the Creative Commons Attribution 4.0 License.
the Creative Commons Attribution 4.0 License.
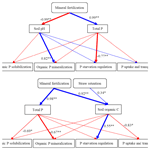
Effect of straw retention and mineral fertilization on P speciation and P-transformation microorganisms in water- extractable colloids of a Vertisol
Shanshan Bai
Yifei Ge
Dongtan Yao
Yifan Wang
Jinfang Tan
Shuai Zhang
Yutao Peng
Xiaoqian Jiang
Water-extractable colloids (WECs) serve as crucial micro-particulate components in soils, playing a vital role in the cycling and potential bioavailability of soil phosphorus (P). Yet, the underlying information regarding soil P species and P-transformation microorganisms at the microparticle scale under long-term straw retention and mineral fertilization is barely known. Here, a fixed field experiment (∼ 13 years) in a Vertisol was performed to explore the impacts of straw retention and mineral fertilization on inorganic P, organic P, and P-transformation microorganisms in bulk soils and WECs through a sequential extraction procedure, P K-edge X-ray absorption near-edge structure (XANES), 31P nuclear magnetic resonance (NMR), and metagenomics analysis. In bulk soil, mineral fertilization led to increases in the levels of total P, available P, acid phosphatase (ACP), high-activity inorganic P fractions (Ca2-P, Ca8-P, Al-P, and Fe-P), and organic P (orthophosphate monoesters and orthophosphate diesters) but significantly decreased the abundances of P-cycling genes including P mineralization, P-starvation response regulation, and P uptake and transport by decreasing soil pH and increasing total P. Straw retention had no significant effects on P species and P-transformation microorganisms in bulk soils but led to increases in organic carbon, total P, and available P concentrations in WECs. Furthermore, compared with mineral fertilization, straw retention caused significantly greater differences in the relative abundances of P-cycling genes between WECs and bulk soils. The abundances of phoD gene and phoD-harboring Proteobacteria in WECs increased significantly under straw retention, suggesting that the P-mineralizing capacity increased. Thus, mineral fertilization reduced microbial P-solubilizing and mineralizing capacity in bulk soil. Straw retention could potentially accelerate the turnover, mobility, and availability of P by increasing the nutrient contents and P-mineralizing capacity at the microscopic colloidal scale.
- Article
(2190 KB) - Full-text XML
-
Supplement
(846 KB) - BibTeX
- EndNote
Phosphorus (P) has a vital function in the productivity of agroecological systems (Jiang et al., 2015). Vertisol (Staff, 2010), also known as a Shajiang black soil in Chinese Soil Taxonomy, covers approximately 4×106 ha in the Huang–Huai–Hai Plain of China (Guo et al., 2022). Vertisol contains abundant calcium, contains scant organic matter, and has poor fertility (Chen et al., 2020). The strong P-fixation capacity by abundant calcium and poor supply capacity of P restrict agricultural production severely (Ma et al., 2019). Straw retention and mineral fertilization are commonly employed to enhance soil nutrient contents in this area (Zhao et al., 2018). Under mineral fertilization and straw retention, dicalcium phosphate (Ca2-P), iron-bound P (Fe-P), and aluminum-bound P (Al-P) contents increased, but the apatite (Ca10-P) concentration was reduced, thereby promoting the transformation of P fractions (Xu et al., 2022). Cao et al. (2022) suggested that the combination of straw retention and mineral fertilization significantly increased both inorganic and organic P-species concentrations. Crop straw, which is rich in organic matter and contains a certain amount of nitrogen (N), P, and other nutrients, has demonstrated potential effects on the cycling and processing of P (Damon et al., 2014).
The assessment of potential bioavailability and mobility of soil P heavily relies on the speciation and distribution of P in soil aggregates (Ranatunga et al., 2013). Agricultural management practices like the application of fertilizer and straw could modify the microhabitat's physicochemical environment through their influence on soil aggregation (Ju et al., 2023). Maize straw promoted the accumulation and stabilization of inorganic and organic P in soil aggregates, particularly in the 250–2000 µm fraction. Additionally, it decreased the relative contribution rates of the < 53 µm fraction to inorganic and organic P fractions compared with mineral fertilizer (Cao et al., 2021). Generally, soil aggregate fractionation contains particle sizes of > 0.25 mm, 0.053–0.25 mm, and < 0.053 mm, and the distribution and dynamics of P in these aggregates have been widely researched (Cheng et al., 2019; Deng et al., 2021). However, there are few studies on the forms and distribution of P in soil water-extractable colloids (WECs; < 2 µm in size), which significantly contribute to P cycling due to the large binding ability, high mobility, and bioavailability of P (Jiang et al., 2023; Fresne et al., 2022). WECs, readily extracted upon water contact, are regarded as indexes of mobile soil colloids (Missong et al., 2018) and main factors that impact the mobility and availability of soil P (Zhang et al., 2021). Colloidal P could contribute to plant-available P as reported by Montavo et al. (2015). Additionally, microaggregates (including colloidal size fractions) provide a favorable habitat for microorganisms, and the biochemical processes functioning at the microparticle scale would also be important for soil P cycling and availability (Totsche et al., 2018). However, information related to how straw retention and mineral fertilization management affect soil P dynamics at scales of WECs remains scarce.
Microorganisms are instrumental in facilitating the transformation of soil P species, P cycling, and P-availability regulation (Bergkemper et al., 2016). The processes of microbial P transformation primarily consist of (1) inorganic P solubilization (e.g., gcd), (2) organic P mineralization (e.g., phoD, phoA, phy), (3) P-starvation response regulation (e.g., phoR, phoB), and (4) P uptake and transport (e.g., pst) (Richardson and Simpson, 2011). Fertilization could further change the abundance and taxonomic assignments of P-cycling gene clusters (Dai et al., 2020; Zhang et al., 2023). For example, continuous N fertilization over an extended period may lead to a decline in soil pH, inhibition of microbial growth, alterations in the composition of the microbial community, and ultimately the reduction of the capacity for P solubilization (Rousk et al., 2010). Additionally, gene expressions related to organic P mineralization, P-starvation regulation, and P uptake and transport are primarily affected by the environmental P supply (Hsieh and Wanner, 2010). Several research studies have shown that an adequate P supply inhibited the gene expressions associated with P-starvation response (e.g., phoR), as well as genes encoding alkaline phosphatase (e.g., phoD) and phytase (e.g., phy) (Yao et al., 2018; Xie et al., 2020). Straw retention could bring an increase in soil organic C, potentially enhancing the diversity and richness of phoD-harboring microbes and the phoD abundance (Cao et al., 2022). Moreover, alterations in the P-transformation genes are driven by the structural effects of soil aggregates in addition to P availability (Neal et al., 2017). However, little is known about the richness and distribution of genes related to P transformation in WEC fraction, with the treatments of straw retention and mineral fertilization, which will offer a new perspective on P cycling and availability from a microbial perspective.
In long-term (∼ 13 years) field experiments modulating straw retention and mineral fertilization, we investigated the responses of P speciation, P-cycling-related genes, and taxonomic assignments in bulk soils and WECs under straw retention and fertilization management strategies. These results could elucidate the underlying mechanisms of soil P cycling and availability under mineral fertilization and straw retention from the microparticle and microbial perspective, providing an important insight into regulating P cycling in agriculture soils.
2.1 Experimental design
In 2008, a field trial was conducted in Mengcheng County (33°9′ N, 116°32′ E), Anhui Province, China, to investigate the rotation of winter wheat and summer maize. The soil is classified as a Vertisol (Staff, 2010), which is derived from fluvio-lacustrine sediments. The region experiences average annual temperature and precipitation of 14.8° and 732.6 mm, respectively.
Six treatments with three replicates (each plot area was 43.2 m2) were carried out: (1) the control treatment without straw retention and mineral fertilizer (W0M0F0), (2) single application of mineral fertilizer (W0M0F1), (3) maize straw retention combined with mineral fertilization (W0M1F1), (4) wheat straw retention combined with mineral fertilization (W1M0F1), (5) both wheat and maize straw retention without fertilization (W1M1F0), and (6) a combination of both wheat and maize straw retention with mineral fertilization (W1M1F1). In the W0M1F1 treatment, maize straw was chopped into fragments approximately 10 cm in length and uniformly distributed in each plot after harvest, while wheat straw was removed. In the W1M0F1 treatment, wheat straw was similarly returned to plots and maize straw was removed. For W1M1F0 and W1M1F1 treatments, maize straw and wheat straw were both returned to plots when they were harvested. The amounts of residue incorporation for wheat and maize were 7500 and 12 000 kg hm−2, respectively. For the W0M0F1 treatments, straw was removed and the roots were left in the field. For the fertilization treatments (i.e., W0M0F1, W0M1F1, W1M0F1, W1M1F1), 240.0 kg hm−2 N (55 % as basal fertilizer and 45 % as topdressing during the reviving–jointing period), 90.0 kg hm−2 P, and 90.0 kg hm−2 K (100 % as basal fertilizer) were applied in each growing season of winter wheat. The 300.0 kg hm−2 N (50 % as basal fertilizer and 50 % as topdressing in the flare opening period), 90.0 kg hm−2 P, and 90.0 kg hm−2 K (100 % as basal fertilizer) were applied in each growing season of summer maize. The fertilizers were comprised of compound and urea fertilizer (N–P2O5–K2O: 15–15–15). The contents of P in maize straw and wheat straw were about 1.5 and 0.8 g kg−1, respectively (Chai et al., 2021). In addition, weeds, disease, and pest control for both wheat and maize were consistent.
2.2 Soil sampling and water-extractable colloids (WECs)
From all six treatment plots soil samples were collected after wheat harvest in June 2021. Five soil cores (0–20 cm) were gathered from each replicate plot using the quincunx sampling method and then blended evenly to create a composite sample. Divisions into three subsamples were made for each sample. The first subsample was preserved at 4 °C to examine soil microbial biomass C (MBC) and microbial biomass P (MBP), along with acid and alkaline phosphatase activity (ACP and ALP). Another sample was at stored −80 °C for metagenomics analysis. For other soil chemical property tests, the last sample was subjected to air drying, grinding, and subsequently sieving through a 2 mm mesh. In this study, the soil fraction consisting of particles smaller than 2 mm was designated as bulk soil.
To further explore the impact of solely straw retention and solely mineral fertilization on P cycling in soil colloids, the particle size fractionation method following Stokes' law (Sequaris and Lewandowski, 2003) was utilized to obtain WECs for the W0M0F0, W0M0F1, and W1M1F0 treatments in this study. The field-fresh soil samples were used for sedimentation to replicate natural conditions where soil exists in its native state, neither completely dry nor saturated, enabling a more accurate study of these natural processes. About 113–116 g of field-fresh soil samples (equivalent to 100 g of dry soil) was blended with 200 mL ultrapure water and then shaken at a speed of 150 rpm for a duration of 6 h. Afterward, we added an extra 600 mL of ultrapure water and blended thoroughly. The particles > 20 µm were allowed to settle for a period of 6 min. The 2–20 µm size fraction was then obtained by eliminating the supernatant following an additional sedimentation of 12 h. The final supernatant containing the colloidal particle fraction (< 2 µm) was obtained and defined as WECs. The soil was classified as sandy loam according to the international soil texture classification standard. The mass proportions of particles with > 20, 2–20, and < 2 µm to bulk soil are shown in Fig. S1 in the Supplement.
2.3 Soil chemical properties
A pH meter (Rex Electric Chemical PHSJ-3F) was utilized to measure soil pH in a 1:2.5 soil–ultrapure water suspension. An elementary analyzer (Vario MAXCNS, Elementar, Germany) was utilized for soil organic carbon (SOC) and total nitrogen (TN). Prior to measuring SOC and TN, the samples were passed through a 0.149 mm sieve. For SOC measurement, 1 M HCl was added to the samples in small increments until effervescence stopped to remove inorganic carbon (Schumacher, 2002). After microwave digestion, total P (TP) concentrations were determined by inductively coupled plasma optical emission spectroscopy (ICP-OES), with no residue left after digestion. The available P (AP, Olsen-P) concentration was quantified using the method described by Olsen and Sommers (1982).
The chloroform fumigation method outlined by Vance et al. (1987) and Brookes et al. (1982) was utilized to quantify the soil MBC and MBP. The extracted C with 0.5 M K2SO4 in non-fumigated and fumigated samples was determined with the Multi N/C 2100S total organic carbon–total nitrogen (TOC–TN) analyzer. The dissolved organic C (DOC) was quantified as the extracted organic C by K2SO4 from the non-fumigated samples (Wu et al., 2019). MBC was quantified by measuring the variation in extractable C content between the non-fumigated and fumigated soil samples using the universal conversion factor of 0.45 (Vance et al., 1987). MBP was calculated as the variation in extractable P with 0.5 M NaHCO3 between the non-fumigated and fumigated soil samples, with a conversion factor of 0.40 (Brookes et al., 1982). The measurement of ACP and ALP followed the procedures outlined by Tabatabai and Bremner (1969).
2.4 Phosphorus sequential extraction procedure and P K-edge XANES spectroscopy
The modified sequential extraction procedure, as described by Jiang and Gu (1989) and Audette et al. (2016), was utilized to extract various P fractions in bulk soils. These fractions included Ca2-P, extracted with 0.25 M NaHCO3 (pH 8.0); Ca8-P, extracted with 0.5 M NH4Ac (pH 4.2); Al-P, extracted with 0.5 M NH4F (pH 8.2); Fe-P, extracted with 0.1 M NaOH–Na2CO3 (pH 12.0); occluded P (O-P), extracted with 0.3 M CD (sodium citrate–dithionite–sodium hydroxide, pH 13); and Ca10-P, extracted with 0.25 M H2SO4 (pH 1.0). Then the method outlined by Murphy and Riley (1962) was utilized to ascertain the concentration of each P fraction.
P K-edge X-ray absorption near-edge structure (XANES) spectra were utilized to clarify the P-bonding fractions in WECs and acquired at Beamline 4B7A of the Beijing Synchrotron Radiation Facility, Beijing, China. Dibasic calcium phosphate dihydrate (DCP, CaHPO4•2H2O), hydroxyapatite (HAP, Ca5(PO4)3OH), aluminum phosphate (Al-P, AlPO4), iron phosphate dihydrate (Fe-P, FePO4•2H2O), and inositol hexakisphosphate (IHP) were chosen as references. For XANES data collection, P standards and soil samples were thinly spread on P-free, double-sided carbon tape. The soil spectra were collected in partial fluorescence yield (PFY) mode with an Si (Li) detector, while the spectra of P standards were obtained in total electron yield (TEY) mode. Multiple spectra were obtained with three duplicates for each sample and then averaged. The spectra were studied using Athena (0.9.26) with the energy calibration at 2149 eV (E0), aligning with the peak position of AlPO4, as described by Beauchemin et al. (2003). Then, we performed linear combination fitting (LCF) within the energy range spanning from −10 to 30 eV relative to E0, and the goodness of fit was determined based on the chi-squared and R values. The most likely P species was considered based on these results. The P K-edge XANES spectra of P reference compounds are shown in Fig. S2.
2.5 Solution 31P NMR spectroscopy
Solution 31P NMR spectroscopy was performed to clarify P species (Turner, 2008). A total of 1 g of bulk soil and WEC sample was mixed with 10 mL of 0.25 M NaOH and 0.05 M Na2EDTA and shaken for 4 h to extract P (Cade-Menun and Liu, 2014; Jiang et al., 2017). The procedure is outlined in our prior study (Bai et al., 2023). The 31P-NMR spectra were acquired using a Bruker 500 MHz spectrometer with 4.32 s relaxation delay, 0.68 s acquisition time, 5000 scans, and 90° pulse width (Cade-Menun et al., 2010).
Compound identification relied on chemical shifts following the calibration of the orthophosphate peak to 6.0 ppm (Table S1 in the Supplement). To validate peak identification, samples were spiked with myo-inositol hexakisphosphate, α- and β-glycerophosphate, and adenosine monophosphate (Fig. S3). Instead of being classified as monoesters, α- and β-glycerophosphate as well as mononucleotides (Glyc + nucl) were categorized as orthophosphate diesters (Doolette et al., 2009). Integration was conducted on spectra with broadening at 7 and 2 Hz to calculate the area under each peak. To quantify the concentrations of P species, the peak areas were multiplied by the concentration of NaOH–Na2EDTA-extractable P. The spectra of bulk soil and WECs were processed using MestReNova 10.0.2 software.
2.6 DNA extraction and metagenomics analysis
Soil DNA was extracted using a FastDNA Spin Kit (MP Biomedicals, USA). The Agilent 5400 was utilized to determine the purity, integrity, and concentration of the extracted DNA. The generation of sequencing libraries was carried out using the NEBNext®Ultra™ DNA Library Prep Kit (PerkinElmer, USA). For each sample, barcodes were incorporated to enable sequence attribution. After end polishing, A tailing, and adapter ligation, the DNA fragments were subsequently subjected to PCR amplification. Finally, a NovaSeq 6000 instrument was utilized for sequencing, generating paired-end reads. The fastp software (v.0.18.0) was used to obtain the clean reads (Chen et al., 2018). To be more specific, reads that contained adapter sequences, N bases that reached more than 10 %, or low-quality bases (quality score ≤ 20) that accounted for above 50 % were removed.
MEGAHIT was used to assemble genomes from the filtered reads (fastq formats) with a de Bruijn graph, with a minimum k-mer size of 21 (Li et al., 2015). The default settings of Prodigal were used to identify the protein-coding genes, as described by Hyatt et al. (2010). For functional annotation, we employed the Diamond software to align the identified genes with Kyoto Encyclopedia of Genes and Genomes (KEGG) databases (Kanehisa, 2019) (best hit with an e value ) following the methodologies outlined by Kanehisa and Goto (2000), Buchfink et al. (2015), and Huson et al. (2016).
According to the prior study of Bergkemper et al. (2016), a total of 29 genes associated with P transformation were identified, along with their corresponding Kyoto Encyclopedia of Genes and Genomes (KEGG) Orthology (KO) numbers. These genes were categorized into four distinct groups: (1) genes associated with inorganic P solubilization, (2) genes associated with organic P mineralization, (3) genes associated with P-starvation regulation, and (4) genes associated with microbial P uptake and transport. Table S2 provides a comprehensive list of the categorized genes along with their names, function descriptions, and KO numbers. The sequence data have been submitted to the NCBI Sequence Read Archive (PRJNA909638).
2.7 Statistical analysis
The IBM SPSS (version 25.0) and R (version 4.2.0) software programs were utilized for statistical analyses and data visualization. The normality distribution (Shapiro–Wilk test) was performed before ANOVA. To identify significant differences among mean values at a significance level of 0.05, the Tukey's honestly significant difference (HSD) test was employed. The differences in soil properties, total P, inorganic P, organic P, ACP, and ALP between bulk soils and WECs were tested by an independent-sample t test. The differences in P-cycling gene composition in bulk soils and WECs were displayed by principal component analysis (PCA) with the R package “FactoMineR” (Lê et al., 2008). Principal coordinate analysis (PCoA) was utilized to present the microbial bacterial β diversity for typical P-solubilization (gcd) and mineralization (phoD) genes with the R packages “vegan” and “ape” (Paradis and Schliep, 2019; Oksanen et al., 2024). The associations between the abundances of P-transformation genes and soil characteristics were assessed using Spearman's correlations with the R package “psych”, with a correlation coefficient (R) > 0.6 and P value < 0.05 (Revelle, 2024). A structural equation model (SEM) was used to explore the relationships among agricultural management types, soil properties, and P-cycling-related genes with Amos (24.0). The model fit was assessed with goodness of fit (GFI) and root square mean error of approximation (RMSEA).
3.1 Soil properties in bulk soils and WECs
Straw retention in combination with mineral fertilization (i.e., W0M1F1, W1M0F1, W1M1F1) decreased soil pH from 6.90 to 5.01 and decreased alkaline phosphatase activity (ALP) by 160.25–183.37 µg g−1 h−1 but significantly increased organic C by 2.66–4.73 g kg−1, total N by 0.36–0.60 g kg−1, total P by 0.17–0.19 g kg−1, available P by 28.11–31.97 mg kg−1, and acid phosphatase activity (ACP) by 174.12–449.25 µg g−1 h−1, respectively, compared with the control treatment (i.e., W0M0F0) (Table 1). The variations primarily resulted from the utilization of mineral fertilizers, as there were no noteworthy distinctions observed in these parameters between straw retention combined with mineral fertilization treatments and solely mineral fertilizer (i.e., W0M0F1). There was no significant effect of solely straw retention (i.e., W1M1F0) detectable except for slight increases in soil MBC and MBP contents compared with the control treatment (Table 1). The outcomes suggested that mineral fertilization had a more prominent impact on soil characteristics compared to straw retention. Mineral fertilization indeed enhanced soil nutrient contents. It also led to soil acidification, which was not effectively alleviated by the return of straw in combination with mineral fertilization.
Table 1Soil properties of bulk soil among six treatments.
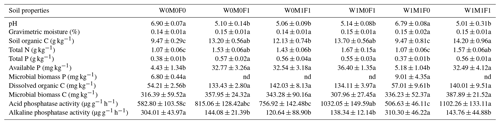
The six treatments were (1) the control treatment without straw retention and mineral fertilizer (W0M0F0), (2) single application of mineral fertilizer (W0M0F1), (3) maize straw retention combined with mineral fertilization (W0M1F1), (4) wheat straw retention combined with mineral fertilizer (W1M0F1), (5) both wheat and maize straw retention with no fertilizer (W1M1F0), and (6) both wheat and maize straw retention combined with mineral fertilizer (W1M1F1). Values are means ± standard error. The notation “nd” indicates that the microbial biomass P was not detected. Significant differences between treatments are indicated by different lowercase letters (p<0.05) in the results of the multiple comparisons following ANOVA. Treatments sharing the same letter are not significantly different from each other.
The WECs accounted for 9.73 %–11.05 % of bulk soils, and the proportions of WECs were not affected by mineral fertilization and straw retention (Fig. S1). Significantly higher concentrations of SOC, TN, TP, and available P were detected in WECs than those in bulk soils for the W0M0F1, W1M1F0, and W0M0F0 treatments (Fig. 1a–d). The influence of either mineral fertilization or straw retention on physicochemical properties of WECs was more remarkable than their effects on bulk soils. Organic C and total N contents in WECs experienced a substantial rise following the implementation of straw retention compared with the control, as depicted in Fig. 1a and b.
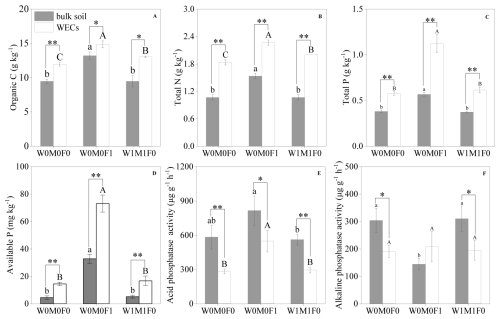
Figure 1Soil properties in bulk soil and water-extractable colloids (WECs) for the W0M0F0, W0M0F1, and W1M1F0 treatments. A: soil organic carbon (SOC), B: total nitrogen (N), C: total phosphorus (P), D: available phosphorus (P), E: acid phosphatase activity (ACP), F: alkaline phosphatase activity (ALP). Significant differences between treatments in bulk soil are indicated by lowercase letters (p< 0.05). Significant differences between treatments in WECs (< 2 µm) are indicated by capital letters (p< 0.05). Significant differences between bulk soil and WECs are as follows: * p< 0.05 and ** p< 0.01 (independent-sample t test).
3.2 P-bonding fractions in bulk soils and WECs
The concentrations of total inorganic P, Ca2-P, Ca8-P, Al-P, and Fe-P under straw retention with incorporated mineral fertilization increased remarkably by 128.93–146.99 mg kg−1, 15.41–17.30 mg kg−1, 3.19–4.38 mg kg−1, 59.74–68.97 mg kg−1, and 44.08–54.46 mg kg−1, respectively, compared with the control as shown in Table 2. Accordingly, marked increases in the proportion of Ca2-P, Ca8-P, Al-P, and Fe-P were observed, while the proportion of Ca10-P decreased remarkably (Fig. S4). These differences were mainly caused by mineral fertilization. There was also no significant difference between straw retention with incorporated mineral fertilization and solely mineral fertilization. The straw retention had little impact on the concentrations of each inorganic P fraction compared with the control.
According to the XANES analysis of WECs, there were notable increases in the proportions of Al-P and Fe-P, but remarkable decreases in the proportions of DCP and IHP were observed after mineral fertilization compared with the control (Table 3 and Fig. S5). However, straw retention brought slight increases in the proportions of Fe-P and IHP.
Table 2Concentrations (mg kg−1) of inorganic P fractions in bulk soil.

The six treatments were (1) the control treatment without straw retention and mineral fertilizer (W0M0F0), (2) single application of mineral fertilizer (W0M0F1), (3) maize straw retention combined with mineral fertilization (W0M1F1), (4) wheat straw retention combined with mineral fertilizer (W1M0F1), (5) both wheat and maize straw retention with no fertilizer (W1M1F0), and (6) both wheat and maize straw retention combined with mineral fertilizer (W1M1F1). Inorganic P fractions include calcium-bound P (Ca-P), aluminum-bound P (Al-P), iron-bound P (Fe-P), and occluded phosphate (O-P). Ca-P can be divided into dicalcium phosphate (Ca2-P), octacalcium phosphate (Ca8-P), and apatite (Ca10-P). Values in each column followed by different lowercase letters indicate significant differences (p<0.05).
Table 3Phosphorus K-edge XANES fitting results (%) showing the relative percent of each P species in water-extractable colloids (WECs) among the W0M0F1, W1M1F0, and W0M0F0 treatments.
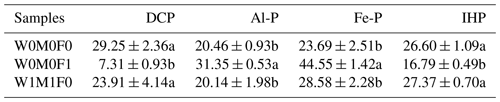
The three treatments were (1) the control treatment without straw retention and mineral fertilizer (W0M0F0), (2) single application of mineral fertilizer (W0M0F1), and (3) both wheat and maize straw retention with no fertilizer (W1M1F0). DCP: dibasic calcium phosphate dihydrate (DCP, CaHPO4•2H2O); Al-P: aluminum phosphate (AlPO4); Fe-P: iron phosphate dihydrate (FePO4•2H2O); IHP: inositol hexakisphosphate. Values in each column followed by different lowercase letters indicate significant differences (p<0.05).
3.3 Solution31P NMR analysis of bulk soils and WECs
The concentrations and proportions of orthophosphate in bulk soils increased by 146.4–182.6 mg kg−1 and 18.6 %–21.3 % under straw retention with incorporated mineral fertilization compared with solely straw retention and the control treatments (Table 4 and Fig. S6). Organic P concentrations also increased under mineral fertilization, among which orthophosphate monoesters and orthophosphate diesters increased by 12.78–27.00 mg kg−1 and 7.55–10.05 mg kg−1, respectively. Furthermore, the concentration of each P species in bulk soil showed no notable difference between straw retention with incorporated mineral fertilization treatments and solely mineral fertilization treatment (Table 4). In comparison with the control, the concentration of orthophosphate monoesters and orthophosphate diesters in bulk soil increased slightly under solely straw retention, but this difference was not statistically significant. These results make the effect of mineral fertilization on P-species concentration more apparent than that of straw retention.
Notably, the concentrations of orthophosphate, orthophosphate monoesters, orthophosphate diesters, and Glyc+nucl (i.e., α- and β-glycerophosphate and mononucleotides) in WECs were significantly greater (∼ 2.5 times) than those in bulk soil for all tested samples (Tables 4 and 5). Mineral fertilization had more significant effects on the concentrations of P species in WECs compared with those in bulk soils. Relative to the control, the concentrations of orthophosphate, orthophosphate monoesters, and orthophosphate diesters rose sharply after mineral fertilization for WECs, while a significant increase in only orthophosphate concentrations was detected for bulk soils. Furthermore, the concentration of these P species in WECs under solely straw retention increased slightly in comparison with the control (Table 5).
Table 4Concentrations (mg kg−1) of P species in bulk soil evaluated in the solution 31P NMR analysis.
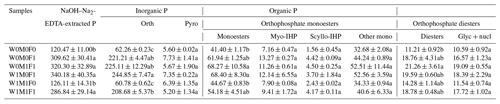
The six treatments were (1) the control treatment without straw retention and mineral fertilizer (W0M0F0), (2) single application of mineral fertilizer (W0M0F1), (3) maize straw retention combined with mineral fertilization (W0M1F1), (4) wheat straw retention combined with mineral fertilizer (W1M0F1), (5) both wheat and maize straw retention with no fertilizer (W1M1F0), and (6) both wheat and maize straw retention combined with mineral fertilizer (W1M1F1). Calculation was done by including diester degradation products (i.e., Glyc + nucl: α- and β-glycerophosphate and mononucleotides) with orthophosphate diesters (Diesters) rather than orthophosphate monoesters (Monoesters). Phosphorus compounds include orthophosphate (Orth), pyrophosphate (Pyro), myo-inositol hexakisphosphate (Myo-IHP), scylloinositol hexakisphosphate (Scyllo-IHP), other monoesters not specifically identified (Other mono), α- and β-glycerophosphate (Glyc), and mononucleotides (nucl). Values in each column followed by different lowercase letters indicate significant differences (p<0.05).
Table 5Concentrations (mg kg−1) of P species in water-extractable colloids (WECs) evaluated in the solution 31P NMR analysis among the W0M0F1, W1M1F0, and W0M0F0 treatments.

The three treatments were (1) the control treatment without straw retention and mineral fertilizer (W0M0F0), (2) single application of mineral fertilizer (W0M0F1), and (3) both wheat and maize straw retention with no fertilizer (W1M1F0). Calculation was done by including diester degradation products (i.e., Glyc+nucl: α- and β-glycerophosphate and mononucleotides) with orthophosphate diesters (Diesters) rather than orthophosphate monoesters (Monoesters). Phosphorus compounds include orthophosphate (Orth), pyrophosphate (Pyro), myo-inositol hexakisphosphate (Myo-IHP), scylloinositol hexakisphosphate (Scyllo-IHP), other monoesters not specifically identified (Other mono), α- and β-glycerophosphate (Glyc), and mononucleotides (nucl). Values in each column followed by different lowercase letters indicate significant differences (p<0.05).
3.4 Genes associated with P transformation in bulk soils and WECs
In bulk soils, there were remarkable decreases in total relative abundances of genes associated with P transformation under the combined application of straw retention and mineral fertilization compared with the control. These genes included those related to organic P mineralization (e.g., phoA, phoD, phy, and ugpQ), P-starvation regulation (e.g., phoR), and P uptake and transport (e.g., phnCDE) as described in Fig. 2a and b. No notable difference was observed in the abundances of these P-transformation genes in bulk soils between straw retention combined with mineral fertilization and solely mineral fertilization, but they were significantly different from those for solely straw retention. Correspondingly, the PCA results also revealed clear separations for the genes related to P cycling between treatments with (i.e., W0M0F1, W1M0F1, W0M1F1, and W1M1F1) and without (i.e., W0M0F0 and WM1F0) mineral fertilization (Fig. 3a).
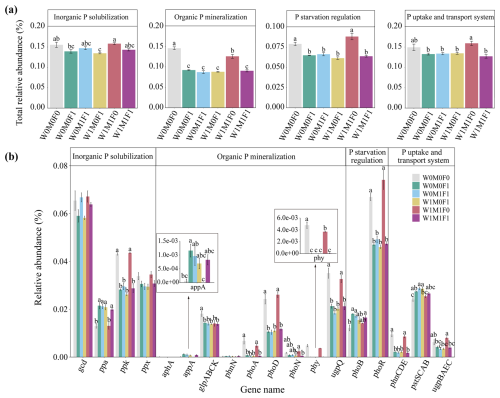
Figure 2Relative abundance of genes responsible for microbial inorganic P solubilization, organic P mineralization, P-starvation regulation, and P uptake and transport (a) as well as the individual gene relative abundance (b) in bulk soil. The relative abundances of genes were calculated related to the annotated reads. Significant differences between treatments in bulk soil are indicated by lowercase letters (p< 0.05). The relative abundance of glp transporter systems was calculated as the average abundances of the genes glpA, glpB, glpC, and glpK. The phn transporter system was calculated as the average abundances of the genes phnC, phnD, and phnE. The pst transporter system was calculated as the average abundances of the genes pstS, pstC, pstA, and pstB. The ugp transporter system was calculated as the average abundances of the genes ugpB, ugpA, ugpE, and ugpC.
The PCA (Fig. 3b) exhibited a clear segregation between the P-cycling genes in WECs and those in bulk soils for the W0M0F1, W1M1F0, and W0M0F0 treatments. Solely straw retention caused significant differences in relative abundance for many gene species including ppa, ppk, phoD, phoN, phy, phoR, phnCDE, and ugpBAEC between WECs and bulk soils. In contrast, solely mineral fertilization caused significant differences in fewer gene species including gcd, ppx, glpABCK, and phoR (Fig. 4b). These results suggested that straw retention caused a greater change in the P-cycling gene between WECs and bulk soils compared with mineral fertilization.
3.5 Taxonomic assignments of phoD and gcd genes
The phoD gene (encoding alkaline phosphatases) and gcd gene (encoding glucose dehydrogenase for synthesizing) serve as critical indicators of P mineralization and solubilization, respectively. As shown in Fig. 4, solely straw retention significantly increased the abundance of the phoD gene, whereas mineral fertilization significantly decreased the abundance of the gcd gene in WECs compared with bulk soils. Thus, we further performed taxonomic assignments of phoD and gcd genes.
For bacterial taxa containing the phoD gene in WECs (Fig. 5a), the abundance of Proteobacteria increased significantly under solely straw retention when compared to that in bulk soils. For bacterial taxa containing the gcd gene in WECs (Fig. 5b), the abundance of Acidobacteria decreased significantly compared with that in bulk soils under mineral fertilization. Additionally, the bacterial β diversity in WECs showed a clear divergence from that in bulk soils for all treatments (Fig. S7).
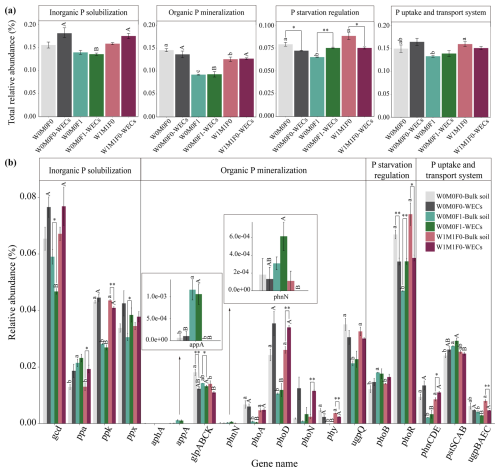
Figure 4Relative abundance of genes responsible for microbial inorganic P solubilization, organic P mineralization, P-starvation regulation, and P uptake and transport (a) as well as the individual gene relative abundance (b) in bulk soil and water-extractable colloids (WECs) among the W0M0F0, W0M0F1, and W1M1F0 treatments. The relative abundances of genes were calculated related to the annotated reads. Significant differences between treatments in bulk soil are indicated by lowercase letters (p< 0.05). Significant differences between treatments in WECs (< 2 µm) are indicated by capital letters (p< 0.05). Significant differences between bulk soil and WECs are as follows: * p< 0.05 and ** p< 0.01 (independent-sample t test). The relative abundance of glp transporter systems was calculated as the average abundances of the genes glpA, glpB, glpC, and glpK. The phn transporter system was calculated as the average abundances of the genes phnC, phnD, and phnE. The pst transporter system was calculated as the average abundances of the genes pstS, pstC, pstA, and pstB. The ugp transporter system was calculated as the average abundances of the genes ugpB, ugpA, ugpE, and ugpC.
3.6 Correlations between P-cycling genes and soil properties, P species in bulk soils, and WECs
According to Spearman's rank correlations (Fig. S8), more P-gene species were correlated with soil properties and nutrients in bulk soils than WECs (R > 0.6, p<0.05), suggesting that the responses of P-cycling genes to soil properties in bulk soil were more sensitive than those in WECs. Specially, a strong correlation was detected between the majority of P-cycling genes and soil nutrients including C, N, and P in bulk soils. In contrast, no consistent trends were observed in WECs.
According to Fig. 6, mineral fertilization influenced the P-cycling genes by decreasing soil pH and increasing total P in bulk soil. The model fit in bulk soil was GFI = 0.939 and RMSEA = 0.036. The chi-square statistic divided by degrees of freedom (chi-square df) was 1.8, which was less than 2 and indicated that the structural equation model (SEM) was a superior fit (Alavi et al., 2020). Furthermore, the decrease in soil pH positively affected the genes involved in organic P mineralization (0.82, p<0.01), and the increase in total P had a negative effect on the genes involved in P-starvation regulation (−0.77, p<0.01). In WECs, mineral fertilization affected the P-cycling genes by increasing total P (0.98, p<0.01) and organic C (0.92, p<0.01). The model fit in WECs was GFI = 0.964 and RMSEA = 0.000. Moreover, total P negatively affected the genes related to organic P mineralization (−0.67, p<0.01) and inorganic P solubilization (−0.69, p<0.05).
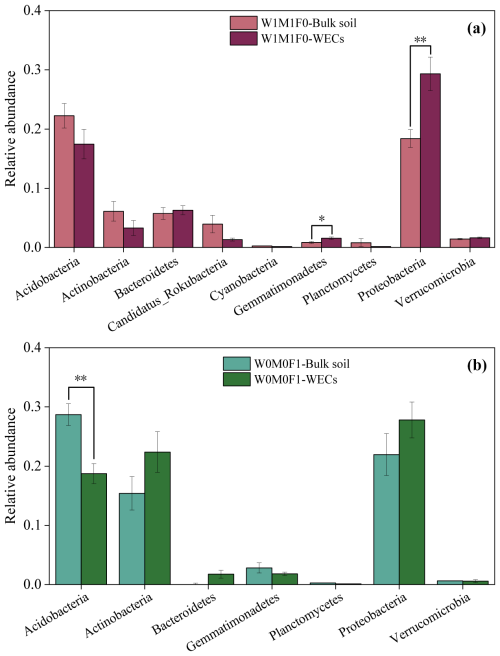
Figure 5Taxonomic assignments at the phylum level of the phoD gene for the W1M1F0 treatment (a) and the gcd gene for the W0M0F1 treatment (b) in bulk soil and water-extractable colloids (WECs).
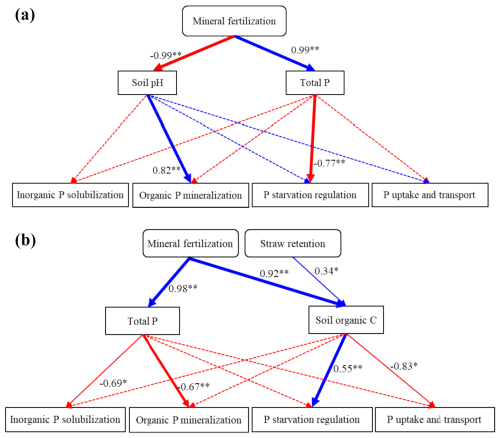
Figure 6Structural equation model (SEM) showing the relationship among mineral fertilization and straw retention, soil properties, and the P-cycling-related gene in bulk soil (a) as well as water-extractable colloids (WECs) (b). The solid blue and red arrows represent the significant positive and negative relationships between different variables. The dashed arrows represent nonsignificant relationships. The numbers near the blue and red arrows are the path coefficients. * p<0.05; p<0.01.
4.1 Mineral-fertilization-restricted genes involved in P transformation in bulk soils
In bulk soil, mineral fertilization decreased soil pH and increased soil TP, thus decreasing the abundances of P-transformation genes (Fig. 6). Soil acidification might be due to the increased proton release from nitrification processes occurring under mineral N fertilization (Guo et al., 2010). The significant increases in soil organic matter and nutrient concentrations under mineral fertilization might be closely associated with the enhanced organic matter from crop residues, root exudates, and the input of fertilizers (Tong et al., 2019).
Generally, the genes for P mineralization, P-starvation regulation, and P uptake and transport were primarily influenced by the environmental availability of P (Hsieh and Wanner, 2010; Richardson and Simpson, 2011). Under conditions of low soil P, microorganisms exhibited an upregulation of genes within the Pho regulon, specifically those encoding phosphatases and phosphate transporters (Vershinina and Znamenskaya, 2002). The expression of phoR and phoD was governed by the presence of P-starvation conditions (Xie et al., 2020). The phytase was inhibited by a high level of phosphate (Yao et al., 2018), and a higher abundance of phy (3-phytase) was observed in P-deficient soils compared to P-rich soils (Siles et al., 2022). The ugpQ gene also usually accumulated in P-starvation conditions as the operon of the glycerophosphodiester-utilizing system (Luo et al., 2009). Therefore, in the control and straw retention treatments with lower P concentrations, higher abundances of phoD, phy, phoR, and ugpQ genes were observed in comparison with the mineral fertilization treatments (Fig. 2). Consistent with previous findings (Ikoyi et al., 2018; Dai et al., 2020), mineral fertilization alone or combined with straw retention reduced the abundance of genes related to P mineralization (e.g., phoA, phoD, phy, ugpQ), P-starvation regulation (e.g., phoR), and P uptake and transport (e.g., phnCDE) significantly (Fig. 2).
Additionally, Chen et al. (2017) identified soil pH as the primary factor influencing the compositions of the microbial community harboring the phoD gene, noting a positive correlation between soil pH and the phoD gene abundance. Studies have provided evidence that a decrease in soil pH could inhibit bacterial and fungal growth (Li et al., 2020), modify microbial community compositions (Rousk et al., 2010), and decrease the relative abundances of Actinobacteria and Proteobacteria for the phoD gene (Luo et al., 2017), which in turn decreases P-mineralization capacity. In this study, Spearman's rank correlations showed that phoD, phoA, phy, ugpQ, and phoR gene abundances were correlated negatively with the contents of orthophosphate, orthophosphate monoesters, and orthophosphate diesters and positively with soil pH (p < 0.05) (Fig. S8a). Thus, the decline in the abundance of P-cycling-related genes (Fig. 2) can be attributed to increased soil P contents and low soil pH (Tables 1 and 4) under mineral fertilization compared with the control treatment.
In bulk soil, straw retention showed no significant impact on soil properties, P species, and transformation genes. Straw decomposition was affected by the composition of straw (e.g., , lignin, cellulose of straw) and soil characteristics (e.g., soil aeration, pH and nutrient contents). The high , lignin, and cellulose in wheat and maize straw might slow down straw decomposition (Talbot and Treseder, 2012). The values in wheat and maize straw (52–73:1) were significantly higher than microorganism C:N values suitable (25–30:1) for straw decomposition (Cai et al., 2018), indicating that microorganisms needed to consume soil original N when decomposing straw. Therefore, straw retention without N addition could limit the decomposition rate of straw. Thus, the straw retention for 13 years did not show any significant impact on soil C, N, and P nutrients (Table 1). Yet it is noteworthy that although the decomposition rate of straw was slow, it started to have slight effects on the accumulation of soil microorganism C and P in bulk soils (Table 1) and was expected to have a more obvious effect in the longer term. The slow decomposition of straw provided nutrients and promoted crop root exudation, consequently fostering the growth of the soil microbial community and augmenting soil MBC (Wang et al., 2021). The increase in MBC resulted in the increase in MBP (Spohn and Kuzyakov, 2013), as shown in Table 1. When N and P fertilizers were added, straw retention with incorporated mineral fertilization could enhance microbial activity, improve soil microbial and , promote straw decomposition, and increase organic C contents (Li et al., 2018). The input of N and P fertilizers brought significant increases in soil N and P contents (Zhang et al., 2018). In this study, straw retention with incorporated mineral fertilization brought remarkable decreases in soil pH and significant increases in soil nutrients, which was significantly different from solely straw retention. Solely straw retention showed minimal effects on soil properties, P species, and transformation genes in bulk soil. Interestingly, it started to have a notable influence on these indicators in the soil colloids (WECs), as discussed below.
4.2 Straw retention increased the abundances of the phoD gene and phoD-harboring Proteobacteria in WECs
The higher concentrations of SOC, TN, TP, AP, and various P species in WECs (Fig. 1 and Table 5) compared with bulk soil (Tables 1 and 4) indicated nutrient enrichment within the WECs. This could be caused by the higher specific surface area of the WECs (Jiang et al., 2014). Significant increases in these indicators suggested that the management practices exerted more substantial impacts on soil properties and P species in WECs than in bulk soils. This highlighted the heightened sensitivity of the physicochemical properties of soil microparticles to environmental disturbances compared to bulk soil. Soil colloids are the most active constituent, representing the micro-particulate phase of soils, and play a fundamental role in the cycling of P (Fresne et al., 2022). Previous studies demonstrated that colloids were the important vectors governing P mobility and bioavailability (Rick and Arai, 2011). According to de Jonge et al. (2004), colloidal P can make a substantial contribution to transportable P, amounting to as much as 75 % in arable soils. More inorganic and organic P accumulated in the WECs compared with bulk soils (Tables 4 and 5), which could improve the potential bioavailability and mobility of P (Krause et al., 2020). Notably, although the practice of straw retention did not result in any significant changes in nutrient contents in bulk soils, it brought significant increases in TN and SOC contents (Fig. 1a and b) and slight increases in the concentrations of TP and each P species for WECs. This indicated that straw retention promoted the accumulation of nutrients in WECs, which could enhance the supply and cycling of P.
Straw retention caused significant differences in relative abundances for more P-cycling genes between WECs and bulk soils than mineral fertilization (Fig. 4b) and led to a significant increase in the phoD gene in WECs compared with bulk soils. For bacterial taxa containing the phoD gene, the abundance of Proteobacteria (Fig. 5a) increased significantly in WECs compared with that in bulk soils under solely straw retention. This indicated that straw retention might increase the phoD gene abundance by influencing phoD-harboring Proteobacteria and then increase P-mineralizing capacity in WECs. Several studies have highlighted that Proteobacteria has been recognized as a crucial group of microorganisms involved in the mineralization of P (Zhang et al., 2023), and the increase in phoD-harboring Proteobacteria could improve potential P mineralization (Xie et al., 2020). Proteobacteria belong to copiotrophic microorganism groups and accumulate in nutrient-rich soils (Wang et al., 2022). Research conducted by Fierer et al. (2012) and Ling et al. (2014) has shown that higher concentrations of total N, P, and organic C could promote the growth of such microorganisms. In our research, the notable increases in SOC, TN, and each P species in WECs under straw retention likely created favorable conditions for the proliferation of copiotrophic bacteria (e.g., Proteobacteria). Generally, the WECs (clay particles), including natural organic matter (e.g., humus) and inorganic colloids (silicate and oxides) (Zhang et al., 2021), were considered to be the best natural microorganism adsorbents (Madumathi, 2017; Zhao et al., 2014). Previously conducted research has indicated that most bacteria (65 %) are associated with < 2 µm soil particulates (Oliver et al., 2007). The population of the bacteria (Pseudomonas putida) attached to clay particles in red soil (Ultisol) was significantly higher compared to the populations found on silt and sand particles (Wu et al., 2012). Furthermore, the increased SOC could improve the surface area and activity of WECs (Zhao et al., 2014), thus increasing microorganism adhesion (Van Gestel et al., 1996). SOC was a key component of P binding in colloids (Sun et al., 2023). Thus, we considered that the P-cycling microorganisms in soil colloids might be influenced by their characteristics and the increased nutrient contents of WECs under straw retention.
In this study, mineral fertilization also caused enhancements of SOC contents in WECs (Fig. 1), which positively influenced the abundance of P-cycling genes. However, it was also noted that mineral fertilization dramatically increased P contents and decreased soil pH by 1.76–1.89 units (Table 1), which restricted the expression and activity of P-cycling genes in both WECs and bulk soils, as discussed before. Therefore, the difference in P-cycling genes between WECs and bulk soil under mineral fertilization was less significant than under straw retention. Additionally, the consistent change trends of the gcd gene and gcd-harboring Acidobacteria indicated that the decrease in gcd gene abundance in WECs might be driven by the gcd-harboring Acidobacteria under mineral fertilization (Khan et al., 2007). The gcd gene coding the membrane-bound quinoprotein glucose dehydrogenase (PQQGDH) was involved in the regulation of the process of making inaccessible mineral P soluble, such as some rock phosphate, hydroxyapatite, and Ca phosphates. Wu et al. (2021) have shown that an increase in gcd-harboring Acidobacteria improved P solubilization. The Acidobacteria were acidophilic and oligotrophic bacteria. Most of their members lived in low-nutrient or high-acidity environments. The abundance of Acidobacteria was often negatively correlated with soil nutrient contents and pH (Rousk et al., 2010; Jones et al., 2009). As mentioned above, soil pH decreased significantly (Table 1), and this might lead to an increase in Acidobacteria in bulk soils after mineral fertilization. The WECs had a strong soil buffering capacity by the exchangeable ion, organic C, and clay particles (Curtin and Trolove, 2013) and could alleviate the pH change, which did not support the growth of Acidobacteria. The pH buffering capacity and greater nutrient contents in WECs might limit the expression of Acidobacteria compared with bulk soils under mineral fertilization, thus causing the significant decrease in gcd gene abundance in WECs compared with the bulk soil.
This study provides valuable insights into P speciation and the role of P-transformation microorganisms at the soil microparticle scale (WECs) in the context of straw retention and mineral fertilization. Our findings underscore the critical influence of these management practices on soil chemistry and microbial dynamics. The decrease in soil pH and increases in soil TP under mineral fertilization hinder the expression of genes related to P transformation in bulk soils, potentially limiting the efficiency of P cycling. In contrast, straw retention enhances the accumulation of organic C and total N on soil colloid scales significantly, thus causing a significant increase in the abundance of gene encoding for alkaline phosphatase (phoD) and phoD-harboring Proteobacteria for WECs. It indicates that straw retention could potentially improve P availability by increasing the P-mineralization capacity of WECs. This information provides innovative evidence that straw retention could potentially affect the turnover, mobility, and availability of P, mainly by changing the physicochemical and biochemical processes involved in the P transformation of soil colloids.
This study utilized existing statistical software and publicly available R packages for data analysis. All R packages used are freely available through the Comprehensive R Archive Network (CRAN) repository (https://CRAN.R-project.org/package=psych, Revelle, 2024). Details of the specific packages, versions, and statistical procedures used are described in the “Materials and methods” section.
The dataset used in this research can be accessed by contacting the corresponding author with a reasonable request.
The supplement related to this article is available online at: https://doi.org/10.5194/bg-22-135-2025-supplement.
XJ, JT, and SB designed and supervised the study. SB, YG, DY, YW, and SZ performed the experimental work. SB, YG, DY, YW, and YP conducted the data analysis. XJ, SB, YG, DY, and YW created the methodological approach. SB wrote the first draft, and all authors engaged in the revision process and discussions.
The contact author has declared that none of the authors has any competing interests.
Publisher’s note: Copernicus Publications remains neutral with regard to jurisdictional claims made in the text, published maps, institutional affiliations, or any other geographical representation in this paper. While Copernicus Publications makes every effort to include appropriate place names, the final responsibility lies with the authors.
The study was funded by the National Natural Science Foundation of China (no. 42377323).
The study was funded by the National Natural Science Foundation of China (no. 42377323).
This paper was edited by Lucia Fuchslueger and reviewed by two anonymous referees.
Alavi, M., Visentin, D. C., Thapa, D. K., Hunt, G. E., Watson, R., and Cleary, M.: Chi-square for model fit in confirmatory factor analysis, J. Adv. Nurs., 76, 2209–2211, https://doi.org/10.1111/jan.14399, 2020.
Audette, Y., O'Halloran, I. P., Evans, L. J., and Voroney, R. P.: Preliminary validation of a sequential fractionation method to study phosphorus chemistry in a calcareous soil, Chemosphere, 152, 369–375, https://doi.org/10.1016/j.chemosphere.2016.03.014, 2016.
Bai, S. S., Tan, J. F., Zhang, Z. Y., Wei, M., Zhang, H. M., and Jiang, X. Q.: Phosphorus speciation and colloidal phosphorus responses to short-term cessation of fertilization in a lime concretion black soil, Pedosphere, 33, 948–959, https://doi.org/10.1016/j.pedsph.2023.01.004, 2023.
Beauchemin, S., Hesterberg, D., Chou, J., Beauchemin, M., Simard, R. R., and Sayers, D. E.: Speciation of phosphorus in phosphorus-enriched agricultural soils using X-ray absorption near-edge structure spectroscopy and chemical fractionation, J. Environ. Qual., 32, 1809–1819, https://doi.org/10.2134/jeq2003.1809, 2003.
Bergkemper, F., Schöler, A., Engel, M., Lang, F., Krüger, J., Schloter, M., and Schulz, S.: Phosphorus depletion in forest soils shapes bacterial communities towards phosphorus recycling systems, Environ. Microbiol., 18, 1988–2000, https://doi.org/10.1111/1462-2920.13188, 2016.
Brookes, P. C., Powlson, D. S., and Jenkinson, D. S.: Measurement of microbial biomass phosphorus in soil, Soil Biol. Biochem., 14, 319–329, https://doi.org/10.1016/0038-0717(82)90001-3, 1982.
Buchfink, B., Xie, C., and Huson, D. H.: Fast and sensitive protein alignment using DIAMOND, Nat. Methods, 12, 59–60, https://doi.org/10.1038/nmeth.3176, 2015.
Cade-Menun, B. and Liu, C. W.: Solution Phosphorus-31 Nuclear Magnetic Resonance Spectroscopy of Soils from 2005 to 2013: A Review of Sample Preparation and Experimental Parameters, Soil Sci. Soc. Am. J., 78, 19–37, https://doi.org/10.2136/sssaj2013.05.0187dgs, 2014.
Cade-Menun, B. J., Carter, M. R., James, D. C., and Liu, C. W.: Phosphorus forms and chemistry in the soil profile under long-term conservation tillage: a phosphorus-31 nuclear magnetic resonance study, J. Environ. Qual., 39, 1647–1656, https://doi.org/10.2134/jeq2009.0491, 2010.
Cai, A., Liang, G., Zhang, X., Zhang, W., Li, L., Rui, Y., Xu, M., and Luo, Y.: Long-term straw decomposition in agro-ecosystems described by a unified three-exponentiation equation with thermal time, Sci. Total Environ., 636, 699–708, https://doi.org/10.1016/j.scitotenv.2018.04.303, 2018.
Cao, D., Lan, Y., Sun, Q., Yang, X., Chen, W., Meng, J., Wang, D., and Li, N.: Maize straw and its biochar affect phosphorus distribution in soil aggregates and are beneficial for improving phosphorus availability along the soil profile, Eur. J. Soil Sci., 72, 2165–2179, https://doi.org/10.1111/ejss.13095, 2021.
Cao, N., Zhi, M., Zhao, W., Pang, J., Hu, W., Zhou, Z., and Meng, Y.: Straw retention combined with phosphorus fertilizer promotes soil phosphorus availability by enhancing soil P-related enzymes and the abundance of phoC and phoD genes, Soil Till. Res., 220, 105390, https://doi.org/10.1016/j.still.2022.105390, 2022.
Chai, R., Xu, Y., Cheng, Q., Wang, Q., Ma, C., Ye, X., Zhang, L., and Gao, H.: Nutrient resource quantity of main crop straw and utilization potential under straw returning in Anhui province, Sci. Agr. Sinica, 54, 95–109, 2021.
Chen, L., Li, F., Li, W., Ning, Q., Li, J. W., Zhang, J. B., Ma, D. H., and Zhang, C. Z.: Organic amendment mitigates the negative impacts of mineral fertilization on bacterial communities in shajiang black soil, Appl. Soil Ecol., 150, 103457, https://doi.org/10.1016/j.apsoil.2019.103457, 2020.
Chen, S., Zhou, Y., Chen, Y., and Gu, J.: Fastp: an ultra-fast all-in-one FASTQ preprocessor, Bioinformatics, 34, i884–i890, https://doi.org/10.1093/bioinformatics/bty560, 2018.
Chen, X. D., Jiang, N., Chen, Z. H., Tian, J. H., Sun, N., Xu, M. G., and Chen, L. J.: Response of soil phoD phosphatase gene to long-term combined applications of chemical fertilizers and organic materials, Appl. Soil Ecol., 119, 197–204, https://doi.org/10.1016/j.apsoil.2017.06.019, 2017.
Cheng, Z. B., Chen, Y., Gale, W. J., and Zhang, F. H.: Inorganic Phosphorus Distribution in Soil Aggregates Under Different Cropping Patterns in Northwest China, J. Soil Sci. Plant Nutr., 19, 157–165, https://doi.org/10.1007/s42729-019-00022-1, 2019.
Curtin, D. and Trolove, S.: Predicting pH buffering capacity of New Zealand soils from organic matter content and mineral characteristics, Soil Res., 51, 494–502, https://doi.org/10.1071/SR13137, 2013.
Dai, Z. M., Liu, G. F., Chen, H. H., Chen, C. R., Wang, J. K., Ai, S. Y., Wei, D., Li, D. M., Ma, B., Tang, C. X., Brookes, P. C., and Xu, J. M.: Long-term nutrient inputs shift soil microbial functional profiles of phosphorus cycling in diverse agroecosystems, The ISME J/, 14, 757–770, https://doi.org/10.1038/s41396-019-0567-9, 2020.
Damon, P. M., Bowden, B., Rose, T., and Rengel, Z.: Crop residue contributions to phosphorus pools in agricultural soils: A review, Soil Biol. Biochem., 74, 127–137, https://doi.org/10.1016/j.soilbio.2014.03.003, 2014.
de Jonge, L. W., Moldrup, P., Rubaek, G. H., Schelde, K., and Djurhuus, J.: Particle leaching and particle-facilitated transport of phosphorus at field scale, Vadose Zone J., 3, 462–470, 2004.
Deng, X., Xu, T. L., Dong, W. W., Zhang, Q., and Liang, Y. J.: Distribution of Organic Phosphorus in Soil Aggregates from Apple-Pear Orchard of China, Eur. Soil Sci., 54, 72–79, https://doi.org/10.1134/s1064229321010038, 2021.
Doolette, A. L., Smernik, R. J., and Dougherty, W. J.: Spiking improved solution phosphorus-31 nuclear magnetic resonance identification of soil phosphorus compounds, Soil Sci. Soc. Am. J., 73, 919–927, https://doi.org/10.2136/sssaj2008.0192, 2009.
Fierer, N., Lauber, C. L., Ramirez, K. S., Zaneveld, J., Bradford, M. A., and Knight, R.: Comparative metagenomic, phylogenetic and physiological analyses of soil microbial communities across nitrogen gradients, ISME Journal, 6, 1007–1017, https://doi.org/10.1038/ismej.2011.159, 2012.
Fresne, M., Jordan, P., Daly, K., Fenton, O., and Mellander, P.-E.: The role of colloids and other fractions in the below-ground delivery of phosphorus from agricultural hillslopes to streams, Catena, 208, 105735, https://doi.org/10.1016/j.catena.2021.105735, 2022.
Guo, J. H., Liu, X. J., Zhang, Y., Shen, J. L., Han, W. X., Zhang, W. F., Christie, P., Goulding, K. W. T., Vitousek, P. M., and Zhang, F. S.: Significant acidification in major Chinese croplands, Science, 327, 1008–1010, https://doi.org/10.1126/science.1182570, 2010.
Guo, Z. C., Li, W., Ul Islam, M., Wang, Y. K., Zhang, Z. B., and Peng, X. H.: Nitrogen fertilization degrades soil aggregation by increasing ammonium ions and decreasing biological binding agents on a Vertisol after 12 years, Pedosphere, 32, 629–636, https://doi.org/10.1016/s1002-0160(21)60091-7, 2022.
Hsieh, Y.-J. and Wanner, B. L.: Global regulation by the seven-component Pi signaling system, Curr. Opinion Microbiol., 13, 198–203, https://doi.org/10.1016/j.mib.2010.01.014, 2010.
Huson, D. H., Beier, S., Flade, I., Górska, A., El-Hadidi, M., Mitra, S., Ruscheweyh, H.-J., and Tappu, R.: MEGAN Community Edition – Interactive Exploration and Analysis of Large-Scale Microbiome Sequencing Data, PLoS Comput. Biol., 12, e1004957, https://doi.org/10.1371/journal.pcbi.1004957, 2016.
Hyatt, D., Chen, G.-L., LoCascio, P. F., Land, M. L., Larimer, F. W., and Hauser, L. J.: Prodigal: prokaryotic gene recognition and translation initiation site identification, BMC Bioinformatics, 11, 119, https://doi.org/10.1186/1471-2105-11-119, 2010.
Ikoyi, I., Fowler, A., and Schmalenberger, A.: One-time phosphate fertilizer application to grassland columns modifies the soil microbiota and limits its role in ecosystem services, Sci. Total Environ., 630, 849–858, https://doi.org/10.1016/j.scitotenv.2018.02.263, 2018.
Jiang, B. F. and Gu, Y. C.: A suggested fractionation scheme of inorganic phosphorus in calcareous soils, Fertil. Res., 20, 159–165, https://doi.org/10.1007/BF01054551, 1989.
Jiang, C. L., Séquaris, J.-M., Wacha, A., Bóta, A., Vereecken, H., and Klumpp, E.: Effect of metal oxide on surface area and pore size of water-dispersible colloids from three German silt loam topsoils, Geoderma, 235–236, 260–270, https://doi.org/10.1016/j.geoderma.2014.07.017, 2014.
Jiang, X., Bol, R., Willbold, S., Vereecken, H., and Klumpp, E.: Speciation and distribution of P associated with Fe and Al oxides in aggregate-sized fraction of an arable soil, Biogeosciences, 12, 6443–6452, https://doi.org/10.5194/bg-12-6443-2015, 2015.
Jiang, X. Q., Amelung, W., Cade-Menun, B. J., Bol, R., Willbold, S., Cao, Z. H., and Klumpp, E.: Soil organic phosphorus transformations during 2000 years of paddy-rice and non-paddy management in the Yangtze River Delta, China, Sci. Rep., 7, 1–12, https://doi.org/10.1038/s41598-017-10071-0, 2017.
Jiang, X. Q., Wulf, A., Bol, R., and Klumpp, E.: Phosphorus content in water extractable soil colloids over a 2000 years chronosequence of paddy-rice management in the Yangtze River Delta, China, Geoderma, 430, 116296, https://doi.org/10.1016/j.geoderma.2022.116296, 2023.
Jones, R. T., Robeson, M. S., Lauber, C. L., Hamady, M., Knight, R., and Fierer, N.: A comprehensive survey of soil acidobacterial diversity using pyrosequencing and clone library analyses, ISME J., 3, 442–453, https://doi.org/10.1038/ismej.2008.127, 2009.
Ju, W., Fang, L., Shen, G., Delgado-Baquerizo, M., Chen, J., Zhou, G., Ma, D., Bing, H., Liu, L., Liu, J., Jin, X., Guo, L., Tan, W., and Blagodatskaya, E.: New perspectives on microbiome and nutrient sequestration in soil aggregates during long-term grazing exclusion, Global Change Biol., 30, e17027, https://doi.org/10.1111/gcb.17027, 2024.
Kanehisa, M.: Toward understanding the origin and evolution of cellular organisms, Protein Sci., 28, 1947–1951, https://doi.org/10.1002/pro.3715, 2019.
Kanehisa, M. and Goto, S.: KEGG: Kyoto Encyclopedia of Genes and Genomes, Nucleic Acids Research, J. Nucleic. Acids Res., 28, 27–30, https://doi.org/10.1093/nar/28.1.27, 2000.
Khan, M. S., Zaidi, A., and Wani, P. A.: Role of phosphate-solubilizing microorganisms in sustainable agriculture – A review, Agr. Sustain. Develop., 27, 29–43, https://doi.org/10.1051/agro:2006011, 2007.
Krause, L., Klumpp, E., Nofz, I., Missong, A., Amelung, W., and Siebers, N.: Colloidal iron and organic carbon control soil aggregate formation and stability in arable Luvisols, Geoderma, 374, 114421, https://doi.org/10.1016/j.geoderma.2020.114421, 2020.
Lê, S., Josse, J., and Husson, F.: FactoMineR: An R Package for Multivariate Analysis, J. Stat. Softw., 25, 1–18, https://doi.org/10.18637/jss.v025.i01, 2008.
Li, C. Y., Hao, Y. h., Xue, Y. L., Wang, Y., and Dang, T. H.: Effects of long-term fertilization on soil microbial biomass carbon, nitrogen, and phosphorus in the farmland of the Loess Plateau, China, J. Agro-Environ. Sci., 39, 1783–1791, 2020.
Li, D., Liu, C.-M., Luo, R., Sadakane, K., and Lam, T.-W.: MEGAHIT: an ultra-fast single-node solution for large and complex metagenomics assembly via succinct de Bruijn graph, Bioinformatics, 31, 1674–1676, https://doi.org/10.1093/bioinformatics/btv033, 2015.
Li, L.-J., Zhu-Barker, X., Ye, R., Doane, T. A., and Horwath, W. R.: Soil microbial biomass size and soil carbon influence the priming effect from carbon inputs depending on nitrogen availability, Soil Biol. Biochem., 119, 41–49, https://doi.org/10.1016/j.soilbio.2018.01.003, 2018.
Ling, N., Sun, Y., Ma, J., Guo, J., Zhu, P., Peng, C., Yu, G., Ran, W., Guo, S., and Shen, Q.: Response of the bacterial diversity and soil enzyme activity in particle-size fractions of Mollisol after different fertilization in a long-term experiment, Biol. Fertil. Soils, 50, 901–911, https://doi.org/10.1007/s00374-014-0911-1, 2014.
Luo, G., Ling, N., Nannipieri, P., Chen, H., Raza, W., Wang, M., Guo, S., and Shen, Q.: Long-term fertilisation regimes affect the composition of the alkaline phosphomonoesterase encoding microbial community of a vertisol and its derivative soil fractions, Biol. Fertil. Soils, 53, 375–388, https://doi.org/10.1007/s00374-017-1183-3, 2017.
Luo, H., Benner, R., Long, R. A., and Hu, J.: Subcellular localization of marine bacterial alkaline phosphatases, P. Natl. Acad. Sci. USA, 106, 21219–21223, https://doi.org/10.1073/pnas.0907586106, 2009.
Ma, L., Guo, Z. B., Wang, D. Z., and Zhao, B. Z.: Effect of long-term application of phosphorus fertilizer on soil bacterial community structure and enzymatic activity in lime concretion black soil, Acta Pedol. Sinica, 56, 1459–1470, 2019.
Madumathi, G.: Transport of E. coli in presence of naturally occuring colloids in saturated porous media, Water Conserv. Sci. Eng., 2, 153–164, https://doi.org/10.1007/s41101-017-0037-z, 2017.
Missong, A., Holzmann, S., Bol, R., Nischwitz, V., Puhlmann, H., v. Wilpert, K., Siemens, J., and Klumpp, E.: Leaching of natural colloids from forest topsoils and their relevance for phosphorus mobility, Sci. Total Environ., 634, 305–315, https://doi.org/10.1016/j.scitotenv.2018.03.265, 2018.
Montavo, D., Degryse, F., and McLaughlin, M. J.: Natural colloidal P and its contribution to plant P uptake, Environ. Sci. Technol., 49, 3427–3434, https://doi.org/10.1021/es504643f, 2015.
Murphy, J. and Riley, J. P.: A modified single solution method for the determination of phosphate in natural waters, Anal. Chim. Acta, 27, 31–36, https://doi.org/10.1016/S0003-2670(00)88444-5, 1962.
Neal, A. L., Rossmann, M., Brearley, C., Akkari, E., Guyomar, C., Clark, I. M., Allen, E., and Hirsch, P. R.: Land-use influences phosphatase gene microdiversity in soils, Environ. Microbiol., 19, 2740–2753, https://doi.org/10.1111/1462-2920.13778, 2017.
Oksanen, J., Simpson, G., Blanchet, F., Kindt, R., Legendre, P., Minchin, P., O'Hara, R, Solymos, P., Stevens, M., Szoecs, E., Wagner, H., Barbour, M., Bedward, M., Bolker, B., Borcard, D., Borman, T., Carvalho, G., Chirico, M., De Caceres, M., Durand, S., Evangelista, H., FitzJohn, R., Friendly, M., Furneaux, B., Hannigan, G., Hill, M., Lahti, L., McGlinn, D., Ouellette, M., Ribeiro Cunha, E., Smith, T., Stier, A., Ter Braak, C., and Weedon, J.: vegan: Community Ecology Package. R package version 2.7-0, https://github.com/vegandevs/vegan, https://vegandevs.github.io/vegan/ (last access: 11 September 2023), 2024.
Oliver, D. M., Clegg, C. D., Heathwaite, A. L., and Haygarth, P. M.: Preferential attachment of escherichia coli to different particle size fractions of an agricultural grassland soil, Water Air Soil Pollut., 185, 369–375, https://doi.org/10.1007/s11270-007-9451-8, 2007.
Olsen, S. R. and Sommers, L. E.: Determination of available phosphorus, Agronomy, 02, 403–430, 1982.
Paradis, E. and Schliep, K.: ape 5.0: an environment for modern phylogenetics and evolutionary analyses in R, Bioinformatics, 35, 526–528, https://doi.org/10.1093/bioinformatics/bty633, 2019.
Ranatunga, T. D., Reddy, S. S., and Taylor, R. W.: Phosphorus distribution in soil aggregate size fractions in a poultry litter applied soil and potential environmental impacts, Geoderma, 192, 446–452, https://doi.org/10.1016/j.geoderma.2012.08.026, 2013.
Revelle, W.: psych: Procedures for Psychological, Psychometric, and Personality Research, R Package Version 2.4.3, Evanston, Illinois, https://CRAN.R-project.org/package=psych (last access: 5 September 2023), 2024.
Richardson, A. E. and Simpson, R. J.: Soil microorganisms mediating phosphorus availability update on microbial phosphorus, Plant Physiol., 156, 989–996, 2011.
Rick, A. R. and Arai, Y.: Role of natural nanoparticles in phosphorus transport processes in ultisols, Soil Sci. Soc. Am. J., 75, 335–347, https://doi.org/10.2136/sssaj2010.0124nps, 2011.
Rousk, J., Bååth, E., Brookes, P. C., Lauber, C. L., Lozupone, C., Caporaso, J. G., Knight, R., and Fierer, N.: Soil bacterial and fungal communities across a pH gradient in an arable soil, The ISME J., 4, 1340–1351, https://doi.org/10.1038/ismej.2010.58, 2010.
Schumacher, B.: Methods for the determination of total organic carbon (TOC) in soils and sediments, Ecological Risk Assessment Support Center Office of Research and Development, US. Environmental Protection Agency, 1–123, 2002.
Sequaris, J. M. and Lewandowski, H.: Physicochemical characterization of potential colloids from agricultural topsoils, Colloid. Surfaces A, 217, 93–99, https://doi.org/10.1016/s0927-7757(02)00563-0, 2003.
Siles, J. A., Starke, R., Martinovic, T., Fernandes, M. L. P., Orgiazzi, A., and Bastida, F.: Distribution of phosphorus cycling genes across land uses and microbial taxonomic groups based on metagenome and genome mining, Soil Biol. Biochem., 174, 108826, https://doi.org/10.1016/j.soilbio.2022.108826, 2022.
Spohn, M. and Kuzyakov, Y.: Phosphorus mineralization can be driven by microbial need for carbon, Soil Biol. Biochem., 61, 69–75, https://doi.org/10.1016/j.soilbio.2013.02.013, 2013.
Staff, S. S.: Keys to soil taxonomy, in: Keys to Soil Taxonomy (13th Edn.), United States Department of Agriculture Natural Resources Conservation Service, Washington, DC., 343–354 pp., 2022.
Sun, X. L., Matthias May, S., Amelung, W., Tang, N., Brill, D., Arenas-Díaz, F., Contreras, D., Fuentes, B., Bol, R., and Klumpp, E.: Water-dispersible colloids distribution along an alluvial fan transect in hyper-arid Atacama Desert, Geoderma, 438, 116650, https://doi.org/10.1016/j.geoderma.2023.116650, 2023.
Tabatabai, M. A. and Bremner, J. M.: Use of p-nitrophenyl phosphate for assay of soil phosphatase activity, Soil Biol. Biochem., 1, 301–307, https://doi.org/10.1016/0038-0717(69)90012-1, 1969.
Talbot, J. M. and Treseder, K. K.: Interactions among lignin, cellulose, and nitrogen drive litter chemistry–decay relationships, Ecology, 93, 345–354, https://doi.org/10.1890/11-0843.1, 2012.
Tong, Z. Y., Quan, G. L., Wan, L. Q., He, F., and Li, X. L.: The effect of fertilizers on biomass and biodiversity on a semi-arid grassland of northern China, Sustainability, 11, 2854, 2019.
Totsche, K. U., Amelung, W., Gerzabek, M. H., Guggenberger, G., Klumpp, E., Knief, C., Lehndorff, E., Mikutta, R., Peth, S., Prechtel, A., Ray, N., and Kögel-Knabner, I.: Microaggregates in soils, J. Plant Nutrit. Soil Sci., 181, 104–136, https://doi.org/10.1002/jpln.201600451, 2018.
Turner, B. L.: Soil organic phosphorus in tropical forests: an assessment of the NaOH–EDTA extraction procedure for quantitative analysis by solution 31P NMR spectroscopy, Eur. J. Soil Sci., 59, 453–466, https://doi.org/10.1111/j.1365-2389.2007.00994.x, 2008.
Van Gestel, M., Merckx, R., and Vlassak, K.: Spatial distribution of microbial biomass in microaggregates of a silty-loam soil and the relation with the resistance of microorganisms to soil drying, Soil Biol. Biochem., 28, 503–510, https://doi.org/10.1016/0038-0717(95)00192-1, 1996.
Vance, E. D., Brookes, P. C., and Jenkinson, D. S.: An extraction method for measuring soil microbial biomass C, Soil Biol. Biochem., 19, 703–707, https://doi.org/10.1016/0038-0717(87)90052-6, 1987.
Vershinina, O. A. and Znamenskaya, L. V.: The pho regulons of bacteria, Microbiology, 71, 497–511, https://doi.org/10.1023/A:1020547616096, 2002.
Wang, M. M., Wu, Y. C., Zhao, J. Y., Liu, Y., Chen, Z., Tang, Z. Y., Tian, W., Xi, Y. G., and Zhang, J. B.: Long-term fertilization lowers the alkaline phosphatase activity by impacting the phoD-harboring bacterial community in rice-winter wheat rotation system, Science The Total Environ., 821, 153406, https://doi.org/10.1016/j.scitotenv.2022.153406, 2022.
Wang, Q. J., Cao, X., Jiang, H., and Guo, Z. H.: Straw application and soil microbial biomass carbon change: a meta-analysis, Clean – Soil Air Water, 49, 2000386, https://doi.org/10.1002/clen.202000386, 2021.
Wu, H., Jiang, D., Cai, P., Rong, X., Dai, K., Liang, W., and Huang, Q.: Adsorption of Pseudomonas putida on soil particle size fractions: effects of solution chemistry and organic matter, J. Soils Sediment., 12, 143–149, https://doi.org/10.1007/s11368-011-0441-5, 2012.
Wu, L., Zhang, W. J., Wei, W. J., He, Z. L., Kuzyakov, Y., Bol, R., and Hu, R. G.: Soil organic matter priming and carbon balance after straw addition is regulated by long-term fertilization, Soil Biol. Biochem., 135, 383–391, https://doi.org/10.1016/j.soilbio.2019.06.003, 2019.
Wu, X., Peng, J., Liu, P., Bei, Q., Rensing, C., Li, Y., Yuan, H., Liesack, W., Zhang, F., and Cui, Z.: Metagenomic insights into nitrogen and phosphorus cycling at the soil aggregate scale driven by organic material amendments, Sci. Total Environ., 785, 147329, https://doi.org/10.1016/j.scitotenv.2021.147329, 2021.
Xie, Y. Y., Wang, F. H., Wang, K., Yue, H. Z., and Lan, X. F.: Responses of bacterial phoD gene abundance and diversity to crop rotation and feedbacks to phosphorus uptake in wheat, Appl. Soil Ecol., 154, 103604, https://doi.org/10.1016/j.apsoil.2020.103604, 2020.
Xu, Y., Chen, X., Wang, Q. Y., Luo, L. C., Zhang, C. C., Li, J. C., Ye, X. X., Gao, H. J., and Chai, R. S.: Effects of long-term wheat and maize straw incorporation on phosphorus fractions in lime concretion black soil, J. Agro-Environ. Sci., 41, 1768–1777, 2022.
Yao, Q. M., Li, Z., Song, Y., Wright, S. J., Guo, X., Tringe, S. G., Tfaily, M. M., Paša-Toliæ, L., Hazen, T. C., Turner, B. L., Mayes, M. A., and Pan, C.: Community proteogenomics reveals the systemic impact of phosphorus availability on microbial functions in tropical soil, Nat. Ecol. Evolut., 2, 499–509, https://doi.org/10.1038/s41559-017-0463-5, 2018.
Zhang, L., Liu, H. H., Sun, J. Q., Li, J. C., and Song, Y. H.: Seedling characteristics and grain yield of maize grown under straw retention affected by sowing irrigation and splitting nitrogen use, Field Crops Res., 225, 22–31, https://doi.org/10.1016/j.fcr.2018.05.016, 2018.
Zhang, Q., Bol, R., Amelung, W., Missong, A., Siemens, J., Mulder, I., Willbold, S., Müller, C., Westphal Muniz, A., and Klumpp, E.: Water dispersible colloids and related nutrient availability in Amazonian Terra Preta soils, Geoderma, 397, 115103, https://doi.org/10.1016/j.geoderma.2021.115103, 2021.
Zhang, Y., Gao, W., Ma, L., Luan, H., Tang, J., Li, R., Li, M., Huang, S., and Wang, L.: Long-term partial substitution of chemical fertilizer by organic amendments influences soil microbial functional diversity of phosphorus cycling and improves phosphorus availability in greenhouse vegetable production, Agr. Ecosyst. Environ., 341, 108193, https://doi.org/10.1016/j.agee.2022.108193, 2023.
Zhao, Q. L., Xin, C. Y., Wang, Y., Wang, J., Liu, Q. H., Li, J. L., and Ma, J. Q.: Characteristics of inorganic phosphorus in lime concretion black soil under continuous straw-return and fertilization in a rice-wheat rotation area, Acta Pratacult. Sinica, 27, 58–68, 2018.
Zhao, W., Walker, S. L., Huang, Q., and Cai, P.: Adhesion of bacterial pathogens to soil colloidal particles: Influences of cell type, natural organic matter, and solution chemistry, Water Res., 53, 35–46, https://doi.org/10.1016/j.watres.2014.01.009, 2014.