the Creative Commons Attribution 4.0 License.
the Creative Commons Attribution 4.0 License.
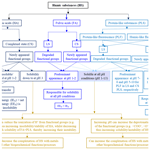
Solubility characteristics of soil humic substances as a function of pH: mechanisms and biogeochemical perspectives
Xuemei Yang
Jie Zhang
Mohammad Mohinuzzaman
H. Henry Teng
Nicola Senesi
Giorgio S. Senesi
Jie Yuan
Yu Liu
Si-Liang Li
Xiaodong Li
Baoli Wang
Cong-Qiang Liu
Soil humic substances (HSs) typically alter their electrochemical behaviours in the pH range of 1–12, which simultaneously regulates the stability of organo-minerals by modifying the HS functionalities. This process facilitates both biotic and abiotic transformations, which consequently leads to the export of degradative byproducts (e.g. HS components, nutrients) from soils into surrounding aquatic environments through water and/or rainwater discharges. However, the solubility features, environmental consequences, and mechanisms of HSs, including humic acids (HAs), fulvic acids (FAs), and protein-like substances (PLSs), under different pHs remain unclear. To respond to these issues, we used two soil extracts which were fractionated in the pH range from 12–1. The pH-dependent presence or absence of fluorescence peaks in the individual HS components reflected their functional group proton/electron exchange features at both low and high pH values, which were related to their solubility or insolubility. In particular, alkaline pH (≥pH 9) yielded the anionic forms ( and ) of phenolic OH and carboxyl groups of HACS, resulting in decreased electron/proton transfer from HS functionalities, as indicated by the decline of fluorescence peak maxima, whereas the protonic functionalities (e.g. –COOH, –OH) of HSs at lower pH resulted in the formation of highly available and remaining uncomplexed HS forms. The solubility of HA fractions increases with increasing pH, whereas their insolubility increases with decreasing pH, which determines their initial precipitation at pH 6 and final precipitation at pH 1, amounting approximately to 39.1 %–49.2 % and 3.1 %–24.1 % of the total dissolved organic matter (DOM), respectively, in the two soils. Elemental analysis results demonstrated that the C and N contents of HALS-pH 6 were lower and that those of O, S, and H were higher than those of HACS-pH 6, suggesting the preservation of C and N without S acquisition in HACS-pH 6, possibly because of their being complexed with minerals, which, in turn, would determine the insolubility of the HACS-pH 6 fraction. FACS + PLSCS showed relatively higher C and S contents and lower O% with respect to FALS + PLSLS, implying that FACS + PLSCS would remain under mineral protection. Fourier transform infrared (FTIR) results show significantly reduced infrared absorptions (e.g. 3300–3600 and 800–1200 cm−1) of HACS-pH 6 with respect to HALS-pH 6, suggesting the existence of strong intermolecular interactions among HA functional groups, possibly due to insoluble forms originally complexed with minerals. However, FALS + PLSLS exhibited stronger bands at 3414–3429 and 1008–1018 cm−1 than FACS + PLSCS, implying a strong interaction among functional groups possibly derived from various organo-mineral complexes in FACS + PLSCS. These results would indicate that HS insolubility arises via organo-metal and organo-mineral interactions at alkaline pH, along with HApH 6 insolubility via rainwater/water discharge, whereas HApH 2 + FA + PLS appears to be soluble at acidic pH, thereby being transported in ambient waters via rainwater/water discharge and groundwater infiltration. Therefore, the pH-dependent behaviour of soil HSs greatly contributes to a better understanding of the progressive transformation, mobility/transportation, and immobility/accumulation of HS components under various environmental conditions, with relevant implications for sustainable soil management practices and soil DOM dynamics.
- Article
(2131 KB) - Full-text XML
-
Supplement
(878 KB) - BibTeX
- EndNote
Soil organic matter (SOM), especially its more chemically active components, humic substances (HSs), is particularly important because it plays a number of fundamental roles, including the control of soil fertility, climate regulation and ecosystem stability (Harden et al., 2018), plant mineral nutrition and growth (Canellas and Olivares, 2014; Schmidt et al., 2007; Trevisan et al., 2010), adsorption/desorption of trace metals and radionuclides (Boguta et al., 2019; Bryan et al., 2012; Chou et al., 2018), and soil structural stability and porosity (Bronick and Lal, 2005; Senesi and Plaza, 2007). Loss of soil organic carbon (SOC) is due to several biotic and abiotic processes (Crowther et al., 2016; Huang and Hall, 2017), including heterotrophic respiration (Bond-Lamberty and Thomson, 2010; Heitmann et al., 2007; Klüpfel et al., 2014; Huang and Hall, 2017) and increasing temperatures due to climate change (Davidson and Janssens, 2006). SOC loss is also affected by soil erosion caused by deforestation, tillage, and other natural degradation processes, including hillslopes, salinization, waterlogging, and wildfires (Ellerbrock et al., 2016; Peinemann et al., 2005; Steinmuller and Chambers, 2019; De la Rosa et al., 2012; Drake et al., 2019). In general, HSs are divided according to their water solubility at various pH values into humic acids (HAs), which are insoluble at pH<2; fulvic acids (FAs) and protein-like substances (PLSs), which are soluble under both acidic and alkaline conditions; and humin, which is insoluble at any pH (Zhang et al., 2023; Senesi and Loffredo, 1999; Mohinuzzaman et al., 2020).
Three-dimensional (3D) fluorescence excitation–emission matrix (EEM) spectroscopy (3D EEMS) is a precise, rapid, and relatively simple technique for measuring filtered environmental surface waters and samples extracted from soils and sediments (Senesi, 1990a; Coble et al., 1990; Coble, 1996; Stedmon et al., 2003; Mostofa et al., 2013; Mohinuzzaman et al., 2020). In particular, this technique allows the characterization of fluorescent components, including soil HSs, autochthonous humic-like substances, PLSs, detergent-like substances, and others, without the need for further pretreatment of the samples (Senesi, 1990a; Coble et al., 1990; Coble, 1996; Stedmon et al., 2003; Mostofa et al., 2013). Recently, 3D EEM spectra combined with parallel factor (PARAFAC) analysis have been used to identify and characterize HA, FA, and PLSs (Stedmon et al., 2003; Gao et al., 2018b; Tadini et al., 2020; Mohinuzzaman et al., 2020). In particular, two typical protein-like fluorescence (PLF) peaks (T and TUV) and a minor component consisting of one or two fluorescence peaks (M and/or A) attributable to humic-like fluorescence (HLF) were identified in PLSs (Mohinuzzaman et al., 2020; Yang et al., 2024).
The HS solubility and insolubility mechanisms are associated with two key factors. Firstly, the soil pH, which varies widely in soils worldwide (Table S1 in the Supplement), influences the ionization level of HS functional groups. In particular, high pH values favour anionic forms, i.e. –COO− and –O− of carboxylic acids and phenolic/alcoholic groups and, consequently, the formation of metal-HS complexes, including insoluble ones (Brady and Weil, 2008; Kleber et al., 2007; Min et al., 2014; Dynarski et al., 2020; Kirsten et al., 2021; Zhang et al., 2023). In contrast, relatively low soil pH values favour protonic forms, such as –COOH and –OH of HS functionalities, which promote proton/electron exchange processes (Klapper et al., 2002; Nurmi and Tratnyek, 2002; Cory and McKnight, 2005; Yang et al., 2016; Wang et al., 2023). Furthermore, the zeta potential (ZP) of HA is minimal in the pH range 5–7, which is most likely caused by the dissociation of acidic functional groups that prevail at lower pH values, whereas disaggregation predominates over dissociation at higher pH values (Jovanović et al., 2013). Secondly, significant variability in the pH of rainwater (Table S2) or any inflowing water can affect both the solubility/transport/mobility and insolubility/immobilization/accumulation of soil HSs. Thus, understanding the solubility/insolubility of SOM/HSs under changing pH conditions is important for understanding the global C cycle.
Earlier studies (Hemingway et al., 2019; Lützow et al., 2006; Marschner et al., 2008; Sollins et al., 1996; Vogel et al., 2014) have not paid much attention to these issues when assessing the solubility and insolubility of SOM/HSs. For example, pH effects were studied to assess the interaction mechanisms of Fe(II) ions with soil HA at pH values of 5 and 7 (Boguta et al., 2019); the binding of Cu and Pb to HA and FA at pH 4–8 (Christl et al., 2005); Cu(II) binding properties of soil FA at pH 7.0 (dos Santos et al., 2020); coagulation mechanisms of HA in metal ion solutions at pH 4.6–7.0 (Ai et al., 2020); coagulation behaviours of HA in Na+ and Mg2+solutions at pH 3.6, 7.1, and 10.0 (Wang et al., 2013); and the disaggregation kinetics of peat HA at pH 3.65–5.56 (Avena and Wilkinson, 2002) but not directly in water- and alkali-extracted soil HA, FA, and PLS fractions. The acidic and alkaline pH conditions in the soil liquid phase alter the electronic configuration of the functional groups of HS components, which in turn affect their complexation capacity (Christl et al., 2005; Zhang et al., 2023; Avena and Wilkinson, 2002). The solubility and insolubility mechanisms of the HS components under different pH conditions remain unknown. In particular, two key fundamental questions regarding the effects of pH on HSs are still unclear, that is, how the electrochemical behaviour of soil HS components changes in the pH range of 1–12 and how these changes affect the solubility/insolubility features of HS components and their mobilization/immobilization during rainwater runoff and groundwater infiltration in soil.
Although the soil pH varies from 2.80–9.39 (Table S1), we fractionated the extracted solution in the pH range from 12–1 for several key reasons. Firstly, HSs bound as organo-minerals primarily liberate HS components and other constituents (e.g. various metals) in the liquid phase under alkaline extraction (0.1 M NaOH≈pH 13.0). Thus, it is crucial to understand how these HS components change their properties from pH 12–1. Secondly, it is essential to investigate how HSs (in particular, HA-bound DOC, due to its insoluble nature) behave within the pH range from 12–1.
Most importantly, the solubility of HS components and their subsequent mineralization are very relevant factors for the availability of soil nutrients and trace elements and the activity of soil microorganisms, while their stability in organo-minerals negatively affects these processes (Malik et al., 2018; Varghese et al., 2024; Lange et al., 1998; Gao et al., 2025; Soti et al., 2015; Yang et al., 2024; Gilbert et al., 2007; Zhang et al., 2023). These issues are concurrently associated with the corresponding biological fixation/sequestration of C, N, and S by soil photosynthetic microorganisms (Green et al., 2019; Varghese et al., 2024; Heckman et al., 2001; Levicán et al., 2008; Ma et al., 2021; Kelly et al., 2021; Gao et al., 2025) and the subsequent release of extracellular polymeric substances and/or HS components in the neoformation of fresh organo-minerals in soil (Whalen et al., 2024; Yu et al., 2020; Paul, 2016; Kallenbach et al., 2016). Therefore, the solubility or insolubility of soil HS components is crucial for a better understanding of both soil management and soil carbon dynamics.
Therefore, the main objective of this study was to ascertain the solubility characteristics of soil HS components under different pH conditions (pH 1–12) by analysing their fluorescence properties following extraction from two different soils using either water or an alkaline solution. Water-extractable HSs are designated labile-state (LS) HSs and are mostly subject to runoff from surface water and leaching from groundwater (Mohinuzzaman et al., 2020; Gao et al., 2018a). Alkali-extractable HSs are designated as complexed-state (CS) HSs and typically occur as organo-mineral and organo-metal complexes in soils (Kirsten et al., 2021; Lalonde et al., 2012; Hemingway et al., 2019; Kleber et al., 2021). Furthermore, to assess the electrochemical behaviour of soil HS components and their molecular-level characteristics based on their pH-dependent solubility, we also analysed HALS/CS precipitated at pH 6 (HALS/CS-pH 6) and pH 1 (HALS/CS-pH 1) and a mixture of FA and PLSs (FALS + PLSLS and FACS + PLSCS) at pH 1. Another key objective of this work was to provide a comprehensive view of the solubility and insolubility of soil HSs based on the mechanisms involved in the electronic configurational changes in HS reactive acidic functional groups, i.e. either in the protonic forms (e.g. –COOH, –OH) or in the anionic forms (e.g. –COO−, –O−) under various pH conditions. This will provide a better understanding of soil properties and processes for sustainable agricultural management.
2.1 Soil samples
Soil samples were taken from two locations in China: a maize field and a rice paddy field (Fig. S1 in the Supplement). The maize field soil is classified as calcaric fluvisol (Schad et al., 2015) and is located near the Beijing–Tianjin highway, approximately 20 km from the city of Tianjin. The rice paddy soil is classified as fluvi-stagnic luvisol (Schad et al., 2015) and is located near Shanghai. The two soils were cultivated for approximately 50 and 30 years, respectively. Importantly, the rationale for selecting paddy and maize soils is based on their distinct characteristics; i.e. paddy soils are submerged for extended periods, while maize soils are relatively less influenced by the presence of water. Therefore, HS components of these two soil types are expected to be altered very differently in their organo-mineral lability and stability in the pH range from 1–12. This study is expected to provide useful information on soil carbon dynamics and contribute to minimizing soil carbon loss during agricultural practices. At each site, three soil subsamples were randomly collected from the top horizon A (0–30 cm) and mixed homogeneously to produce a spatially representative sample at the field scale. After oven-drying to constant weight at 40 °C, the samples were passed through a 2 mm sieve. Table S3 provides information on the sampling sites, vegetation cover, and major physicochemical characteristics of the two soil types.
The soil particle size was measured using the hydrometer method with a Mastersizer 3000 (Malvern; Table S3). The soil extracts (see below) were obtained from 0.2 mm sieved soils after mortar-grinding with a pestle.
2.2 Protocol used to extract water- and alkali-soluble SOM/HSs
In the first part of the experiment, the soil liquid phase was extracted from the two soils using either water or an alkaline solution (0.1 M NaOH), which operationally represent, respectively, the water-extractable labile state (LS) and the water-insoluble alkali-extractable complexed state (CS) of SOM/HSs (Mohinuzzaman et al., 2020). The detailed extraction procedure is shown in the flow diagram in Fig. S2. Briefly, the water extracts were obtained using ultrapure water (18.2 MΩ cm, Mill-Q, Millipore) with a soilwater ratio of 1:10. The mixtures were vortexed for 1 min in closed 500 mL brown bottles before being shaken for 24 h in an orbital shaker (200 rpm) at 25 °C. The mixtures were then centrifuged for 20 min at 4000 rpm (Thermo Fisher Scientific Sorvall ST 16) for removing suspended solids. The supernatant solutions were then filtered through a 0.45 µm glass fibre filter (GF/F type, Shanghai Xin Ya Purification Equipment Co. Ltd, China) to remove any remaining particulate matter. The solutions were then frozen at −20 °C.
To obtain the alkaline extracts, the suspended soil residues from water extraction were sequentially extracted under N2 with a 0.1 M NaOH solution at a soil residue/alkaline solution ratio of 1:10 (Fig. S2). In this case, the mixtures were also vortexed for 1 min, shaken at 200 rpm for 3 h at 25 °C, and then centrifuged for 20 min at 4000 rpm using the same centrifuge as before to remove suspended solids. The supernatant solutions were then filtered through a 0.45 µm membrane filter (polytetrafluoroethylene (PTFE) membrane, Shanghai Xin Ya Purification Equipment Co. Ltd, China) to remove any remaining particulate matter. Under alkaline conditions, PTFE filters are highly effective at separating solutions from particulate matter (Mohinuzzaman et al., 2020). The remaining solid residue was extracted with a fresh alkaline solution for 3 h, and the above procedure was repeated. The supernatant solutions were then mixed with former solutions and frozen at −20 °C for further processing. The original pH values for the water-extracted paddy and maize samples were 8.13 and 7.92, respectively, while alkali-extracted samples were 13.02 and 12.98, respectively.
2.3 Protocol used to isolate solid HA and FA + PLS samples by acidification of water and alkaline extracts
The second part of the experiment involved two distinct approaches. To adjust the pH from 12–1, aliquots of 45 mL of water or alkaline extracts were placed in 50 mL glass bottles, and then the pH was progressively adjusted to certain values in the range 12–1 by adding 0.1 and 1 mol L−1 NaOH or HCl solutions with a 10 µL chromatographic sampler (minimum scale 0.2 µL). As the maximum amount of acid/base reagent added to each sample was <1.0 mL, the dilution effect could be ignored. A Thermo Orion water quality tester, calibrated before each measurement, was used to determine the pH of the solutions prior to further analytical measurements. Three replicates (n=3) were used for each pH adjustment experiment. All experiments were performed under a laboratory ambient temperature of 25 °C.
In the other approach, approximately 400 mL of water extracts or alkaline extracts was placed in individual 500 mL glass bottles, and the pH was adjusted to 6 using HCl (0.1 and 1 mol L−1) and left for 10 h at 25°C to allow the precipitation of HALS and HACS, respectively (Fig. S2). The precipitates, denoted as HALS at pH 6 (HALS-pH 6) and HACS at pH 6 (HACS-pH 6), were separated by centrifugation (Thermo Fisher Scientific, Sorvall ST 16) at 3000 rpm for 5 min. The supernatants were then adjusted to pH 1 using the same procedure described above, yielding HALS (HALS-pH 1) and HACS (HACS-pH 1). The remaining supernatants at pH 1 were classified as FALS + PLSLS and FACS + PLSCS mixtures. The HA precipitates and FA + PLS solutions were freeze-dried before further analysis.
2.4 Analytical methods
The elemental compositions of the HA isolated at pH 6 and 1 and the freeze-dried mixture of FA + PLS were measured using an elemental analyser (Vario EL III, Elementar, Germany). Approximately 20 mg of each dried, ground, and homogenized sample was placed in a clean, carbon-free, pre-combusted tin boat placed on an autosampler rack assembly and loaded onto the elemental analyser. Sulfanilamide was used as the standard after every 10 measurements. The O content was calculated by the difference formula:
Fluorescence (excitation–emission matrix, EEM) spectra were obtained using a fluorescence spectrophotometer (F-7000, Hitachi, Japan), as previously described (Mohinuzzaman et al., 2020; Yang et al., 2021). To ensure instrument performance and data quality, every 10th sample was measured with ultrapure (18.2 MΩ cm) water as a blank. The water EEM spectra were subtracted from the sample EEM spectra. A 4 µg L−1 quinine sulfate (QS) solution in 0.01 N H2SO4 was used for fluorescence normalization. The fluorescence intensities of each sample were calibrated using the intensity of the QS ( unit (QSU)) peak at (Mohinuzzaman et al., 2020). To avoid inner-filter effects and fluorescence quenching, the extracted solutions were diluted prior to EEM measurements based on the initially measured DOC concentration (Tadini et al., 2018). The fluorescence intensity of each peak was rechecked and corrected using the absorbance-based method proposed by Kothawala et al. (2013).
The pre-processed EEM data were then subjected to PARAFAC analysis using the N-way Toolbox for MATLAB (Andersson and Bro, 2000), as previously described (Stedmon et al., 2003). Firstly, the Rayleigh and Raman peaks and the ultrapure water blank spectrum were subtracted from each experimental EEM spectrum using a homemade Excel program (Mohinuzzaman et al., 2020). To avoid mixing the fluorescent components of different soil samples, which could produce artefacts (Mostofa et al., 2019), PARAFAC analysis was performed individually for each selective sample. Finally, nonnegative constraints were applied to the PARAFAC model. The detailed procedure used for PARAFAC analysis of the EEM spectra has been described previously (Mohinuzzaman et al., 2020).
The Fourier transform infrared (FTIR) spectra were recorded on 2 mg aliquots of each dehydrated HA isolated at pH 6 and 1 and on each freeze-dried mixture of FA + PLS, which were mixed with 200 mg of dried KBr and pelletized by pressing under reduced pressure. The spectra were measured over the range of 4000–400 cm−1 by averaging 30 scans at a 4 cm−1 resolution using an IRAffinity-1S spectrometer (Shimadzu, Japan) that included a high-energy ceramic light source; a temperature-controlled, high-sensitivity DLATGS detector; and a high-throughput optical element, which allowed the optimization of the electrical and optical systems to achieve the highest signal-to-noise (SN) ratio.
3.1 Fluorescence spectra
The fluorescence peaks of HA, FA, and PLSs in the EEM spectra of the water and alkaline extracts of each sample (original and adjusted pH) were identified individually by applying the PARAFAC model (Figs. 1 and 2; Table 1). The fluorescence properties of the original samples were similar to those measured in an earlier study (Mohinuzzaman et al., 2020), but the EEM images and fluorescence peaks of all three components (HA, FA, and PLSs) identified in the pH-adjusted samples exhibited distinct differences. Such differences could be attributed to the pH-influenced changes in protonation/deprotonation of the each component's functional groups, which could either suppress or favour electron transfer processes from the functional groups to the solution (Mostofa et al., 2013; Senesi, 1990b). Two fluorescence peaks were identified in the HA (peaks C and A) and FA (peaks M and A) components, while four peaks were identified in the PLS fraction: peaks M and A for HLF and peaks T and TUV for PLF (Mohinuzzaman et al., 2020).
Table 1Excitation–emission (Ex/Em) wavelengths (nm) of fluorescence peaks of HA, FA, and PLSs identified by PARAFAC analysis applied individually to EEM spectra of original water and alkaline extracts from paddy and maize soils and of their pH-adjusted solutions at pH 1–2, pH 3–4, pH 5–6, pH 7–8, pH 9–10, and pH 11–12.
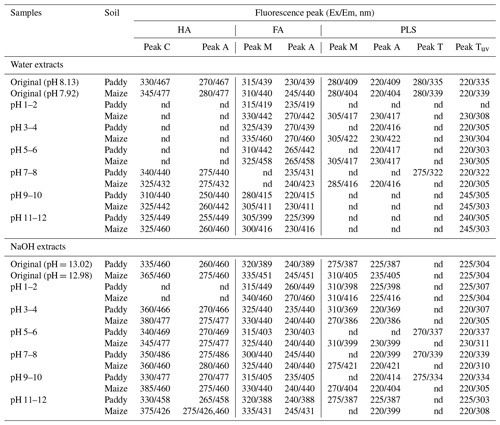
nd: not detected.
3.1.1 Characteristics of HALS-pH 6/pH 1 and solubility of HACS
The EEM-PARAFAC model detected no fluorescence in water-extracted HALS at acidic pH ranging from 6–1 (Fig. 1). This causes HA to precipitate at pH 6 (HALS-pH 6) and at pH 1 (HALS-pH 1), accounting for approximately 48.3 %–49.2 % and 3.1 %–10.8 % of total DOCLS, respectively, based on initial DOCLS concentrations of 13.4 and 24.5 mg L−1, respectively, in paddy and maize soils (Fig. 3). Absence of fluorescence or precipitation at pH<7 suggests that HALS may naturally stabilize in soil during rainfall/water runoff at pH values≤6 due to its water insolubility. However, at higher pH levels, the fluorescence peak maxima (C: 310–340/432–460 nm; A: 250–275/432–460 nm) and intensities varied significantly (Figs. 1 and 3; Table 1). The highest C and A peak intensities of HALS were observed at pH 7, with a gradual decrease as pH increased in both types of soil HALS. At pH 7–8 (C: 325–340/432–440 nm; A: 275/432–440 nm; Table 1), the functional groups can donate their electrons, thus increasing their fluorescence intensity, whereas the blue shift and decreasing intensity of the fluorescence peaks with increasing pH are caused by the deprotonation/ionization of COOH and OH functional groups. The deprotonated functional groups would form organo-metal complexes through donation of π electrons to d orbitals of metal ions, particularly Fe ions (Zhang et al., 2023), which insolubilize HSs/SOC in soil (Kirsten et al., 2021; Six et al., 2002; Lalonde et al., 2012; Hemingway et al., 2019; Kleber et al., 2021; Makiel et al., 2022). In contrast, the red shift of the fluorescence peaks could be attributed to easier electron transfer from the functional groups of HA (Mostofa et al., 2013; Senesi, 1990b).
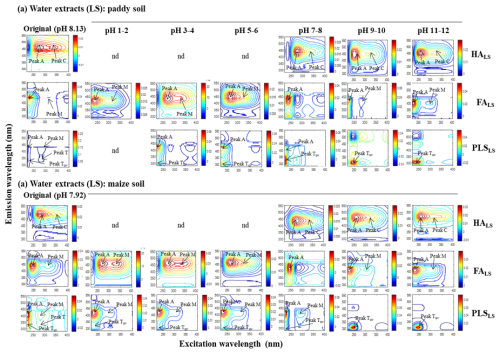
Figure 1Fluorescence spectra and peaks identified using EEM-PARAFAC modelling in the original solution before pH adjustment and water extracts from paddy and maize soils adjusted at various pH levels. Water-extractable humic acid (HA) is completely precipitated in both paddy and maize soils, as evidenced by a substantial decrease in dissolved organic carbon (DOC) concentrations of 48.3 % and 49.2 % at pH 6, respectively. Consequently, it yields a result of not detected (nd) by the EEM-PARAFAC model. Similarly, nd for protein-like substances (PLSs) in paddy soil may be due to its highly degradative nature, as its minor peaks are detected in the original solution at pH 8.13, whereas the corresponding functionalities disappear at highly acidic pH 1–2.
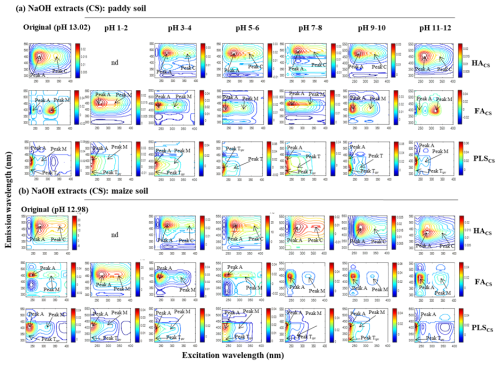
Figure 2Fluorescence spectra and peaks identified using EEM-PARAFAC modelling in the original solution before pH adjustment and alkaline extracts from paddy and maize soils adjusted at various pH levels. Alkali-extractable HA is completely precipitated at pH 1 in both paddy and maize soils, which is a well-known phenomenon in soil, also evidenced by a corresponding decrease of 48.1 % and 53.8 % of DOC concentration at pH 1, with respect to their extracted original solutions (pH∼13). Consequently, HA at pH 1–2 yields a result of “not detected” by the EEM-PARAFAC model. It is noteworthy that the detection of alkali-extractable HA at pH levels 3–6, in contrast to its absence in water-extractable HA, may result from a significantly higher release of HA from organo-minerals, which is evidenced by the DOC in alkali-extractable HA that is 2.6–3.2 times greater than that in water-extractable HA. In particular, water-extractable HA is highly degradative in nature and shows relatively low concentrations, which correspondingly result in its precipitation at pH 6.
Unlike HALS, the excitation–emission peaks of HACS at pH 1–10 in maize soil were detected at wavelengths (C: 345–385/460–477; A: 275–280/460–477 nm) that were longer than those of the corresponding HALS at pH 7–10 (C: 325–345/432–477; A: 260–280/432–477 nm) (Fig. 2 and Table 1). These results would suggest that HA at pH 2 may be the insoluble form of HSs bound to various minerals/metals (Kirsten et al., 2021; Curtin et al., 2011; Lalonde et al., 2012; Hemingway et al., 2019), whereas the longer-wavelength peaks (C and A) of alkali-extractable HACS functionality remain under mineral protection (Mohinuzzaman et al., 2020). The two peak maxima at longer wavelengths (350/486 and 275/486 nm) at pH 7–8 in HACS from paddy soil and at 380/477 and 275/477 nm at pH 3–4 in HACS from maize soil might be ascribed to electron transfer from thiol- and/or N-containing functional groups and/or highly aromatic ring structures in alkaline-extracted HA (Fulda et al., 2013; Haitzer et al., 2002, 2003; Szulczewski et al., 2001) and to binding sites reacting with metal ions (Wu et al., 2004a, b). These groups are significantly affected by environmental factors and soil conditions (Jiang et al., 2015; Vidali et al., 2010). In particular, an increase in acidity might shift peak C of soil HACS from a shorter to a longer excitation wavelength but does not affect peak A detected at pH 3–4 (C: 360–380/466–477 nm; A: 270–275/466–477 nm) and pH 5–6 (C: 340–345/469–477 nm; A: 270–275/469–477 nm) (Fig. 2; Table 1). These results would imply that increasing the acidity promotes electron transfer from the peak-C-type functional groups of HACS.
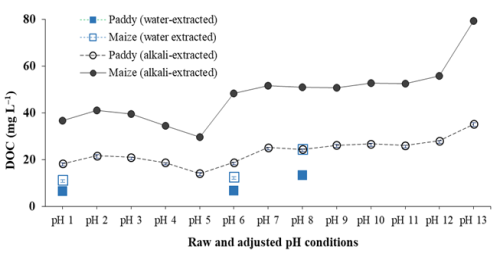
Figure 3Variation in DOC concentrations of the pH-adjusted HSLS and HSCS from paddy and maize soils.
The shorter emission maxima of peaks C and A in HACS at pH 11–12, i.e. 458 and 458 nm and 426 and 460 nm, respectively, for paddy and maize soils (Table 1), would suggest that electrons released from HACS functional groups were primarily suppressed by extreme alkalinity conditions, as they would require higher energy, as confirmed by the appearance of peaks of decreased intensity at shorter wavelengths (Fig. 3). Thus, HA showed a higher electron transfer capacity in paddy soils than in maize soils (Xi et al., 2018).
Furthermore, the significant decrease in the two peak intensities in HACS at pH 1–6 would be primarily due to precipitation at pH 6 and pH 1, which amounted approximately to 39.1 %–46.4 % and 3.1 %–24.1 %, respectively, of the initial DOCCS concentrations of 35.2 and 79.4 mg L−1, respectively, in paddy and maize soils (Fig. 3). The higher peak intensities at pH 1–2 than at pH 3–4 (Fig. 4) would suggest that some functional groups were labile at this pH, thus favouring electron transfer from HACS in both soils; this was also confirmed by the longer wavelength of the excitation–emission peak C at pH 1–2.
3.1.2 Behaviour of FALS and FACS as a function of pH
Both FALS and FACS showed higher intensities of the two peak maxima at pH 3–4 (i.e. 325–335/439–460 and 270/439–460 nm, respectively) and at pH 1–4 (i.e. 315–340/449–460 and 260–270/449–460 nm, respectively) than at alkaline pH, with the former showing a blue shift with respect to the latter for both soils (Figs. 1 and 2; Table 1). The pH-dependent differences arising in FALS might be due to existing environmental factors (e.g. moisture, temperature/climatic warming, redox properties, mineral matrix, agricultural practices, vegetation, and microbial activities), whereas those in FACS might remain under mineral protection because of their occurrence in organo-mineral complexes (Kirsten et al., 2021; Mohinuzzaman et al., 2020; Lehmann and Kleber, 2015; Gao et al., 2018a). Moreover, peak M disappeared at pH 7–8 and a minor peak appeared in the original FALS, suggesting degradation of the functional groups in FALS at pH 7–8. The longer-wavelength peak maxima measured at extremely acidic pH 1–4 would indicate easier electron/proton transfer from the protonated phenolic groups in both FALS and FACS (Klapper et al., 2002; Nurmi and Tratnyek, 2002; Cory and McKnight, 2005; Yang et al., 2016; Wang et al., 2023). In contrast, increasing the pH would imply the ionization of phenolic groups, which would necessitate more energy for the electron transfer process, resulting in peak maxima at shorter wavelengths under alkaline conditions.
The wavelength differences detected for peak maxima were accompanied by differences in their intensity, which was the highest for peak M of FALS at pH 6 and increased by approximately 362 % and 20.0 %, respectively, in paddy and maize soil FALS, compared with the original FALS. This indicates that protonated functional groups can transfer electrons more easily than deprotonated functional groups. In contrast to FALS, the highest peak M intensity of FACS from paddy soil occurred at pH 12 and gradually decreased to pH 5, whereas FACS from maize soil showed the highest intensity at pH 3 and decreased up to pH 9 (Fig. 4), suggesting a difference in peak M functional groups between the two soils. These features may be ascribed to the different environmental conditions in the two soils; that is, long-term submersion in paddy soil and a drier state in maize soil (Mohinuzzaman et al., 2020).
Peak A intensity followed a similar trend for both soils, peaking at pH 10 and 8, then gradually decreasing to pH 5–6 by 57 % for the paddy soil and to pH 3–4 by 41 % for the maize soils (Fig. 4). These results suggest that peak A functional groups in the FACS of the two soils behave similarly. The highest peak intensity of FACS in the two soils was detected at pH 3, but these peaks were absent in FALS (Figs. 1 and 2). These results suggest the presence of new functional groups in FACS that are absent in water-soluble FALS. The decreasing intensity of peak A toward either extremely acidic (pH 1–2) or alkaline (pH 11–12) conditions suggests an increased suppression of electron release at either very high or very low pH conditions. Notably, both FALS and FACS exhibited the highest solubility under acidic conditions, such as pH 3 and pH 6, respectively.
3.1.3 Behaviour of PLSs as a function of pH
Peak M of HLF in the PLSLS from maize soil was most prominent at acidic pH, with very low intensity at pH 7–8 and disappearing entirely at pH 9–12 (Figs. 1 and 4). These results might be ascribed to the easy electron transfer from the corresponding functional groups under acidic conditions and to the suppression of electron release under alkaline conditions. However, this peak was completely absent in the PLSLS from the paddy soil at any pH condition, possibly due to the long-term favoured hydrolysis occurring under submerged conditions, which does not occur in the drier maize soil where this fraction is not degraded (Mohinuzzaman et al., 2020).
The PLSLS samples from both soils exhibited two PLF peaks (that is, T and TUV) at pH 7–8, with peak T (245/303 nm) that completely disappeared at acidic pH 1–6 but was dominant at pH 9–12. This may imply a marked influence of the pH on the ionization of the functional groups. In contrast, the PLSCS from the paddy soil showed PLF peaks (T and TUV) in the pH range of 3–10, whereas, in the PLSCS from maize soil, they were predominant at acidic pH 1–6, appeared as minor peaks at alkaline pH 7–10, and disappeared at pH 11–12 (Fig. 2 and Table 1). Notably, PLSCS, like FALS/CS, might undergo rapid electron/proton exchange reactions that result in the appearance of predominant peak maxima under acidic conditions, whereas the disappearance of PLF peaks at pH 11–12 might arise, similarly to FALS/CS, from the anionic forms of PLSs, which might be involved in stable organo-mineral complexes. In this case, the submerged conditions existing in the paddy soil are primarily responsible for the predominant occurrence of PLF peaks in the PLSCS, whereas the drier conditions of maize soil (high temperature and low precipitation) cause extensive degradation of the PLF components, with the predominant presence of the HLF components. However, the significant increase in the peak intensities of both HLF and PLF in PLSCS at pH 6 implies that the responsible functional groups would remain in a protonated state (Fig. 4), which suggests a marked pH effect on the functional groups of PLSCS. Similar pH-influenced changes in the peak TUV intensities have been reported for extracellular polymeric substances (Zhang et al., 2010).
Finally, the predominant presence of PLF and HLF components in PLSCS compared to PLSLS suggests their origin from newly formed insoluble complexes with minerals/metals (Ciceri and Allanore, 2015; Curtin et al., 2011; Mohinuzzaman et al., 2020; Song et al., 2016). Furthermore, the presence of a PLF peak at 240–245/303–305 nm at pH 9–12 in PLSLS, which was not detected in PLSCS, supports its origin in PLS degradation under environmental conditions. The dominant presence of the HLF peaks in both PLSLS and PLSCS may facilitate electron transfer from the corresponding functional groups, which is a key factor in their solubility under acidic conditions.
3.2 Soil properties and elemental composition of HSs
The soil total carbon (STC) and soil organic carbon (SOC) in the paddy soil (14.22 and 10.82 mg g−1, respectively) were higher than in the maize soil (13.13 and 8.76 mg g−1, respectively), whereas the soil total nitrogen (STN) in maize soil (0.78 mg g−1) was higher than that of paddy soil (Table 1 in Mohinuzzaman et al., 2020). The clay and silt contents were significantly higher in the maize soil (8.6 % and 57.6 %, respectively) than in the paddy soil (2.5 % and 38.3 %, respectively), whereas the sand content in the paddy soil (36.0 %) was higher than that in the maize soil.
The C and N contents of HALS-pH 6 from both soils were lower than those of O, S, and H, and all atomic ratios were higher than those of HACS-pH 6 (Table 2). These results would suggest the preservation of C and N without S acquisition in HACS-pH 6, possibly because of their complex state with minerals (Hemingway et al., 2019; Marschner et al., 2008; Vogel et al., 2014), which, in turn, determines the insolubility of the HACS-pH 6 fraction. In contrast, the lower levels of C and N and the high content of S that characterize HALS-pH 6 would suggest the degradation of the N-containing functional groups (Mohinuzzaman et al., 2020; Li and Vaughan, 2018; Senesi and Loffredo, 1999) and the acquisition of S-containing compounds, possibly from soil fungi (Masaki et al., 2016; Saito et al., 2002; Whelan and Rhew, 2015), which, in turn, would determine the solubility of the HALS-pH 6 fraction.
Table 2Elemental composition (%, moisture- and ash-free) and atomic ratios of HALS-pH 6, HALS-pH 1, HACS-pH 6, HACS-pH 1, FALS + PLSLS at pH 1, and FACS + PLSCS at pH 1.
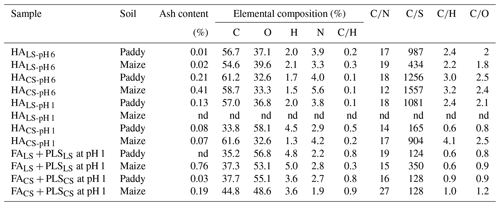
nd: not detected due to lack of sample.
Due to the lack of sample HALS-pH 1 from maize soil, no comparison was possible with the corresponding HACS-pH 1. However, HACS-pH 1 from paddy soil showed extremely low C%, N%, and atomic ratios and very high O%, H%, and S% compared to the corresponding HALS-pH 1, indicating its insolubility at pH 1; that is, this HA fraction would remain under mineral protection in soil (Hemingway et al., 2019; Marschner et al., 2008; Vogel et al., 2014). It is possible that the decrease in C and increase in O in the HACS-pH 1 fraction in paddy soil were affected by high water availability and microbial respiration (Fang et al., 2005; Huang and Hall, 2017; Yu et al., 2020; Chen et al., 2020).
The main features of all the FA + PLS samples were their very low C, N, CS, CH, and CO ratios and very high O%, H%, and S% with respect to the corresponding HA fractions discussed above (Table 2). In particular, FACS + PLSCS showed relatively higher C and S contents and CH and CO ratios and lower O% with respect to FALS + PLSLS, which would suggest that, similarly to HACS samples, FACS + PLSCS would remain under mineral protection in the soil. The higher S content of FALS + PLSLS from paddy soil than that of maize soil might be ascribed to the uptake and conversion of carbonyl sulfide (COS), possibly operated by soil fungi or microorganisms in the paddy soil (Li et al., 2010; Masaki et al., 2016; Saito et al., 2002; Whelan and Rhew, 2015), whereas S would be rapidly degraded by biotic and abiotic processes in the drier maize soil (Liu et al., 2007; Masaki et al., 2016; Whelan and Rhew, 2015). Similarly, the relatively lower C% in FALS + PLSLS and FACS + PLSCS from paddy soil compared with maize soil might be ascribed to extended oxidative degradation and/or hydrolysis processes occurring in paddy soil, which lead to extended mineralization processes (Fang et al., 2005; Huang and Hall, 2017; Yu et al., 2020; Chen et al., 2020). Finally, the high O% in the FA + PLS samples might have contributed to the presence of O-rich PLSs extracted together with FA.
3.3 Fourier transform infrared (FTIR) spectra
The FTIR spectra of all tested samples (Fig. 5 and Table 3) were typical of soil HSs (Senesi and Loffredo, 1999), but they exhibited a number of different characteristics. Firstly, HACS-pH 6 had significantly lower IR absorptions than HALS-pH 6 in both soils, particularly in the ranges of 3300–3600 and 800–1200 cm−1. This suggests strong intermolecular interactions among HA functional groups, possibly due to insoluble forms complexed with minerals/metals (Gabor et al., 2015; Mostofa et al., 2018). This has an impact on the overall bonding system in the conjugated macromolecular HA structure. Furthermore, these insoluble forms require relatively high energy for electron transfer, resulting in a decrease in the relative intensity of all bands in HACS-pH 6 compared to HALS-pH 6. Secondly, the band at 3421–3429 cm−1 is stronger for HALS-pH 1 than for HALS-pH 6, indicating the presence of more free NH or OH functional groups (Demyan et al., 2012; Kunlanit et al., 2014; Senesi et al., 2003). Thirdly, the weak band at 1015–1030 cm−1 (possibly attributed to S=O and C–O–S stretching of S-containing functional groups) in HALS-pH 1 of the paddy soil and its absence in maize soil (Demyan et al., 2012; Singh et al., 2011; Shammi et al., 2017; Senesi et al., 2003) might be due to the degradative nature of HALS-pH 1 compared to HALS-pH 6. HALS-pH 1 degradation is primarily caused by the degradation of its functional groups in the presence of existing environmental factors (Xie et al., 2004; Mohinuzzaman et al., 2020; Lehmann and Kleber, 2015). Fourthly, the samples FALS + PLSLS generally exhibited stronger bands at 3414–3429 and 1008–1018 cm−1 than FACS + PLSCS (Gabor et al., 2015; Kunlanit et al., 2014; Mostofa et al., 2018), which suggested a strong interaction among functional groups possibly generated from various silicates/mineral complexes in FACS + PLSCS, whereas a weak interaction would have yielded free functional groups in LS samples featuring strong bands by loosely bound electrons in functional groups. Fifthly, the presence of two relatively intense bands at 3711–3745 and 3838–3873 cm−1 in all HA samples could be attributed to aromatic C–H stretching in individual aromatic ring structures, while aromatic C–H in conjugated systems absorb at 3080–3030 cm−1 (Kunlanit et al., 2014; Demyan et al., 2012; Senesi et al., 2003).
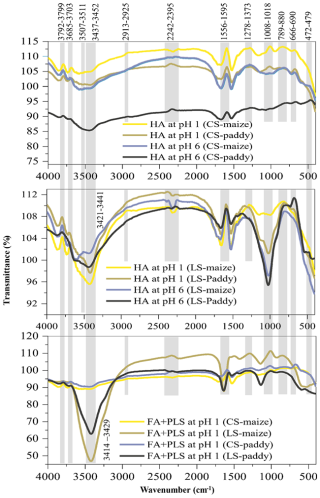
Figure 5FTIR spectra of HALS-pH 6, HALS-pH 1, HACS-pH 6, HACS-pH 1, FALS + PLSLS at pH 1, and FACS + PLSCS at pH 1.
3.4 Mechanisms determining the insolubility/solubility of HA and FA + PLS
Two molecular parameters, the electrochemical force (EF), also known as the intermolecular force, and the intramolecular force (IF), are thought to control the mechanisms underlying the solubility/insolubility of HA and FA + PLS (Fig. 6). In particular, EF includes intermolecular van der Waals forces, London forces, dipole–dipole and ion–dipole interactions, and hydrogen bonds between molecules, whereas IF refers to the intramolecular forces between bonded atoms in a molecule (Aeschbacher et al., 2010). In particular, the decrease in the net EF (EFN) could be attributed to the protonation of the functional groups in HA, which decreases their electron-donating capacity in aqueous solutions (Ai et al., 2020; Chassapis et al., 2010; Ritchie and Michael Perdue, 2003). In contrast, an increase in net IF (IFN) can be attributed to increased intramolecular interactions between various functional groups via hydrogen bonding in HA (Ai et al., 2020; Benes, 2009; Boguta et al., 2019; Noy et al., 1997; Vezenov et al., 2005, 1997). Strong competition exists between EFN and IFN; when IFN > EFN under acidic conditions, all functional groups associate, resulting in HA precipitation from the solution.
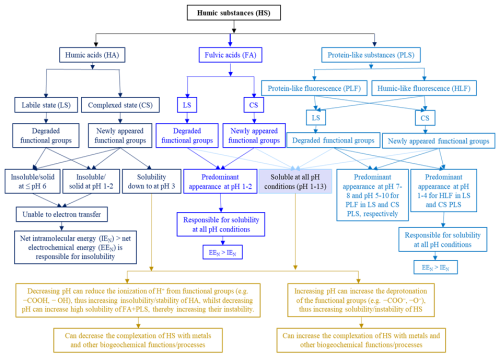
Figure 6Conceptual model developed referring to HSLS and HSCS, including HA, FA, and PLSs, based on the presence or absence of the corresponding fluorescence peaks in different pH conditions.
The solubility of FALS/CS + PLSLS/CS at all acidic pH values was related to their higher total acidity, which resulted from a higher number of elemental oxygen atoms (Table 2) which belong to oxygenated functional groups and have a relatively lower molecular size than HA (Leenheer et al., 1995; Robarge, 2018). These features would cause a relatively low IFN value and a relatively high EFN value owing to the formation of external H-bonding with the solution components. This interpretation was supported by the presence of two peaks for each FA and an HLF peak in the PLSs at pH 1–4 (Figs. 2 and 4; Table 1). These results would confirm the easier electron transfer from the functional groups to the solution at acidic pH, resulting in EFN > IFN, implying their dissolution at extremely acidic pH (Fig. 6).
3.5 Solubility/insolubility characteristics of soil HSs and their environmental consequences
The solubility/insolubility of the HS components was influenced by each specific pH unit, with the involvement of various functional groups (Fig. 6) (Avena and Wilkinson, 2002; Boguta et al., 2019, 2016; Garcia-Mina, 2006; Hernández et al., 2006), which might occur through various processes such as complexation, ion exchange, adsorption, aggregation/coagulation, and flocculation (Avena and Wilkinson, 2002; Lippold et al., 2007; Wang et al., 2013; Jovanović et al., 2013). In particular, (a) HACS-pH 6/HALS-pH 6 and HACS-pH 1/HALS-pH 1 would remain in suspension under acidic conditions, whereas IF interactions preferentially increase with increasing acidity owing to the enhanced occurrence of protonic forms of their functional groups, and (b) the disappearance of fluorescence peaks (C, M, A, T, or TUV) of specific functional groups of individual HS components under any pH condition in solution would cause their interactions either with other functional groups or coagulation/precipitation with metals or minerals (Chen et al., 2014; Helms et al., 2013; Zhang et al., 2023; Hemingway et al., 2019; Lützow et al., 2006; Marschner et al., 2008; Sollins et al., 1996; Vogel et al., 2014). Furthermore, each individual pH unit may sterically affect the HS functional groups (Boguta et al., 2019; Senesi, 1990a, b), which would result in either the appearance or disappearance of a fluorescence peak and/or a change in the fluorescence intensity of specific peaks (Figs. 2–4 and Table 1). These effects may be associated with an increase or decrease in the electron donation capacity of the fluorescent functional groups in HSs (Cory and McKnight, 2005; Senesi, 1990a; Klapper et al., 2002; Karadirek et al., 2016; Wang et al., 2023), thus determining their solubility/insolubility.
An overall conceptual model of the possible processes and mechanisms is outlined in Fig. 6 and summarized below.
-
The deprotonated state of the functional groups (e.g. –COO−) in HALS constantly donates electrons to various soil components, thus activating a series of biogeochemical processes. Rainwater (usually at pH≤6) or water discharge/runoff cannot dissolve HALS and, partly, HACS. In particular, HALS/CS-pH 6 is insoluble/not mobile in soil during rainwater events and water runoff at pH≤6, suggesting natural protection during transport along the soil profile and in ambient surface waters. In contrast, HALS/CS-pH 1 is mobile and transported to ambient surface waters via rainwater, leaching, and groundwater infiltration (Ronchi et al., 2013; Stolpe et al., 2013; Mostofa et al., 2019).
-
Under acidic conditions, down to pH 1, the functional groups of HACS/LS-pH 1 remained protonated, thus reducing electron transfer capacity. This feature of HACS/LS-pH 1 might explain some recent results, e.g. the decline in metal binding capacity of HSs at low pH (Christl et al., 2005), the low effect of HA on plant growth (Asli and Neumann, 2010; Mora et al., 2012), the decline in HA capacity in binding organic pollutants (Jones and Tiller, 1999; Tremblay et al., 2005), and the decrease in carbon mineralization at low pH with a 5-fold decrease in bacterial growth and a 5-fold increase in fungal growth (Rousk et al., 2009).
-
Higher pH increases the deprotonation of functional groups (e.g. –COO−) of HALS/CS, allowing easier electron transfer to soil components like minerals and fungi (Chen et al., 2020; Yu et al., 2020) and increasing the solubility of metal ions (Firestone et al., 1983; Flis et al., 1993), e.g. from metal sulfides (Chou et al., 2018), soil respiration, and carbon mineralization (Pietikäinen et al., 2005; Rousk et al., 2009), and the degradation of –COOH/–OH upon exposure to UV–Vis light (Spence and Kelleher, 2016; Ward et al., 2013; Xie et al., 2004).
-
The predominant presence of two FALS/CS peaks at pH 1–2, which were absent at neutral or alkaline pH (Figs. 2 and 4), suggests the solubility of these HS components under acidic conditions. In turn, this condition affects the capacity for complexation/decomplexation and/or sorption/desorption of metal ions and organic pollutants, thus modifying their mobility/transport by rainwater/water discharge/runoff and groundwater leaching (Tadini et al., 2020; Mostofa et al., 2019) and their distribution, toxicity, and bioavailability in soil (Anastasiou et al., 2014; dos Santos et al., 2020; Tadini et al., 2020; Zhu and Ryan, 2016). In particular, the FALS/CS fractions in acidic conditions easily leached down the soil profile via rainwater discharge, as occurs in the podsolization process (Lundström et al., 2000).
-
The predominance of HLF in PLSLS and of PLF in PLSCS at acidic pH (Figs. 2 and 4) may be primarily responsible for their high solubility under acidic conditions, which implies high mobility and easy transport in ambient water environments and groundwater leaching (Gao et al., 2018a; Mohinuzzaman et al., 2020).
-
The knowledge of the molecular-level solubility of the three HS components is essential for a better understanding and management of agricultural practices, as affected by their individual solubility, tendency to precipitate, and variable capability to form organo-minerals, which can occur more or less rapidly/slowly (Underwood et al., 2024; Zhang et al., 2023). For instance, the HA fractions of acidic soils may either partially precipitate or remain in suspension due to an increase in IFN from increasing intramolecular interactions among various functional groups via hydrogen bonding, influenced by acidic conditions as discussed earlier. As a result, precipitated HA fractions would enhance C stability, while suspended HA fractions are highly prone to leaching by rainwater runoff. Furthermore, the high solubility of FA and PLSs under acidic conditions would result from prolonged water saturation occurring in paddy fields, which will lead to soil C loss by their transport due to rainwater runoff. Simultaneously, these conditions of HSs are expected to contribute to increasing the salinity levels in such types of soils (Varghese et al., 2024; Ma et al., 2021). Therefore, high-water-demand crops such as rice may not be suitable for maintaining C stability in acidic soils. In contrast, low-water-demanding crops like maize and wheat would be more effective in minimizing C loss from acidic soils. On the other hand, alkaline soils can support the cultivation of a wide variety of crops while minimizing C loss, along with the presence of relatively high levels of HSs bound to organo-minerals. In particular, the pH levels of the paddy and maize soils, which are the object of this study, are slightly alkaline (8.13 and 7.92, respectively), making them reasonably suitable for diverse types of crops. Furthermore, both soils exhibit relatively high levels of HS-bound organo-minerals, with significant increases in DOCCS stability (2.6 and 3.2 times higher, respectively) compared to DOCLS lability.
-
The strategies for addressing pH-affected soil carbon loss primarily involve the significant loss of dissolved HSs, particularly fulvic acids and protein-like fractions, from acidic soils due to water and rainwater runoff. Therefore, it is crucial to implement an efficient, rapid, and sustainable drainage system that operates on a relatively short timescale so that HS components may become less likely to dissolve in water and rainwater. Effective and timely drainage from the soil surface can help prevent carbon loss from acidic soils.
Finally, the HSs/SOM appeared to undergo progressive transformation under various environmental conditions (Mohinuzzaman et al., 2020), yielding various forms of HS components (Figs. 2 and 4). Furthermore, pH appears to control the chemical nature and electronic configuration of HA/FA/PLS functional groups, influencing their solubility/insolubility and consequently their mobilization/immobilization and transport/accumulation, thereby markedly affecting all biogeochemical functions and processes in the soil. The features and extension of such processes would depend mostly on the existing environmental conditions and factors, such as pH, soil type, organisms (e.g. bacteria, fungi, and vegetation), temperature variations due to climate change, and precipitation frequency and intensity (Mohinuzzaman et al., 2020; Pietikäinen et al., 2005; Rousk et al., 2009).
The presence, absence, or variable relative intensity of the fluorescence peaks of HS components under different pH conditions and their relationship with electron release from their functional groups appeared to be an excellent indicator of the HS component status. In particular, an alkaline or elevated pH level would result in anionic forms (–O− and –COO−) of phenolic OH and carboxyl groups of HA, FA, and PLSs, which ultimately contributes to the insolubilization and stability of HSs through the formation of organo-mineral complexes in soils. In contrast, at acidic pH, the electron and proton transfer processes would be facilitated by the availability of uncomplexed metal ions, with subsequent insolubility of HALS+CS-pH 6, which would remain insoluble in soils during rainwater events or water runoff at pH 6, whereas HALS+CS-pH 1 would remain soluble and thus mobile and would be transported in ambient surface waters via rainwater, leaching, and groundwater infiltration (Ronchi et al., 2013; Stolpe et al., 2013; Mostofa et al., 2019).
The highly soluble FA and PLSs at acidic conditions would facilitate an easy transport to ambient surface waters via rainwater and groundwater discharge (Ronchi et al., 2013; Stolpe et al., 2013; Mostofa et al., 2019). Furthermore, the predominant presence of PLF peaks in PLSCS from pH 5–10 in paddy soil is indicative of solubility, whereas the relatively high degradability of PLSLS and PLSCS in maize soil may be attributed to the dry conditions (Mohinuzzaman et al., 2020).
Finally, the insolubility of individual HS components would arise when IFN > EFN, which would be related to the formation of hydrogen bonds between the HS functional groups and the aqueous phase, whereas the solubility of HS components would occur when EFN > IFN. Future research directions should focus on investigating acidic soils, which are beyond the scope of this study. These soils are expected to be significantly affected by the individual conditions of HA, FA, and PLSs in acidic environments. In conclusion, pH was confirmed to be a very important factor in determining the solubility or insolubility of HA, FA, and PLSs in soil and should be considered with the aim of preserving soil organic carbon.
Abbreviations | Full name |
DOC | Dissolved organic carbon |
DOM | Dissolved organic matter |
EEM | Excitation–emission matrix |
HSs | Humic substances |
HAs | Humic acids |
FAs | Fulvic acids |
PLSs | Protein-like substances |
PLF | Protein-like fluorescence |
HLF | Humic-like fluorescence |
EF | Electrochemical force |
IF | Intramolecular force |
EFN | Net EF |
IFN | Net IF |
LS | Labile state |
CS | Complexed state |
HSLS | LS HS |
HSCS | CS HS |
HALS-pH 6 | LS HA deposited at pH 6 |
HACS-pH 6 | CS HA deposited at pH 6 |
HALS-pH 1 | LS HA deposited at pH 1 |
HACS-pH 1 | CS HA deposited at pH 1 |
FALS | LS FA |
PLSLS | LS PLS |
FACS | CS FA |
PLSCS | CS PLS |
SOC | Soil organic carbon |
SOM | Soil organic matter |
The water and alkaline extraction procedures from soils are available in Mohinuzzaman et al. (2020). The EEM-PARAFAC model used to obtain fluorescent components from fluorescence spectra is available in Mostofa et al. (2019) and Yang et al. (2021).
The supplement related to this article is available online at https://doi.org/10.5194/bg-22-1745-2025-supplement.
KMGM and CQL designed, conceived, and supervised the project. XY performed the main experiments, analysed all data, and prepared all figures. JZ partly performed the extraction of humic acids (HAs), fulvic acids (FAs), and samples of protein-like substances (PLSs) from soil solution and conducted the elemental analyses and FTIR spectroscopy. MM performed the soil sampling and preprocessing. JY and XY conducted the EEM-PARAFAC analysis. KMGM, NS, and XY wrote the article; HHT and GSS reviewed and edited the article. All authors discussed the data and revised the article.
The contact author has declared that none of the authors has any competing interests.
Publisher's note: Copernicus Publications remains neutral with regard to jurisdictional claims made in the text, published maps, institutional affiliations, or any other geographical representation in this paper. While Copernicus Publications makes every effort to include appropriate place names, the final responsibility lies with the authors.
This work was financially supported by the Natural Science Foundation of China (grant nos. 42293262, 41925002, U1612441, and 42230509) and by the Key Construction Programme of the National “985” Project, Tianjin University, China.
This research has been supported by the National Natural Science Foundation of China (grant nos. 42293262, 41925002, U1612441, and 42230509).
This paper was edited by Jianming Xu and reviewed by four anonymous referees.
Aeschbacher, M., Sander, M., and Schwarzenbach, R. P.: Novel electrochemical approach to assess the redox properties of humic substances, Environ. Sci. Technol., 44, 87–93, https://doi.org/10.1021/es902627p, 2010.
Ai, Y., Zhao, C., Sun, L., Wang, X., and Liang, L.: Coagulation mechanisms of humic acid in metal ions solution under different pH conditions: A molecular dynamics simulation, Sci. Total Environ., 702, 135072, https://doi.org/10.1016/j.scitotenv.2019.135072, 2020.
Anastasiou, E., Lorentz, K. O., Stein, G. J., and Mitchell, P. D.: Prehistoric schistosomiasis parasite found in the Middle East, Lancet Infect. Dis., 14, 553–554, https://doi.org/10.1016/S1473-3099(14)70794-7, 2014.
Andersson, C. A. and Bro, R.: The N-way Toolbox for MATLAB, Chemometr. Intell. Lab., 52, 1–4, https://doi.org/10.1016/S0169-7439(00)00071-X, 2000.
Asli, S. and Neumann, P. M.: Rhizosphere humic acid interacts with root cell walls to reduce hydraulic conductivity and plant development, Plant Soil, 336, 313–322, https://doi.org/10.1007/s11104-010-0483-2, 2010.
Avena, M. J. and Wilkinson, K. J.: Disaggregation kinetics of a peat humic acid: Mechanism and pH effects, Environ. Sci. Technol., 36, 5100–5105, https://doi.org/10.1021/es025582u, 2002.
Benes, P.: Radiotracer study of thorium complexation with humic acid at pH 2–11 using free-liquid electrophoresis, Radiochim. Acta, 97, 273–281, https://doi.org/10.1524/ract.2009.1611, 2009.
Boguta, P., D'Orazio, V., Sokołowska, Z., and Senesi, N.: Effects of selected chemical and physicochemical properties of humic acids from peat soils on their interaction mechanisms with copper ions at various pH, J. Geochem. Explor., 168, 119–126, https://doi.org/10.1016/j.gexplo.2016.06.004, 2016.
Boguta, P., D'Orazio, V., Senesi, N., Sokołowska, Z., and Szewczuk-Karpisz, K.: Insight into the interaction mechanism of iron ions with soil humic acids. The effect of the pH and chemical properties of humic acids, J. Environ. Manage., 245, 367–374, https://doi.org/10.1016/j.jenvman.2019.05.098, 2019.
Bond-Lamberty, B. and Thomson, A.: Temperature-associated increases in the global soil respiration record, Nature, 464, 579–582, https://doi.org/10.1038/nature08930, 2010.
Brady, C. N. and Weil, R. R.: The Nature and Properties of Soils, 14th edn., 980 pp., ISBN 978-0-13-227938-3, 2008.
Bronick, C. J. and Lal, R.: Soil structure and management: A review, Geoderma, 124, 3–22, https://doi.org/10.1016/j.geoderma.2004.03.005, 2005.
Bryan, N. D., Abrahamsen, L., Evans, N., Warwick, P., Buckau, G., Weng, L., and Van Riemsdijk, W. H.: The effects of humic substances on the transport of radionuclides: Recent improvements in the prediction of behaviour and the understanding of mechanisms, Appl. Geochem., 27, 378–389, https://doi.org/10.1016/j.apgeochem.2011.09.008, 2012.
Canellas, L. P. and Olivares, F. L.: Physiological responses to humic substances as plant growth promoter, Chemical and Biological Technologies in Agriculture, 1, 1–11, https://doi.org/10.1186/2196-5641-1-3, 2014.
Chassapis, K., Roulia, M., and Nika, G.: Fe(III)-humate complexes from Megalopolis peaty lignite: A novel eco-friendly fertilizer, Fuel, 89, 1480–1484, https://doi.org/10.1016/j.fuel.2009.10.005, 2010.
Chen, C., Hall, S. J., Coward, E., and Thompson, A.: Iron-mediated organic matter decomposition in humid soils can counteract protection, Nat. Commun., 11, 1–13, https://doi.org/10.1038/s41467-020-16071-5, 2020.
Chen, H., Abdulla, H. A. N., Sanders, R. L., Myneni, S. C. B., Mopper, K., and Hatcher, P. G.: Production of Black Carbon-like and Aliphatic Molecules from Terrestrial Dissolved Organic Matter in the Presence of Sunlight and Iron, Environ. Sci. Tech. Let., 1, 399–404, https://doi.org/10.1021/ez5002598, 2014.
Chou, P. I., Ng, D. Q., Li, I. C., and Lin, Y. P.: Effects of dissolved oxygen, pH, salinity and humic acid on the release of metal ions from PbS, CuS and ZnS during a simulated storm event, Sci. Total Environ., 624, 1401–1410, https://doi.org/10.1016/j.scitotenv.2017.12.221, 2018.
Christl, I., Metzger, A., Heidmann, I., and Kretzschmar, R.: Effect of humic and fulvic acid concentrations and ionic strength on copper and lead binding, Environ. Sci. Technol., 39, 5319–5326, https://doi.org/10.1021/es050018f, 2005.
Ciceri, D. and Allanore, A.: Microfluidic leaching of soil minerals: Release of K+ from K feldspar, PLoS One, 10, 1–10, https://doi.org/10.1371/journal.pone.0139979, 2015.
Coble, P. G.: Characterization of marine and terrestrial DOM in sea water using excitation-emission matrix spectroscopy, Mar. Chem., 52, 325–346, 1996.
Coble, P. G., Green, S. A., Blough, N. V., and Gagosian, R. B.: Characterization of dissolved organic matter in the Black Sea by fluorescence spectroscopy, Nature, 348, 432–435, 1990.
Cory, R. M. and McKnight, D. M.: Fluorescence spectroscopy reveals ubiquitous presence of oxidized and reduced quinones in dissolved organic matter, Environ. Sci. Technol., 39, 8142–8149, https://doi.org/10.1021/ES0506962, 2005.
Crowther, T. W., Todd-Brown, K. E. O., Rowe, C. W., Wieder, W. R., Carey, J. C., Machmuller, M. B., Snoek, B. L., Fang, S., Zhou, G., Allison, S. D., Blair, J. M., Bridgham, S. D., Burton, A. J., Carrillo, Y., Reich, P. B., Clark, J. S., Classen, A. T., Dijkstra, F. A., Elberling, B., Emmett, B. A., Estiarte, M., Frey, S. D., Guo, J., Harte, J., Jiang, L., Johnson, B. R., Kroël-Dulay, G., Larsen, K. S., Laudon, H., Lavallee, J. M., Luo, Y., Lupascu, M., Ma, L. N., Marhan, S., Michelsen, A., Mohan, J., Niu, S., Pendall, E., Peñuelas, J., Pfeifer-Meister, L., Poll, C., Reinsch, S., Reynolds, L. L., Schmidt, I. K., Sistla, S., Sokol, N. W., Templer, P. H., Treseder, K. K., Welker, J. M., and Bradford, M. A.: Quantifying global soil carbon losses in response to warming, Nature, 540, 104–108, https://doi.org/10.1038/nature20150, 2016.
Curtin, D., Beare, M. H., Chantigny, M. H., and Greenfield, L. G.: Controls on the Extractability of Soil Organic Matter in Water over the 20 to 80 °C Temperature Range, Soil Sci. Soc. Am. J., 75, 1423–1430, https://doi.org/10.2136/sssaj2010.0401, 2011.
Davidson, E. A. and Janssens, I. A.: Temperature sensitivity of soil carbon decomposition and feedbacks to climate change, Nature, 440, 165–173, https://doi.org/10.1038/nature04514, 2006.
De la Rosa, J. M., Faria, S. R., Varela, M. E., Knicker, H., González-Vila, F. J., González-Pérez, J. A., and Keizer, J.: Characterization of wildfire effects on soil organic matter using analytical pyrolysis, Geoderma, 191, 24–30, https://doi.org/10.1016/J.GEODERMA.2012.01.032, 2012.
Demyan, M. S., Rasche, F., Schulz, E., Breulmann, M., Müller, T., and Cadisch, G.: Use of specific peaks obtained by diffuse reflectance Fourier transform mid-infrared spectroscopy to study the composition of organic matter in a Haplic Chernozem, Eur. J. Soil Sci., 63, 189–199, 2012.
dos Santos, J. V., Fregolente, L. G., Mounier, S., Hajjoul, H., Ferreira, O. P., Moreira, A. B., and Bisinoti, M. C.: Fulvic acids from Amazonian anthropogenic soils: Insight into the molecular composition and copper binding properties using fluorescence techniques, Ecotox. Environ. Safe., 205, 111173, https://doi.org/10.1016/j.ecoenv.2020.111173, 2020.
Drake, T. W., Van Oost, K., Barthel, M., Bauters, M., Hoyt, A. M., Podgorski, D. C., Six, J., Boeckx, P., Trumbore, S. E., Cizungu Ntaboba, L., and Spencer, R. G. M.: Mobilization of aged and biolabile soil carbon by tropical deforestation, Nat. Geosci., 12, 541–546, https://doi.org/10.1038/s41561-019-0384-9, 2019.
Dynarski, K. A., Bossio, D. A., and Scow, K. M.: Dynamic Stability of Soil Carbon: Reassessing the “Permanence” of Soil Carbon Sequestration, Front. Environ. Sci., 8, 514701, https://doi.org/10.3389/FENVS.2020.514701, 2020.
Ellerbrock, R. H., Gerke, H. H., and Deumlich, D.: Soil organic matter composition along a slope in an erosion-affected arable landscape in North East Germany, Soil Till. Res., 156, 209–218, https://doi.org/10.1016/J.STILL.2015.08.014, 2016.
Fang, C., Smith, P., Moncrieff, J. B., and Smith, J. U.: Similar response of labile and resistant soil organic matter pools to changes in temperature, Nature, 433, 57–59, https://doi.org/10.1038/nature03138, 2005.
Firestone, M. K., Killham, K., and McColl, J. G.: Fungal toxicity of mobilized soil aluminum and manganese, Appl. Environ. Microb., 46, 758–761, 1983.
Flis, S. E., Glenn, A. R., and M. J. Dilworth. The interaction between aluminium and root nodule bacteria, Soil Biol. Biochem., 25, 403–417, 1993.
Fulda, B., Voegelin, A., Maurer, F., Christl, I., and Kretzschmar, R.: Copper Redox Transformation and Complexation by Reduced and Oxidized Soil Humic Acid. 1. X-ray Absorption Spectroscopy Study, Environ. Sci. Technol., 47, 10903–10911, 2013.
Gabor, R. S., Burns, M. A., Lee, R. H., Elg, J. B., Kemper, C. J., Barnard, H. R., and McKnight, D. M.: Influence of leaching solution and catchment location on the fluorescence of water-soluble organic matter, Environ. Sci. Technol., 49, 4425–4432, https://doi.org/10.1021/es504881t, 2015.
Gao, J., Lv, J., Wu, H., Dai, Y., and Nasir, M.: Impacts of wheat straw addition on dissolved organic matter characteristics in cadmium-contaminated soils: Insights from fluorescence spectroscopy and environmental implications, Chemosphere, 193, 1027–1035, https://doi.org/10.1016/j.chemosphere.2017.11.112, 2018a.
Gao, L., Zhou, Z., Reyes, A. V., and Guo, L.: Yields and Characterization of Dissolved Organic Matter From Different Aged Soils in Northern Alaska, J. Geophys. Res.-Biogeo., 123, 2035–2052, https://doi.org/10.1029/2018JG004408, 2018b.
Gao, X., Zhang, J., Mostofa, K. M. G., Zheng, W., Liu, C. Q., Senesi, N., Senesi, G. S., Vione, D., Yuan, J, Liu, Y., Mohinuzzaman, M., Li, L., and Li, S. L.: Sulfur-mediated transformation, export and mineral complexation of organic and inorganic C, N, P and Si in dryland soils, Sci. Rep., 15, 9850, https://doi.org/10.1038/s41598-025-94920-3, 2025.
Garcia-Mina, J. M.: Stability, solubility and maximum metal binding capacity in metal-humic complexes involving humic substances extracted from peat and organic compost, Org. Geochem., 37, 1960–1972, https://doi.org/10.1016/j.orggeochem.2006.07.027, 2006.
Gilbert, B., Lu, G., and Kim, C. S.: Stable cluster formation in aqueous suspensions of iron oxyhydroxide nanoparticles, J. Colloid Interf. Sci., 313, 152–159, 2007.
Green, J. K., Seneviratne, S. I., Berg, A. M., Findell, K. L., Hagemann, S., Lawrence, D. M., and Gentine, P.: Large influence of soil moisture on long-term terrestrial carbon uptake, Nature, 565, 476–479, https://doi.org/10.1038/s41586-018-0848-x, 2019.
Haitzer, M., Aiken, G. R., and Ryan, J. N.: Binding of mercury(II) to dissolved organic matter: The role of the mercury-to-DOM concentration ratio, Environ. Sci. Technol., 36, 3564–3570, https://doi.org/10.1021/es025699i, 2002.
Haitzer, M., Aiken, G. R., and Ryan, J. N.: Binding of mercury(II) to aquatic humic substances: Influence of pH and source of humic substances, Environ. Sci. Technol., 37, 2436–2441, https://doi.org/10.1021/es026291o, 2003.
Harden, J. W., Hugelius, G., Ahlström, A., Blankinship, J. C., Bond-Lamberty, B., Lawrence, C. R., Loisel, J., Malhotra, A., Jackson, R. B., Ogle, S., Phillips, C., Ryals, R., Todd-Brown, K., Vargas, R., Vergara, S. E., Cotrufo, M. F., Keiluweit, M., Heckman, K. A., Crow, S. E., Silver, W. L., DeLonge, M., and Nave, L. E.: Networking our science to characterize the state, vulnerabilities, and management opportunities of soil organic matter, Glob. Change Biol., 24, e705–e718, https://doi.org/10.1111/gcb.13896, 2018.
Heckman, D. S., Geiser, D. M., Eidell, B. R., Stauffer, R. L., Kardos, N. L., and Hedges, S. B.: Molecular Evidence for the Early Colonization of Land by Fungi and Plants, Science, 293, 1129–1133, https://doi.org/10.1126/science.1061457, 2001.
Heitmann, T., Goldhammer, T., Beer, J., and Blodau, C.: Electron transfer of dissolved organic matter and its potential significance for anaerobic respiration in a northern bog, Glob. Change Biol., 13, 1771–1785, https://doi.org/10.1111/j.1365-2486.2007.01382.x, 2007.
Helms, J. R., Mao, J., Schmidt-Rohr, K., Abdulla, H., and Mopper, K.: Photochemical flocculation of terrestrial dissolved organic matter and iron, Geochim. Cosmochim. Ac., 121, 398–413, https://doi.org/10.1016/j.gca.2013.07.025, 2013.
Hemingway, J. D., Rothman, D. H., Grant, K. E., Rosengard, S. Z., Eglinton, T. I., Derry, L. A., and Galy, V. V.: Mineral protection regulates long-term global preservation of natural organic carbon, Nature, 570, 228–231, https://doi.org/10.1038/s41586-019-1280-6, 2019.
Hernández, D., Plaza, C., Senesi, N., and Polo, A.: Detection of Copper(II) and zinc(II) binding to humic acids from pig slurry and amended soils by fluorescence spectroscopy, Environ. Pollut., 143, 212–220, https://doi.org/10.1016/j.envpol.2005.11.038, 2006.
Huang, W. and Hall, S. J.: Elevated moisture stimulates carbon loss from mineral soils by releasing protected organic matter, Nat. Commun., 8, 1774, https://doi.org/10.1038/s41467-017-01998-z, 2017.
Jiang, T., Skyllberg, U., Wei, S., Wang, D., Lu, S., Jiang, Z., and Flanagan, D. C.: Modeling of the structure-specific kinetics of abiotic, dark reduction of Hg(II) complexed by ON and S functional groups in humic acids while accounting for time-dependent structural rearrangement, Geochim. Cosmochim. Ac., 154, 151–167, https://doi.org/10.1016/j.gca.2015.01.011, 2015.
Jones, K. D. and Tiller, C. L.: Effect of solution chemistry on the extent of binding of phenanthrene by a soil humic acid: A comparison of dissolved and clay bound humic, Environ. Sci. Technol., 33, 580–587, https://doi.org/10.1021/es9803207, 1999.
Jovanović, U. D., Marković, M. M., Cupać, S. B., and Tomić, Z. P: Soil humic acid aggregation by dynamic light scattering and laser Doppler electrophoresis, J. Plant Nutr. Soil Sc., 176, 674–679, https://doi.org/10.1002/JPLN.201200346, 2013.
Kallenbach, C., Frey, S., and Grandy, A.: Direct evidence for microbial-derived soil organic matter formation and its ecophysiological controls, Nat. Commun., 7, 13630, https://doi.org/10.1038/ncomms13630, 2016..
Karadirek, Ş., Kanmaz, N., Balta, Z., Demirçivi, P., Üzer, A., Hizal, J., and Apak, R.: Determination of total antioxidant capacity of humic acids using CUPRAC, Folin–Ciocalteu, noble metal nanoparticle- and solid–liquid extraction-based methods, Talanta, 153, 120–129, https://doi.org/10.1016/J.TALANTA.2016.03.006, 2016.
Kelly, B., Carrizo, G. E., Edwards-Hicks, J., Sanin, D. E., Stanczak, M. A., Priesnitz, C., Flachsmann, L. J., Curtis, J. D., Mittler, G., Musa, Y., Becker, T., Buescher, J. M., and Pearce, E. L.: Sulfur sequestration promotes multicellularity during nutrient limitation, Nature, 591, 471–476, https://doi.org/10.1038/s41586-021-03270-3, 2021.
Kirsten, M., Mikutta, R., Vogel, C., Thompson, A., Mueller, C. W., Kimaro, D. N., Bergsma, H. L. T., Feger, K. H., and Kalbitz, K.: Iron oxides and aluminous clays selectively control soil carbon storage and stability in the humid tropics, Sci. Rep., 11, 1–12, https://doi.org/10.1038/s41598-021-84777-7, 2021.
Klapper, L., McKnight, D. M., Fulton, J. R., Blunt-Harris, E. L., Nevin, K. P., Lovley, D. R., and Hatcher, P. G.: Fulvic acid oxidation state detection using fluorescence spectroscopy, Environ. Sci. Technol., 36, 3170–3175, https://doi.org/10.1021/ES0109702, 2002.
Kleber, M., Sollins, P., and Sutton, R.: A conceptual model of organo-mineral interactions in soils: Self-assembly of organic molecular fragments into zonal structures on mineral surfaces, Biogeochemistry, 85, 9–24, https://doi.org/10.1007/s10533-007-9103-5, 2007.
Kleber, M., Bourg, I. C., Coward, E. K., Hansel, C. M., Myneni, S. C. B., and Nunan, N.: Dynamic interactions at the mineral–organic matter interface, Nature Reviews Earth and Environment 6, 402–421, https://doi.org/10.1038/s43017-021-00162-y, 2021.
Klüpfel, L., Piepenbrock, A., Kappler, A., and Sander, M.: Humic substances as fully regenerable electron acceptors in recurrently anoxic environments, Nat. Geosci., 7, 195–200, https://doi.org/10.1038/ngeo2084, 2014.
Kothawala, D. N., Murphy, K. R., Stedmon, C. A., Weyhenmeyer, G. A., and Tranvik, L. J.: Inner filter correction of dissolved organic matter fluorescence, Limnol. Oceanogr.-Meth., 11, 616–630, https://doi.org/10.4319/lom.2013.11.616, 2013.
Kunlanit, B., Vityakon, P., Puttaso, A., Cadisch, G., and Rasche, F.: Mechanisms controlling soil organic carbon composition pertaining to microbial decomposition of biochemically contrasting organic residues: Evidence from midDRIFTS peak area analysis, Soil Biol. Biochem., 76, 100–108, 2014.
Lalonde, K., Mucci, A., Ouellet, A., and Gélinas, Y.: Preservation of organic matter in sediments promoted by iron, Nature, 483, 198–200, https://doi.org/10.1038/nature10855, 2012.
Lange, O. L., Belnap, J., and Reichenberger, H.: Photosynthesis of the cyanobacterial soil-crust lichen Collema tenax from arid lands in southern Utah, USA: Role of water content on light and temperature responses of CO2 exchange, Funct. Ecol., 12, 195–202, 1998.
Leenheer, J. A., Wershaw, R. L., and Reddy, M. M.: Strong-Acid, Cariioxyl-Group Structures in Fulvic Acid from the Suwannee River, Georgia. 2. Major Structures, Environ. Sci. Technol., 29, 399–405, https://doi.org/10.1021/es00002a016, 1995.
Lehmann, J. and Kleber, M.: The contentious nature of soil organic matter, Nature, 528, 60–68, https://doi.org/10.1038/nature16069, 2015.
Levicán, G., Ugalde, J. A., Ehrenfeld, N., Alejandro Maass, A., and Parada, P.: Comparative genomic analysis of carbon and nitrogen assimilation mechanisms in three indigenous bioleaching bacteria: predictions and validations, BMC Genomics, 9, 581, https://doi.org/10.1186/1471-2164-9-581, 2008.
Li, H. and Vaughan, J. C.: Switchable Fluorophores for Single-Molecule Localization Microscopy, Chem. Rev., 118, 9412–9454, https://doi.org/10.1021/acs.chemrev.7b00767, 2018.
Li, Q., Chen, X., Veroustraete, F., Bao, A. M., Liu, T., and Wang, J. L.: Validation of soil moisture retrieval in arid and semi-arid areas, Shuikexue Jinzhan/Advances in Water Science, 21, 201–207, 2010.
Lippold, H., Evans, N. D. M., Warwick, P., and Kupsch, H.: Competitive effect of iron(III) on metal complexation by humic substances: Characterisation of ageing processes, Chemosphere, 67, 1050–1056, https://doi.org/10.1016/j.chemosphere.2006.10.045, 2007.
Liu, J., Mu, Y., Geng, C., Yu, Y., He, H., and Zhang, Y.: Uptake and conversion of carbonyl sulfide in a lawn soil, Atmos. Environ., 41, 5697–5706, https://doi.org/10.1016/j.atmosenv.2007.02.039, 2007.
Lundström, U. S., Van Breemen, N., and Bain, D.: The podzolization process. A review, Geoderma, 94, 91–107, https://doi.org/10.1016/S0016-7061(99)00036-1, 2000.
Lützow, M. V., Kögel-Knabner, I., Ekschmitt, K., Matzner, E., Guggenberger, G., Marschner, B., and Flessa, H.: Stabilization of organic matter in temperate soils: Mechanisms and their relevance under different soil conditions – A review, Eur. J. Soil Sci., 57, 426–445, https://doi.org/10.1111/j.1365-2389.2006.00809.x, 2006.
Ma, H., Mao, P., Imran, S., Raza, T., Gao, R., and Lin, Y.: Rice Planting Increases Biological Nitrogen Fixation in Acidic Soil and the Influence of Light and Flood Layer Thickness, J. Soil Sci. Plant Nut., 21, 341–348, 2021.
Makiel, M., Skiba, M., Kisiel, M., Maj-Szeliga, K., Błachowski, A., Szymański, W., and Salata, D.: Formation of iron oxyhydroxides as a result of glauconite weathering in soils of temperate climate, Geoderma, 416, 115780, https://doi.org/10.1016/J.GEODERMA.2022.115780, 2022.
Malik, A. A., Puissant, J., Buckeridge, K. M., Goodall, T., Jehmlich, N., Chowdhury, S., Gweon, H. S., Peyton, J. M., Mason, K. E., van Agtmaal, M., Blaud, A., Clark, I. M., Whitaker, J., Pywell, R. F., Ostle, N., Gleixner, G., and Griffiths, R. I.: Land use driven change in soil pH affects microbial carbon cycling processes, Nat. Commun., 9, 3591, https://doi.org/10.1038/s41467-018-05980-1, 2018.
Marschner, B., Brodowski, S., Dreves, A., Gleixner, G., Gude, A., Grootes, P. M., Hamer, U., Heim, A., Jandl, G., Ji, R., Kaiser, K., Kalbitz, K., Kramer, C., Leinweber, P., Rethemeyer, J., Schäffer, A., Schmidt, M. W. I., Schwark, L., and Wiesenberg, G. L. B.: How relevant is recalcitrance for the stabilization of organic matter in soils?, J. Plant Nutr. Soil Sc., 171, 91–110, https://doi.org/10.1002/jpln.200700049, 2008.
Masaki, Y., Ozawa, R., Kageyama, K., and Katayama, Y.: Degradation and emission of carbonyl sulfide, an atmospheric trace gas, by fungi isolated from forest soil, FEMS Microbiol. Lett., 363, 3–5, https://doi.org/10.1093/femsle/fnw197, 2016.
Min, K., Lehmeier, C. A., Ballantyne, F., Tatarko, A., and Billings, S. A.: Differential effects of pH on temperature sensitivity of organic carbon and nitrogen decay, Soil Biol. Biochem., 76, 193–200, https://doi.org/10.1016/J.SOILBIO.2014.05.021, 2014.
Mohinuzzaman, M., Yuan, J., Yang, X., Senesi, N., Li, S. L., Ellam, R. M., Mostofa, K. M. G., and Liu, C. Q.: Insights into solubility of soil humic substances and their fluorescence characterisation in three characteristic soils, Sci. Total Environ., 720, 1–38, https://doi.org/10.1016/j.scitotenv.2020.137395, 2020.
Mora, V., Baigorri, R., Bacaicoa, E., Zamarreño, A. M., and García-Mina, J. M.: The humic acid-induced changes in the root concentration of nitric oxide, IAA and ethylene do not explain the changes in root architecture caused by humic acid in cucumber, Environ. Exp. Bot., 76, 24–32, https://doi.org/10.1016/j.envexpbot.2011.10.001, 2012.
Mostofa, K. M. G., Yoshioka, T., Mottaleb, M. A., and Vione, D.: Photobiogeochemistry of Organic Matter: Principles and Practices in Water Environments, Springer, Berlin, Germany, https://doi.org/10.1007/978-3-642-32223-5, 2013.
Mostofa, K. M. G., Li, W., Wu, F., Liu, C. Q., Liao, H., Zeng, L., and Xiao, M.: Environmental characteristics and changes of sediment pore water dissolved organic matter in four Chinese lakes, Environ. Sci. Pollut. R., 25, 2783–2804, https://doi.org/10.1007/s11356-017-0545-6, 2018.
Mostofa, K. M. G., Jie, Y., Sakugawa, H., and Liu, C. Q.: Equal Treatment of Different EEM Data on PARAFAC Modeling Produces Artifact Fluorescent Components That Have Misleading Biogeochemical Consequences, Environ. Sci. Technol., 53, 561–563, https://doi.org/10.1021/acs.est.8b06647, 2019.
Noy, A., Vezenov, D., and Lieber, C.: Chemical force microscopy Annu. Rev. Mater. Sci., 27, 381–421, https://doi.org/10.1146/annurev.matsci.27.1.381, 1997.
Nurmi, J. T. and Tratnyek, P. G.: Electrochemical properties of natural organic matter (NOM), fractions of NOM, and model biogeochemical electron shuttles, Environ. Sci. Technol., 36, 617–624, https://doi.org/10.1021/ES0110731, 2002.
Paul, E. A.: The nature and dynamics of soil organic matter: Plant inputs, microbial transformations, and organic matter stabilization, Soil Biol. Biochem., 98, 109–126, 2016.
Peinemann, N., Guggenberger, G., and Zech, W.: Soil organic matter and its lignin component in surface horizons of salt-affected soils of the Argentinian Pampa, Catena, 60, 113–128, https://doi.org/10.1016/J.CATENA.2004.11.008, 2005.
Pietikäinen, J., Pettersson, M., and Bååth, E.: Comparison of temperature effects on soil respiration and bacterial and fungal growth rates, FEMS Microbiol. Ecol., 52, 49–58, https://doi.org/10.1016/j.femsec.2004.10.002, 2005.
Ritchie, J. D. and Michael Perdue, E.: Proton-binding study of standard and reference fulvic acids, humic acids, and natural organic matter, Geochim. Cosmochim. Ac., 67, 85–96, https://doi.org/10.1016/S0016-7037(02)01044-X, 2003.
Robarge, W. P.: Precipitation/dissolution reactions in soils, in: Soil Physical Chemistry, 2nd edn., edited by: Sparks, D. L., CRC Press, Boca Raton, 193–238, https://doi.org/10.1201/9780203739280, 2018.
Ronchi, B., Clymans, W., Barão, A. L. P., Vandevenne, F., Struyf, E., Batelaan, O., Dassargues, A., and Govers, G.: Transport of Dissolved Si from Soil to River: A Conceptual Mechanistic Model, Silicon-Neth, 5, 115–133, https://doi.org/10.1007/s12633-012-9138-7, 2013.
Rousk, J., Brookes, P. C., and Bååth, E.: Contrasting soil pH effects on fungal and bacterial growth suggest functional redundancy in carbon mineralization, Appl. Environ. Microb., 75, 1589–1596, https://doi.org/10.1128/AEM.02775-08, 2009.
Saito, T., Nagasaki, S., and Tanaka, S.: Molecular fluorescence spectroscopy and mixture analysis for the evaluation of the complexation between humic acid and , Radiochim. Acta, 90, 545–548, https://doi.org/10.1524/ract.2002.90.9-11_2002.545, 2002.
Schad, P., van Huyssteen, C., and Michéli, E.: World Reference Base for Soil Resources 2014, Organización de las Naciones Unidas para la Alimentación y la Agricultura (FAO), Update 2015, ISBN 978-92-5-108369-7, 978-92-5-108370-3, http://www.fao.org/3/i3794en/I3794en.pdf (last access: 30 March 2025), 2015.
Schmidt, W., Santi, S., Pinton, R., and Varanini, Z.: Water-extractable humic substances alter root development and epidermal cell pattern in Arabidopsis, Plant Soil, 300, 259–267, https://doi.org/10.1007/s11104-007-9411-5, 2007.
Senesi, N.: Molecular and quantitative aspects of the chemistry of fulvic acid and its interactions with metal ions and organic chemicals. Part II. The fluorescence spectroscopy approach, Anal. Chim. Acta, 232, 77–106, https://doi.org/10.1016/S0003-2670(00)81226-X, 1990a.
Senesi, N.: Molecular and quantitative aspects of the chemistry of fulvic acid and its interactions with metal ions and organic chemicals. Part I. The electron spin resonance approach, Anal. Chim. Acta, 232, 51–75, https://doi.org/10.1016/S0003-2670(00)81225-8, 1990b.
Senesi, N. and Loffredo, E.: The Chemistry of Soil Organic Matter in Soil Physical Chemistry, 2nd edn., https://doi.org/10.1201/9780203739280, 1999.
Senesi, N. and Plaza, C.: Role of humification processes in recycling organic wastes of various nature and sources as soil amendments, Clean-Soil Air Water, 35, 26–41, https://doi.org/10.1002/clen.200600018, 2007.
Senesi, N., D'Orazio, V., and Ricca, G.: Humic acids in the first generation of EUROSOILS, Geoderma, 116, 325–344, https://doi.org/10.1016/S0016-7061(03)00107-1, 2003.
Shammi, M., Pan, X., Mostofa, K. M. G., Zhang, D., Liu, C. Q.: Photo-flocculation of algal biofilm extracellular polymeric substances and its transformation into transparent exopolymer particles. Chemical and spectroscopic evidences, Sci. Rep, 7, 9074, https://doi.org/10.1038/s41598-017-09066-8, 2017.
Singh, R. P., Shukla, M. K., Mishra, A., Kumari, P., Reddy, C. R. K., and Jha, B.: Isolation and characterization of exopolysaccharides from seaweed associated bacteria Bacillus licheniformis, Carbohyd. Polym., 84, 1019–1026, 2011.
Six, J., Conant, R. T., Paul, E. A., and Paustian, K.: Stabilization mechanisms of soil organic matter: Implications for C-saturation of soils, Plant Soil, 241, 155–176, 2002.
Sollins, P., Homann, P., and Caldwell, B. A.: Stabilization and destabilization of soil organic matter: mechanisms and controls Phillip, Geoderma, 74, 65–105, 1996.
Song, Z., McGrouther, K., and Wang, H.: Occurrence, turnover and carbon sequestration potential of phytoliths in terrestrial ecosystems, Earth-Sci. Rev., 158, 19–30, https://doi.org/10.1016/j.earscirev.2016.04.007, 2016.
Soti, P. G., Jayachandran, K., Koptur, S., and Volin, J. C.: Effect of soil pH on growth, nutrient uptake, and mycorrhizal colonization in exotic invasive Lygodium microphyllum, Plant Ecol., 216, 989–998, 2015.
Spence, A. and Kelleher, B. P.: Photodegradation of major soil microbial biomolecules is comparable to biodegradation: Insights from infrared and diffusion editing NMR spectroscopies, J. Mol. Struct., 1107, 7–13, https://doi.org/10.1016/j.molstruc.2015.11.025, 2016.
Stedmon, C. A., Markager, S., and Bro, R.: Tracing dissolved organic matter in aquatic environments using a new approach to fluorescence spectroscopy, Mar. Chem., 82, 239–254, https://doi.org/10.1016/S0304-4203(03)00072-0, 2003.
Steinmuller, H. E. and Chambers, L. G.: Characterization of coastal wetland soil organic matter: Implications for wetland submergence, Sci. Total Environ., 677, 648–659, https://doi.org/10.1016/J.SCITOTENV.2019.04.405, 2019.
Stolpe, B., Guo, L., and Shiller, A. M.: Binding and transport of rare earth elements by organic and iron-rich nanocolloids in alaskan rivers, as revealed by field-flow fractionation and ICP-MS, Geochim. Cosmochim. Ac., 106, 446–462, https://doi.org/10.1016/j.gca.2012.12.033, 2013.
Szulczewski, M. D., Helmke, P. A., and Bleam, W. F.: XANES spectroscopy studies of Cr(VI) reduction by thiols in organosulfur compounds and humic substances, Environ. Sci. Technol., 35, 1134–1141, https://doi.org/10.1021/es001301b, 2001.
Tadini, A. M., Nicolodelli, G., Senesi, G. S., Ishida, D. A., Montes, C. R., Lucas, Y., Mounier, S., Guimarães, F. E. G., and Milori, D. M. B. P.: Soil organic matter in podzol horizons of the Amazon region: Humification, recalcitrance, and dating, Sci. Total Environ., 613–614, 160–167, https://doi.org/10.1016/j.scitotenv.2017.09.068, 2018.
Tadini, A. M., Mounier, S., and Milori, D. M. B. P.: Modeling the quenching of fluorescence from organic matter in Amazonian soils, Sci. Total Environ., 698, 134067, https://doi.org/10.1016/j.scitotenv.2019.134067, 2020.
Tremblay, L., Kohl, S. D., Rice, J. A., and Gagné, J. P.: Effects of temperature, salinity, and dissolved humic substances on the sorption of polycyclic aromatic hydrocarbons to estuarine particles, Mar. Chem., 96, 21–34, https://doi.org/10.1016/j.marchem.2004.10.004, 2005.
Trevisan, S., Pizzeghello, D., Ruperti, B., Francioso, O., Sassi, A., Palme, K., Quaggiotti, S., and Nardi, S.: Humic substances induce lateral root formation and expression of the early auxin-responsive IAA19 gene and DR5 synthetic element in Arabidopsis, Plant Biol., 12, 604–614, https://doi.org/10.1111/j.1438-8677.2009.00248.x, 2010.
Underwood, T. R., Bourg, I. C., and Rosso, K. M.: Mineral-associated organic matter is heterogeneous and structured by hydrophobic, charged, and polar interactions, P. Natl. Acad. Sci. USA, 121, e2413216121, https://doi.org/10.1073/pnas.2413216121, 2024.
Varghese, E. M., Kour, B., Ramya, S., Krishna, P. D., Nazla, K. A., Sudheer, K., Anith, K. N., Jisha, M. S., and Ramakrishnan, B.: Rice in acid sulphate soils: Role of microbial interactions in crop and soil health management, Appl. Soil Ecol., 196, 105309, https://doi.org/10.1016/j.apsoil.2024.105309, 2024.
Vezenov, D. V., Noy, A., Rozsnyai, L. F., and Lieber, C. M.: Force titrations and ionization state sensitive imaging of functional groups in aqueous solutions by chemical force microscopy, J. Am. Chem. Soc., 119, 2006–2015, https://doi.org/10.1021/ja963375m, 1997.
Vezenov, D. V., Noy, A., and Ashby, P.: Chemical force microscopy: Probing chemical origin of interfacial forces and adhesion, J. Adhes. Sci. Technol., 19, 313–364, https://doi.org/10.1163/1568561054352702, 2005.
Vidali, R., Remoundaki, E., and Tsezos, M.: Humic acids copper binding following their photochemical alteration by simulated solar light, Aquat. Geochem., 16, 207–218, https://doi.org/10.1007/s10498-009-9080-5, 2010.
Vogel, C., Mueller, C. W., Höschen, C., Buegger, F., Heister, K., Schulz, S., Schloter, M., and Kögel-Knabner, I.: Submicron structures provide preferential spots for carbon and nitrogen sequestration in soils, Nat. Commun., 5, 1–7, https://doi.org/10.1038/ncomms3947, 2014.
Wang, C., Cheng, T., Zhang, D., and Pan, X.: Electrochemical properties of humic acid and its novel applications: A tip of the iceberg, Sci. Total Environ., 863, 160755, https://doi.org/10.1016/J.SCITOTENV.2022.160755, 2023.
Wang, L. F., Wang, L. L., Ye, X. D., Li, W. W., Ren, X. M., Sheng, G. P., Yu, H. Q., and Wang, X. K.: Coagulation kinetics of humic aggregates in mono- and Di-valent electrolyte solutions, Environ. Sci. Technol., 47, 5042–5049, https://doi.org/10.1021/es304993j, 2013.
Ward, N. D., Keil, R. G., Medeiros, P. M., Brito, D. C., Cunha, A. C., Dittmar, T., Yager, P. L., Krusche, A. V., and Richey, J. E.: Degradation of terrestrially derived macromolecules in the Amazon River, Nat. Geosci., 6, 530–533, https://doi.org/10.1038/NGEO1817, 2013.
Whalen, E. D., Grandy, A. S., Geyer, K. M., Morrison, E. W., and Frey, S. D.: Microbial trait multifunctionality drives soil organic matter formation potential, Nat. Commun., 15, 10209, https://doi.org/10.1038/s41467-024-53947-2, 2024.
Whelan, M. E. and Rhew, R. C.: Carbonyl sulfide produced by abiotic thermal and photodegradation of soil organic matter from wheat field substrate, J. Geophys. Res.-Biogeo., 120, 54–62, https://doi.org/10.1002/2014JG002661, 2015.
Wu, F., Cai, Y., Evans, D., and Dillon, P.: Complexation between Hg(II) and Dissolved Organic Matter in Stream Waters: An Application of Fluorescence Spectroscopy, Biogeochemistry, 71, 339–351, 2004a.
Wu, F., Mills, R. B., Evans, R. D., and Dillon, P. J.: Kinetics of Metal–Fulvic Acid Complexation Using a Stopped-Flow Technique and Three-Dimensional Excitation Emission Fluorescence Spectrophotometer, Anal. Chem., 76, 110–113, 2004b.
Xi, M., Zi, Y., Wang, Q., Wang, S., Cui, G., and Kong, F.: Assessment of the content, structure, and source of soil dissolved organic matter in the coastal wetlands of Jiaozhou Bay, China, Phys. Chem. Earth, 103, 35–44, https://doi.org/10.1016/j.pce.2017.03.004, 2018.
Xie, H., Zafiriou, O. C., Cai, W. J., Zepp, R. G., and Wang, Y.: Photooxidation and its effects on the carboxyl content of dissolved organic matter in two coastal rivers in the southeastern United States, Environ. Sci. Technol., 38, 4113–4119, https://doi.org/10.1021/es035407t, 2004.
Yang, X., Yuan, J., Yue, F. J., Li, S. L., Wang, B., Mohinuzzaman, M., Liu, Y., Senesi, N., Lao, X., Li, L., Liu, C. Q., Ellam, R. M., Vione, D., and Mostofa, K. M. G.: New insights into mechanisms of sunlight- and dark-mediated high-temperature accelerated diurnal production-degradation of fluorescent DOM in lake waters, Sci. Total Environ., 760, 143377, https://doi.org/10.1016/j.scitotenv.2020.143377, 2021.
Yang, X., Gao, X., Mostofa, K. M. G., Zheng, W., Senesi, N., Senesi, G. S., Vione, D., Yuan, J., Li, S. L., Li, L., and Liu, C. Q.: Mineral states and sequestration processes involving soil biogenic components in various soils and desert sands of Inner Mongolia, Sci. Rep., 14, 28530, https://doi.org/10.1038/s41598-024-80004-1, 2024.
Yang, Z., Kappler, A., and Jiang, J.: Reducing capacities and distribution of redox-active functional groups in low molecular weight fractions of humic acids, Environ. Sci. Technol., 50, 12105–12113, https://doi.org/10.1021/ACS.EST.6B02645, 2016.
Yu, G. H., Chi, Z. L., Kappler, A., Sun, F. S., Liu, C. Q., Teng, H. H., and Gadd, G. M.: Fungal Nanophase Particles Catalyze Iron Transformation for Oxidative Stress Removal and Iron Acquisition, Curr. Biol., 30, 2943–2950.e4, https://doi.org/10.1016/j.cub.2020.05.058, 2020.
Zhang, D., Pan, X., Mostofa, K. M. G., Chen, X., Mu, G., Wu, F., Liu, J., Song, W., Yang, J., Liu, Y., and Fu, Q.: Complexation between Hg(II) and biofilm extracellular polymeric substances: An application of fluorescence spectroscopy, J. Hazard. Mater., 175, 359–365, https://doi.org/10.1016/j.jhazmat.2009.10.011, 2010.
Zhang, J., Mostofa, K. M. G., Yang, X., Mohinuzzaman, M., Liu, C. Q., Senesi, N., Senesi, G. S., Sparks, D. L., Teng, H. H., Li, L., Yuan, J., and Li, S. L.: Isolation of dissolved organic matter from aqueous solution by precipitation with FeCl3: mechanisms and significance in environmental perspectives, Sci. Rep., 13, 1–15, https://doi.org/10.1038/s41598-023-31831-1, 2023.
Zhu, B. and Ryan, D. K.: Characterizing the interaction between uranyl ion and fulvic acid using regional integration analysis (RIA) and fluorescence quenching, J. Environ. Radioactiv., 153, 97–103, https://doi.org/10.1016/j.jenvrad.2015.12.004, 2016.