothorn et al., 2008) to investigate differences in the categorical variables included in the minimal model (season and water mass). Additionally, after confirming the seasonal change in PrCAs, we aimed to investigate whether the seasonal change differed by dive type. To do this, we included an interaction term between Julian date (day of the year) and dive type, with sex, water mass, and year as candidate explanatory variables, and identified the best model. To examine diurnal patterns, we subsequently examined the effect of time periods on the dive depth, number of dives, and prey capture attempts. Throughout this process, we created a linear mixed-effects model using the nlme R package (Pinheiro et al., 2022), in which we set each identity as a random effect and included a temporal autocorrelation term. The models were estimated using restricted maximum likelihood. To ensure the robustness of our models, we performed Monte Carlo cross-validation (CV) with a 4:1 train–test split and 100 iterations for each model. This approach allowed us to assess the stability and generalizability of the models. The standard deviations of the R2 were all below 0.02, further confirming the consistency and reliability of our models.
The telemetry data revealed that the Weddell seals in this study dispersed from the tagged region (near Jang Bogo Station; 62.2° S, 58.8° W) and traveled throughout continental shelf regions in the Ross Sea (Fig. 1). Among the 64 014 dives observed, 11 741 were categorized as benthic dives, while 52 273 were pelagic.
Seal CTD sensors have been used to observe five water masses in the continental shelf region of the Ross Sea: AASW, MCDW, MSW, ISW, and HSSW (Fig. 2; Orsi and Wiederwohl, 2009). When compared to the ship-based CTD data collected in the TNB during the austral summer of the same year, the seal-tagging data showed a wider range of temperature and salinity of AASW (Fig. 2). The wide range of temperature and salinity values of the AASW represents its seasonal variation, being icy cold and fresh during the sea ice melting period (mainly austral summer) and subsequently transitioning to being warm and saline due to latent heat release and brine rejection during the sea ice formation period (mainly austral winter). The 27.8 kg m−3 isopycnal exhibited a shoaling trend from mid-March onwards, eventually disappearing via salinity increase in the surface due to brine rejection and vigorous mixing through the whole water column by May (Fig. 3). After May, HSSW and MSW, which are colder than −1.7° and denser than 27.8 kg m−3, were mainly identified in the TNB (Fig. 3). These results support the notion that our seal-tagging data captured the increase in the density of AASW over the period between austral summer and winter. The dive depth shows an increasing trend from March to July as the water temperature decreased while salinity and density increased (Fig. 3c).
Moreover, the presence of MCDW was more discernable in the seal-tagging profiles compared to the ship-based CTD data obtained from the TNB, despite its limited occurrence (only 125 depths of 13 058 profiles) (Figs. 1, 2, and S3). This prominence arises because of seals diving into the Drygalski and Joides troughs near the continental shelf break region (Fig. 1). Among seal data, more profiles were obtained near the shelf break and the eastern part of continental shelf regions in 2021 and 2022 than those in 2023 (Fig. 1). Due to this difference in spatial sampling, MCDW was identified more clearly in 2021 and 2022 compared to 2023 (Figs. 2 and S3). Furthermore, the ISW observed across the continental shelf region of the Ross Sea demonstrates a wider salinity range than the ISW observed in the TNB (Fig. 2), consistent with previous studies (e.g., Budillon et al., 2011). In 2021, 2022, and 2023, properties of HSSW were well detected (Fig. 2), and it was mainly observed in the western part of the continental shelf region of the Ross Sea where polynyas exist (Figs. 2 and S3).
Table 1The best model for dive depth AIC, BIC, and backward elimination approaches revealed that sex, season (month), and year are important variables for predicting dive depth. Statistical significance value (p values lower than 0.05) is highlighted in bold.
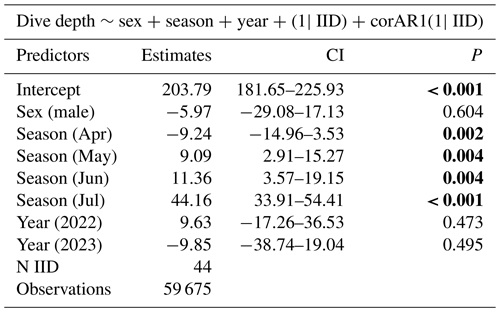
Table 2Post hoc (Tukey honestly significant difference, HSD) test for the “season” variable included in the best model for dive depth. Statistical significance (p values lower than 0.05) is highlighted in bold.
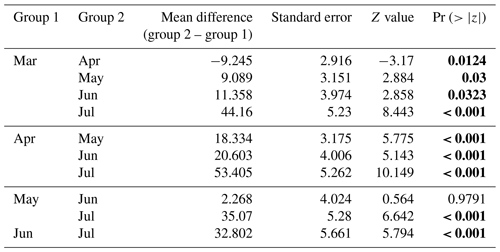
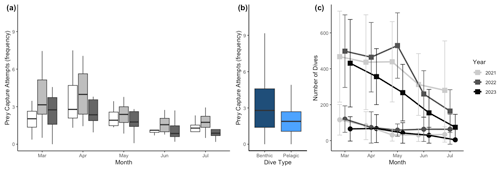
Figure 4Prey capture attempts (PrCAs) among (a) seasons (month) and (b) dive types (benthic or pelagic) and seasonal change in the dive frequency. Prey capture attempts were highest in April and lowest in June. Prey capture attempts were higher in benthic dives compared to pelagic dives. The dark blue box indicates the number of PrCA events per dive during benthic dives, whereas the lighter blue box represents the same statistic during pelagic dives. In panel (c), curves with square markers represent the total dives, while curves with circle markers represent the number of benthic dives of each month. The error bars represent the mean ± standard deviation.
In all 3 years, the Weddell seals tagged in this study exhibited distinct diving behaviors across months. Figure 3 illustrates the seasonal changes in dive depth. The dive depth shows an increasing trend from March to July, whereas the number of PrCA events decreases in June and July compared to March and April. When considering diving depth (p<0.001; log-likelihood ratio test between the best model and a model excluding the variable “season”), the shallowest dives were undertaken in April, whereas the deepest diving occurred in July (200±137 m in April, 265±154 m in July; mean ± standard deviation) (Fig. 3; Tables 1 and 2). In terms of PrCA events (p<0.001; log-likelihood ratio test between the best model and a model excluding the variable “season”), the highest number was observed in April, whereas the lowest occurred in June (3.29±6.11 in April, 1.56±2.59 in June) (Fig. 4a; Tables 3 and 4). Additionally, PrCA values varied based on water mass and dive type (benthic or pelagic) (p<0.001 for both; log-likelihood ratio test between the best model and a model excluding the variables “water mass” and “dive type”). Based on our water mass definition, Weddell seals performed many dives (76.76 % of total dives), and there was a high frequency of observations of PrCAs (86.7 % of total PrCA events) in MSW. The kernel density plots of dive distributions on a temperature and salinity diagram are shown in Fig. S4. Notably, Weddell seals displayed a higher number of PrCA events per dive in HSSW, MSW, and ISW compared to AASW (additional 1.14, 0.66, and 0.65 in PrCAs per dive for HSSW, MSW and ISW, respectively; Tables 3 and 5). Our seals had 0.58 more PrCAs during benthic dives than during pelagic dives (Fig. 4b; Table 2), despite the fact that benthic dives were not predominant (11 741 out of a total of 64 014 dives; Fig. 4c). From March to July, PrCAs consistently decreased during pelagic dives, whereas no significant decrease was observed during benthic dives (Fig. 5; Table S5).
Table 3The best model for prey capture attempts using AIC, BIC, and backward elimination approaches revealed that water mass type, season (month), and dive type (benthic or pelagic) are important variables for predicting prey capture attempts. Statistical significance (p values lower than 0.05) is highlighted in bold.
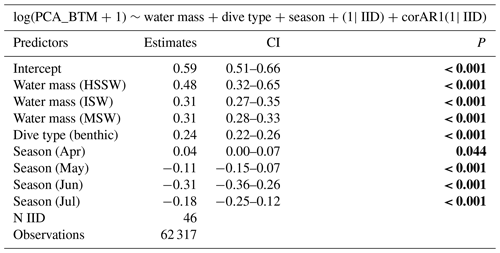
Table 4Post hoc (Tukey HSD) test for the “season” variable included in the best model for prey capture attempts. Statistic significance (p values lower than 0.05) is highlighted in bold.
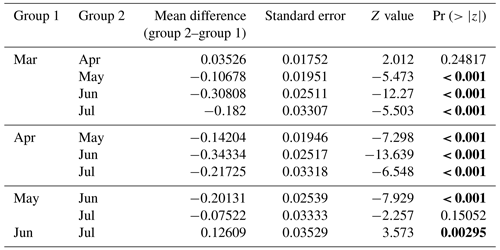
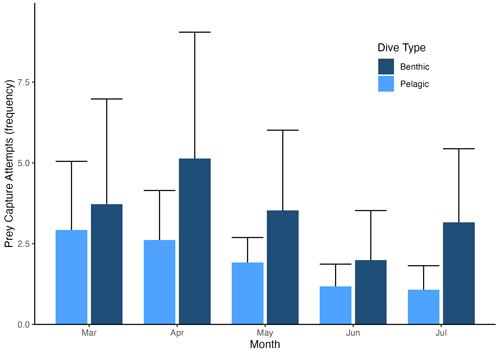
Figure 5Seasonal change in prey capture attempts (PrCAs) per dive by dive type (benthic or pelagic dive). PrCA values were consistently higher during benthic dives compared to pelagic dives. While PrCAs during pelagic dives decreased as winter approached, they decreased less significantly during benthic dives. PrCAs during benthic dives are shown in dark blue, while PrCAs during pelagic dives are represented in light blue.
Weddell seals demonstrated different diving behaviors between daytime and nighttime, delineated by solar altitude. During daylight hours, seals dove an average of 76.4 m deeper and had a higher proportion of benthic dives compared to nighttime (Figs. 6a and d, Table 6). Additionally, seals demonstrated higher PrCA events during the daytime, with an average of 4.89 foraging attempts per dive, compared to 2.13 attempts during the nighttime (Fig. 6b; Table 6). Interestingly, no discernible difference was observed in the number of dives between the day and night (Fig. 6c; Table 6).
In this study, we observed a distinct seasonal pattern and water mass preference in the foraging behavior of Weddell seals. Shallow and deeper diving was observed in April and July, respectively, and foraging frequencies were the highest in April and lowest in June. The detected water masses from the seal CTD were MCDW, MSW, ISW, AASW, and HSSW (Figs. 2 and S3). Among these, Weddell seals exhibited a significantly higher number of PrCA events per dive for HSSW, MSW, and ISW over AASW. In contrast, MCDW was rarely detected. Furthermore, more PrCA events were observed during benthic dives than those in pelagic dives. Finally, a diel diving pattern among the seals was observed, with an increase in the proportion of benthic dives, foraging frequency, diving depths, and the number of dives during the day compared to night.
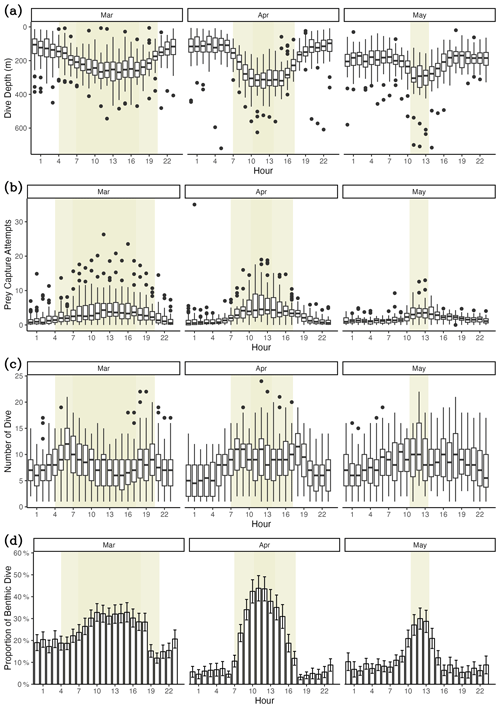
Figure 6Diel variation in diving behaviors. (a) Dive depth, (b) prey capture attempts, (c) number of dives, (d) proportion of benthic dives. The yellow-shaded area denotes the duration of sunlight exposure during the day. The lighter yellow shaded area indicates the period of daylight at the beginning of the month. In comparison, the darker yellow shaded area represents the daylight period at the end of the month.
Table 5Post hoc (Tukey HSD) test for the “water mass” variable included in the best model for prey capture attempts. Statistical significance (p values lower than 0.05) is highlighted in bold.
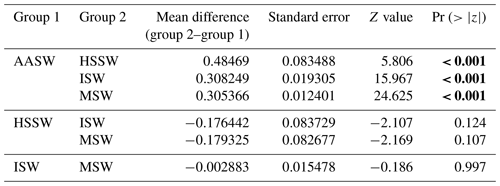
Table 6Regression analyses of dive parameters (dive depths, prey capture attempts per dive, number of dives per day) concerning the presence of sunlight (day or night). Statistical significance (p values lower than 0.05) is highlighted in bold.
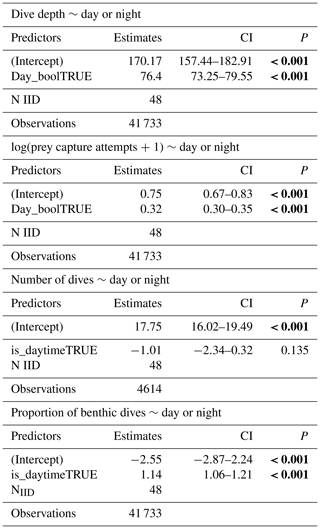
To the best of our knowledge, this is the first study to measure the prey capture attempts of Weddell seals in the winter season directly using head acceleration with CTD. Previous studies have estimated foraging behaviors from indirect information, including horizontal location, vertical swim speed, dive time, and dive depth, rather than being directly measured (Nachtsheim et al., 2019; Kokubun et al., 2021; Goetz et al., 2023). While these proxies are indirect indices and should be interpreted cautiously, acceleration data like the data our CTD obtained are particularly beneficial as they can directly detect PrCAs, providing a more accurate measure of foraging activity (Heerah et al., 2019; Allegue et al., 2023). This allows us to correlate foraging activities with the recorded environmental conditions, providing a clearer understanding of how these animals interact with their habitats. We presume that the combination of CTD and acceleration data offers a comprehensive view of both the physical environment and the behavioral responses of the seals, leading to more accurate and insightful conclusions.
Our results conclusively illustrate a seasonal shift in diving depth and the number of PrCAs per dive. This phenomenon can be attributed to fluctuations in oceanographic and light conditions. Notably, Weddell seals preferred MSW or ISW over AASW during their foraging dives. As the lower boundary of the AASW shifted downward during June and July, the seals engaged in progressively deeper dives during the winter months, possibly to follow the MSW or ISW. Secondly, a seasonal decrease in sunlight could limit prey accessibility, particularly pelagic fish species. The number of daylight hours in this region significantly decreased from March to July. On 1 March, daylight duration is over 16 h with a meridian altitude of over 23° (based on data at Jang Bogo Station), but the onset of the polar night in early May (5 May 2021; 6 May 2022; 6 May 2023) resulted in continuous darkness without sunrise. In the Ross Sea, the euphotic zone, where sufficient light for photosynthesis is available, is situated at a depth of 34±13 m in spring, 26±9 m in summer (mean ± standard deviation), and within a range of 14–66 m (range) in winter (Fabiano et al., 1993; Smith et al., 2013). Below the euphotic zone lies the dysphotic zone, where light is present; however, it is insufficient for photosynthesis to occur. Based on the findings of Sipler and Connelly (2015), the dysphotic zone in the Ross Sea extends to a depth of 170 m. Notably, Antarctic silverfish and holopelagic prey in the Ross Sea are found at depths of 0–700 m (De Witt et al., 1990), and their prey abundance is high in the upper water layers (50–200 m, Mintenbeck, 2008). This implies that Antarctic silverfish may inhabit the euphotic and/or dysphotic zones. Weddell seals have been reported to use light and other senses, including vibrissal sensations, for swimming and detecting and catching prey (Wartzok et al., 1992; Davis et al., 2004). Therefore, when sunlight is available, Weddell seals employ a combination of visual and other sensory inputs to capture pelagic or cryopelagic prey. Conversely, when sunlight is unavailable or benthic prey are the target, they must rely solely on non-visual sensory inputs for effective foraging. The diminished light conditions experienced in June and July posed challenges for seals in locating prey, thereby leading to a decrease in PrCA events per dive and an increase in diving depths during these months compared to March. Our data also showed that during the polar night in June and July, the PrCA per dive decreased in pelagic dives, while benthic dives showed no notable change (Fig. 5, Table S5). This suggests that benthic dives may play a crucial role in Weddell seals' foraging strategy during the winter months, when light conditions are diminished, making benthic prey potentially more reliable than pelagic prey.
The seasonal changes in diving behavior likely reflect corresponding seasonal changes in the distribution or composition of prey. Previous studies analyzing the diet of Weddell seals in the Ross Sea through scat or stomach contents have highlighted Antarctic silverfish as the primary pelagic prey consumed by Weddell seals across all seasons (Dearborn et al., 1965; Plötz et al., 1991; Burns et al., 1998; Goetz et al., 2017). Therefore, the increased dive depth of Weddell seals may suggest that the distribution of Antarctic silverfish, their main prey and the only holopelagic fish in the Ross Sea, shifts deeper as winter approaches. Although the seasonal variations in the vertical distribution of Antarctic silverfish remain unknown, Antarctic krill (Euphausia superba), one of their primary food sources, may migrate to deeper waters during winter when the sea surface is covered with ice and food in the upper waters becomes scarce (Schmidt et al., 2011; Meyer et al., 2017). This could imply that Antarctic silverfish may migrate to deeper waters as winter approaches. As Antarctic silverfish mature, they tend to inhabit deeper waters (La Mesa and Eastman, 2012), suggesting a shift in the prey composition towards larger and deeper-dwelling adult Antarctic silverfish as winter approaches. Another plausible factor behind this seasonal shift in diving behavior could be a corresponding shift in dietary preferences, involving greater consumption of benthic fish than pelagic or cryopelagic fish. Additionally, seasonal variations in interspecific competition, particularly involving emperor penguins, another apex predator species with a year-round presence in the Ross Sea (Burns and Kooyman, 2001; Smith et al., 2012), could affect the foraging behavior of Weddell seals. In winter, emperor penguins must actively seek sustenance to nurture their offspring, potentially intensifying interspecific competition with Weddell seals (Burns and Kooyman, 2001). Given that the diving capacity of emperor penguins is lower than that of adult Weddell seals (Kooyman et al., 1980; Kooyman and Kooyman, 1995; Burns, 1999), Weddell seals may forage at greater depths to minimize interspecific competition. Notably, in our data, deep dives (exceeding 350 m) occurred at a rate of 22.4 % in July for Weddell seals, whereas emperor penguins performed deep dives at a rate of less than 10 % (Burns and Kooyman, 2001). This suggests a potential seasonal adjustment in foraging strategy, although direct evidence for this behavior remains limited.
A previous study on Weddell seals in the Ross Sea showed that seasonal changes for foraging effort were observed, with dive depth and prey search effort (estimated by search effort time in a given space) increasing from summer to winter (Goetz et al., 2023). Additionally, prey search effort was also higher in benthic dives (Goetz et al., 2023). The seasonal increase in dive depth agreed with our findings, but the prey search effort showed the opposite of PrCAs, our foraging measurement. Goetz et al. (2023) observed that the prey search effort was the highest in winter of 2010 to 2012. This could be due to the different seasonal prey availability across the seasons. During winter, our study indicated that prey capture was lower, while the previous study by Goetz et al. (2023) showed the prey search effort was higher. To combine the two studies, it seems that the seals had to spend more time to search for prey despite the low foraging success in winter. Still, it is difficult to compare the two studies since there is an approximate 10-year difference. The diet composition of Weddell seals exhibits considerable interannual variability in the Ross Sea area (Goetz et al., 2017). The sea ice extent and the food availability for top predators can vary annually (Ainley et al., 2020). Such variations in sea ice extent can possibly influence plankton blooms and seasonal prey abundance for seals between the two studies (Arrigo et al., 2004; Lorrain et al., 2009). Our measurement also has a limitation when comparing the seasonal change. Prey capture attempts were estimated by “jerk” from acceleration sensors attached on heads. Prey capture attempts do not necessarily correlate with the quality or quantity of the prey successfully obtained. For example, jerk could be overestimated when handling larger prey items, as the number of handling movements increases (a case of Australian fur seals, Arctocephalus pusillus doriferus; Volpov et al., 2015). These limitations of foraging proxies may account for the observed differences in their seasonal trends.
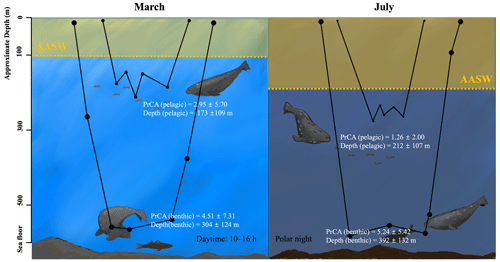
Figure 7Schematic summary of seasonal variation in oceanographic conditions and foraging behaviors. The area shaded in yellow represents the AASW, and the dashed line indicates the lower boundaries. In March, the AASW is positioned at shallower depths, whereas in July, the AASW shifts to deeper locations. AASW is a water mass that is less preferred by Weddell seals, possibly due to reduced prey availability, which appears to result in deeper dive depths during pelagic dives for Weddell seals. The black line graph in the figure represents typical examples of benthic and pelagic dives in March and July. The sizes of the dots are proportional to the PrCA values. PrCA and depth values are presented as mean ± standard deviation. Note that the high variability of PrCA events results in SD values being larger than the mean values.
Our seal CTD data revealed a dynamic change in vertical stratification by season. During early austral fall, the water columns within the Ross Sea are structured with HSSW, MSW, and AASW from the bottom up; however, this stratification weakens as winter advances. Strong mixing owing to the influence of winds coupled with active sea ice formation at the surface diminishes the stratification over the whole water column (Fig. 3b and c). Additionally, ISW exists near the ice shelves instead of spreading out to the central part of the continental shelf region (Fig. S3). This behavior might be associated with the relatively low rates of basal melt and meltwater flux of ice shelves in the Ross Sea (Rignot et al., 2013, 2019).
Weddell seals exhibited more frequent feeding behavior in HSSW, MSW, and ISW than in AASW, and they rarely ventured into MCDW. These findings might reflect the inherent nutrient composition of each water mass. HSSW is the densest water mass (potential density >28 kg m−3) in the Ross Sea (Budillon et al., 2011; Yoon et al., 2020), and the biological products from the surface are being transported to depth, accumulating nutrient contents of HSSW (Arrigo et al., 2008; DeJong et al., 2017; Ingrosso et al., 2022). It provides unique habitats for benthic fish for survival and distribution (La Mesa et al., 2004). In our results, dives in HSSW were mostly performed in the benthic areas (69.4 %). Thus, HSSW may contain prey, usually benthic species, for seals.
MSW is formed by mixing shelf water with surrounding water masses, including MCDW, within the continental shelf region. MCDW is crucial in heat and nutrient cycling in the Southern Ocean because it is warm and nutrient-rich (Smith et al., 2012; Kustka et al., 2015; Gerringa et al., 2020). MCDW contains a significantly higher concentration of macro-nutrients and contributes to the basal melt of ice shelves, which may increase primary production. However, the dissolved oxygen (DO) levels in MCDW are notably low, falling below 5 mL L−1 (Jenkins et al., 2018; Yoon et al., 2020). This is lower than the critical threshold of oxygen concentration for krill, implying that the prey availability for seals in MCDW would be limited (Brierley and Cox, 2010). However, MSW may contain high amounts of nutrients from MCDW and sufficient oxygen contents (Orsi and Wiederwohl, 2009; Smith et al., 2014). According to ship-based CTD observations with SBE43 DO sensor values in TNB during the austral summer 2021, 2022, and 2023, it was found that the DO of MSW was over 6.5 mL L−1.
ISW is a water mass formed by melting ice shelves and is characterized by a potential temperature below the freezing point. This water mass can harbor essential nutrients, such as iron, which may be present on ice shelves. This potentially makes ISW a nutrient source (Sedwick and DiTullio, 1997; Smith et al., 2014). Nutrient-rich hydrographic conditions may be related to the high prey availability. Weddell seals exhibit increased foraging behavior under nutrient-rich conditions in other regions (Heerah et al., 2013; Nachtsheim et al., 2019; Kokubun et al., 2021). Moreover, ISW also has relatively high oxygen; for example, DO sensor values of ISW in TNB during the austral summer of 2021 and 2022 are higher than 6.4 mL L−1. AASW is generally deficient in nutrients due to vigorous biological processes despite the high DO. Therefore, Weddell seals could have higher PrCA events in MSW and ISW than AASW and MCDW because they are rich in nutrients and have high DO.
Although only 18.8 % of all dives were categorized as benthic dives, more foraging attempts were observed during these dives. From an energy efficiency perspective, the costs associated with the diving behavior of Weddell seals increase as the duration of their dives increases. In particular, dives lasting longer than 23 min entail additional anaerobic costs. Despite the substantial energetic costs associated with prolonged dives, the benthic zone is a habitat for numerous sizable prey species weighing over 1 kg, including the Antarctic toothfish and icefish (La Mesa, 2004; Goetz et al., 2017). Hence, Weddell seals can reap substantial benefits in the benthic zone. This dynamic could result in a higher frequency of foraging attempts per dive during benthic dives than pelagic dives for larger prey. Furthermore, PrCA instances were estimated by tallying the occurrences of jerks (the temporal derivatives of acceleration) surpassing the predefined threshold (250 m s−3), as recorded on the bio-logger attached to the head of the Weddell seal. Benthic prey in the Ross Sea predominantly comprises hefty fish, such as icefish or Antarctic toothfish, and other fish heavier than the Antarctic silverfish, the only holopelagic fish in the Ross Sea whose adult form exceeds 50 g. Diving predators require increased mobility to effectively handle larger prey, resulting in higher variance in behavioral data, including acceleration (Watanabe and Takahashi, 2013; Volpov et al., 2015). Weddell seals also handle large prey such as Antarctic toothfish, the flesh of which is exclusively consumed by them (Davis et al., 2004; Ainley and Sniff, 2009; Goetz et al., 2017). Therefore, this study acknowledges the likelihood that foraging frequency may have been overestimated when Weddell seals handle larger prey.
Differences in diving behavior between day and night were also observed. Weddell seals performed deeper dives during the day, which was marked by a higher incidence of benthic dives and PrCA events; however, no significant differences between the time periods were observed. The variation in diel patterns of diving depth could potentially be attributed to the vertical migration behavior of pelagic prey. This migration phenomenon is well documented among pelagic fish species, including Antarctic pelagic fish, which exhibit a diel vertical migration pattern. These fish dive to greater depths as the amount of light at the surface increases, effectively reducing their vulnerability to visual predators that rely on light to locate and pursue prey (Childress, 1995; Fuiman et al., 2002; Hays, 2003; Robison, 2003; Sutton, 2013). Moreover, seals rely on their visual senses to detect prey (Davis et al., 1999). Thus, these seals can dive to greater depths during the day, corresponding to the migratory behavior of pelagic prey in the Ross Sea. Additionally, the energy expenditure associated with hunting pelagic prey may increase with deeper dives during the day. In contrast, the cost of hunting benthic prey may decrease as light increases. Therefore, the proportion of benthic dives increases during the day. As visual predators, Weddell seals are more adept at hunting during daytime, predominantly based on their vision. Consequently, the number of PrCA events increased during the daylight hours.
Concurrently analyzing hydrographic and behavioral data from the Ross Sea revealed seasonal variations in the foraging behavior of Weddell seals that were closely linked to shifts in oceanographic environmental conditions (Figs. 7 and S5). The seals demonstrated a preference for water masses, which could potentially be both nutrient-rich and high-DO areas, and exhibited distinct foraging strategies depending on the light conditions during the day and night. This study demonstrates that Weddell seals adjust their foraging behavior, spatially and temporally adapting to environmental factors. Over the last several decades, the hydrography of the Ross Sea has undergone considerable changes with an increasingly warming world (Castagno et al., 2019; Silvano et al., 2020; Thomas et al., 2020; Yoon et al., 2020). This suggests a continuous adaptation process in the foraging behaviors of marine mammals, including Weddell seals, as they navigate changing marine environments. Therefore, continuous monitoring of the foraging behavior of marine mammals in the Ross Sea is necessary. Our findings serve as a baseline and establish a foundational understanding for future research, particularly concerning the impact of marine environmental changes on the ecosystem of the Ross Sea MPA.
The behavioral and oceanographic data related to this study can be accessed at the Korea Polar Data Center (KPDC) website, https://kpdc.kopri.re.kr (last access: 19 November 2024). The datasets are available under the following DOIs: https://doi.org/10.22663/KOPRI-KPDC-00002402.1 (Chung et al., 2023); https://doi.org/10.22663/KOPRI-KPDC-00002401.1 (Lee et al., 2023); https://doi.org/10.22663/KOPRI-KPDC-00002077.1 (Chung et al., 2022); https://doi.org/10.22663/KOPRI-KPDC-00001658.4 (Lee et al., 2021); and https://doi.org/10.22663/KOPRI-KPDC-00002199 (Chung et al., 2023).
The supplement related to this article is available online at: https://doi.org/10.5194/bg-21-5199-2024-supplement.
HC, STY, and WYL designed the research, and all authors contributed to the conceptualization. JP conducted the investigation in 2020. MP, YK, and UC conducted the investigation in 2021. WY and JSN conducted the investigation in 2023. HAC and STY performed the quality control of raw data for formal analysis. SY and WSL participated the methodology and project administration. HC did the data curation and contribute to formal analysis. HC, STY, and WYL prepared the original draft manuscript and revised it with comments from all authors.
The contact author has declared that none of the authors has any competing interests.
Publisher's note: Copernicus Publications remains neutral with regard to jurisdictional claims made in the text, published maps, institutional affiliations, or any other geographical representation in this paper. While Copernicus Publications makes every effort to include appropriate place names, the final responsibility lies with the authors.
This work was supported by a Korea Polar Research Institute (KOPRI) grant funded by the Ministry of Oceans and Fisheries (KOPRI PE21140, 22140, PE23140; Won Young Lee, Jikang Park, Hyunjae Chung, Mijin Park, Yejin Kim, and Unyoung Chun) and supported by the Korea Institute of Marine Science & Technology Promotion (KIMST) funded by the Ministry of Oceans and Fisheries (RS-2023-00256677; PM23020; Won Young Lee, Hyunjae Chung, Jikang Park, Sukyoung Yun, Won Sang Lee, Hyun A. Choi, Ji Sung Na, and Seung-Tae Yoon). This research was also supported by the Basic Science Research Program through the National Research Foundation of Korea (NRF) funded by the Ministry of Education (2022R1I1A3063629; Seung-Tae Yoon, and Hyun A. Choi).
This research has been supported by the Korea Institute of Marine Science & Technology Promotion (KIMST) funded by the Ministry of Oceans and Fisheries (RS-2023-00256677; PM23020) and by a Korea Polar Research Institute (KOPRI) grant funded by the Ministry of Oceans and Fisheries (KOPRI PE23140). It has also been supported by the National Research Foundation of Korea (grant no. 2022R1I1A3063629).
This paper was edited by Andrew Thurber and reviewed by Fabien Roquet and one anonymous referee.
Ainley, D. G. and Siniff, D. B.: The importance of Antarctic toothfish as prey of Weddell seals in the Ross Sea, Antarct. Sci., 21, 317, https://doi.org/10.1017/S0954102009001953, 2009.
Ainley, D. G., Larue, M. A., Stirling, I., Stammerjohn, S., and Siniff, D. B.: An apparent population decrease, or change in distribution, of Weddell seals along the Victoria Land coast, Mar. Mammal. Sci., 31, 1338–1361, https://doi.org/10.1111/mms.12220, 2015.
Ainley, D. G., Joyce, T. W., Saenz, B., Pitman, R. L., Durban, J. W., Ballard, G., Daly, K., and Kim, S.: Foraging patterns of Antarctic minke whales in McMurdo Sound, Ross Sea, Antarct. Sci., 32, 454–465, https://doi.org/10.1017/S0954102020000310, 2020.
Allegue, H., Réale, D., Picard, B., and Guinet, C.: Track and dive-based movement metrics do not predict the number of prey encountered by a marine predator, Mov. Ecol., 11, 3, https://doi.org/10.1186/s40462-022-00361-2, 2023.
Arce, F., Hindell, M. A., McMahon, C. R., Wotherspoon, S. J., Guinet, C., Harcourt, R. G., and Bestley, S.: Elephant seal foraging success is enhanced in Antarctic coastal polynyas, P. Roy. Soc. B, 289, 2021242, https://doi.org/10.1098/RSPB.2021.2452, 2022.
Arrigo, K. R., van Dijken, G. L., and Bushinsky, S.: Primary production in the Southern Ocean, 1997–2006, J. Geophys. Res.-Oceans, 113, 8004, https://doi.org/10.1029/2007JC004551, 2008.
Aubone, N., Saraceno, M., Torres Alberto, M. L., Campagna, J., Ster, L. Le, Picard, B., Hindell, M., Campagna, C., and Guinet, C. R.: Physical changes recorded by a deep diving seal on the Patagonian slope drive large ecological changes, J. Marine Syst., 223, 103612, https://doi.org/10.1016/j.jmarsys.2021.103612, 2021.
Bailleul, F., Charrassin, J. B., Ezraty, R., Girard-Ardhuin, F., McMahon, C. R., Field, I. C., and Guinet, C.: Southern elephant seals from Kerguelen Islands confronted by Antarctic Sea ice. Changes in movements and in diving behaviour, Deep-Sea Res. Pt. II, 54, 343–355, https://doi.org/10.1016/J.DSR2.2006.11.005, 2007.
Boehme, L., Lovell, P., Biuw, M., Roquet, F., Nicholson, J., Thorpe, S. E., Meredith, M. P., and Fedak, M.: Technical Note: Animal-borne CTD-Satellite Relay Data Loggers for real-time oceanographic data collection, Ocean Sci., 5, 685–695, https://doi.org/10.5194/os-5-685-2009, 2009.
Brierley, A. S. and Cox, M. J.: Shapes of Krill Swarms and Fish Schools Emerge as Aggregation Members Avoid Predators and Access Oxygen, Curr. Biol., 20, 1758–1762, https://doi.org/10.1016/j.cub.2010.08.041, 2010.
Brooks, C. M., Bloom, E., Kavanagh, A., Nocito, E. S., Watters, G. M., and Weller, J.: The Ross Sea, Antarctica: A highly protected MPA in international waters, Mar. Policy, 134, 104795, https://doi.org/10.1016/j.marpol.2021.104795, 2021.
Budillon, G., Castagno, P., Aliani, S., Spezie, G., and Padman, L.: Thermohaline variability and Antarctic bottom water formation at the Ross Sea shelf break, Deep-Sea Res. Pt. I, 58, 1002–1018, https://doi.org/10.1016/J.DSR.2011.07.002, 2011.
Burns, J. M.: The development of diving behavior in juvenile Weddell seals: pushing physiological limits in order to survive, Can. J. Zool., 77, 737–747, https://doi.org/10.1139/z99-022, 1999.
Burns, J. M. and Kooyman, G. L.: Habitat Use by Weddell Seals and Emperor Penguins Foraging in the Ross Sea, Antarctica, Am. Zool., 41, 90–98, https://doi.org/10.1093/icb/41.1.90, 2001.
Burns, J. M., Trumble, S. J., Castellini, M. A., and Testa, J. W.: The diet of Weddell seals in McMurdo Sound, Antarctica as determined from scat collections and stable isotope analysis, Polar Biol., 19, 272–282, https://doi.org/10.1007/s003000050245, 1998.
Castagno, P., Capozzi, V., DiTullio, G. R., Falco, P., Fusco, G., Rintoul, S. R., Spezie, G., and Budillon, G.: Rebound of shelf water salinity in the Ross Sea, Nat. Commun., 10, 5441, https://doi.org/10.1038/s41467-019-13083-8, 2019.
Childress, J. J.: Are there physiological and biochemical adaptations of metabolism in deep-sea animals?, Trends Ecol. Evol., 10, 30–36, https://doi.org/10.1016/S0169-5347(00)88957-0, 1995.
Chung, H., Lee, W. Y., and Lee, W. S.: Nansen ice sheet seal-CTD profiles in 2023, Korea Polar Data Center [data set], https://doi.org/10.22663/KOPRI-KPDC-00002199, 2023.
Chung, H., Kim, S., and Lee, W. L.: Terra Nova Bay seal-CTD profiles in 2022, Korea Polar Data Center [data set], https://doi.org/10.22663/KOPRI-KPDC-00002077.1, 2022.
Chung, H., Kim, S., and Lee, W. Y.: Movement Tracking Data of Weddell Seals in the Ross Sea (2022), Korea Polar Data Center [data set], https://doi.org/10.22663/KOPRI-KPDC-00002402.1, 2023.
Davis, R. W., Fuiman, L. A., Williams, T. M., Collier, S. O., Hagey, W. P., Kanatous, S. B., Kohin, S., and Horning, M.: Hunting Behavior of a Marine Mammal Beneath the Antarctic Fast Ice, Science, 283, 993–996, https://doi.org/10.1126/science.283.5404.993, 1999.
Davis, R. W., Fuiman, L. A., Williams, T. M., Horning, M., and Hagey, W.: Classification of Weddell seal dives based on 3 dimensional movements and video-recorded observations, Mar. Ecol. Prog. Ser., 264, 109–122, https://doi.org/10.3354/MEPS264109, 2003.
Davis, R. W., Hagey, W., and Horning, M.: Monitoring the behavior and multi-dimensional movements of Weddell seals using an animal-borne video and data recorder, Memoirs of National Institute of Polar Research, Special issue, 58, 148–154, 2004.
Dearborn, J. H.: Food of Weddell Seals at McMurdo Sound, Antarctica, J. Mammal, 46, 37–43, https://doi.org/10.2307/1377814, 1965.
DeJong, H. B., Dunbar, R. B., Koweek, D. A., Mucciarone, D. A., Bercovici, S. K., and Hansell, D. A.: Net community production and carbon export during the late summer in the Ross Sea, Antarctica, Global Biogeochem. Cy., 31, 473–491, https://doi.org/10.1002/2016GB005417, 2017.
DeWitt, H. H., Heemstra, P. C., and Gon, O.: Nototheniidae, in: Fishes of the Southern Ocean, edited by: Heemstra, P. C. and Gon, O., JLB Smith Institute of Ichthyology, Grahamstown, 279–331, https://doi.org/10.5962/bhl.title.141868, 1990.
Doney, S. C., Ruckelshaus, M., Emmett Duffy, J., Barry, J. P., Chan, F., English, C. A., Galindo, H. M., Grebmeier, J. M., Hollowed, A. B., Knowlton, N., Polovina, J., Rabalais, N. N., Sydeman, W. J., and Talley, L. D.: Climate Change Impacts on Marine Ecosystems, 4, 11–37, https://doi.org/10.1146/annurev-marine-041911-111611, 2011.
Dorschel, B., Hehemann, L., Viquerat, S., Warnke, F., Dreutter, S., Tenberge, Y. S., Accettella, D., An, L., Barrios, F., Bazhenova, E., Black, J., Bohoyo, F., Davey, C., De Santis, L., Dotti, C. E., Fremand, A. C., Fretwell, P. T., Gales, J. A., Gao, J., Gasperini, L., Greenbaum, J. S., Jencks, J. H., Hogan, K., Hong, J. K., Jakobsson, M., Jensen, L., Kool, J., Larin, S., Larter, R. D., Leitchenkov, G., Loubrieu, B., Mackay, K., Mayer, L., Millan, R., Morlighem, M., Navidad, F., Nitsche, F. O., Nogi, Y., Pertuisot, C., Post, A. L., Pritchard, H. D., Purser, A., Rebesco, M., Rignot, E., Roberts, J. L., Rovere, M., Ryzhov, I., Sauli, C., Schmitt, T., Silvano, A., Smith, J., Snaith, H., Tate, A. J., Tinto, K., Vandenbossche, P., Weatherall, P., Wintersteller, P., Yang, C., Zhang, T., and Arndt, J. E.: The International Bathymetric Chart of the Southern Ocean Version 2, Sci. Data, 9, 275, https://doi.org/10.1038/s41597-022-01366-7, 2022.
Fabiano, M., Povero, P., and Danovaro, R.: Distribution and composition of particulate organic matter in the Ross Sea (Antarctica), Polar Biol., 13, 525–533, https://doi.org/10.1007/BF00236394, 1993.
Fuiman, L. A., Davis, R. W., and Williams, T. M.: Behavior of midwater fishes under the Antarctic ice: observations by a predator, Mar. Biol., 140, 815–822, https://doi.org/10.1007/S00227-001-0752-Y, 2002.
Gerringa, L. J. A., Alderkamp, A. C., van Dijken, G., Laan, P., Middag, R., and Arrigo, K. R.: Dissolved Trace Metals in the Ross Sea, Front. Mar. Sci., 7, 577098, https://doi.org/10.3389/fmars.2020.577098, 2020.
Goetz, K. T., Burns, J. M., Hückst?dt, L. A., Shero, M. R., and Costa, D. P.: Temporal variation in isotopic composition and diet of Weddell seals in the western Ross Sea, Deep-Sea Res. Pt. II, 140, 36–44, https://doi.org/10.1016/j.dsr2.2016.05.017, 2017.
Goetz, K. T., Dinniman, M. S., Hückstädt, L. A., Robinson, P. W., Shero, M. R., Burns, J. M., Hofmann, E. E., Stammerjohn, S. E., Hazen, E. L., Ainley, D. G., and Costa, D. P.: Seasonal habitat preference and foraging behaviour of post-moult Weddell seals in the western Ross Sea, R. Soc. Open Sci., 10, 220500, https://doi.org/10.1098/rsos.220500, 2023.
Harcourt, R. G., Kingston, J. J., Waas, J. R., and Hindell, M. A.: Foraging while breeding: alternative mating strategies by male Weddell seals?, Aquat. Conserv., 17, S68–S78, https://doi.org/10.1002/AQC.915, 2007.
Harcourt, R. G., Hindell, M. A., McMahon, C. R., Goetz, K. T., Charrassin, J.-B., Heerah, K., Holser, R., Jonsen, I. D., Shero, M. R., Hoenner, X., Foster, R., Lenting, B., Tarszisz, E., and Pinkerton, M. H.: Regional Variation in Winter Foraging Strategies by Weddell Seals in Eastern Antarctica and the Ross Sea, Front. Mar. Sci., 8, 720335, https://doi.org/10.3389/fmars.2021.720335, 2021.
Hays, G. C.: A review of the adaptive significance and ecosystem consequences of zooplankton diel vertical migrations, Hydrobiologia, 503, 163–170, https://doi.org/10.1023/B:HYDR.0000008476.23617.b0, 2003.
Heerah, K., Andrews-Goff, V., Williams, G., Sultan, E., Hindell, M., Patterson, T., and Charrassin, J.-B.: Ecology of Weddell seals during winter: Influence of environmental parameters on their foraging behaviour, Deep-Sea Res. Pt. II, 88–89, 23–33, https://doi.org/10.1016/j.dsr2.2012.08.025, 2013.
Heerah, K., Cox, S. L., Blevin, P., Guinet, C., and Charrassin, J.-B.: Validation of Dive Foraging Indices Using Archived and Transmitted Acceleration Data: The Case of the Weddell Seal, Front. Ecol. Evol., 7, 30, https://doi.org/10.3389/fevo.2019.00030, 2019.
Holmgren, W. F., Hansen, C. W., and Mikofski, M. A.: pvlib python: a python package for modeling solar energy systems, Journal of Open Source Software, 3, 884, https://doi.org/10.21105/joss.00884, 2018.
Hothorn, T., Bretz, F., and Westfall, P.: Simultaneous Inference in General Parametric Models, Biometrical J., 50, 346–363, https://doi.org/10.1002/BIMJ.200810425, 2008.
Huang, T., Sun, L., Stark, J., Wang, Y., Cheng, Z., Yang, Q., and Sun, S.: Relative Changes in Krill Abundance Inferred from Antarctic Fur Seal, PLoS One, 6, e27331, https://doi.org/10.1371/journal.pone.0027331, 2011.
Hückstädt, L. A., Piñones, A., Palacios, D. M., McDonald, B. I., Dinniman, M. S., Hofmann, E. E., Burns, J. M., Crocker, D. E., and Costa, D. P.: Projected shifts in the foraging habitat of crabeater seals along the Antarctic Peninsula, Nat. Clim. Change, 10, 472–477, https://doi.org/10.1038/s41558-020-0745-9, 2020.
Ingrosso, G., Giani, M., Kralj, M., Comici, C., Rivaro, P., Budillon, G., Castagno, P., Zoccarato, L., and Celussi, M.: Physical and biological controls on anthropogenic CO2 sink of the Ross Sea, Front. Mar. Sci., 9, 954059, https://doi.org/10.3389/fmars.2022.954059, 2022.
Jacobs, S. S., Giulivi, C. F., and Mele, P. A.: Freshening of the Ross Sea During the Late 20th Century, Science, 297, 386–389, https://doi.org/10.1126/science.1069574, 2002.
Jendersie, S., Williams, M. J. M., Langhorne, P. J., and Robertson, R.: The Density-Driven Winter Intensification of the Ross Sea Circulation, J. Geophys. Res.-Oceans, 123, 7702–7724, https://doi.org/10.1029/2018JC013965, 2018.
Jenkins, A., Shoosmith, D., Dutrieux, P., Jacobs, S., Kim, T. W., Lee, S. H., Ha, H. K., and Stammerjohn, S.: West Antarctic Ice Sheet retreat in the Amundsen Sea driven by decadal oceanic variability, Nat. Geosci., 11, 733–738, https://doi.org/10.1038/s41561-018-0207-4, 2018.
Kokubun, N., Tanabe, Y., Hirano, D., Mensah, V., Tamura, T., Aoki, S., and Takahashi, A.: Shoreward intrusion of oceanic surface waters alters physical and biological ocean structures on the Antarctic continental shelf during winter: Observations from instrumented seals, Limnol. Oceanogr., 66, 3740–3753, https://doi.org/10.1002/lno.11914, 2021.
Kooyman, G. L. and Kooyman, T. G.: Diving Behavior of Emperor Penguins Nurturing Chicks at Coulman Island, Antarctica, Condor, 97, 536–549, https://doi.org/10.2307/1369039, 1995.
Kooyman, G. L., Wahrenbrock, E. A., Castellini, M. A., Davis, R. W., and Sinnett, E. E.: Aerobic and anaerobic metabolism during voluntary diving in Weddell seals: Evidence of preferred pathways from blood chemsitry and behavior, J. Comp. Physiol. B, 138, 335–346, https://doi.org/10.1007/BF00691568, 1980.
Kustka, A. B., Kohut, J. T., White, A. E., Lam, P. J., Milligan, A. J., Dinniman, M. S., Mack, S., Hunter, E., Hiscock, M. R., Smith, W. O., and Measures, C. I.: The roles of MCDW and deep water iron supply in sustaining a recurrent phytoplankton bloom on central Pennell Bank (Ross Sea), Deep-Sea Res. Pt. I, 105, 171–185, https://doi.org/10.1016/j.dsr.2015.08.012, 2015.
Labrousse, S., Ryan, S., Roquet, F., Picard, B., McMahon, C. R., Harcourt, R., Hindell, M., Le Goff, H., Lourenco, A., David, Y., Sallée, J.-B. B., and Charrassin, J.-B. B.: Weddell seal behaviour during an exceptional oceanographic event in the Filchner-Ronne Ice Shelf in 2017, Antarct. Sci., 33, 252–264, https://doi.org/10.1017/S0954102021000092, 2021.
La Mesa, M. and Eastman, J. T.: Antarctic silverfish: life strategies of a key species in the high-Antarctic ecosystem, Fish Fish., 13, 241–266, https://doi.org/10.1111/j.1467-2979.2011.00427.x, 2012.
La Mesa, M., Eastman, J. T., and Vacchi, M.: The role of notothenioid fish in the food web of the Ross Sea shelf waters: a review, Polar Biol., 27, 321–338, https://doi.org/10.1007/s00300-004-0599-z, 2004.
LaRue, M., Salas, L., Nur, N., Ainley, D., Stammerjohn, S., Pennycook, J., Dozier, M., Saints, J., Stamatiou, K., Barrington, L., and Rotella, J.: Insights from the first global population estimate of Weddell seals in Antarctica, Sci. Adv., 7, 3674–3698, https://doi.org/10.1126/SCIADV.ABH3674, 2021.
Lee, W Y., Chung, H., Park, J., and Kim, S.: CTD profiles from seal-tagging CTD bio-loggers, Korea Polar Data Center [data set], https://doi.org/10.22663/KOPRI-KPDC-00001658.4, 2021.
Lee, W. Y., Kim, S., Park, J., and Chung, H.: Movement Tracking Data of Weddell Seals in the oss Sea (2021), Korea Polar Data Center [data set], https://doi.org/10.22663/KOPRI-KPDC-00002401.1, 2023.
Lorrain, A., Graham, B., Ménard, F., Popp, B., Bouillon, S., Breugel, P. van, and Cherel, Y.: Nitrogen and carbon isotope values of individual amino acids: a tool to study foraging ecology of penguins in the Southern Ocean, Mar. Ecol. Prog. Ser., 391, 293–306, https://doi.org/10.3354/meps08215, 2009.
McMahon, C. R., Burton, H., Slip, D., McLean, S., and Bester, M.: Field immobilisation of southern elephant seals with intravenous tiletamine and zolazepam, Vet. Rec., 146, 251–254, https://doi.org/10.1136/vr.146.9.251, 2000.
McMahon, C. R., Roquet, F., Baudel, S., Belbeoch, M., Bestley, S., Blight, C., Boehme, L., Carse, F., Costa, D. P., Fedak, M. A., Guinet, C., Harcourt, R., Heslop, E., Hindell, M. A., Hoenner, X., Holland, K., Holland, M., Jaine, F. R. A., Jeanniard du Dot, T., Jonsen, I., Keates, T. R., Kovacs, K. M., Labrousse, S., Lovell, P., Lydersen, C., March, D., Mazloff, M., McKinzie, M. K., Muelbert, M. M. C., O'Brien, K., Phillips, L., Portela, E., Pye, J., Rintoul, S., Sato, K., Sequeira, A. M. M., Simmons, S. E., Tsontos, V. M., Turpin, V., van Wijk, E., Vo, D., Wege, M., Whoriskey, F. G., Wilson, K., and Woodward, B.: Animal Borne Ocean Sensors – AniBOS – An Essential Component of the Global Ocean Observing System, Front. Mar. Sci., 8, 751840, https://doi.org/10.3389/fmars.2021.751840, 2021.
Meyer, B., Freier, U., Grimm, V., Groeneveld, J., Hunt, B. P. V., Kerwath, S., King, R., Klaas, C., Pakhomov, E., Meiners, K. M., Melbourne-Thomas, J., Murphy, E. J., Thorpe, S. E., Stammerjohn, S., Wolf-Gladrow, D., Auerswald, L., Götz, A., Halbach, L., Jarman, S., Kawaguchi, S., Krumpen, T., Nehrke, G., Ricker, R., Sumner, M., Teschke, M., Trebilco, R., and Yilmaz, N. I.: The winter pack-ice zone provides a sheltered but food-poor habitat for larval Antarctic krill, Nat. Ecol. Evol., 1, 1853–1861, https://doi.org/10.1038/s41559-017-0368-3, 2017.
Mintenbeck, K.: Trophic interactions within high Antarctic shelf communities – Food web structure and the significance of fish, hdl:10013/epic.30692.d001, 2008.
Nachtsheim, D. A., Ryan, S., Schröder, M., Jensen, L., Oosthuizen, W. C., Bester, M. N., Hagen, W., and Bornemann, H.: Foraging behaviour of Weddell seals (Leptonychotes weddellii) in connection to oceanographic conditions in the southern Weddell Sea, Prog. Oceanogr., 173, 165–179, https://doi.org/10.1016/j.pocean.2019.02.013, 2019.
Noren, S. R., Pearson, L. E., Davis, J., Trumble, S. J., and Kanatous, S. B.: Different Thermoregulatory Strategies in Nearly Weaned Pup, Yearling, and Adult Weddell Seals (Leptonychotes weddelli), Physiol. Biochem. Zool., 81, 868–879, https://doi.org/10.1086/588489, 2008.
Oliver, M. A. and Webster, R.: Kriging: a method of interpolation for geographical information systems, Int. J. Geogr. Inf. Syst., 4, 313–332, https://doi.org/10.1080/02693799008941549, 1990.
Orsi, A. H. and Wiederwohl, C. L.: A recount of Ross Sea waters, Deep-Sea Res. Pt. II, 56, 778–795, https://doi.org/10.1016/j.dsr2.2008.10.033, 2009.
Orsi, A. H., Johnson, G. C., and Bullister, J. L.: Circulation, mixing, and production of Antarctic Bottom Water, Prog. Oceanogr., 43, 55–109, https://doi.org/10.1016/S0079-6611(99)00004-X, 1999.
Park, S., Thiebot, J.-B., Kim, J.-H., Kim, K. W., Chung, H., and Lee, W. Y.: Mare incognita: Adélie penguins foraging in newly exposed habitat after calving of the Nansen Ice Shelf, Environ. Res., 201, 111561, https://doi.org/10.1016/j.envres.2021.111561, 2021.
Pebesma, E. J.: Multivariable geostatistics in S: the gstat package, Comput. Geosci., 30, 683–691, https://doi.org/10.1016/J.CAGEO.2004.03.012, 2004.
Photopoulou, T., Heerah, K., Pohle, J., and Boehme, L.: Sex-specific variation in the use of vertical habitat by a resident Antarctic top predator, P/ Roy/ Soc. B, 287, 20201447, https://doi.org/10.1098/rspb.2020.1447, 2020.
Pinheiro, J., Bates, D., and R Core Team: nlme: Linear and Nonlinear Mixed Effects Models, https://CRAN.R-project.org/package=nlme (last access: 24 April 2024), 2022.
Piñones, A., Hofmann, E. E., Costa, D. P., Goetz, K., Burns, J. M., Roquet, F., Dinniman, M. S., and Klinck, J. M.: Hydrographic variability along the inner and mid-shelf region of the western Ross Sea obtained using instrumented seals, Prog. Oceanogr., 174, 131–142, https://doi.org/10.1016/j.pocean.2019.01.003, 2019.
Plötz, J., Ekau, W., and Reijnders, P. J. H.: Diet of Weddell Seals Leptonychotes Weddellii at Vestkapp, Eastern Weddell Sea (antarctica), in Relation to Local Food Supply, Mar. Mammal Sci., 7, 136–144, https://doi.org/10.1111/j.1748-7692.1991.tb00560.x, 1991.
Rignot, E., Jacobs, S., Mouginot, J., and Scheuchl, B.: Ice-shelf melting around antarctica, Science, 341, 266–270, https://doi.org/10.1126/science.1235798, 2013.
Rignot, E., Mouginot, J., Scheuchl, B., van den Broeke, M., van Wessem, M. J., and Morlighem, M.: Four decades of Antarctic Ice Sheet mass balance from 1979–2017, P. Natl. Acad. Sci. USA, 116, 1095–1103, https://doi.org/10.1073/pnas.1812883116, 2019.
Robison, B. H.: What drives the diel vertical migrations of Antarctic midwater fish?, J. Mar. Biol. Assoc. UK, 83, 639–642, https://doi.org/10.1017/S0025315403007586h, 2003.
Roquet, F., Charrassin, J.-B., Marchand, S., Boehme, L., Fedak, M., Reverdin, G., and Guinet, C.: Delayed-Mode Calibration of Hydrographic Data Obtained from Animal-Borne Satellite Relay Data Loggers, J. Atmos. Ocean. Technol., 28, 787–801, https://doi.org/10.1175/2010JTECHO801.1, 2011.
Rusciano, E., Budillon, G., Fusco, G., and Spezie, G.: Evidence of atmosphere–sea ice–ocean coupling in the Terra Nova Bay polynya (Ross Sea–Antarctica), Cont. Shelf Res., 61–62, 112–124, https://doi.org/10.1016/j.csr.2013.04.002, 2013.
Sahade, R., Lagger, C., Torre, L., Momo, F., Monien, P., Schloss, I., Barnes, D. K. A., Servetto, N., Tarantelli, S., Tatián, M., Zamboni, N., and Abele, D.: Climate change and glacier retreat drive shifts in an Antarctic benthic ecosystem, Sci. Adv., 1, e1500050, https://doi.org/10.1126/sciadv.1500050, 2015.
Schofield, O., Ducklow, H. W., Martinson, D. G., Meredith, M. P., Moline, M. A., and Fraser, W. R.: How Do Polar Marine Ecosystems Respond to Rapid Climate Change?, Science, 328, 1520–1523, https://doi.org/10.1126/science.1185779, 2010.
Sedwick, P. N. and Ditullio, G. R.: Regulation of algal blooms in Antarctic Shelf Waters by the release of iron from melting sea ice, Geophys. Res. Lett., 24, 2515–2518, https://doi.org/10.1029/97GL02596, 1997.
Siegelman, L., Roquet, F., Mensah, V., Rivière, P., Pauthenet, E., Picard, B., and Guinet, C.: Correction and Accuracy of High- and Low-Resolution CTD Data from Animal-Borne Instruments, J. Atmos. Ocean. Tech., 36, 745–760, https://doi.org/10.1175/JTECH-D-18-0170.1, 2019.
Silvano, A., Foppert, A., Rintoul, S. R., Holland, P. R., Tamura, T., Kimura, N., Castagno, P., Falco, P., Budillon, G., Haumann, F. A., Naveira Garabato, A. C., and Macdonald, A. M.: Recent recovery of Antarctic Bottom Water formation in the Ross Sea driven by climate anomalies, Nat. Geosci., 13, 780–786, https://doi.org/10.1038/s41561-020-00655-3, 2020.
Silvano, A., Purkey, S., Gordon, A. L., Castagno, P., Stewart, A. L., Rintoul, S. R., Foppert, A., Gunn, K. L., Herraiz-Borreguero, L., Aoki, S., Nakayama, Y., Naveira Garabato, A. C., Spingys, C., Akhoudas, C. H., Sallée, J.-B., De Lavergne, C., Abrahamsen, E. P., Meijers, A. J. S., Meredith, M. P., Zhou, S., Tamura, T., Yamazaki, K., Ohshima, K. I., Falco, P., Budillon, G., Hattermann, T., Janout, M. A., Llanillo, P., Bowen, M. M., Darelius, E., Østerhus, S., Nicholls, K. W., Stevens, C., Fernandez, D., Cimoli, L., Jacobs, S. S., Morrison, A. K., Hogg, A. McC., Haumann, F. A., Mashayek, A., Wang, Z., Kerr, R., Williams, G. D., and Lee, W. S.: Observing Antarctic Bottom Water in the Southern Ocean, Front. Mar. Sci., 10, 1221701, https://doi.org/10.3389/fmars.2023.1221701, 2023.
Sipler, R. E. and Connelly, T. L.: Bioavailability of surface dissolved organic matter to aphotic bacterial communities in the Amundsen Sea Polynya, Antarctica, Elementa: Science of the Anthropocene, 3, 000060, https://doi.org/10.12952/journal.elementa.000060, 2015.
Schmidt, K., Atkinson, A., Steigenberger, S., Fielding, S., Lindsay, M. C. M., Pond, D. W., Tarling, G. A., Klevjer, T. A., Allen, C. S., Nicol, S., and Achterberg, E. P.: Seabed foraging by Antarctic krill: Implications for stock assessment, bentho-pelagic coupling, and the vertical transfer of iron, Limnol. Oceanogr., 56, 1411–1428, https://doi.org/10.4319/lo.2011.56.4.1411, 2011.
Smith, W. O., Sedwick, P., Arrigo, K., Ainley, D., and Orsi, A.: The Ross Sea in a Sea of Change, Oceanography, 25, 90–103, https://doi.org/10.5670/oceanog.2012.80, 2012.
Smith, W. O., Tozzi, S., Long, M. C., Sedwick, P. N., Peloquin, J. A., Dunbar, R. B., Hutchins, D. A., Kolber, Z., and DiTullio, G. R.: Spatial and temporal variations in variable fluoresence in the Ross Sea (Antarctica): Oceanographic correlates and bloom dynamics, Deep-Sea Res. Pt. I, 79, 141–155, https://doi.org/10.1016/j.dsr.2013.05.002, 2013.
Smith, W. O., Ainley, D. G., Arrigo, K. R., and Dinniman, M. S.: The Oceanography and Ecology of the Ross Sea, Annu. Rev. Mar. Sci., 6, 469–487, https://doi.org/10.1146/annurev-marine-010213-135114, 2014.
SMRU Instrumentation: CTD Oceanography SRDL (Argos), https://www.smru.st-andrews.ac.uk/Instrumentation/CTD/, last access: 20 May 2024.
Speakman, C. N., Hoskins, A. J., Hindell, M. A., Costa, D. P., Hartog, J. R., Hobday, A. J., and Arnould, J. P. Y.: Environmental influences on foraging effort, success and efficiency in female Australian fur seals, Sci. Rep., 10, 1–16, https://doi.org/10.1038/s41598-020-73579-y, 2020.
Sutton, T. T.: Vertical ecology of the pelagic ocean: classical patterns and new perspectives, J. Fish. Biol., 83, 1508–1527, https://doi.org/10.1111/jfb.12263, 2013.
Thomas, G., Purkey, S. G., Roemmich, D., Foppert, A., and Rintoul, S. R.: Spatial Variability of Antarctic Bottom Water in the Australian Antarctic Basin From 2018–2020 Captured by Deep Argo, Geophys. Res. Lett., 47, https://doi.org/10.1029/2020GL089467, 2020.
Treasure, A., Roquet, F., Ansorge, I., Bester, M., Boehme, L., Bornemann, H., Charrassin, J.-B., Chevallier, D., Costa, D., Fedak, M., Guinet, C., Hammill, M., Harcourt, R., Hindell, M., Kovacs, K., Lea, M.-A., Lovell, P., Lowther, A., Lydersen, C., McIntyre, T., McMahon, C., Muelbert, M., Nicholls, K., Picard, B., Reverdin, G., Trites, A., Williams, G., and de Bruyn, P. J. N.: Marine Mammals Exploring the Oceans Pole to Pole: A Review of the MEOP Consortium, Oceanography, 30, 132–138, https://doi.org/10.5670/oceanog.2017.234, 2017.
Viviant, M., Trites, A. W., Rosen, D. A. S. S., Monestiez, P., and Guinet, C.: Prey capture attempts can be detected in Steller sea lions and other marine predators using accelerometers, Polar Biol., 33, 713–719, https://doi.org/10.1007/s00300-009-0750-y, 2010.
Volpov, B. L., Hoskins, A. J., Battaile, B. C., Viviant, M., Wheatley, K. E., Marshall, G., Abernathy, K., and Arnould, J. P. Y.: Identification of Prey Captures in Australian Fur Seals (Arctocephalus pusillus doriferus) Using Head-Mounted Accelerometers: Field Validation with Animal-Borne Video Cameras, PLoS One, 10, e0128789, https://doi.org/10.1371/JOURNAL.PONE.0128789, 2015.
Wartzok, D., Elsner, R., Stone, H., Kelly, B. P., and Davis, R. W.: Under-ice movements and the sensory basis of hole finding by ringed and Weddell seals, Can. J. Zool., 70, 1712–1722, https://doi.org/10.1139/z92-238, 1992.
Watanabe, Y. Y. and Takahashi, A.: Linking animal-borne video to accelerometers reveals prey capture variability, P. Natl. Acad. Sci. USA, 110, 2199–2204, https://doi.org/10.1073/pnas.121624411, 2013.
Wheatley, K. E., Bradshaw, C. J. A., Davis, L. S., Harcourt, R. G., and Hindell, M. A.: Influence of Maternal Mass and Condition on Energy Transfer in Weddell Seals, J. Anim. Ecol., 75, 724–733, 2006.
Wheatley, K. E., Bradshaw, C. J. A., Harcourt, R. G., and Hindell, M. A.: Feast or famine: evidence for mixed capital–income breeding strategies in Weddell seals, Oecologia, 155, 11–20, https://doi.org/10.1007/s00442-007-0888-7, 2008.
Ydesen, K. S., Wisniewska, D. M., Hansen, J. D., Beedholm, K., Johnson, M., and Madsen, P. T.: What a jerk: Prey engulfment revealed by high-rate, super-cranial accelerometry on a harbour seal (Phoca vitulina), J. Exp. Biol., 217, 2239–2243, https://doi.org/10.1242/JEB.100016, 2014.
Yoon, S.-T., Lee, W. S., Stevens, C., Jendersie, S., Nam, S., Yun, S., Hwang, C. Y., Jang, G. I., and Lee, J.: Variability in high-salinity shelf water production in the Terra Nova Bay polynya, Antarctica, Ocean Sci., 16, 373–388, https://doi.org/10.5194/os-16-373-2020, 2020.
Zheng, Y., Heywood, K. J., Webber, B. G. M., Stevens, D. P., Biddle, L. C., Boehme, L., and Loose, B.: Winter seal-based observations reveal glacial meltwater surfacing in the southeastern Amundsen Sea, Commun. Earth Environ., 2, 40, https://doi.org/10.1038/s43247-021-00111-z, 2021.