the Creative Commons Attribution 4.0 License.
the Creative Commons Attribution 4.0 License.
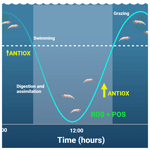
Reviews and syntheses: On increasing hypoxia in eastern boundary upwelling systems – zooplankton under metabolic stress
Leissing Frederick
Mauricio A. Urbina
Eastern boundary upwelling systems (EBUSs) are ecologically and economically important marine regions of the world ocean. In these systems, zooplankton play a pivotal role in transferring primary production up through the food web. Recent studies show that global warming is causing a gradual deoxygenation of the world ocean, while in EBUSs, an expansion and intensification of the subsurface oxygen minimum zone (OMZ) is taking place, further exacerbating hypoxic conditions for zooplankton inhabiting the upwelling zone. Hypoxia can affect zooplankton by limiting their aerobic respiration and constraining the migration, energy budget, reproduction, and development. These effects, however, depend on some specific adaptations evolved in habitats, permanently or episodically, subjected to low-oxygen waters. Various metabolic, physiological, behavioural, and morphological adaptations have been described in zooplankton interacting with the OMZ. Adjustment of the aerobic respiration under variable oxygen levels deserves special attention since such adaptive responses to endure mild or severe hypoxia may involve trade-offs in energy usage that impact other metabolic functions or energy-demanding processes. In addition, the oxidative stress resulting from exposure to highly fluctuating oxygen conditions in the upwelling zone can impose further energy expenses. New demands imply a reduction in the energy budget otherwise available for escape, migration, growth, feeding, and reproduction with further ecological consequences for population and community dynamics. This paper reviews and explores the existence or lack of such adaptive metabolic responses along with potential effects of oxidative stress and their role in zooplankton dynamics in EBUSs with major consequences for the pelagic food web and biological productivity.
- Article
(4841 KB) - Full-text XML
- BibTeX
- EndNote
It is widely recognised that the increase in atmospheric CO2 and other greenhouse gases is driving the warming of the Earth's surface and ocean (Oschlies et al., 2018) with several other physical consequences, such as increased events of strong winds, storms in some regions, and changes in ocean circulation (Schmidtko et al., 2017). The warming of the upper layers of the ocean drives greater stratification of the water column, reduces vertical mixing, and affects ocean ventilation. Additionally, a warmer ocean lowers oxygen solubility and increases metabolic demands of ectotherms (Breitburg et al., 2018), further challenging marine life. In this scenario, deoxygenation has led to approximately a 2 % loss of oxygen in the open ocean and coastal waters since the mid-20th century (Stramma et al., 2008; Schmidtko et al., 2017; Breitburg et al., 2018).
The persistent and expanding oxygen minimum zones (OMZs), characterised by extremely low oxygen concentrations (<20–45 µmol kg−1) (Stramma et al., 2008; Grégoire et al., 2021) and associated with highly productive coastal and oceanic regions (Gilly et al., 2013), are becoming increasingly critical as they reach shallower depths. This shoaling of the upper boundary, along with the descent of the lower boundary, has led to a vertical expansion and increased the total volume of OMZs (Stramma et al., 2010). These changes reduce the vertical habitat available for zooplankton, limiting their distribution and potentially altering their ecological roles (Köhn et al., 2022). Additionally, further reductions in oxygen concentrations within OMZ cores have been observed, intensifying their effects on aerobic organisms (Chan et al., 2008). Also, extreme events caused by mesoscale eddies can produce intense episodes of hypoxia in the upwelling zone (Köhn et al., 2022) and even transport OMZ waters off the coast (Auger et al., 2021).
In some areas, mainly at mid-latitudes, of the four major eastern boundary current systems (EBUSs) (Chavez and Messié, 2009), the effect of climate change has been associated with an intensification of the physical forcings driving coastal upwelling (Bakun et al., 2010; Xiu et al., 2018; Bograd et al., 2023), leading to several changes in the physical-chemical properties of the water column, including a gradual cooling in the last few decades (Santos et al., 2012; Schneider et al., 2016). However, other studies have found no evidence of increasing upwelling or trends in upwelling intensity based on time series observations for several decades in the same EBUSs (e.g. Pardo et al., 2011; Bode et al., 2019). Trends of upwelling intensity in EBUSs are therefore still a matter of controversy, and the predictive models reveal a lot of uncertainty in the future of upwelling regarding its spatial and temporal variability (Bograd et al., 2023). Upon a potential increase in upwelling, alongshore winds in EBUSs bring colder water and more frequent occurrences of upwelling events (Breitburg et al., 2018), although some modelling work also suggests an extension of the upwelling period and spatial homogenisation of upwelling alongshore (Wang et al., 2015). Stronger upwelling is ultimately thought to be a response to the strengthening of large-scale pressure gradients linked to global-scale climate change (Garcia-Reyes and Largier, 2009). The closely linked effects of potentially increasing upwelling, cooling of the water column, and shoaling of the OMZ in EBUSs driven by global warming are illustrated in Fig. 1.
The ongoing combined processes, deoxygenation, increasing upwelling, and OMZ expansion will alter the oxygen conditions in the upper layers (<50 m) in EBUSs, where plankton concentrate, with various ecological and biogeochemical consequences. In this respect, Ekau et al. (2010) demonstrated that hypoxic conditions can alter the zooplankton community composition in the Benguela EBUS. This is due to variable tolerances to hypoxia in distinctive groups, such as euphausiids, which better adapted to low oxygen (<0.1 mL L−1) compared to copepods. Escribano et al. (2009) also described a strong vertical zonation of zooplankton depending on variable tolerance to hypoxia in the northern upwelling zone of Chile. Variable tolerance to hypoxia is also reflected in some species-dependent physiological rates of copepods, as found in the calanoids Acartia tonsa and Calanus chilensis, whose egg production rate and hatching success were strongly and positively correlated to oxygen concentration under laboratory conditions (Ruz et al., 2015; Choi et al., 2024). In the same context, not only vertical distribution, but also the vertical amplitude of the diel vertical migration can be strongly modulated by hypoxic conditions forced by the position of the OMZ core and its upper boundary (Tutasi and Escribano, 2020; Riquelme-Bugueño et al., 2020; Wishner et al., 2020).
Aerobic metazooplankton inhabiting the upwelling zone is thus expected to be exposed to variable levels of oxygenation from normoxia to mild or severe hypoxia, depending on their distribution and migrating behaviour. Their responses also depend on the existence, absence, or development of new adaptations. In this paper, we review such adaptive responses of zooplankton and the ecological consequences driven by hypoxia, aiming at establishing the physiological and metabolic bases and directions when addressing issues related to the future of zooplankton dynamics in EBUSs subjected to ongoing climate change.
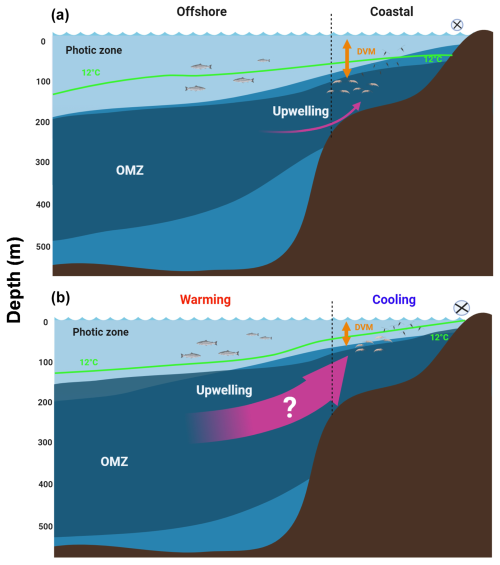
Figure 1Projected effects of the expansion of the OMZ in eastern boundary upwelling systems (EBUSs). (a) Under present (initial) conditions, wind-driven upwelling raises the OMZ system and brings cold water into shallow depths at the inshore, as illustrated by the 12 °C isotherm, and so fertilises the photic zone and promotes plankton aggregation. (b) Ocean warming effects manifest mainly at surface in the offshore region, while a vertically expanded OMZ along with an eventual (although uncertain) increase in upwelling may cool down the coastal zone and further shoaling the OMZ at the inshore area, spreading hypoxia and vertically reducing the oxygenated habitat. Vertical compression of the oxygenated habitat may restrict the vertical distribution and diel vertical migration (DVM) of zooplankton.
Oxygen plays a key role in the structuring and functioning of marine ecosystems and so modulates the spatial–temporal distribution of many marine organisms. This is mainly because low oxygen levels challenge the maintenance of the aerobic metabolism and therefore pose a challenge to the survival of most of the biota (Vaquer-Sunyer and Duarte, 2008; Ekau et al., 2010; Wishner et al., 2018; Breitburg et al., 2018). The effects of depleted oxygen can affect organisms in many ways, including acute natatory and physiological impairment, diminished growth and reproductive success; altered behaviour of mobile forms when searching for more favourable oxygen regimes (Wishner et al., 2018); reduced metabolic rates, including respiration and ammonium excretion (Kiko et al., 2016); and lower recruitment due to a reduction in egg hatching (Ruz et al., 2018; Choi et al., 2024).
The adaptation of animals to low oxygen has driven a strong selective pressure for maintaining the aerobic metabolism by optimising and enhancing oxygen uptake from hypoxic waters (Childress and Seibel, 1998), or alternatively by suppressing their metabolic rate to reduce the oxygen demands (Seibel, 2011). At the upper end of the oxygen cascade, oxygen uptake is satisfied by two adaptive modes: as an oxygen-conformer organism by reducing the aerobic metabolic rate as environmental oxygen decreases or as an oxygen-regulator organism by maintaining the aerobic metabolism down to an oxygen level known as critical oxygen tension (Pcrit) (Rogers et al., 2016). The difference between these two adaptive modes can be illustrated by the changes in the metabolic rate as a function of oxygen pressure (Fig. 2).
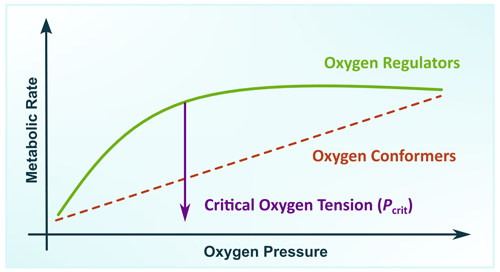
Figure 2Metabolic rate as a function of oxygen pressure illustrating two adaptive modes in marine zooplankton where an oxygen-conformer organism reduces the aerobic metabolic rate as environmental oxygen decreases while an oxygen regulator maintains aerobic metabolism down to an oxygen pressure known as critical oxygen tension (Pcrit) (modified from Pörtner and Grieshaber, 1993).
The adaptive responses to variable levels of oxygen illustrated in Fig. 2 may take place over short timescales (hours) as driven by the exposure to vertical gradients of oxygen in the water column when performing diel vertical migration (DVM) in migrating species or, in the case of non-migratory species, due to vertical mixing in the water column forced by upwelling pulses or by changes in wind conditions promoting mixing, both processes occurring over a synoptic (2–5 d) timescale in the upwelling zone (Sobarzo et al., 2007). Both migratory and non-migratory species can thus be exposed to variable oxygenation in the upwelling zone, which is characterised by a marked oxygen-stratified water column. This condition is well illustrated by the oxygen distribution in the water column of central–southern Chile throughout an annual cycle (Fig. 3). Within the photic zone (about 50 m in the coastal zone), the annual cycle of oxygen conditions reveals the existence of normoxic, mild, and severely hypoxic habitats, which vertically migratory and non-migratory zooplankton must face depending on their vertical distribution (Fig. 3). In addition, shallower strata are more variable on dissolved oxygen during intense upwelling (above 30 m deep), while the deeper strata (30–50 m deep) are more variable in oxygen during a depressed upwelling in the winter.
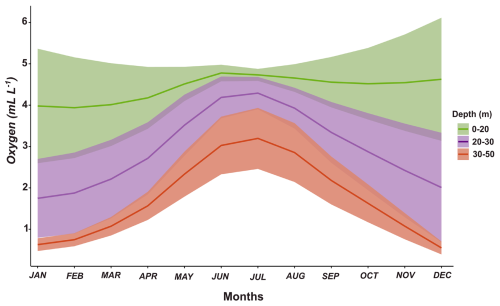
Figure 3The annual variability (monthly climatology) of dissolved oxygen in three strata in the upper 50 m layer off central–southern Chile (36°30′ S). Oxygen data are from the time series study at Station 18 off central–southern Chile during the period of 2002–2016 (Frederick et al., 2024). Shallower strata exhibit greater variability during intense upwelling in summer, while deeper strata show more variability during depressed upwelling in winter. The shaded area corresponds to the overlap of the distribution range of the organisms.
Furthermore, the response to oxygen variation illustrated in Fig. 2 may promote different metabolic adaptations, for instance, by reducing Pcrit to cope with severe hypoxia or a lack of adjustment of the capacity. This adaptive response has been shown to vary among copepod species (Frederick et al., 2024) and can therefore favour some species above others that are negatively impacted. Such differential species-specific responses are shown in Fig. 4, which describes the metabolic rate as a function of oxygen condition from normoxia to hypoxia. A non-adaptive response (Fig. 4a) is reflected in a direct relationship between the metabolic rate (MR) and oxygen levels, which also implies a change in Pcrit (fixed α). In contrast, adaptive response 1 (Fig. 4b) represents a constant MR at the cost of a changing Pcrit (variable α), while adaptive response 2 (Fig. 4c) maintains a constant Pcrit with a consequent change in MR (also variable α) as a function of oxygen levels. Among these, only adaptive response 1 (Fig. 4b) does not compromise regarding the aerobic scope, allowing for better regulation of physiological processes throughout the year (Fig. 3) but at the expense of not taking advantage of favourable periods such as phytoplankton blooms. In contrast, organisms with adaptive response 2 can better capitalise on favourable conditions in a changing ocean (likely spring and summer blooms) at the expense of reducing their aerobic scope.
It has recently been suggested that Pcrit is the point at which the oxygen supply capacity (α) reaches its maximum physiological limit (uptake and delivery) and as such is a species- and temperature-specific value (Seibel et al., 2021). For instance, Pcrit is constant within a given fish species (Rogers et al., 2016) and thus supports the validity of the maximum oxygen supply capacity within species. Yet, the potential plasticity of Pcrit has rarely been explored on invertebrates inhabiting fluctuating oxygen regimes, such as copepods living around OMZs (Wishner et al., 2018; Frederick et al., 2024). Furthermore, many of these pelagic invertebrates, which can produce several cohorts throughout the year, combine maternal effects with plasticity, therefore promoting shifts in either Pcrit or MR in some species of planktonic copepods depending on the season during the year cycle (Frederick et al., 2024). Such differential responses will ultimately alter the species composition with consequences for the food web structure. However, even for those oxygen-regulator species, severe hypoxia may be lower than Pcrit, resulting in severe stress or deleterious effects on organisms.
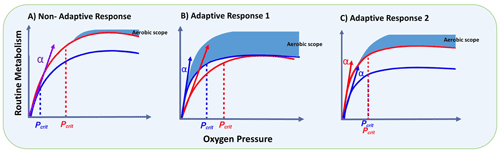
Figure 4The expected metabolic rate (MR) and critical partial pressure (Pcrit) as a function of environmental oxygen. The MR is theorised for two seasonal conditions: autumn–winter (blue line) and spring–summer (red line) in a coastal upwelling zone, where Pcrit will move following changes in MR and the slope (α) of the oxyconforming segment remains constant (a); adaptive response 1 in which Pcrit increases upon a changing oxyconforming slope (α) to maintain a constant MR (b); and adaptive response 2, where Pcrit is maintained constant by increasing α during the upwelling season (c) (modified from Frederick et al., 2024). Here, the oxyconforming segment refers to the metabolic rate under oxygen levels below Pcrit, while the aerobic scope represents the range of MR between the basal and maximum metabolism for a given species.
Other adaptive responses include a shift in the anaerobic metabolism when entering extremely low oxygen concentrations, such as those found in the core of the OMZ. This adaptation has been reported in actively migrating species, such as the krill Euphausia (Riquelme-Bugueño et al., 2020). Several adaptive responses have already been described for zooplankton inhabiting or entering the OMZ. For instance, crustaceans inside the OMZ can experience long-term physiological adjustments involving enhanced ventilatory capability, enlarged gill surfaces, shortened diffusion distances, and increasing respiratory proteins with high oxygen affinity (Childress and Seibel, 1998). For example, in vertically migrating zooplankton entering the OMZ, Antezana (2002) observed that Euphausia mucronata had enlarged gill surfaces while actively respiring and swimming under depleted oxygen, indicating oxygen usage in the OMZ. In another study, Spicer et al. (1999) investigated the anaerobic metabolism in the krill species Meganyctiphanes norvegica and found a significant increase in lactate concentrations as oxygen levels decreased to hypoxic conditions, allowing the species to withstand these conditions for extended periods. Euphausia mucronata has also been reported to have an elevated lactate dehydrogenase activity, enabling it to further tolerate prolonged exposure to hypoxic conditions (Gonzales and Quiñones, 2002).
Finally, changes in behaviour and distribution may reflect specific adaptive responses to hypoxia, as seen in many dominant zooplankton species avoiding the OMZ by restricting their vertical migration (Escribano et al., 2009; Tutasi and Escribano, 2020; Kiko and Hauss, 2019). In the copepod Acartia tonsa, exposed to seasonal hypoxia driven by estuarine conditions (rather than the presence of an OMZ), avoidance of hypoxic bottom waters has been shown (Decker et al., 2003).
At the ecosystem level, the different tolerances to low oxygen across species will determine their survival and so lead to changes in community structure and trophic webs. This is due to not only mortality, but also changes in predator–prey interactions because of changes in abundance, migration, and habitat compression (Tutasi and Escribano, 2020). While several species will be negatively affected (including commercially exploited species), others that are more hypoxia-tolerant may expand their range of distribution, exploit new niches (Stramma et al., 2010), and therefore have access to new resources.
Oxidative stress is a significant biological response to fluctuating oxygen levels, but it is rarely considered in marine environments. Driven by oxygen fluctuations, oxidative stress appears to be related to a state of respiratory imbalance in terms of O2 uptake, supply, and use, during which animals are unable to maintain constant tissue oxygenation/demand, and instead they experience rapid shifts between under-oxygenation and hyperoxygenation (Tremblay et al., 2010). This response is particularly evident in organisms exposed to oxygen levels below their Pcrit values (as illustrated in Fig. 4) and subsequently subjected to reoxygenation. Such conditions frequently occur in eastern boundary upwelling systems (EBUSs), where oxygen levels can fluctuate dramatically from normoxia to hypoxia over short spatial and temporal scales (hours). Under such conditions, reactive oxygen species (ROSs), produced as by-products of aerobic respiration, play a critical role by signalling molecules. The production of ROSs along with the release of the cytochrome complex, AMP-activated protein kinase (AMPK), mitochondrial DNA (mtDNA), and metabolites from the tricarboxylic acid (TCA) cycle can all originate in the mitochondria (Martínez-Reyes and Chandel, 2022). Since all signalling molecules are highly dependent on the oxygen availability for mitochondrial functioning, their regulation is likely impaired by the exposure to variable oxygen levels near the OMZ.
A higher production of ROSs in the organisms may alter the DNA structure, result in the modification of proteins and lipids, and trigger the activation of several stress-induced transcription factors (Birben et al., 2012). Available evidence suggests that oxidative stress can generate a significant physiological cost in life expectancy, reproduction, and immune response in addition to the effect on metabolism and growth (Zheng et al., 2021).
Molecular oxygen (O2) is the main biological electron acceptor, crucial in regulating cell functions. However, it is also the precursor of reactive oxygen species (ROS) formation because of normal cellular metabolism. The three major ROSs of physiological significance are superoxide anion (O2−), hydroxyl radical (−OH), and hydrogen peroxide (H2O2) (Guérin et al., 2001). The generation of these reactive oxygen species (ROSs) has been extensively studied (Welker et al., 2013; Moreira et al., 2016; Giraud-Billoud et al., 2019). When animals are re-oxygenated after hypoxic exposure, ROS formation occurs, and if not neutralised by the body's antioxidant defences, it may cause oxidative damage and eventually cellular disorder and death. ROS production is species-dependent, which can further vary as a function of the intensity of hypoxia and exposure time to an oxygen-deficient habitat. However, ROSs are not only produced by re-oxygenation after hypoxic exposure; in fact, ROS production has also been reported to occur in hypoxic conditions for a variety of organisms, as reviewed by Hermes-Lima (2015). The exposure to hypoxia may lead to ROS production and eventually to the production of antioxidant compounds as an adaptive response, a phenomenon described as preparation for oxidative stress (POS) (Hermes-Lima et al., 1998, 2015; Moreira et al., 2016). Tremblay et al. (2010) showed that krill species adapted to hypoxia have a sufficiently high antioxidant protection, whereas less adapted species suffer a strong oxidative stress measurable as lipid peroxidation. Thus, antioxidants play an important role in neutralising the oxidative action of free radicals.
The mechanisms of cellular protection against ROS production include several antioxidant enzymes, such as superoxide dismutase (SOD), catalase (CAT), peroxidase, glutathione S-transferase (GST), and glutathione peroxidase (GPx), and non-enzymatic substances, such as ascorbic acid (Vitamin C), glutathione (GSH), tocopherol, and carotenoids, where their role is critical in ROS detoxification processes in organisms subjected to oxidative stress. An imbalance between the level of ROS production and antioxidant protection can result in oxidative damage to tissues and a state of oxidative stress (Birben et al., 2012).
Linked to the presence of these radicals, a defence mechanism is the synthesis of antioxidant molecules capable of neutralising the oxidative action of these free radicals. Several studies have described the presence of ROS production and corresponding antioxidant responses in zooplankton, and this information is summarised in Table 1.
Table 1Zooplankton species exposed to different stressors leading to ROS production. DVM (daily vertical migration) can be Y (yes migration) or N (non-migration) and NI (no information). Arrows in column 5 indicate the increase or decrease in the biomarker signal in relation to the stressor agent.
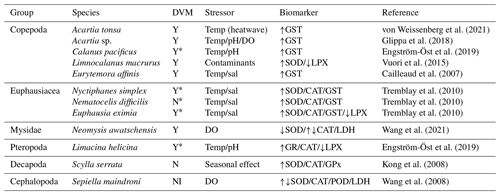
* The asterisk indicates that the samples were obtained from areas having an oxygen minimum zone. SOD: superoxide dismutase, CAT: catalase, GST: glutathione S-transferase, LPX: lipid peroxidation, GPx: glutathione peroxidase, LDH: lactate dehydrogenase, POD: peroxidase, GR: glutathione reductase, temp: temperature, sal: salinity.
POS has been proposed as a mechanism to strengthen antioxidant defences (Hermes-Lima et al., 1998, 2015; Moreira et al., 2016). In the context of ROSs associated with hypoxia, it is widely assumed that the antioxidant responses in a large variety of animals from marine, estuarine, and freshwater systems (listed in Hermes-Lima et al., 2015) can be preceded by changes at the molecular and biochemical levels after exposure to variable oxygen levels from anoxia to hyperoxia, and such a combined response is therefore known as the POS phenomenon (Welker et al., 2013).
Considering the metabolic responses outlined in Fig. 4, adaptive response 1 (Fig. 4b) is likely less stressful than the adaptive 2 and the non-adaptive response from the oxidative stress perspective as lacking large changes to the aerobic metabolism (or its demands) should keep ROS production better regulated. Adaptive response 2 and the non-adaptive response (Fig. 4a and c), instead, both demand better regulation of antioxidant defences or POS since changes in MR (changes in O2 demand) and thus O2 balance will likely generate ROSs at transitions between stable O2 supply–demand states. Critical oxygen tensions (Pcrits) measured in copepod species inhabiting the same area depicted in Fig. 3, in terms of oxygen variability, range from 2.77 ± 0.89 (P. cf. indicus) to 4.9 ± 0.59 kPa (A. tonsa), with C. patagoniensis in between with a Pcrit value of 3.83 ± 1.89 kPa (Frederick et al., 2024). Considering these values and the oxygen variability found in their environment (Fig. 3), only P. cf. indicus could maintain an aerobic metabolism stable if it stays in the shallower 20 m strata, while the other two species will inevitably transit from the aerobic to the anaerobic metabolism in some seasons during the year. These metabolic transitions likely generate ROSs and may also impact organisms performing diel vertical migration (DVM) as they traverse water layers with varying dissolved oxygen levels, as illustrated in Fig. 3. Altogether, how this oxidative balance and POS operate in zooplankton-inhabited areas subjected to a shallow OMZ is an open question. The time required for developing antioxidant defences also becomes an important issue in both migratory and non-migratory zooplankton because of the short time (a few hours) within which animals are exposed to hypoxia–normoxia conditions during migration or due to the irregular pulses of upwelling causing the ascent or descent of the OMZ. In the same context, and as mentioned above, most zooplankton avoid hypoxic waters by restricting their vertical distribution to the narrow and shallow normoxic layer in coastal waters or limiting the DVM by avoiding entering the OMZ or at least the extremely low oxygen layer found at the OMZ core (Kiko and Haus, 2019; Tutasi and Escribano, 2020). The highly variable DVM behaviour may thus play a key role in the adaptive response to hypoxia in the context of ROS production and POS processes.
Zooplankton performing extensive DVM can indeed enter the core of the OMZ, as described in several euphausiid species (Escribano et al., 2009; Riquelme-Bugueño et al., 2020). The incursions in extremely low oxygen waters and rapid re-oxygenation when ascending to near-surface at nighttime may trigger ROS production and certainly an antioxidant response. A possible adaptive response to this periodic exposure to low or high levels of oxygen is possible via POS by increasing ROSs during the hypoxic phase of the DVM, as seen in Fig. 5. In euphausiids that perform prolonged DVM, they are exposed to normoxic oxygen levels of >200 µM in the photic zone, followed by hypoxia (O2 < 3 µM). Their migration to deep zones (up to 200 m at dawn) takes less than 3 h (Riquelme-Bugueño et al., 2020), which means they are exposed to a reduction/increase in oxygenation of >30 % per hour while swimming up or down. Such stressful oxygen conditions over a short timescale can indeed trigger ROS production (Tremblay et al., 2010) and potentially POS during the nighttime phase (Fig. 5).
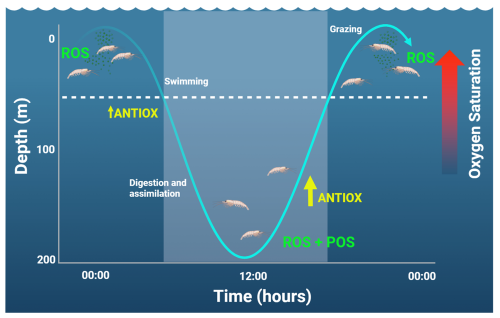
Figure 5Changes in the daily vertical migration and short-term response to oxidative stress in zooplankton. The lighter area shows the daytime. The vertical amplitude of migration represents that of euphausiids (Riquelme-Bugueño et al., 2020). At nighttime, an increase in the production of reactive oxidant species (ROSs) is expected as production of ROSs and the preparation for an antioxidant response (POS) can take place during the daytime, while animals stay below the photic zone (here represented by the dashed line at about 50 m).
The DVM behaviour, considered an adaptive response evolved to avoid visual predators during daylight conditions (Giraud-Billoud et al., 2019), likely led to the adaptation of POS and hence allows a mechanism to mitigate the effects of ROSs. Diel cycles triggering antioxidant responses to ROS production have been shown to occur in planktonic organisms exposed to light–dark conditions, such as Daphnia pulex (Cai et al., 2020), suggesting the existence of circadian rhythms of ROS production and subsequent POS. Several energy-intensive processes occur during DVM, such as grazing under oxygenated conditions followed by a descent (increased activity) to digest ingested food (specific dynamic action) under hypoxic conditions. The contribution of all these to ROS production is still unknown, and their production will likely mirror the gradients between available oxygen in the environment and the metabolic demands.
However, not all zooplankton perform DVM. Copepods, for instance, significantly contribute to the bulk of zooplankton biomass in the global ocean and remain within the upper 50 m of the water column (Escribano et al., 2009). These species, however, may still be exposed to hypoxia when upwelling intensity increases, allowing for the incursion of the upper limit of the OMZ (<3 kPa O2) into near-surface waters (Schneider et al., 2016). In the case of strong upwelling or extreme events driven by the intrusion of mesoscale eddies into the coastal zone, they may also cause episodes of severe hypoxia in shallow waters and thus affect non-migratory copepods.
Over the annual cycle in EBUSs, depending on latitude, the period of active upwelling has a strong seasonal variation in temperate areas or a weak and semi-permanent seasonal signal throughout the year in subtropical regions. For example, along the Chilean coast at mid-latitudes (30–40° S), strong spring–summer southern winds drive an intense upwelling period, resulting in a shallow OMZ. In contrast, autumn–winter is characterised by reduced upwelling, a condition commonly referred to as the downwelling period. This seasonal variation can temporarily expose non-migrating zooplankton populations to hypoxia during high-frequency changes (hours to days) in the upwelling season and potentially triggering ROS production during the transitional period before of upwelling. The combined effects of seasonal upwelling conditions, vertical distribution of the OMZ, and lack of DVM behaviour within the upper 50 m layer promoting ROS production and POS are illustrated in Fig. 6 for present and projected conditions. This figure illustrates a condition of downwelling (autumn–winter) with the upper limit of the OMZ below the photic zone, where non-migratory zooplankton is dispersed and normoxic conditions prevail. This oxic condition promotes the existence of ROS production, whereas strong upwelling and the shoaling of the OMZ into the photic zone can expose non-migratory zooplankton to changing hypoxia–normoxia conditions, which result in POS + ROSs. The expansion of the OMZ may exacerbate this condition in the future of EBUSs by further compressing the vertical extent of the oxygenated habitat (Fig. 6).
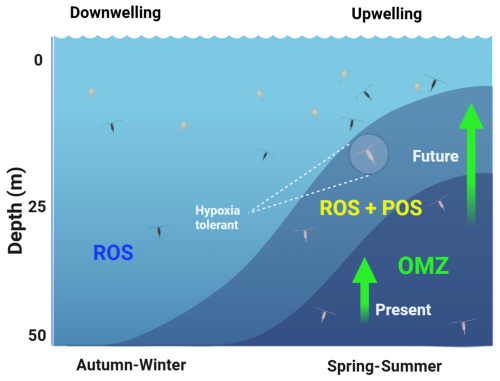
Figure 6The seasonal cycle of oxygen conditions of the photic zone (50 m) in EBUSs at temperate regions. Downwelling conditions prevail in the autumn–winter without the presence of the oxygen minimum zone (OMZ) within the photic zone and therefore promoting oxidative stress (ROS). Contrasting conditions of spring–summer (active upwelling), characterised by the presence of the OMZ, promote both ROSs and the adaptive response (POS). Future conditions may exacerbate the expansion of the OMZ also compressing the oxygenated habitat for zooplankton.
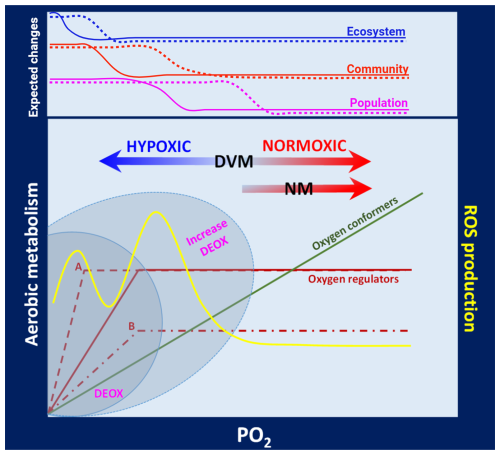
Figure 7Conceptual model of the aerobic metabolism in zooplankton. This scheme illustrates the different adaptive modes of zooplankton when responding to variable oxygenation (hypoxic to normoxic conditions) in the water column of EBUSs. Upon variable oxygen pressure (PO2), the aerobic metabolism of oxygen conformers and oxygen regulators is shown. Oxygen regulators may exhibit two types of responses: A, changing Pcrit but maintaining a constant metabolic rate (MR), or B, maintaining a constant Pcrit but modifying MR. Reducing Pcrit and maintaining a constant MR (adaptive mode A) may confer a greater capacity to withstand severe hypoxia satisfying the metabolic demands, while adaptive mode B implies a better exploitation of favourable conditions at the expense of decreasing the aerobic scope while maintaining a constant Pcrit. These metabolic responses can be further exacerbated under increasing deoxygenation in the upwelling zone (grey areas). Individuals performing DVM between hypoxic–normoxic conditions are more likely to be exposed to oxidative stress (yellow line) as they are subjected to strong fluctuations in PO2 in the proximity of Pcrit. At population, community, and ecosystem levels, ecological changes are expected to increase with hypoxic conditions but while exhibiting a lag among organisation levels. These changes can go from altered abundances and biomass in populations to altering community structure and modifying the whole food web in the ecosystem. Changes should become stronger under increased deoxygenation (dotted lines).
It is challenging to carry out these studies in organisms inhabiting the water column, such as zooplankton, given the high variability in the physical–chemical parameters to which they are exposed. For example, planktonic copepods are very abundant, and they make up about 80 % of the zooplankton biomass in EBUSs, so it is imperative to further explore the effects of changes in temperature, pH and dissolved oxygen (DO). An increase in antioxidant enzymatic activities indicates the activation of an antioxidant defence mechanism to deal with environment changes. This effect was reported by von Weissenberg et al. (2021) in Acartia. exposed to heatwaves in experiments carried out at temperatures ranging between 9 and 16 °C. The authors observed a positive relationship between the increases in glutathione in response to increased environmental temperature and showed the deleterious effect over reproductive success during warming.
Regarding the dominant zooplankton in EBUSs, a relevant issue to consider is the short life cycle of copepods (<2 months) and some numerically dominant euphausiids (<1 year). Under a seasonal upwelling regime, the exposure to hypoxia–normoxia may occur to different cohorts, and therefore the adaptive response (e.g. POS) might be seasonally adjusted. Although, if the OMZ continues its vertical expansion, there may not be sufficient time for developing adaptive responses with deleterious consequences for non-migratory populations, which comprise most upwelling inhabitant species.
3.1 Natural antioxidant agents for zooplankton
In coastal upwelling environments, zooplankton must face extremely variable conditions, which are likely stressful for most organisms. In this very same environment, however, the presence of an exogenous source of antioxidants provided, for example, by diatoms might help with mitigating the effect of oxidative stress due to such environmental fluctuations. Some studies show that planktonic diatoms have a high antioxidant potential (Goiris et al., 2012). Diatoms are rich in carotenoids, such as fucoxanthin and astaxanthin, which play a crucial role in protection against UV rays. Some diatoms, such as Skeletonema marinoi and Odontella aurita, can synthesise and accumulate ascorbic acid (Vitamin C) and phenolic compounds, which also have antioxidant properties (Smerilli et al., 2019; Hemalatha et al., 2015).
The concentrations of antioxidants in diatoms are species-specific. For instance, Foo et al. (2017) quantified the total content of some antioxidants and their bioactive capacity in six diatom species and found that Chaetoceros calcitrans (16.92 ± 0.87 mg TE g−1 DW, where TE is the Trolox equivalent and DW the dry weight) and Isochrysis galbana (21.55 ± 1.58 mg TE g−1 DW) showed the highest antioxidant activity, followed by Odontella sinensis and Skeletonema costatum, which exhibited moderate bioactivity (<13 mg TE g−1 DW), while Phaeodactylum tricornutum and Saccharina japonica displayed the lowest antioxidant activity (<5 mg TE g−1 DW) among the examined algae species. Most of these diatoms dominate the upwelling zone, specially Chaetoceros, Odontella, and Skeletonema, during the upwelling season (spring–summer) (Anabalón et al., 2007), and they are an important item of the zooplankton diet (Vargas et al., 2006).
Ocean deoxygenation and the loss of oxygen in EBUSs is an ongoing phenomenon, and planktonic organisms must inevitably cope with this gradually increased hypoxia (on average) and a changing dynamic of the ocean (change in occurrence and extreme events). The ecological consequences are far from understood and will largely depend on the ability of zooplankton to strengthen their capacity to tolerate mild or severe hypoxia, exploit plasticity and maternal effects to their maximum, or develop new adaptations. In any case, these responses may likely come at some cost and with trade-offs concerning other metabolic/energy-demanding processes. The outcome of the new demands implies a reduction in energy otherwise available for growth, feeding, and reproduction with further consequences in the population dynamics. The high dynamism of the water column in EBUSs, with factors such as light, oxygen, CO2, temperature, and food along with zooplankton processes like feeding, DVM, and digestion makes it challenging to identify the most challenging water strata, especially when considering temporal variation. Hypoxia conditions can also lead to changes in behaviour (upon stress) and spatial distribution and so alter, for example, prey–predator interactions, although such consequences are difficult to predict. The pelagic food web and community structure will likely be affected, with biogeochemical consequences in the context of the C and N recycling and ecosystem productivity. A conceptual model illustrating how variable oxygen levels in the water column can modulate the metabolic rates, drive their adaptive modes, and exert ecological consequences at population, community, and ecosystem levels in zooplankton inhabiting the upwelling zone of EBUSs is shown in Fig. 7. Further consequences driven by potential oxidative stress (ROS) are also included (Fig. 7).
Ocean deoxygenation will potentially increase in the coming decades, and the marine aerobic biota will gradually be exposed to more stressing conditions in terms of oxygen levels. In EBUSs, zooplankton is expected to perhaps better cope with increasing hypoxia, considering their high plasticity and the permanent presence of the OMZ to which different adaptations have already been evolved. However, the extent to which increasing hypoxia can impact specific populations in the upwelling zone requires attention since potential alterations at the base of the food web can have further consequences for the whole marine ecosystem. In this context, the assessment of plankton community structure through time series observations in the upwelling zone constitutes the most suitable proxy to examine the community responses to ongoing deoxygenation, and so long-term time series are extremely valuable for accurate predictions. Also, the use of molecular methods to examine how individuals can modify their gene expression to cope with hypoxia and eventually activate antioxidant responses are also necessary approaches when aiming to understand and predict the ecological consequences upon the expected severe conditions of an oxygen-deprived water column. New molecular methods, such as proteomic, which may recognise specific enzymatic systems actively participating in metabolic processes and biosynthesis of antioxidant compounds, can offer novel lines of research to understand adaptive and non-adaptive (phenotypic plasticity) responses to increasing hypoxia at the molecular level.
Data on dissolved oxygen during the time series at Station 18 are available at Zenodo respository https://doi.org/10.5281/zenodo.15128045 (Frederick, 2025).
LF, RE, and MAU conceived the work; LF wrote the first draft; and RE and MAU reviewed and edited the manuscript.
The contact author has declared that none of the authors has any competing interests.
Publisher’s note: Copernicus Publications remains neutral with regard to jurisdictional claims made in the text, published maps, institutional affiliations, or any other geographical representation in this paper. While Copernicus Publications makes every effort to include appropriate place names, the final responsibility lies with the authors.
We thank Erika Jorquera for their criticism and suggestions to the work and Pamela Hidalgo, who provided ideas and directions regarding ocean deoxygenation and its biological effects. Two anonymous reviewers and their rigorous revisions of early versions of the paper provided extremely valuable suggestions on how to improve the work. We are also grateful to Ciavatta Stefano for their comments and suggestions. This work is a contribution to the Millennium Nucleus on Ocean Deoxygenation (DEOXS).
This research has been supported by the Agencia Nacional para la Investigación y Desarrollo (ANID), Chile (grant nos. Fondecyt 1210071 and ICN12_019-IMO) to Mauricio A. Urbina and Ruben Escribano; Instituto Milenio de Oceanografía (IMO) (grant no. AIM23-0003) to Ruben Escribano and Mauricio A. Urbina; ANID PhD scholarship (grant no. 21181372) to Leissing Frederick; and grant no. ANILLO ACT210073.
This paper was edited by Ciavatta Stefano and reviewed by two anonymous referees.
Anabalón, V., Morales, C. E., Escribano, R., and Varas, M. A: The contribution of nano- and micro-planktonic assemblages in the surface layer (0–30) under different hydrographic conditions in the upwelling area off Concepción, central Chile, Prog. Oceanogr. 75, 396–414, 2007.
Antezana, T.: Adaptive behaviour of Euphausia mucronata in relation to the oxygen minimum layer of the Humboldt Current, Oceanography of the Eastern Pacific, II, 29–40, 2002.
Auger, P. A., Bento, J. P., Hormazabal, S., Morales, C. E., and Bustamante, A.: Mesoscale Variability in the Boundaries of the Oxygen Minimum Zone in the Eastern South Pacific: Influence of Intrathermocline Eddies, J. Geophys. Res.-Oceans, 126, e2019JC015272, https://doi.org/10.1029/2019JC015272, 2021.
Bakun, A., Field, D. B., Redondo-Rodriguez, A., and Weeks, S. J.: Greenhouse gas, upwelling-favourable winds, and the future of coastal ocean upwelling ecosystems, Glob. Change Biol., 16, 1213–1228, https://doi.org/10.1111/j.1365-2486.2009.02094.x, 2010.
Birben, E., Sahiner, U. M., Sackesen, C., Erzurum, S., and Kalayci, O.: Oxidative stress and antioxidant defence, World Allergy Organization Journal, 5, 9–19, https://doi.org/10.1097/WOX.0b013e3182439613, 2012.
Bode, A., Álvarez, M., Ruíz-Villarreal, M., and Varela, M. M.: Changes in phytoplankton production and upwelling intensity off A Coruña (NW Spain) for the last 28 years, Ocean Dynam., 69, 861–73, https://doi.org/10.1007/s10236-019-01278-y, 2019.
Bograd, S. J., Jacox, M. G., Hazen, E. L., Lovecchio, E., Montes, I., Pozo Buil, M., Shannon, L. J., Sydeman, W. J., and Rykaczewski, R. R.: Climate Change Impacts on Eastern Boundary Upwelling Systems, Annu. Rev. Mar. Sci., 16, 303–328, https://doi.org/10.1146/annurev-marine-032122-021945, 2023.
Breitburg, D., Levin, L. A., Oschlies, A., Gregoire, M., Chavez, F. P., Conley, D. J., Garcon, V., Gilbert, D., Gutierrez, D., and Isensee, K.: Declining oxygen in the global ocean and coastal waters, Science, 359, eaam7240, https://doi.org/10.1126/science.aam7240, 2018.
Cai, M., Liu, Z., Yu, P., Jiao, Y., Chen, Q., Jiang, Q., and Zhao, Y.: Circadian rhythm regulation of the oxidation–antioxidant balance in Daphnia pulex, Comp. Biochem. Phys. B, 240, 110387, https://doi.org/10.1016/j.cbpb.2019.110387, 2020.
Cailleaud, K., Maillet, G., Budzinski, H., Souissi, S., and Forget-Leray, J.: Effects of salinity and temperature on the expression of enzymatic biomarkers in Eurytemora affinis (Calanoida, Copepoda), Comp. Biochem. Phys. A, 147, 841–849, https://doi.org/10.1016/j.cbpa.2006.09.012, 2007.
Chan, F., Barth, J. A., Lubchenco, J., Kirincich, A., Weeks, H., Peterson, W. T., and Menge, B. A.: Emergence of anoxia in the California Current large marine ecosystem, Science, 319, 920–920, https://doi.org/10.1126/science.1149016, 2008.
Chavez, F. P. and Messié, M.: A comparison of eastern boundary upwelling ecosystems, Prog. Oceanogr., 83, 80–96, https://doi.org/10.1016/j.pocean.2009.07.032, 2009.
Childress, J. and Seibel, B.: Life at stable low oxygen levels: Adaptations of animals to oceanic oxygen minimum layers, J. Exp. Biol., 201, 1223–1232, https://doi.org/10.1242/jeb.201.8.1223, 1998.
Choi, S. Y., Belmonte, G., Hyun, B., Jang, P.-G., Lee, W.-J., Young, H., Min, S., Seo, H., Seo, J.-Y., Shin, K., and Jang, M. C.: Effects of hypoxia on copepod egg hatching success: An in situ study, J. Marine Syst., 245, 103979, https://doi.org/10.1016/j.jmarsys.2024.103979, 2024.
Decker, M. B., Breitburg, D. L., and Marcus, N. H.: Geographical differences in behavioural responses to hypoxia: local adaptation to anthropogenic stressor?, Ecol. Appl., 13, 1104–1109, https://www.jstor.org/stable/4134746 (last access: April 2025), 2003.
Ekau, W., Auel, H., Pörtner, H.-O., and Gilbert, D.: Impacts of hypoxia on the structure and processes in pelagic communities (zooplankton, macro-invertebrates and fish), Biogeosciences, 7, 1669–1699, https://doi.org/10.5194/bg-7-1669-2010, 2010.
Engström-Öst. J., Glippa, O., Feely, R. A., Kanerva, M., Keister, J. E., Alin, S. R.,Carter, B. R., McLaskey, A. K., Vuori, K. A., and Bednaršek, N.: Eco-physiological responses of copepods and pteropods to ocean warming and acidification, Scientific Report, 9, 4748, https://doi.org/10.1038/s41598-019-41213-1, 2019.
Escribano, R., Hidalgo, P., and Krautz, C.: Zooplankton associated with the oxygen minimum zone system in the northern upwelling region of Chile during March 2000, Deep-Sea Res. II, 56, 1083–1094, https://doi.org/10.1016/j.dsr2.2008.09.009, 2009.
Foo, S. C., Yusoff, F. M., Ismail, M., Basri, M., Yau, S. K., Khong, N. M. H., Chan, K. W., and Ebrahimi, M.: Antioxidant capacities of fucoxanthin-producing algae as influenced by their carotenoid and phenolic contents, J. Biotechnol., 241, 175–183, https://doi.org/10.1016/j.jbiotec.2016.11.026, 2017.
Frederick, L.: Time Series- Station 18-chile, Zenodo [data set], https://doi.org/10.5281/zenodo.15128045, 2025.
Frederick, L., Urbina-Foneron, M., and Escribano, R.: Adjusting metabolic rates and critical oxygen tension in planktonic copepods under increasing hypoxia in highly productive coastal upwelling zones, Limnol. Oceanogr., 69, 1115–1128, https://doi.org/10.1002/lno.12556, 2024.
Garcia-Reyes, M. and Largier, J. L.: Seasonality of coastal upwelling off central and northern California: New insights, including temporal and spatial variability, J. Geophys. Res., 117, C03028, https://doi.org/10.1029/2011JC007629, 2009.
Gilly, W. G., Beman, M., Litvin, S. Y., and Robison, B. H.: Oceanographic and biological effects of shoaling of the oxygen minimum zone, Annu. Rev. Mar. Sci., 5, 393–420, https://doi.org/10.1146/annurev-marine-120710-100849, 2013.
Giraud-Billoud, M., Rivera-Ingraham, G. A., Moreira, D. C., Burmesterd, T., Castro-Vazqueza, A., Carvajalino-Fernández, J. M., Dafre, A., Niu, C., Tremblay, N., Paital, B., Rosa, R., Storey, J. M., Vega, I. A., Zhang, W., Yepiz-Plascencial, G., Zenteno-Savinm, T., Storey, K. B., and Hermes-Lima, M.: Twenty years of the “Preparation for Oxidative Stress” (POS) theory: Ecophysiological advantages and molecular strategies, Comparative Biochemical and Physiology: Molecular and Integrative Physiology Part A, 234, 36–49, https://doi.org/10.1016/j.cbpa.2019.04.004, 2019.
Glippa, O., Engström-Öst, J., Kanerva, M., Rein, A., and Vuori, K.: Oxidative stress and antioxidant defense responses in Acartia copepods in relation to environmental factors, PLoS One, 13, e0195981, https://doi.org/10.1371/journal.pone.0195981, 2018.
Goiris, K., Muylaert, K., Fraeye, I., Foubert, I., De Brabanter, J., and De Cooman, L.: Antioxidant potential of microalgae in relation to their phenolic and carotenoid content, J. Appl. Phycol., 24, 1477–1486, https://doi.org/10.1007/s10811-012-9804-6, 2012.
Gonzalez, R. and Quiñones, R.: Ldh activity in Euphausia mucronata and Calanus chilensis: implications for vertical migration behavior, J. Plankton Res., 24, 1349–1356, https://doi.org/10.1093/plankt/24.12.1349, 2002.
Grégoire, M., Garçon, V., Garcia, H., Breitburg, D., Isensee, K., Oschlies, A., Telszewski, M., Barth, A., Bittig, H. C., Carstensen, J., Carval, T., Chai, F., Chavez, F., Conley, D., Coppola, L., Crowe, S., Currie, K., Dai, M., Deflandre, B., Dewitte, B., Diaz, R., Garcia-Robledo, E., Gilbert, D., Giorgetti, A., Glud, R., Gutierrez, D., Hosoda, S., Ishii, M., Jacinto, G., Langdon, Ch., Lauvset, S. K., Levin, L. A., Limburg, K. E., Mehrtens, H., Montes, I., Naqvi, W., Paulmier, A., Pfeil, B., Pitcher, G., Pouliquen, S., Rabalais, N., Rabouille, C., Recape,V., Roman, M., Rose, K., Rudnick, D., Rummer, J., Schmechtig, C., Schmidtko, S., Seibel, B., Slomp, C., Sumalia, U. R., Tanhua, T., Thierry, V., Uchida, H., Wanninkhof, R., and Yasuhara, M.: A Global Ocean Oxygen Database and Atlas for Assessing and Predicting Deoxygenation and Ocean Health in the Open and Coastal Ocean, Front. Mar. Sci., 8, 724913, https://doi.org/10.3389/fmars.2021.724913, 2021.
Guérin, P., El Mouatassim, S., and Ménézo, Y.: Oxidative stress and protection against reactive oxygen species in the pre-implantation embryo and its surroundings, Hum. Reprod. Update, 7, 175–189, https://doi.org/10.1093/humupd/7.2.175, 2001
Hemalatha, A., Parthiban, C., Saranya, C., Girija. K., and Anatharaman, P.: Evaluation of antioxidant activities and total phenolic contents of different solvent extracts of selected marine diatoms, Indian J. Geo-Mar. Sci., 44, 1630–1636, https://doi.org/10.1186/s40538-017-0110-z, 2015.
Hermes-Lima, M., Storey, J. M., and Storey, K. B.: Antioxidant defences and metabolic depression. The hypothesis of preparation for oxidative stress in land snails, Comp. Biochem. Physiol. B., 120, 437–448, https://doi.org/10.1016/S0305-0491(98)10053-6, 1998.
Hermes-Lima, M., Moreira, D. C., Rivera-Ingraham, G. A., Giraud-Billoud, M., Genaro-Mattos, T. C., and Campos, E. G.: Preparation for oxidative stress under hypoxia and metabolic depression: Revisiting the proposal two decades later, Free Radic. Biol. Med., 89, 1122–1143, https://doi.org/10.1016/j.freeradbiomed.2015.07.156, 2015.
Kiko, R., Hauss, H., Buchholz, F., and Melzner, F.: Ammonium excretion and oxygen respiration of tropical copepods and euphausiids exposed to oxygen minimum zone conditions, Biogeosciences, 13, 2241–2255, https://doi.org/10.5194/bg-13-2241-2016, 2016.
Kiko, R. and Hauss, H.: On the Estimation of Zooplankton-Mediated Active Fluxes in Oxygen Minimum Zone Regions, Front. Mar. Sci., 6, 741, https://doi.org/10.3389/fmars.2019.00741, 2019.
Kong, X., Wang, G., and Li, S.: Seasonal variation of ATPasa activity and antioxidant defences in gills of the mud crab Scylla serra (Crustacea, Decapoda), Mar. Biol., 154, 269–276, https://doi.org/10.1007/s00227-008-0920-4, 2008.
Köhn, E. E., Münnich, M., Vogt, M., Desmet, F., and Gruber, N.: Strong habitat compression by extreme shoaling events of hypoxic waters in the Eastern Pacific, J. Geophys. Res.-Oceans, 127, e2022JC018429, https://doi.org/10.1029/2022JC018429, 2022.
Martínez-Reyes, I. and Chandel, N. S.: Mitochondrial TCA cycle metabolites control physiology and disease, Nat. Commun., 11, 102, https://doi.org/10.1038/s41467-019-13668-3, 2022.
Moreira, D., Venancio, L., Sabino, M., and Hermes-Lima, M.: How widespread is preparation for oxidative stress in the animal kingdom, Comparative Biochemical and Physiology: Molecular Integrative and Physiology, 200, 64–78, https://doi.org/10.1016/j.cbpa.2016.01.023, 2016.
Oschlies, A., Brandt,,P., Stramma, L., and Schmidtko, S.: Drivers and mechanisms of ocean deoxygenation, Nat. Geosci., 11, 467–473, https://doi.org/10.1038/s41561-018-0152-2, 2018.
Pardo, P., Padín, X., Gilcoto, M., Farina-Busto, L., and Pérez, F.: Evolution of upwelling systems coupled to the long term variability in sea surface temperature and Ekman transport, Clim. Res., 48, 231–46, https://doi.org/10.3354/cr00989, 2011.
Pörtner, H. O. and Grieshaber, M. K.: Critical PO2(s) in oxyconforming and oxyregulating animals: gas exchange, metabolic rate and the mode of energy production, in: The vertebrate gas transport cascade adaptations to environment and mode of life, edited by: Bicudo J. E. P. W., CRC Press, Boca Raton, FL, https://doi.org/10013/epic.13032, 1993.
Riquelme-Bugueño, R., Pérez Santos, I., Alegría, N., Vargas, C. A., Urbina, M. A., and Escribano, R.: Diel vertical migration into anoxic and high pCO2 waters: acoustic and net based krill observations in the Humboldt Current Ramiro, Nature, Sci. Rep., 10, 17181, https://doi.org/10.1038/s41598-020-73702-z, 2020.
Rogers, N. J., Urbina, M. A., Reardon, E. E., McKenzie, D. J., and Wilson, R. W.: A new analysis of hypoxia tolerance in fishes using a database of critical oxygen level (Pcrit), Conserv. Physiol., 4, cow012, https://doi.org/10.1093/conphys/cow012, 2016.
Ruz, P. M., Hidalgo, P., Yañez, S., Escribano, R., and Keister, J. E.: Egg production and hatching success of Calanus chilensis and Acartia tonsa in the northern Chile upwelling zone (23° S), Humboldt Current System, J. Mar. Syst., 148, 200–212, https://doi.org/10.1016/j.jmarsys.2015.03.007, 2015.
Ruz, P. M., Hidalgo, P., Escribano, R., Keister, J. E., Yebra, L., and Franco-Cisterna, B.: Hypoxia effects on females and early stages of Calanus chilensis in the Humboldt current ecosystem (23° S), J. Exp. Mar. Biol. Ecol., 498, 61–71, https://doi.org/10.1016/j.jembe.2017.09.018, 2018.
Santos, F., Gomez-Gesteira, M., de Castro, M., and Alvarez, I.: Differences in coastal and oceanic SST trends due the strengthening of coastal upwelling along the Benguela Current System, Cont. Shelf Res. 34, 79–86, https://doi.org/10.1016/j.csr.2011.12.004, 2012.
Schmidtko, S., Stramma, L., and Visbeck, M.: Decline in global oceanic oxygen content during the past five decades, Nature, 542, 335–339, https://doi.org/10.1016/j.csr.2011.12.004, 2017.
Schneider, W., Donoso, D., Garcés-Vargas, J., and Escribano, R.: Water-column cooling and sea surface salinity increase in the upwelling region off central-south Chile driven by a poleward displacement of the South Pacific, Prog. Oceanogr., 141, 38–58, https://doi.org/10.1016/j.pocean.2016.11.004, 2016.
Seibel, B. A.: Critical oxygen levels and metabolic suppression in oceanic oxygen minimum zones, J. Exp. Biol., 214, 326–336, https://doi.org/10.1242/jeb.049171, 2011.
Seibel, B. A., Andres, A., Birk, M. A., Burns, A. L., Shaw, T. C., Timpe, A. W., and Welsh, C. J.: Oxygen supply capacity breathes new life into critical oxygen partial pressure (Pcrit), J. Ex. Biol., 224, jeb242210, https://doi.org/10.1242/jeb.242210, 2021.
Smerilli, A., Balzano, S., Maselli, M., Blasio, M., Orefice, I., Galasso, C., Sansone, C., and Brunet, C.: Antioxidant and photoprotection networking in the coastal diatom Skeletonema marinoi, Antioxidant, 8, 154, https://doi.org/10.3390/antiox8060154, 2019.
Spicer, J. I., Thomasson, M. A., and Strömberg, J.: Possessing a poor anaerobic capacity does not prevent the diel vertical migration of Nordic krill Meganyctiphanes norvegica into hypoxic waters, Mar. Ecol. Prog. Ser., 185, 181–187, https://www.jstor.org/stable/24853208 (last access: April 2025), 1999.
Sobarzo, M., Kipp Shearman, R., and Lentz, S.: Near-inertial motions over the continental shelf off Concepción, central Chile, Prog. Oceanogr., 75, 348–362, https://doi.org/10.1016/j.pocean.2007.08.021, 2007.
Stramma, L., Johnson, G. C., Sprintall, J., and Mohrholz. V.: Expanding oxygen minimum zones in the tropical oceans, Science, 320, 655–658, https://doi.org/10.1126/science.1153847, 2008.
Stramma, L., Schmidtko, S., Levin, L., and Johnson, G.: Ocean oxygen minima expansions and their biological impacts, Deep-Sea Res., 57, 587–595, https://doi.org/10.1016/j.dsr.2010.01.005, 2010.
Tremblay, N., Gómez-Gutiérrez, J., Zenteno-Savín, T., Robinson, C. J., and Sánchez-Velascoa, L.: Role of oxidative stress in seasonal and daily vertical migration of three krill species in the Gulf of California, Limnol. Oceanogr., 55, 2570–2584, https://doi.org/10.4319/lo.2010.55.6.2570, 2010.
Tutasi, P. and Escribano, R.: Zooplankton diel vertical migration and downward C flux into the oxygen minimum zone in the highly productive upwelling region off northern Chile, Biogeosciences, 17, 455–473, https://doi.org/10.5194/bg-17-455-2020, 2020.
Vaquer-Sunyer, R. and Duarte, C. M.: Thresholds of hypoxia for marine biodiversity, P. Natl. Acad. Sci. USA, 105, 15452–15457, https://doi.org/10.1073/pnas.0803833105, 2008.
Vargas, C., Escribano, R., and Poulet, S.: Phytoplankton diversity determines time-windows for successful zooplankton reproductive pulses, Ecology 87, 2992–2999, 2006.
Vuori, K. A., Lehtonen, K. K., Kanerva, M., Peltonen, H., Nikinmaa, M., Berezina, N. A., and Boikova, E.: Oxidative stress biomarkers in the copepod Limnocalanus macrurus from the northern Baltic Sea: effects of hydrographic factors and chemical contamination, Mar. Ecol. Progr. Ser., 538, 131–144, https://doi.org/10.3354/meps11471, 2015.
Wang, C. L., Wu, D. H., Dong, T. Y., and Jiang, X. M.: Oxygen consumption rate and effects of hypoxia stress on enzyme activities of Sepiella maindron, Chin. J. Appl. Ecol., 19, 2420–2427, 2008.
Wang, D., Gouhier, T. C., Menge, B. A., and Ganguly, A. R.: Intensification and spatial homogenization of coastal upwelling under climate change, Nature, 518, 390–394, https//doi.org/10.1038/nature14235, 2015.
Wang, Q., Li, X., Yan, T., Song, J., Yu, R., and Zhou, M.: Detrimental impact of hypoxia on the mortality, growth, reproduction, and enzyme activities of planktonic mysid Neomysis awatschensis, Aquat. Ecol., 55, 849–859, https://doi.org/10.1007/s10452-021-09864-3, 2021.
von Weissenberg. E., Jansson, A., Vuori, K. A., and Engström-Öst, J.: Copepod reproductive effort and oxidative status as responses to warming in the marine environment, Ecol. Evol., 12, e8594, https://doi.org/10.1002/ece3.8594, 2021.
Welker, A. F., Moreira, D. C., Campos, E. G., and Hermes-Lima, M.: Role of redox metabolism for adaptation of aquatic animals to drastic changes in oxygen availability, Comparative Biochemistry and Physiology: Molecular and Integrative Physiology 165, 384–404, https://doi.org/10.1016/j.cbpa.2013.04.003, 2013.
Wishner, K. F., Seibel, B. A., Roman, C., Deutsch, C., Outram, D., Shaw, C. T., Birk, M. A., Mislan, K. S., Adams, T. J., Moore, D., and Riley, S.: Ocean deoxygenation and zooplankton: Very small oxygen differences matter, Sci. Adv., 4, eaau5180, https://doi.org/10.1126/sciadv.aau5180, 2018.
Wishner, K. F., Seibel, B., and Outram, D.: Ocean deoxygenation and copepods: coping with oxygen minimum zone variability, Biogeosciences, 17, 2315–2339, https://doi.org/10.5194/bg-17-2315-2020, 2020.
Xiu, P., Chai, F., Curchitser, E. N., Frederic, S., and Castruccio, S.: Future changes in coastal upwelling ecosystems with global warming: The case of the California Current System, Sci. Rep., 8, 2866, https://doi.org/10.1038/s41598-018-21247-7, 2018.
Zheng, C., Zhao, Q., Li, E., Zhao, D., and Sun, S.: Role of hypoxia in the behaviour, physiology, immunity and response mechanisms of crustaceans: A review, Rev. Aquac., 14, 676–687, https://doi.org/10.1111/raq.12618, 2021.