the Creative Commons Attribution 4.0 License.
the Creative Commons Attribution 4.0 License.
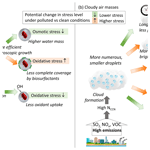
Ideas and perspectives: Microorganisms in the air through the lenses of atmospheric chemistry and microphysics
Pierre Amato
Kifle Aregahegn
Muriel Joly
Amina Khaled
Tiphaine Labed-Veydert
Frédéric Mathonat
Leslie Nuñez López
Raphaëlle Péguilhan
Minghui Zhang
Microorganisms in the atmosphere comprise a small fraction of the Earth's microbiome. A significant portion of this aeromicrobiome consists of bacteria that typically remain airborne for a few days before being deposited. Unlike bacteria in other spheres (e.g., litho-, hydro-, phyllo-, cryospheres), atmospheric bacteria are aerosolized, residing in individual particles and separated from each other. In the atmosphere, bacteria encounter chemical and physical conditions that affect their stress levels and survival. This article goes beyond previous overviews by placing these conditions in the context of fundamental chemical and microphysical concepts related to atmospheric aerosols. We provide ranges of water amounts surrounding bacterial cells both inside and outside clouds and suggest that the small volumes of individual cloud droplets lead to nutrient and oxidant limitations. This may result in greater nutrient limitation but lower oxidative stress in clouds than previously thought. Various chemical and microphysical factors may enhance or reduce microbial stress (e.g., oxidative, osmotic, UV-induced), affecting the functioning and survival of atmospheric bacteria. We illustrate that these factors could impact stress levels under polluted conditions, indicating that conclusions about the role of pollutants in directly causing changes to microbial abundance can be erroneous. The perspectives presented here aim to motivate future experimental and modeling studies to disentangle the complex interplay of chemical and microphysical factors with the atmospheric microbiome. Such studies will help to comprehensively characterize the role of the atmosphere in modifying the Earth' microbiome, which regulates the stability of global ecosystems and biodiversity.
- Article
(5537 KB) - Full-text XML
-
Supplement
(7311 KB) - BibTeX
- EndNote
Microorganisms are the most abundant organisms on Earth and comprise about 15 % of the total biological mass (Bar-On et al., 2018). This “unseen majority” of life (Whitman et al., 1998) is an essential actor in ecosystem functioning, biogeochemical nutrient cycling, soil formation, and decomposition processes and is crucial for preserving the health of the planet (Cardinale et al., 2012). Diversity within microbial communities usually leads to higher resilience towards environmental disturbances, such as pollution and climate change, and may be beneficial in terms of more robust and healthy systems (Robinson and Breed, 2023).
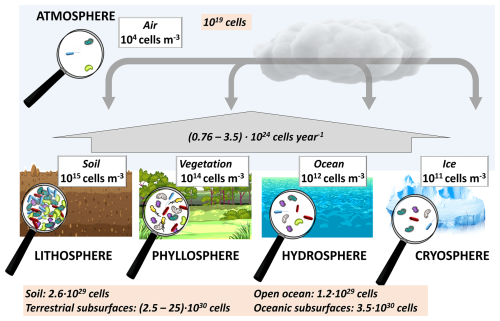
Figure 1Schematic of bacteria cell concentrations in the spheres of the Earth. References: soil (prokaryotes) (Whitman et al., 1998), vegetation (Lindow and Brandl, 2003), assuming a ratio of leaf volume to leaf area of ∼ 500 cm3 m−2 (Poorter et al., 2009), surface waters (prokaryotes) (Whitman et al., 1998), sea ice (Boetius et al., 2015), and the atmosphere (Burrows et al., 2009b); the values in the orange box at the bottom are from Whitman et al. (1998), emissions to the atmosphere are from Burrows et al. (2009a), and the atmospheric cell number is from Šantl-Temkiv et al. (2022).
A significant portion of Earth's microbiome consists of bacteria; Fig. 1 provides an overview of the bacteria concentrations and abundances in major aquatic and terrestrial “spheres” of the Earth. The highest (average) bacteria cell concentrations are found in soil (1015 cells m−3). The concentrations that are lower by several orders of magnitude in vegetation (phyllosphere), water (hydrosphere), and ice (cryosphere). The surfaces of these spheres are connected by the atmosphere that harbors significantly smaller cell concentrations, both related to air volume (∼ 104 cells m−3) and in absolute numbers (∼ 1019 cells), which comprise an apparently negligible fraction ( %) of the total Earth microbiome (>1030 cells).
In comparison to the microbially more densely populated spheres, the atmospheric microbiome (“aeromicrobiome”) is relatively poorly characterized. In the past, the main focus on studies of airborne microorganisms addressed their role as pathogens (Pasteur, 1861). The rapid air movements and emission–deposition cycles in the highly dynamic atmosphere lead to efficient transport and displacement of microorganisms. While the atmospheric microbial diversity close to the ground resembles that of the underlying surface (“footprint”), air masses mix at higher altitudes, which results in complex mixtures of microbial populations. Microbes follow major air movements along “microbial highways”. However, the exact patterns of such aerobiological trajectories cannot be resolved to date since samples are relatively sparse (Smith et al., 2018). A fundamental difference in the atmosphere – as opposed to microbial environments in other Earth parts – is the fact that microorganisms are aerosolized; i.e., they are surrounded by a finite hydration shell, limiting their access to nutrients and water. Aerosol particles are continuously exposed to light, trace gases, oxidants, and other chemical pollutants, which leads to unique conditions that rapidly change and may expose the microorganisms to different levels of stress on a variety of spatial and temporal scales. These particular conditions, which differ greatly from those in the (more) homogeneous aquatic and terrestrial environments, are usually not taken into account in atmospheric microbiological studies.
The present article fills this gap by providing a new perspective on the temporal and spatial scales of atmospheric chemical and microphysical factors and processes that may affect the atmospheric microbiome. In Sect. 2, we discuss various microscale (physico-) chemical aerosol properties relevant for the atmospheric bacterial environments. All underlying fundamental equations and parameters are summarized in the Supplement. Each subsection is introduced by a question we neither attempt nor intend to comprehensively answer; instead, these questions are posed to motivate future studies to explore factors that potentially control microbial stress, survival, and diversity in the atmosphere. Section 3 places the preceding considerations into the context of atmospheric scenarios, specifying various environmental factors and their potential role in microbial stress under polluted and/or cloudy conditions. In the concluding Sect. 4, we give recommendations for future studies to advance our understanding of the atmosphere in shaping the microbiome. We point out the need of interdisciplinary efforts merging atmospheric (aerosol) sciences, microbial ecology, and aerobiology.
2.1 Aerosolized bacteria: does social distancing between cells matter for their functioning?
The formation of agglomerates and/or biofilms in aquatic and terrestrial habitats provides multiple advantages to bacteria in terms of protection and collective resources. Such social traits enable microbes to adapt to diverse environments, optimize resource utilization, and enhance their survival and reproductive success. Bacteria in the atmosphere are detached from such community structures as they can only be airborne upon aerosolization. Bacteria-containing particles contain a single – or, at most, a few – bacteria cell(s). The presence of more than a single cell in a particle leads to a larger particle. However, the resulting total particle surface area might not scale proportionally with the number of cells since the particle shape and total volume might be mostly determined by the hydration shell. In addition to gravitational settling, other particle properties and processes affect the atmospheric residence time, including horizontal transport and the ability to act as cloud condensation nuclei (CCN) or ice nuclei (IN) (Sect. S1.1). Burrows et al. (2009a) demonstrated that bacteria acting as cloud condensation nuclei are generally deposited faster and, thus, have a global atmospheric residence time that is approximately half as long as bacteria not activated into droplets. Therefore, the distances that CCN- and/or IN-active bacteria travel in the atmosphere are generally comparably short.
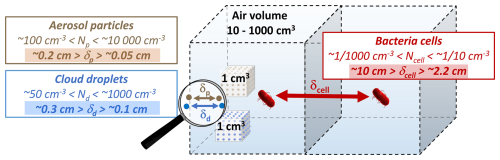
Figure 2Schematic illustration of the distance between bacteria cells vs. aerosol particles and cloud droplets (δcell, δp, δdr) for typical atmospheric concentrations (N [cm−3]); the distance δ [cm] corresponds to (for calculation and wider ranges, see Sect. S1.2 in the Supplement).
Atmospheric concentrations of bacteria cells are typically in the range of 0.001–0.1 cells cm (Burrows et al., 2009a; Després et al., 2012), with typical sizes being on the order of 100 nm–1 µm (Sattler et al., 2001; Pöschl and Shiraiwa, 2015). The total atmospheric number concentration of aerosol particles of such sizes (“fine particles”) ranges from 103 to 105 particles cm (Seinfeld and Pandis, 2006). The comparison of these numbers reveals that bacteria comprise ≪1 % of all atmospheric aerosol particles. A cloud droplet forms by means of water vapor condensation on an individual particle, i.e., on CCN that are typically in the size range of fine particles. Since single bacterial cells are often similar in size to these particles, it is likely that each particle hosts only one cell (Fankhauser et al., 2019). The fact that the bacteria number concentration is much smaller than the total CCN concentration in the atmosphere led Fankhauser et al. (2019) and Ervens and Amato (2020) to conclude that only 1 out of ∼ 10 000 cloud droplets contains a bacteria cell. Zhang et al. (2021) showed that such low CCN number concentrations likely have a negligible effect on the formation and properties of warm clouds. However, in the same study, it was pointed out that information on the hygroscopic properties of bacteria is essential as it determines the volume of the aquatic environment that is important for microbial growth, survival, and functioning (Sect. 2.2).
The low bacteria concentrations in the atmosphere imply that bacteria cells are separated by considerable distances. Figure 2 shows schematically the average distance between cells (δcell) for typical atmospheric bacteria cell concentrations (0.001 cm cm−3) (Sect. S1.2). The schematic shows that bacteria can be expected to be separated, on average, by several centimeters (∼2.2–10 cm), whereas other aerosol particles or cloud droplets are separated by several millimeters (δp, δdr). This social distancing of bacteria impairs the collective traits and functions that bacteria can benefit from in other environments (Ross and Whiteley, 2020). In addition to such mutualistic behavior, bacteria also exhibit antagonistic interactions in communities, i.e., benefiting from cell separation (Russel et al., 2017; Peterson et al., 2020). Such antagonistic effects include the competition for limited resources, particularly among metabolically similar species, as encountered in the atmosphere. The functioning and survival of bacterial communities are usually due to a balance between mutualism and antagonism. However, the specific conditions facilitating such balance in different ecosystems may shift under atmospheric conditions, therefore influencing bacterial metabolism and survival. Reduced antagonism in the atmosphere due to the physical separation of cells through aerosolization may partly explain the sustained activity and survival of atmospheric bacteria despite the overall harsh environmental conditions.
The role of such substantial distances between cells in the atmosphere has not been explored yet as lab experiments are usually performed on bulk samples. For example, Vaïtilingom et al. (2010, 2011) derived biodegradation rates in cloud water samples (∼100 mL) that combined billions of droplets containing several ten thousands of cells. Such bulk experiments are convenient and are often the only way to monitor signals above the detection limit, but they do not reflect the same conditions that microorganisms experience in dispersed cloud droplets (Sect. 2.3 and 2.4.2). In addition, the chemical microstructure in individual droplets may be different to that in bulk solutions (Wei et al., 2018). Novel experimental set-ups that allow the investigation of levitated droplets containing microorganisms seem promising with regard to overcoming current methodological limitations (Fernandez et al., 2019).
2.2 Water availability: are atmospheric microbial oases limited to clouds?
All organisms, including microorganisms, need water for their biological functioning. The water content of surface waters is unlimited (at least – presumably – from a microbial perspective); the water content of soils is typically on the order of several volume percent. Clouds represent air masses with the largest amounts of liquid water in the atmosphere, yet, they only comprise 10−5–10−4 volume % of the total atmosphere, corresponding to typical liquid water contents of 0.1< LWCcloud<1 g m−3. Even in the absence of clouds, aerosol particles are never completely dry. In fact, aerosol water is often a substantial fraction of a particle mass depending on particle hygroscopicity and the surrounding relative humidity (RH). The total aerosol water content might amount to several hundreds of micrograms per cubic meter of air (µg m), corresponding to volume %.
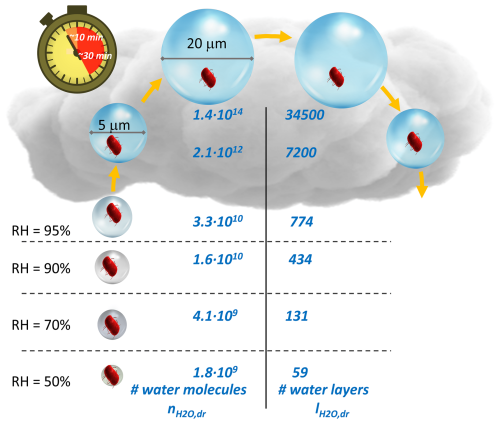
Figure 3Number of water molecules () and water layers () under subsaturated conditions (RH <100 %) and for cloud droplets with diameters of 5 and 20 µm (Sect. S2.1). The schematic suggests that, under a wide range of atmospheric RH conditions, bacteria cells are surrounded by at least ∼60 water layers, which corresponds to about 20 mass % of the water in a bacteria cell ( molecules water per cell). Since typical lifetimes of cloud droplets are on the order of 10–30 min, the largest water volumes may only represent very short-lived “oases” (Sect. S2.2).
The water content of atmospheric aerosol particles is often expressed by means of the hygroscopicity parameter κ. Strictly, the concept of hygroscopicity does not apply to bacteria cells as they do not fully dissolve in water; their water uptake may be triggered by the formation of biosurfactants that are present at the air–water interface and that attract water and slow down water evaporation to trap the water in the immediate surroundings of the cell (Gill et al., 1983).
Using κbact=0.1 to estimate the amount of water around a cell, the number and layers of water molecules, and , can be derived (Fig. 3 and Sect. S2.1): at RH ∼90 %, the water masses inside and outside of a cell are approximately equal (∼1010 water molecules). At RH ∼50 %, there are several tens of layers of water around a bacteria cell (), corresponding to about 20 % of the water inside a bacteria cell. Figure 3 summarizes and values over the range of RH or water activities (aw∼ RH/100 %) in which microbial activity, including cell division, has been observed (Stevenson et al., 2015, 2017). The presented and values are possibly biased low as they imply hygroscopic growth that often occurs only immediately after particle emission in the atmosphere. After their first deliquescence event, particles usually stay in a metastable state above their efflorescence RH, which implies that they retain condensed water due to hysteresis of evaporation. In addition, during their residence time in the atmosphere, hygroscopic material likely condenses on bacteria-containing particles, resulting in even more water uptake. Moreover, Nielsen et al. (2024) showed that electrolytes associated with the cell, such as sodium chloride, may significantly increase the water uptake.
When RH exceeds 100 %, cloud droplets may form, depending on the supersaturation that is a function of the cooling rate (vertical velocity) as a source and the available drop surface area as a condensational sink. While clouds can exist for several hours or even days, they are highly dynamic systems. Cloud droplets form near the cloud base and grow while ascending, followed by shrinkage in descending (i.e., warming) air masses (Fig. 3). These upward and downward motions constrain the lifetime of an individual cloud droplet to about 10–30 min, depending on cloud thickness and vertical velocity (Sect. S2.2). Thus, such high-water-volume conditions may only exist for very short times during which bacteria need to adapt to the in-cloud environment, which differs greatly from the environment outside clouds in terms of water availability, light, temperature, etc. Lab experiments in (artificial) cloud water revealed lag times of several hours before bacteria started to efficiently biodegrade organic cloud water constituents (Vaïtilingom et al., 2013). This lag time is comparable to that observed for bacteria growth after the rewetting of soil (“Birch effect”) (Leizeaga et al., 2022). If, indeed, conditions of high bacterial activity were limited to the in-cloud time, hysteresis effects might impede enhanced microbial activity inside clouds since droplets evaporated before the end of the lag period.
Clouds have been previously termed “microbial oases” in the atmosphere (Amato et al., 2017), implying that they provide the most ideal atmospheric conditions for bacterial activity in a transient habitat. However, given the very rapid changes in the environmental conditions over short timescales, clouds may actually trigger several stress responses that differ from those under lower RH (20 %–90 %) conditions (Péguilhan et al., 2023, 2024). Provided that bacteria are surrounded by a considerable amount of water at ≳90 % RH, the range of biologically favorable conditions might actually extend to much longer temporal and spatial scales than estimated previously (Ervens and Amato, 2020). Such considerations might be relevant for regions and/or periods of high relative humidity (e.g., in the tropics and polar regions and/or during the nighttime). It remains to be explored whether microorganisms in the atmosphere adjust their stress responses and biological functions over the full continuum of RH conditions rather than switching behaviors at specific RH thresholds (e.g., inside or outside clouds).
2.3 Accessibility to nutrients: are nutrient levels in droplets high enough?
In the atmosphere, bacteria cells may exhibit different levels of metabolic activity, which range from mere survival strategies, i.e., activity focused solely on repairing cellular damage, to dormancy, during which cells sustain their essential biological functions, to growth and multiplication as the most energy-intensive activities (Price and Sowers, 2004). Cells may become dormant under water-limited conditions (Haddrell and Thomas, 2017) or due to other stressors (Šantl-Temkiv et al., 2022). In cloud water, Sattler et al. (2001) observed cell activity at 0 °C compatible with cell growth, whereas dormancy was observed outside clouds (Smets et al., 2016). Given that particles (including bacteria calls) only spend a fraction of their time inside clouds (Ervens and Amato, 2020), it can thus be expected that many bacteria may be dormant for long periods of their atmospheric residence time. Dormancy has been shown in other environments to be an efficient response to harsh conditions, ultimately being beneficial for survival (Jones and Lennon, 2010).
In most natural waters, the concentrations of organic nutrients are sufficiently high that they do not lead to carbon-limiting conditions for heterotrophs (Eiler et al., 2003). In oligotrophic ocean regions or lakes, bacteria develop strategies to adapt to the lower nutrient levels, including the optimization of their energy use by producing less bacterial secondary mass while enhancing respiration (del Giorgio and Cole, 1998). The main organic nutrients for bacteria in rivers are low-molecular-weight (LMW with ≲500 Da) compounds that comprise a major fraction of the total dissolved organic carbon (TOC) (Catalán et al., 2017). The total concentration of biodegradable LMW compounds in surface waters is on the order of 50 µmol L−1 (0.005–0.4 µmol L−1 for individual compounds; Table S3 in the Supplement), which overlaps with the range found for individual compounds in fog and cloud water (0.1–10 µmol L−1, Herckes et al., 2013). This similarity is not surprising as volatile, water-soluble compounds are continuously emitted and/or formed in the connected spheres, followed by (thermodynamic) partitioning at the atmosphere–water interfaces of cloud droplets and surface waters. The incorporation of bacteria cells into the aqueous phase does not follow the same thermodynamic principles, yet, bacteria cell concentrations in rivers and seawater (∼103–105 cell mL−1) are comparable to those in cloud water (104–106 cell mL−1) (Amato et al., 2017).
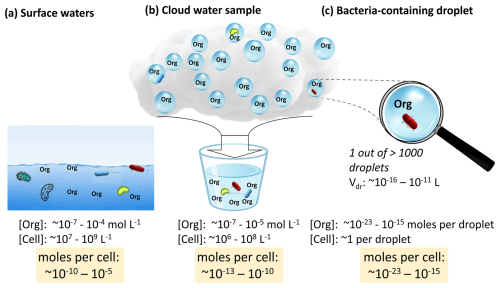
Figure 4Typical concentrations of bacteria cells and organics in surface waters (Table S3) and cloud water samples (Herckes et al., 2013) and resulting organic-to-cell ratios. Given that 1 out of >1000 cloud droplets contains a bacteria cell, this bulk ratio is reduced by several orders of magnitude in individual bacteria-containing cloud droplets (–10−15 mol cell−1; Sect. S3), potentially suggesting limited availability of organic nutrients in cloud droplets.
The similarity in organic nutrient levels and bacteria cell concentrations in the different water phases may suggest equivalent organic-to-cell ratios. However, this conclusion only applies to the comparison of bulk aqueous volumes, e.g., cloud water samples where all bacteria-containing and bacteria-free cloud droplets are combined (Fig. 4b). In contrast, within the microcosms of individual bacteria-containing cloud droplets, the organic-to-cell ratio is much smaller since the cell concentration is 1 per droplet (0 in >99.9 % of the droplets), while the solute concentration [mol L−1] is that of the bulk cloud water sample. This results in organic-to-cell ratios in cloud droplets of mol cell−1 as opposed to a range of 10−13 to 10−10 mol cell−1 in surface waters (Fig. 4c and Sect. S3). As a consequence, the steady-state nutrient levels per cell in the bacteria-containing droplets are at least 2, possibly up to 10, orders of magnitude lower than those in other aquatic environments.
Previous lab experiments conducted to derive biodegradation rates in cloud water used organic-to-cell ratios in the range of 10−12–10−10 mol cell−1 (Vaïtilingom et al., 2010, 2011). Process models explored the role of biodegradation in chemical budgets in the atmospheric multiphase system (Khaled et al., 2021; Nuñez López et al., 2024) and found that the nutrient uptake from the gas phase may not be sufficiently fast to replenish biodegraded organics in the small subset of droplets, in which microorganisms are present and potentially active, resulting in even lower nutrient-to-cell ratios. In the same studies, it was shown that simplified model assumptions of an “averaged cell concentration” in each droplet (i.e., <0.001 cells per droplet, e.g., Pailler et al., 2023) may lead to wrong conclusions regarding the role of biodegradation as the nutrient-to-cell ratio is substantially overestimated. In light of these considerations, we suggest that lab and model studies should be performed for droplet-relevant organic-to-cell ratios to systematically explore potential limitation thresholds for individual bacteria strains and nutrients. Results from such studies would lead to a more accurate categorization of the atmosphere in terms of the trophic level. They could be also used to support (or refute) the classification of bacteria in rain as “extremophiles”, as put forward by Guillemette et al. (2023) based on much lower TOC levels in rain as compared to in marine surface waters and in the deep sea.
Extrapolating the conclusions as made above for clouds to potential biological activity in aerosol particles (diameter ≲1 µm) possibly suggests even greater nutrient limitation at RH <100 %. However, the partitioning of LMW volatile organics into aerosol water can greatly differ from that to (relatively) dilute droplets. For example, the fraction of formic acid partitioned to aerosol water is up to 7 orders of magnitude higher than that predicted based on Henry's law (Liu et al., 2012). Such strong partitioning could compensate for the lower water volume. Thus, one may hypothesize that nutrient-to-cell ratios in wet aerosol particles and cloud droplets are similar. However, since the water and oxidant contents are different in aerosol particles and cloud droplets (Sect. 2.2 and 2.4.2), the resulting osmotic and oxidative stress levels in these two different aqueous regimes might lead to different nutrient utilization rates.
2.4 Sunlight and oxidants: is the high photochemical activity in the atmosphere always stressful?
2.4.1 Solar radiation
The photic zone of surface waters, i.e., the layer penetrated by sunlight, is limited to the first few meters below the air–water interface; in soil, this layer is even shallower and restricted to a few millimeters to centimeters. In all environments, including the atmosphere, the photolysis of dissolved organic matter results in LMW compounds that are often more biodegradable. At the same time, products of photochemical reactions include reactive oxygen species (ROS), such as the OH radical, that oxidize organics reducing the concentration levels of organic nutrients (Scully et al., 2003). In addition, ROS cause oxidative stress to bacteria cells as they can damage the cellular structure. Therefore, sunlight has both beneficial and adverse effects on microbial functioning and survival. Airborne particles are exposed to sunlight for about half of their atmospheric residence time (during the day) as opposed to the much lower photochemical activity in surface environments. DNA damage due to UV light in the atmosphere can be parameterized as a function of irradiance and bacteria type (Madronich et al., 2018).
The actinic flux inside clouds represents a particular challenge as it can be enhanced or reduced as compared to cloud-free air depending on cloud optical density and the height in the cloud (Ryu et al., 2017). Enhanced actinic fluxes occur when light is scattered or reflected multiple times inside “bright” clouds with low optical thickness and few and/or small droplets. Conversely, sunlight cannot readily penetrate through dense, “dark” clouds, possibly resulting in lower UV-induced stress for bacteria. Thus, the macrostructures and microstructures of clouds (e.g., vertical profiles of density) have to be taken into account to estimate the timescales during which bacteria are exposed to detrimental UV conditions. To counteract such UV-induced stress, bacteria may develop strategic mechanisms such as the formation of pigments as a protective shield. However, such mechanisms may only become effective after some lag time that has not been quantified under atmospheric conditions.
2.4.2 OH radical
The OH radical is one of the most powerful oxidants and “cleansing agents” in the atmosphere and in aquatic environments. Its main formation pathways in surface water include the direct photolysis of hydrogen peroxide (H2O2) and the Fenton reaction (Fe(II) oxidation by H2O2). In the atmosphere, it is mainly formed in the gas phase via the photolysis of ozone and HONO but also via the reaction of the hydroxy peroxy radical (HO2) with NO. The rate of the latter process is significantly reduced in cloudy air masses since the highly soluble HO2 partitions into cloud water, where it is quickly consumed, while the less soluble NO remains in the gas phase. This reactant separation leads to significantly lower total HO2 and OH concentrations in the presence of clouds (reductions of up to 70 % and 80 %, respectively; Ervens, 2015).
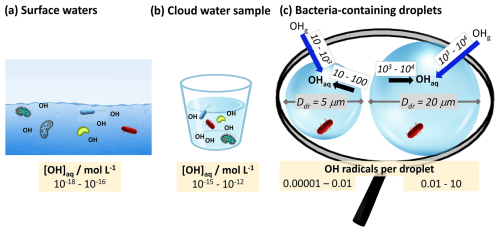
Figure 5Concentrations of the OH radical in (a) seawater (Mopper and Zhou, 1990), (b) cloud water (Arakaki et al., 2013), and (c) individual cloud droplets (Ddr=5, 20 µm). The blue arrows in panel (c) denote the uptake rates of OH radicals per second, and the black arrows denote their production rate inside a droplet (see Fig. S6c for individual reactions).
At typical cloud liquid water contents, less than 0.1 % of all atmospheric OH radicals reside in cloud water. The aqueous-phase concentration in cloud water [mol L−1] is greater by about 3 orders of magnitude than in seawater (Fig. 5a vs. b). This comparison may imply – at first sight – higher oxidative stress due to OH in the atmosphere. However, this conclusion may need to be carefully reconsidered if one defines the number of OH radicals in the immediate surroundings of a bacteria cell as a direct measure of the OH oxidative stress for bacteria. Figure 5c shows exemplarily that the steady-state OH concentration per droplet only exceeds 1 in droplets with diameters Ddr>20 µm (Sect. S4). It should be kept in mind, though, that steady-state concentrations are a result of OH source and loss rates that usually (nearly) cancel each other out. OH sources in cloud droplets include the direct uptake from the gas phase and chemical reactions with rates of ∼10–10 000 OH radicals per second (Fig. 5c), with the Fenton reaction being one of the main sources (Fig. S6b in the Supplement). However, unlike the other aqueous-phase OH sources (e.g., H2O2 or photolysis), the Fenton reaction only occurs in the subset of cloud droplets that contain iron (∼10 %, Khaled et al., 2022; Ervens, 2022). Thus, the oxidative stress due to Fenton chemistry, as observed in other aquatic environments (Cabiscol et al., 2000), may be much lower in the atmosphere and may only occur in 0.01 % of all droplets or particles ( assuming a statistical distribution of iron and bacteria across a drop population). The OH uptake from the gas phase is often not sufficiently fast to replenish the consumed radicals and reach equilibrium concentrations ( mol L−1), resulting in the lowest OH concentrations in large droplets (Ervens et al., 2014).
Microorganisms apply strategies to scavenge oxidants and respond to oxidative stress. The conditions under which oxidant scavenging rates cannot be compensated for by defense mechanisms depend on numerous factors, including the bacteria species and oxidant (concentration and species) and environmental conditions, such as temperature, relative humidity, and nutrient availability. Systematic studies to constrain such conditions have not been performed yet in dispersed droplets. The aqueous photooxidation experiments conducted on living bacteria in the presence of OH by Liu et al. (2023) might serve as a first indication; however, the observed maximum survival time of 6 h in a large aqueous-phase volume likely represents a lower limit as compared to realistic in-cloud processing times, during which OH-induced stress levels may be lower.
2.4.3 Other ROS and oxidants (e.g., 3C*, 1O2, H2O2)
While the OH radical is one of the most powerful chemical oxidants in the atmosphere, other oxidants are present at high concentrations as well, including H2O2, HO2, triplet states (3C*), singlet oxygen (1O2), and ROS formed by photosensitizers, which all cumulatively contribute to the oxidative stress of bacteria in clouds. Due to its high total concentration and solubility, H2O2 represents (one of) the most abundant ROS in cloud droplets. Wirgot et al. (2019) showed that microorganisms can metabolize H2O2 in aqueous solution with a chemical composition similar to that of cloud water. The experiments were conducted in a closed system; thus, it was not assessed whether biodegradation was sufficiently fast to completely detoxify a droplet that is continuously exposed to a gas-phase H2O2 reservoir. Simultaneous measurements of H2O2 in the atmospheric gas and aqueous phases usually show thermodynamic equilibrium. However, such measurements reflect the conditions in the bulk cloud water; they do not allow the detection of small deviations due to biodegradation in a few droplets.
The concentrations of the more reactive 3C* and 1O2 in cloud droplets are 1 to 2 orders of magnitude higher than that of OH (Kaur and Anastasio, 2018). Thus, scaling up the results shown for the OH radical in Fig. S6a by a factor of 10–100 suggests that there are a few 3C* and 1O2 molecules in each cloud droplet. The formation rates of these oxidants under typical cloud conditions have not been fully characterized and implemented in multiphase chemistry models, and their metabolic consumption is even less constrained. Thus, the extent to which the particular oxidant mix in cloud water – which is likely to be different from that in other aquatic environments – affects oxidative stress levels and, ultimately, metabolic functioning and survival in the atmosphere cannot be assessed. As compared to cloud droplets, the concentrations of 3C*, 1O2, and H2O2 in aerosol water are higher by 1 to 2 orders of magnitude (Arellanes et al., 2006; Ma et al., 2023), whereas OH concentrations are similar in both systems. However, the chemical conversion rates of the oxidants might be much higher than in droplets due to higher precursor concentrations (e.g., ). At the same time, the microorganisms are exposed to higher ionic strength in the much less dilute particles, which might alter their ability to apply efficient anti-oxidative defense mechanisms. Such mechanisms could include the formation of biosurfactants that slow down or even impede uptake from or evaporation to the gas phase (Gill et al., 1983). Due to the lack of kinetic and mechanistic data to describe chemical formation and loss rates of ROS in aerosol particles, the ROS budgets in aerosol water cannot be speciated on a molecular level. Therefore, dedicated lab experiments and process model studies should be designed to constrain oxidant levels in the atmospheric aqueous phases to ultimately determine their effect on microbial oxidative stress levels.
2.4.4 pH value
Numerous studies point to the fact that atmospherically relevant bacteria (e.g., Pseudomonas sp.) show the highest growth and activity rates at only mildly acidic or neutral pH values. However, they have developed various strategies to survive pH conditions outside these ranges. They include intracellular buffering to maintain the pH within the cytoplasm or proton pumps that regulate the intracellular proton concentrations (Kobayashi et al., 2000; Lund et al., 2020). Such mechanisms may explain the weak dependence of biodegradation rates on pH, as found in lab studies of (artificial) cloud water (Vaïtilingom et al., 2010), as the intracellular pH is kept at (nearly) neutral values. Liu et al. (2023) found different trends when they examined the pH dependence of the survival and biodegradation rates of two strains of Enterobacter bacteria isolated from ambient air in a polluted environment: they showed that, in the presence of light, the survival rate decreased, particularly at pH ≤5. These trends may point to different sensitivities of this particular bacteria type to pH as compared to the responses by bacteria in cloud water (Vaïtilingom et al., 2013). The concurrent responses to a low pH and the presence of sunlight may suggest some photolytic or photochemical mechanism that influences the biodegradation activity. In the limited volume of a cloud droplet or aqueous aerosol particle, the number of protons is small as compared to that in bulk aqueous phases. Thus, the individual cells in such volumes have to “combat” a limited number of protons (Fig. S7). Thus, the adjustment of the pH of the surrounding aqueous phase, as observed in other environments (Ratzke and Gore, 2018), might be easier in the small droplet or particle volumes. Such rates of buffering-agent production and proton transfer likely depend on the bacteria types and environmental factors and therefore should be explored for atmospheric conditions.
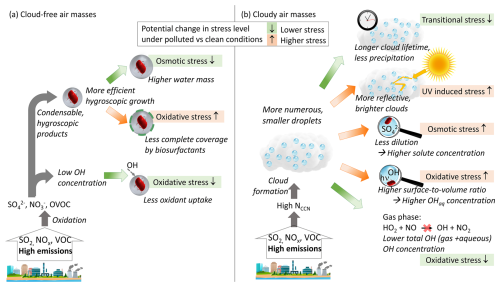
Figure 6Potential microbial stress responses due to chemical or microphysical processes in polluted air masses. It is not implied that each of these factors is necessarily important or significant with regard to having beneficial or adverse effects on bacteria. Instead, the illustration intends to demonstrate that correlations between atmospheric pollutants do not imply causation. (a) Cloud-free air masses: osmotic stress may be lowered due to the addition of the hygroscopic mass that attracts more water (Sect. 2.2), whereas oxidative stress may increase if particles exceed sizes too large to be covered by biosurfactants to shield the particles from oxidant uptake. Total atmospheric oxidant levels may be comparably small in a polluted atmosphere since OH is efficiently titrated (Sect. 2.4.2). (b) Cloudy air masses: high aerosol number concentrations lead to clouds with more numerous but smaller cloud droplets (“cloud lifetime effect”). Such clouds are less likely to precipitate, but are, at the same time, less dense and brighter as light reflections between droplets are amplified (Sect. 2.4.1). Smaller droplets typically contain higher solute and oxidant (OH) concentrations, which may enhance both osmotic and oxidative stressors. The latter may be (partially) compensated for by the fact that total (gas + aqueous) OH and HO2 concentrations are usually much lower in the presence of clouds due to smaller formation rates in the gas phase (Sect. 2.4.2).
Observations of atmospheric trends between chemical concentrations and bacterial abundance and community structure (including diversity) are, to a large extent, determined by air mass types, history, and age (Gandolfi et al., 2013; Innocente et al., 2017). Several atmospheric studies aimed to identify atmospheric chemical or physical parameters that influence the abundance, diversity, and/or viability of atmospheric microorganisms. Such studies often resulted in contradictory conclusions regarding the impact of specific atmospheric conditions (e.g., pollution or clouds) on the atmospheric microbiome. Lebowitz and O'rourke (1991) cautioned against concluding on links between aerobiological and chemico-physical contaminants in determining factors that potentially trigger adverse health effects. In view of the microscale chemical and microphysical factors discussed in Sect. 2, similarly, we emphasize the importance of not confusing causation with correlation with regard to atmospheric parameters affecting microbial stress.
Figure 6 summarizes the chemical or microphysical factors in polluted air masses that may affect different types of microbial stress levels (oxidative, osmotic, UV-induced, transitional). None of the shown effects imply a direct adverse or beneficial influence by a specific pollutant (e.g., SO2, NOx, volatile organic compounds (VOCs), or their oxidation products) on bacteria activity level, viability, diversity, or abundance. We do not suggest that such effects do not exist; however, to the best of our knowledge, corresponding biomarkers (specific response genes, enzymes) have not been identified yet. Also, we do not propose that, indeed, all listed chemical or microphysical parameters trigger significant stress responses or that any of the indicated responses dominate or cancel each other. Instead, the schematic may be used as a qualitative – or even speculative – illustration to motivate studies quantifying the potential role of the individual factors in targeted experiments. The results of such studies may then be used to make conclusions regarding the role of each of these factors to derive robust cause-and-effect relationships. Falsely derived conclusions of causation may ultimately result in inappropriate strategies to maintain healthy ecosystems and biodiversity in a changing atmosphere.
In cloud-free air masses, an increase in the hygroscopicity of the bacteria-containing particle may lead to lower osmotic stress but, possibly, to more efficient ROS uptake from the gas phase (Fig. 6a). Whether such a protection mechanism is significant at all or negligible as compared to the lower OH levels that exist in polluted air masses remains to be seen.
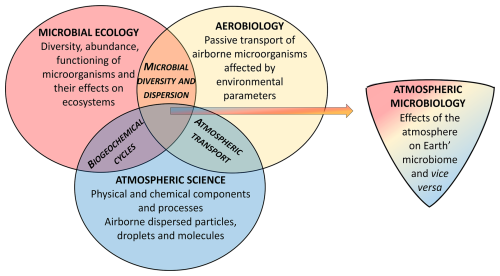
Figure 7Atmospheric microbiology forms the intersection of atmospheric sciences, microbial ecology, and aerobiology.
As pointed out in the previous sections, many microphysical processes determine the microscopic and macroscopic structure of clouds, including droplet size distributions that determine droplet lifetime (smaller droplets are less likely to precipitate) and cloud optical thickness (Sect. 2.4.1), which may have opposite effects on stress levels (Fig. 6b). In turn, higher particle concentrations in polluted air masses result in smaller, more numerous droplets with higher solute and OH concentrations, i.e., enhancing osmotic and oxidative stress. However, since OH (and several other oxidant) levels are reduced in the presence of clouds (Sect. 2.4.2), an apparent lower stress level in the presence of clouds could possibly just be ascribed to exposure to lower oxidant levels.
Such considerations are likely to be just a small subset of bio-physico-chemical feedbacks in the atmospheric multiphase system used to explain observed trends. Adding to the vagueness is the lack of comprehensive, speciated bacterial emission maps and patterns. Bacteria are detached from the surface and lifted by mechanical forces (e.g., strong wind), just like any other primary particle. In addition, there might be biological selection criteria that trigger aerosolization and emission of specific microorganisms. Since bacteria emission fluxes into the atmosphere are poorly constrained, observed trends between bacteria-related parameters and other environmental factors may just be coincidental since the atmospheric composition is a result of the mixing of air masses of different origins and ages. “Chemical clocks”, e.g., the ratio of co-emitted but differently reactive compounds, are often used to determine the “chemical age of air”. Equivalent measures to determine similar indicators for the “biological age of air” are still lacking. Such indicators would not only be useful in determining the viability and survival along air mass trajectories but would also allow us to make conclusions regarding emission patterns at different locations.
Atmospheric microbiology is an interdisciplinary research field at the intersection of atmospheric sciences, aerobiology, and microbial ecology. Figure 7 illustrates their overlap and lists some shared research objectives (biogeochemical cycles, microbial diversity and dispersion, and atmospheric transport). Scientific concepts of all three areas have to be taken into account for a comprehensive characterization of atmospheric microorganisms as a small but important dynamically, rapidly changing and evolving fraction of Earth's microbiome.
Numerous previous studies focused on the role of microorganisms in the atmosphere in terms of their impact on clouds or chemical concentrations. By now, there is general consensus that this role may be limited to specific regions, conditions, and processes, such as ice nucleation near 0 °C or biodegradation of selected compounds as a significant atmospheric sink. However, despite the limited global influence of microorganisms on the atmosphere and climate, further studies of the microbial bio-physico-chemical properties are needed to explore the ability of microorganisms with regard to taking up water and/or being incorporated into clouds (hygroscopicity, ice nucleation ability). This information will be important to correctly estimate the atmospheric processing times of microorganisms and, therefore, their fate. Such studies can apply the same methodologies used for other atmospheric particles but should be motivated by research questions targeted at understanding the role of the atmosphere in microorganisms rather than the reverse. Similarly, the rationale for exploring biodegradation rates in cloud water could be extended from focusing on potential impacts on chemical budgets to consequences of limited nutrient availability for levels of metabolic activity, including dormancy, starvation, and survival.
Studies on microbial optimization strategies for water uptake or nutrient utilization are not new; they have been conducted for many decades in the context of aquatic and terrestrial environments. Transferring such established methods to the atmospheric microbiome is challenging for (at least) two reasons: firstly, atmospheric-bacteria number concentrations are extremely low. Thus, collecting statistically meaningful samples requires large volumes and/or long timescales. This complicates the identification of patterns and sources as air masses efficiently mix over time. Despite the difficulties associated with the statistically relevant sampling of atmospheric microorganisms, the identification of individual environmental factors that control the concentration (Archer et al., 2019; Gusareva et al., 2019), diversity (Tong and Lighthart, 1997; Bryan et al., 2019), and selection (Smith et al., 2011; Joly et al., 2015) of atmospheric microorganisms has begun. While it remains difficult to perform sampling with a sufficiently high resolution and frequency, dedicated strategies should be developed to constrain the role of individual environmental parameters (e.g., UV light, temperature) for microbial diversity and survival in the atmosphere. Such studies should be accompanied by suitable lab or chamber studies under controlled conditions to test hypotheses that may be formed based on ambient observations.
Secondly, unlike in other environments where bacteria can form aggregates and biofilms or are suspended in water, atmospheric bacteria are aerosolized before they are lifted and dispersed in air. The immediate environment of airborne bacteria is constrained by particle or drop volumes. We highlight such specific conditions due to bacteria aerosolization, which implies distances of several centimeters between cells, which makes their living environments different from those in (more) homogeneous environments. Previously, conclusions on potential stress factors or nutrient availability in the atmosphere were drawn based on the concentration levels in atmospheric bulk samples, where ≲0.1 % bacteria-containing droplets are mixed with ≳99.9 % bacteria-free droplets (particles). In dispersed droplets (particles), the concentration ratios of cells to nutrients or oxidants, respectively, should be considered on a per-droplet (per-particle) basis. The much lower cell-to-nutrient ratio in an individual droplet () as compared to that in bulk samples implies a much lower trophic regime for bacteria than previously thought. The atmosphere is considered to be a harsh environment for microorganisms due to numerous factors, including high photochemical activity. However, droplets and particles contain only a few oxidant molecules (e.g., OH, 3C*,1O2) that are quickly transformed chemically but are possibly also metabolized. To describe the particular mixtures of nutrients and oxidants that bacteria are exposed to in the aerosol and/or droplet microcosms, process models should be applied with detailed atmospheric multiphase chemistry, microphysics, and data on biological processes (e.g., biotransformation of nutrients or ROS). However, these latter data are largely lacking, particularly from studies on individual (e.g., levitated) droplets or particles. Given the challenges associated with such experimental set-ups, we recommend that bulk experiments should at least take into account realistic solute mixtures.
In summary, we provide a new perspective on atmospheric microbiology within the context of atmospheric chemistry and microphysics. We emphasize the importance of specific spatial and temporal scales of microbial microcosms in individual particles and droplets. Such considerations are essential to enhance our understanding of the atmosphere as an extension of the more well-characterized microbial environments such as oceans and soil. Ultimately, this will lead to a complete characterization of the Earth microbiome and its cycling that ensures global ecological stability and functioning.
The Supplement contains all equations that were applied for calculations using the cited literature values.
The supplement related to this article is available online at: https://doi.org/10.5194/bg-22-243-2025-supplement.
BE developed the idea and the methodology of the paper and wrote its initial draft. PA, KA, MJ, AK, TLV, FM, LNL, RP, and MZ contributed by means of critical review and commentaries to the initial and revised versions of the manuscript.
The contact author has declared that none of the authors has any competing interests.
Publisher's note: Copernicus Publications remains neutral with regard to jurisdictional claims made in the text, published maps, institutional affiliations, or any other geographical representation in this paper. While Copernicus Publications makes every effort to include appropriate place names, the final responsibility lies with the authors.
We thank Zeina Bourhane, Anne-Marie Delort, Florent Rossi, and Jonathan Vyskocil for the useful discussions.
This research has been supported by the Agence Nationale de la Recherche (grant no. ANR-17-MPGA-0013).
This paper was edited by Paul Stoy and reviewed by two anonymous referees.
Amato, P., Joly, M., Besaury, L., Oudart, A., Taib, N., Moné, A. I., Deguillaume, L., Delort, A., and Debroas, D.: Active microorganisms thrive among extremely diverse communities in cloud water, PLOS One, 12, e0182869, https://doi.org/10.1371/journal.pone.0182869, 2017. a, b
Arakaki, T., Anastasio, C., Kuroki, Y., Nakajima, H., Okada, K., Kotani, Y., Handa, D., Azechi, S., Kimura, T., Tsuhako, A., and Miyagi, Y.: A general scavenging rate constant for reaction of hydroxyl radical with organic carbon in atmospheric waters, Environ. Sci. Technol., 47, 8196–8203, https://doi.org/10.1021/es401927b, 2013. a
Archer, S. D. J., Lee, K. C., Caruso, T., Maki, T., Lee, C. K., Cary, S. C., Cowan, D. A., Maestre, F. T., and Pointing, S. B.: Airborne microbial transport limitation to isolated Antarctic soil habitats, Nat. Microbiol., 4, 925–932, https://doi.org/10.1038/s41564-019-0370-4, 2019. a
Arellanes, C., Paulson, S. E., Fine, P. M., and Sioutas, C.: Exceeding of Henry's Law by Hydrogen Peroxide Associated with Urban Aerosols, Environ. Sci. Technol., 40, 4859–4866, https://doi.org/10.1021/es0513786, 2006. a
Bar-On, Y. M., Phillips, R., and Milo, R.: The biomass distribution on Earth, P. Natl. Acad. Sci. USA, 115, 6506–6511, https://doi.org/10.1073/pnas.1711842115, 2018. a
Boetius, A., Anesio, A. M., Deming, J. W., Mikucki, J. A., and Rapp, J. Z.: Microbial ecology of the cryosphere: sea ice and glacial habitats, Nat. Rev. Microbiol., 13, 677–690, https://doi.org/10.1038/nrmicro3522, 2015. a
Bryan, N. C., Christner, B. C., Guzik, T. G., Granger, D. J., and Stewart, M. F.: Abundance and survival of microbial aerosols in the troposphere and stratosphere, ISME J., 13, 2789–2799, https://doi.org/10.1038/s41396-019-0474-0, 2019. a
Burrows, S. M., Butler, T., Jöckel, P., Tost, H., Kerkweg, A., Pöschl, U., and Lawrence, M. G.: Bacteria in the global atmosphere – Part 2: Modeling of emissions and transport between different ecosystems, Atmos. Chem. Phys., 9, 9281–9297, https://doi.org/10.5194/acp-9-9281-2009, 2009a. a, b, c
Burrows, S. M., Elbert, W., Lawrence, M. G., and Pöschl, U.: Bacteria in the global atmosphere – Part 1: Review and synthesis of literature data for different ecosystems, Atmos. Chem. Phys., 9, 9263–9280, https://doi.org/10.5194/acp-9-9263-2009, 2009b. a
Cabiscol, E., Tamarit, J., and Ros, J.: Oxidative stress in bacteria and protein damage by reactive oxygen species, International microbiology: the official journal of the Spanish Society for Microbiology, 3, 3–8, 2000. a
Cardinale, B. J., Duffy, J. E., Gonzalez, A., Hooper, D. U., Perrings, C., Venail, P., Narwani, A., Mace, G. M., Tilman, D., Wardle, D. A., Kinzig, A. P., Daily, G. C., Loreau, M., Grace, J. B., Larigauderie, A., Srivastava, D. S., and Naeem, S.: Biodiversity loss and its impact on humanity, Nature, 486, 59–67, https://doi.org/10.1038/nature11148, 2012. a
Catalán, N., Casas-Ruiz, J. P., von Schiller, D., Proia, L., Obrador, B., Zwirnmann, E., and Marcé, R.: Biodegradation kinetics of dissolved organic matter chromatographic fractions in an intermittent river, J. Geophys. Res.-Biogeo., 122, 131–144, https://doi.org/10.1002/2016JG003512, 2017. a
del Giorgio, P. A. and Cole, J. J.: Bacterial growth efficiency in natural aquatic systems, Annu. Rev. Ecol. Syst., 29, 503–541, https://doi.org/10.1146/annurev.ecolsys.29.1.503, 1998. a
Després, V. R., Huffman, J. A., Burrows, S. M., Hoose, C., Safatov, A. S., Buryak, G., Fröhlich-Nowoisky, J., Elbert, W., Andreae, M. O., Pöschl, U., and Jaenicke, R.: Primary biological aerosol particles in the atmosphere: a review, Tellus B, 64, 15598, https://doi.org/10.3402/tellusb.v64i0.15598, 2012. a
Eiler, A., Langenheder, S., Bertilsson, S., and Tranvik, L. J.: Heterotrophic Bacterial Growth Efficiency and Community Structure at Different Natural Organic Carbon Concentrations, Appl. Environ. Microb., 69, 3701–3709, https://doi.org/10.1128/AEM.69.7.3701-3709.2003, 2003. a
Ervens, B.: Modeling the Processing of Aerosol and Trace Gases in Clouds and Fogs, Chem. Rev., 115, 4157–4198, https://doi.org/10.1021/cr5005887, 2015. a
Ervens, B.: Average cloud droplet size and composition: Good assumptions for predicting oxidants in the atmospheric aqueous phase?, J. Phys. Chem. A, 126, 8295–8304, https://doi.org/10.1021/acs.jpca.2c05527, 2022. a
Ervens, B. and Amato, P.: The global impact of bacterial processes on carbon mass, Atmos. Chem. Phys., 20, 1777–1794, https://doi.org/10.5194/acp-20-1777-2020, 2020. a, b, c
Ervens, B., Sorooshian, A., Lim, Y. B., and Turpin, B. J.: Key parameters controlling OH-initiated formation of secondary organic aerosol in the aqueous phase (aqSOA), J. Geophys. Res.-Atmos., 119, 3997–4016, https://doi.org/10.1002/2013JD021021, 2014. a
Fankhauser, A. M., Antonio, D. D., Krell, A., Alston, S. J., Banta, S., and Mc Neill, V. F.: Constraining the Impact of Bacteria on the Aqueous Atmospheric Chemistry of Small Organic Compounds, ACS Earth Space Chem, 3, 1485–1491, https://doi.org/10.1021/acsearthspacechem.9b00054, 2019. a, b
Fernandez, M. O., Thomas, R. J., Garton, N. J., Hudson, A., Haddrell, A., and Reid, J. P.: Assessing the airborne survival of bacteria in populations of aerosol droplets with a novel technology, J. R. Soc. Interface, 16, 20180779, https://doi.org/10.1098/rsif.2018.0779, 2019. a
Gandolfi, I., Bertolini, V., Ambrosini, R., Bestetti, G., and Franzetti, A.: Unravelling the bacterial diversity in the atmosphere, Applied Microbiology and Biotechnology, 97, 4727–4736, https://doi.org/10.1007/s00253-013-4901-2, 2013. a
Gill, P. S., Graedel, T. E., and Weschler, C. J.: Organic films on atmospheric aerosol particles, fog droplets, cloud droplets, raindrops, and snowflakes, Rev. Geophys., 21, 903–920, https://doi.org/10.1029/RG021i004p00903, 1983. a, b
Guillemette, R., Harwell, M. C., and Brown, C. A.: Metabolically active bacteria detected with click chemistry in low organic matter rainwater, PLOS ONE, 18, e0285816, https://doi.org/10.1371/journal.pone.0285816, 2023. a
Gusareva, E. S., Acerbi, E., Lau, K. J. X., Luhung, I., Premkrishnan, B. N. V., Kolundžija, S., Purbojati, R. W., Wong, A., Houghton, J. N. I., Miller, D., Gaultier, N. E., Heinle, C. E., Clare, M. E., Vettath, V. K., Kee, C., Lim, S. B. Y., Chénard, C., Phung, W. J., Kushwaha, K. K., Nee, A. P., Putra, A., Panicker, D., Yanqing, K., Hwee, Y. Z., Lohar, S. R., Kuwata, M., Kim, H. L., Yang, L., Uchida, A., Drautz-Moses, D. I., Junqueira, A. C. M., and Schuster, S. C.: Microbial communities in the tropical air ecosystem follow a precise diel cycle, P. Natl. Acad. Sci. USA, 116, 201908493, https://doi.org/10.1073/pnas.1908493116, 2019. a
Haddrell, A. E. and Thomas, R. J.: Aerobiology: Experimental Considerations, Observations, and Future Tools, Appl. Environ. Microbiol., 83, e00809-17, https://doi.org/10.1128/AEM.00809-17, 2017. a
Herckes, P., Valsaraj, K. T., and Collett Jr., J. L.: A review of observations of organic matter in fogs and clouds: Origin, processing and fate, Atmos. Res., 132–133, 434–449, https://doi.org/10.1016/j.atmosres.2013.06.005, 2013. a, b
Innocente, E., Squizzato, S., Visin, F., Facca, C., Rampazzo, G., Bertolini, V., Gandolfi, I., Franzetti, A., Ambrosini, R., and Bestetti, G.: Influence of seasonality, air mass origin and particulate matter chemical composition on airborne bacterial community structure in the Po Valley, Italy, Sci. Total Environ., 593–594, 677–687, https://doi.org/10.1016/j.scitotenv.2017.03.199, 2017. a
Joly, M., Amato, P., Sancelme, M., Vinatier, V., Abrantes, M., Deguillaume, L., and Delort, A. M.: Survival of microbial isolates from clouds toward simulated atmospheric stress factors, Atmos. Environ., 117, 92–98, https://doi.org/10.1016/j.atmosenv.2015.07.009, 2015. a
Jones, S. E. and Lennon, J. T.: Dormancy contributes to the maintenance of microbial diversity, P. Natl. Acad. Sci. USA, 107, https://doi.org/10.1073/pnas.0912765107, 2010. a
Kaur, R. and Anastasio, C.: First Measurements of Organic Triplet Excited States in Atmospheric Waters, Environ. Sci. Technol., 52, 5218–5226, https://doi.org/10.1021/acs.est.7b06699, 2018. a
Khaled, A., Zhang, M., Amato, P., Delort, A.-M., and Ervens, B.: Biodegradation by bacteria in clouds: an underestimated sink for some organics in the atmospheric multiphase system, Atmos. Chem. Phys., 21, 3123–3141, https://doi.org/10.5194/acp-21-3123-2021, 2021. a
Khaled, A., Zhang, M., and Ervens, B.: The number fraction of iron-containing particles affects OH, HO2 and H2O2 budgets in the atmospheric aqueous phase, Atmos. Chem. Phys., 22, 1989–2009, https://doi.org/10.5194/acp-22-1989-2022, 2022. a
Kobayashi, H., Saito, H., and Kakegawa, T.: Bacterial strategies to inhabit acidic environments, J. Gen. Appl. Microbiol., 46, 235–243, https://doi.org/10.2323/jgam.46.235, 2000. a
Lebowitz, M. D. and O'rourke, M. K.: The significance of air pollution in aerobiology, Grana, 30, 31–43, https://doi.org/10.1080/00173139109427766, 1991. a
Leizeaga, A., Meisner, A., Rousk, J., and Bååth, E.: Repeated drying and rewetting cycles accelerate bacterial growth recovery after rewetting, Biol. Fert. Soils, 58, 365–374, https://doi.org/10.1007/s00374-022-01623-2, 2022. a
Lindow, S. E. and Brandl, M. T.: Microbiology of the phyllosphere, Appl. Environ. Microb., 69, 1875–1883, https://doi.org/10.1128/AEM.69.4.1875-1883.2003, 2003. a
Liu, J., Zhang, X., Parker, E. T., Veres, P. R., Roberts, J. M., de Gouw, J. A., Hayes, P. L., Jimenez, J. L., Murphy, J. G., Ellis, R. A., Huey, L. G., and Weber, R. J.: On the gas-particle partitioning of soluble organic aerosol in two urban atmospheres with contrasting emissions: 2. Gas and particle phase formic acid, J. Geophys. Res.-Atmos., 117, D00V21, https://doi.org/10.1029/2012jd017912, 2012. a
Liu, Y., Lee, P. K. H., and Nah, T.: Emerging investigator series: Aqueous photooxidation of live bacteria with hydroxyl radicals under clouds-like conditions: Insights into the production and transformation of biological and organic matter originating from bioaerosols, Environmental Science: Processes & Impacts, https://doi.org/10.1039/D3EM00090G, 2023. a, b
Lund, P. A., De Biase, D., Liran, O., Scheler, O., Mira, N. P., Cetecioglu, Z., Fernández, E. N., Bover-Cid, S., Hall, R., Sauer, M., and O'Byrne, C.: Understanding How Microorganisms Respond to Acid pH Is Central to Their Control and Successful Exploitation, Front. Microbiol., 11, 556140, https://doi.org/10.3389/fmicb.2020.556140, 2020. a
Ma, L., Worland, R., Tran, T., and Anastasio, C.: Evaluation of Probes to Measure Oxidizing Organic Triplet Excited States in Aerosol Liquid Water, Environ. Sci. Technol., 57, 6052–6062, https://doi.org/10.1021/acs.est.2c09672, 2023. a
Madronich, S., Björn, L. O., and McKenzie, R. L.: Solar UV radiation and microbial life in the atmosphere, Photoch. Photobio. Sci., 17, 1918–1931, https://doi.org/10.1039/C7PP00407A, 2018. a
Mopper, K. and Zhou, X.: Hydroxyl radical photoproduction in the sea and its potential impact on marine processes, Science, 250, 661–664, https://doi.org/10.1126/science.250.4981.661, 1990. a
Nielsen, L. S., Šantl-Temkiv, T., Palomeque Sánchez, M., Massling, A., Ward, J. C., Jensen, P. B., Boesen, T., Petters, M., Finster, K., Bilde, M., and Rosati, B.: Water Uptake of Airborne Cells of P. syringae Measured with a Hygroscopicity Tandem Differential Mobility Analyzer, Environ. Sci. Technol., 58, 19211–19221, https://doi.org/10.1021/acs.est.4c01817, 2024. a
Nuñez López, L., Amato, P., and Ervens, B.: Bacteria in clouds biodegrade atmospheric formic and acetic acids, Atmos. Chem. Phys., 24, 5181–5198, https://doi.org/10.5194/acp-24-5181-2024, 2024. a
Pailler, L., Wirgot, N., Joly, M., Renard, P., Mouchel-Vallon, C., Bianco, A., Leriche, M., Sancelme, M., Job, A., Patryl, L., Armand, P., Delort, A.-M., Chaumerliac, N., and Deguillaume, L.: Assessing the efficiency of water-soluble organic compound biodegradation in clouds under various environmental conditions, Environmental Science: Atmospheres, 3, 731–748, https://doi.org/10.1039/D2EA00153E, 2023. a
Pasteur, L.: Mémoire sur les corpuscles organisés qui existent dans l'atmosphère, examen de la doctrine des générations spontanées, Masson, 1861. a
Péguilhan, R., Rossi, F., Joly, M., Nasr, E., Batut, B., Enault, F., Ervens, B., and Amato, P.: Clouds, oases for airborne microbes – Differential metagenomics/metatranscriptomics analyses of cloudy and clear atmospheric situations, bioRxiv [preprint], 2023.12.14.571671, https://doi.org/10.1101/2023.12.14.571671, 2023. a
Péguilhan, R., Rossi, F., Joly, M., Nasr, E., Batut, B., Enault, F., Ervens, B., and Amato, P.: Clouds influence the functioning of airborne microorganisms, EGUsphere [preprint], https://doi.org/10.5194/egusphere-2024-2338, 2024. a
Peterson, S. B., Bertolli, S. K., and Mougous, J. D.: The Central Role of Interbacterial Antagonism in Bacterial Life, Curr. Biol., 30, R1203–R1214, https://doi.org/10.1016/j.cub.2020.06.103, 2020. a
Poorter, H., Niinemets, Ü., Poorter, L., Wright, I. J., and Villar, R.: Causes and consequences of variation in leaf mass per area (LMA): a meta-analysis, New Phytol., 182, 565–588, https://doi.org/10.1111/j.1469-8137.2009.02830.x, 2009. a
Pöschl, U. and Shiraiwa, M.: Multiphase Chemistry at the Atmosphere–Biosphere Interface Influencing Climate and Public Health in the Anthropocene, Chem. Rev., 115, 4440–4475, https://doi.org/10.1021/cr500487s, 2015. a
Price, P. B. and Sowers, T.: Temperature dependence of metabolic rates for microbial growth, maintenance, and survival, P. Natl. Acad. Sci. USA, 101, 4631–4636, https://doi.org/10.1073/pnas.0400522101, 2004. a
Ratzke, C. and Gore, J.: Modifying and reacting to the environmental pH can drive bacterial interactions, PLOS Biol., 16, e2004248, https://doi.org/10.1371/journal.pbio.2004248, 2018. a
Robinson, J. M. and Breed, M. F.: The aerobiome–health axis: a paradigm shift in bioaerosol thinking, Trends Microbiol., 31, 661–664, https://doi.org/10.1016/j.tim.2023.04.007, 2023. a
Ross, B. N. and Whiteley, M.: Ignoring social distancing: advances in understanding multi-species bacterial interactions, Faculty Reviews, 9, 23, https://doi.org/10.12703/r/9-23, 2020. a
Russel, J., Røder, H. L., Madsen, J. S., Burmølle, M., and Sørensen, S. J.: Antagonism correlates with metabolic similarity in diverse bacteria, P. Natl. Acad. Sci. USA, 114, 10684–10688, https://doi.org/10.1073/pnas.1706016114, 2017. a
Ryu, Y.-H., Hodzic, A., Descombes, G., Hall, S., Minnis, P., Spangenberg, D., Ullmann, K., and Madronich, S.: Improved modeling of cloudy-sky actinic flux using satellite cloud retrievals, Geophys. Res. Lett., 44, 1592–1600, https://doi.org/10.1002/2016GL071892, 2017. a
Šantl-Temkiv, T., Amato, P., Casamayor, E. O., Lee, P. K. H., and Pointing, S. B.: Microbial ecology of the atmosphere, FEMS Microbiol. Rev., 46, fuac009, https://doi.org/10.1093/femsre/fuac009, 2022. a, b
Sattler, B., Puxbaum, H., and Psenner, R.: Bacterial growth in supercooled cloud droplets, Geophys. Res. Lett., 28, 239–242, https://doi.org/10.1029/2000GL011684, 2001. a, b
Scully, N. M., Cooper, W. J., and Tranvik, L. J.: Photochemical effects on microbial activity in natural waters: the interaction of reactive oxygen species and dissolved organic matter, FEMS Microbiol. Ecol., 46, 353–357, https://doi.org/10.1016/S0168-6496(03)00198-3, 2003. a
Seinfeld, J. H. and Pandis, S. N.: Atmospheric Chemistry and Physics – From air pollution to climate change, John Wiley & Sons, Inc., Hoboken, New Jersey, 2nd edn., ISBN 9780471720188, 2006. a
Smets, W., Moretti, S., Denys, S., and Lebeer, S.: Airborne bacteria in the atmosphere: Presence, purpose, and potential, Atmos. Environ., 139, 214–221, https://doi.org/10.1016/j.atmosenv.2016.05.038, 2016. a
Smith, D. J., Griffin, D. W., McPeters, R. D., Ward, P. D., and Schuerger, A. C.: Microbial survival in the stratosphere and implications for global dispersal, Aerobiologia, 27, 319–332, https://doi.org/10.1007/s10453-011-9203-5, 2011. a
Smith, D. J., Ravichandar, J. D., Jain, S., Griffin, D. W., Yu, H., Tan, Q., Thissen, J., Lusby, T., Nicoll, P., Shedler, S., Martinez, P., Osorio, A., Lechniak, J., Choi, S., Sabino, K., Iverson, K., Chan, L., Jaing, C., and McGrath, J.: Airborne Bacteria in Earth's Lower Stratosphere Resemble Taxa Detected in the Troposphere: Results From a New NASA Aircraft Bioaerosol Collector (ABC), Front. Microbiol., 9, 1752, https://doi.org/10.3389/fmicb.2018.01752, 2018. a
Stevenson, A., Burkhardt, J., Cockell, C. S., Cray, J. A., Dijksterhuis, J., Fox-Powell, M., Kee, T. P., Kminek, G., McGenity, T. J., Timmis, K. N., Timson, D. J., Voytek, M. A., Westall, F., Yakimov, M. M., and Hallsworth, J. E.: Multiplication of microbes below 0.690 water activity: implications for terrestrial and extraterrestrial life, Environ. Microbiol., 17, 257–277, https://doi.org/10.1111/1462-2920.12598, 2015. a
Stevenson, A., Hamill, P. G., O'Kane, C. J., Kminek, G., Rummel, J. D., Voytek, M. A., Dijksterhuis, J., and Hallsworth, J. E.: Aspergillus penicillioides differentiation and cell division at 0.585 water activity, Environ. Microbiol., 19, 687–697, https://doi.org/10.1111/1462-2920.13597, 2017. a
Tong, Y. and Lighthart, B.: Solar radiation has a lethal effect on natural populations of culturable outdoor atmospheric bacteria, Atmos. Environ., 31, 897–900, https://doi.org/10.1016/S1352-2310(96)00235-X, 1997. a
Vaïtilingom, M., Amato, P., Sancelme, M., Laj, P., Leriche, M., and Delort, A.-M.: Contribution of Microbial Activity to Carbon Chemistry in Clouds, Appl. Environ. Microb., 76, 23–29, https://doi.org/10.1128/AEM.01127-09, 2010. a, b, c
Vaïtilingom, M., Charbouillot, T., Deguillaume, L., Maisonobe, R., Parazols, M., Amato, P., Sancelme, M., and Delort, A.-M.: Atmospheric chemistry of carboxylic acids: microbial implication versus photochemistry, Atmos. Chem. Phys., 11, 8721–8733, https://doi.org/10.5194/acp-11-8721-2011, 2011. a, b
Vaïtilingom, M., Deguillaume, L., Vinatier, V., Samcelme, M., Amato, P., N, C., and A.-M, D.: Potential impact of microbial activity on the oxidant capacity and organic carbon budget in clouds, P. Natl. Acad. Sci. USA, 110, 559–564, https://doi.org/10.1073/pnas.1205743110, 2013. a, b
Wei, H., Vejerano, E. P., Leng, W., Huang, Q., Willner, M. R., Marr, L. C., and Vikesland, P. J.: Aerosol microdroplets exhibit a stable pH gradient, P. Natl. Acad. Sci. USA, 115, 7272–7277, https://doi.org/10.1073/pnas.1720488115, 2018. a
Whitman, W. B., Coleman, D. C., and Wiebe, W. J.: Prokaryotes: The unseen majority, P. Natl. Acad. Sci. USA, 95, 6578–6583, https://doi.org/10.1073/pnas.95.12.6578, 1998. a, b, c, d
Wirgot, N., Lagrée, M., Traïkia, M., Besaury, L., Amato, P., Canet, I., Sancelme, M., Jousse, C., Diémé, B., Lyan, B., and Delort, A.-M.: Metabolic modulations of Pseudomonas graminis in response to H2O2 in cloud water, Sci. Rep., 9, 12799, https://doi.org/10.1038/s41598-019-49319-2, 2019. a
Zhang, M., Khaled, A., Amato, P., Delort, A.-M., and Ervens, B.: Sensitivities to biological aerosol particle properties and ageing processes: potential implications for aerosol–cloud interactions and optical properties, Atmos. Chem. Phys., 21, 3699–3724, https://doi.org/10.5194/acp-21-3699-2021, 2021. a
- Abstract
- Introduction
- Atmospheric bacteria: temporal and spatial scales
- Trends of atmospheric microorganisms and pollutants: correlation, causation, or coincidence?
- Conclusions
- Code and data availability
- Author contributions
- Competing interests
- Disclaimer
- Acknowledgements
- Financial support
- Review statement
- References
- Supplement
- Abstract
- Introduction
- Atmospheric bacteria: temporal and spatial scales
- Trends of atmospheric microorganisms and pollutants: correlation, causation, or coincidence?
- Conclusions
- Code and data availability
- Author contributions
- Competing interests
- Disclaimer
- Acknowledgements
- Financial support
- Review statement
- References
- Supplement