the Creative Commons Attribution 4.0 License.
the Creative Commons Attribution 4.0 License.
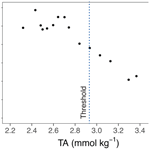
Growth response of Emiliania huxleyi to ocean alkalinity enhancement
Mathias Haunost
Allanah Joy Paul
Anne Ulrike Christiane Tietz
Ulf Riebesell
The urgent necessity of reducing greenhouse gas emissions is coupled with a pressing need for widespread implementation of carbon dioxide removal (CDR) techniques to limit the increase in mean global temperature to levels below 2 °C compared to pre-industrial times. One proposed CDR method, ocean alkalinity enhancement (OAE), mimics natural rock weathering processes by introducing suitable minerals into the ocean, thereby increasing ocean alkalinity and promoting CO2 chemical absorption. While theoretical studies hold promise for OAE as a climate mitigation strategy, careful consideration of its ecological implications is essential. Indeed, the ecological impact of enhanced alkalinity on marine organisms remains a subject of investigation, as it may lead to changes in species composition. OAE implicates favorable conditions for calcifying organisms by enhancing the saturation state of calcium carbonate and decreasing the energetic costs for calcification. This may affect marine primary production by improving conditions for calcifying phytoplankton, among which coccolithophores play the leading role. They contribute < 10 % to the global marine primary production but are responsible for a large proportion of the marine calcite deposition. While previous research has extensively studied the effects of ocean acidification on coccolithophores, fewer studies have explored the impacts of elevated pH and alkalinity. In this context, we studied the sensitivity of Emiliania huxleyi, the most widespread coccolithophore species, to ocean alkalinity enhancement in a culture experiment. We monitored the species' growth and calcification response to progressively increasing levels of total alkalinity (TA). Above a change in total alkalinity (ΔTA) of ∼ 600 µmol kg−1, as CO2 concentrations decreased, the E. huxleyi growth rate diminished, suggesting a threshold CO2 concentration of ∼ 100 µatm necessary for optimal growth. The cellular calcite to organic carbon ratio (PIC : POC) remained stable over the total alkalinity range. Due to the decreasing growth rate in response to alkalinity enhancement, total carbonate formation was lower.
OAE is rapidly advancing and has already reached the field-testing stage. Hence, our study contributes to the most critical part of investigations required to comprehend potential biological implications before large-scale OAE is adopted.
- Article
(1325 KB) - Full-text XML
- BibTeX
- EndNote
Governments have recognized the need to constrain human-induced climate change and declared to keep the mean global temperature rise well below 2 °C compared to pre-industrial values to prevent dangerous consequences associated with a further increase in temperature (United Nations Framework Convention on Climate Change, 2015). Currently, the rate at which greenhouse gas emissions are being cut is slow and insufficient to meet this target. Therefore the use of negative emission technologies (NETs) to remove atmospheric CO2 is expected to be inevitable in supporting efforts to limit global temperature rise (IPCC, 2021). One proposed method to remove CO2 from the atmosphere mimics the natural process of rock weathering, whereby suitable minerals (i.e., olivine, basalt, carbonate) are extracted and introduced into the surface ocean (ocean alkalinity enhancement, OAE; Gattuso et al., 2015; GESAMP, 2019). The dissolution of the respective minerals consumes protons, and conservative cations are released, which leads to a shift in the carbonate chemistry species from CO2 towards HCO and CO, thus to an increase in alkalinity (Wolf-Gladrow et al., 2007; Bach et al., 2019). As a result, the seawater becomes undersaturated with CO2, which is then balanced by the additional absorption of atmospheric CO2. Hence, through OAE, CO2 is chemically absorbed and subsequently safely stored mainly as bicarbonate over geological timescales (e.g., Hartmann et al., 2013). Theoretical studies indicate that OAE has the theoretical potential to remove 3 to 30 Gt of carbon dioxide yr−1 (Renforth and Henderson, 2017; Feng et al., 2017) from the atmosphere and thus contribute to global climate change mitigation efforts (e.g., Keller et al., 2014; Taylor et al., 2016; Renforth and Henderson, 2017). This provides a basis for testing the applicability, efficiency, and ecological impacts of this approach in practice. Key factors in this context are the impacts of the seawater chemistry changes arising from enhanced alkalinity on primary production and biogenic calcification. In a recent paper, Bednaršek et al. (2024) offered a conceptual synthesis of the responses of marine calcifiers to OAEs, utilizing insights from multiple ocean acidification (OA) studies. This study evidenced that only 40 % of the analyzed groups show a neutral response upon alkalinity addition. For marine microbes that rely on dissolved carbon to fuel primary production, the shifts in seawater carbonate chemistry are relevant for their physiological processes. Below certain thresholds, dissolved CO2 concentrations can indeed limit phytoplankton growth and lead to reduced primary production (Riebesell et al., 1993). In addition, OAE leads to beneficial changes in carbonate chemistry for biogenic calcification due to an increased pH and carbonate saturation state and thus may promote calcifying organisms (Bach et al., 2019; Bednaršek et al., 2024). The formation of calcium carbonate, however, consumes alkalinity and is a CO2 source (Zeebe and Wolf-Gladrow, 2001), so increased calcification rates would counteract CO2 drawdown through OAE.
Coccolithophores are the most important calcifiers in marine phytoplankton. They contribute significantly to the marine primary production in the surface ocean and the deposition of calcite in deep-sea sediments (Broecker and Clark, 2009; Poulton et al., 2007). In the last decades, many studies investigated the impact of OA on the growth and calcification of coccolithophores (e.g., Riebesell et al., 2000; Langer et al., 2006; Bach et al., 2013; Faucher et al., 2020; Paul and Bach, 2020) and evidenced the sensitivity of these organisms to changes in carbonate chemistry. Increased pCO2 and lower pH induce significant effects on the growth and calcification of coccolithophores (e.g., Riebesell et al., 2000; Beaufort et al., 2011; Bach et al., 2011, 2015). Most efforts focused on manipulating the level of pCO2 rather than alkalinity. Only a few of them investigated the effect of higher pH and lower pCO2 on these organisms (e.g., Langer et al., 2006; Bach et al., 2015), indicating a reduction in the growth rate of the species tested when pCO2 is below ∼ 100 µatm (Sett et al., 2014; Bach et al., 2011, 2015), and offered first insights into the potential effects of OAE on coccolithophore growth.
OAE is based on adding alkalinity through different approaches, which raises carbonate chemistry parameters in various ways. Recently, Gately et al. (2023) showed a neutral response of two phytoplankton species, the coccolithophore Emiliania huxleyi and the diatom Chaetoceros sp., to OAE in an experiment where the culture media were bubbled for some days to equilibrate with the atmosphere before the algae were inoculated. This approach avoided a strong perturbation of the carbonate chemistry (i.e., avoidance of pH spikes and pCO2-limiting conditions). Their results show that, even in the highest alkalinity treatment (ΔTA ∼ 2700 µmol kg−1), the pCO2 was above 300 µatm and the pH only slightly increased by 0.4 units. The apparent resilience of these species to increased alkalinity in the study of Gately et al. (2023) is encouraging, considering a potential application of OAE. However, in a non-equilibrated OAE approach where atmospheric equilibration of CO2 is prevented (sensu Hartmann et al., 2023; Suitner et al., 2024), the carbonate chemistry shifts towards lower pCO2 and higher [CO], pH, and saturation states (Ω) for calcite and aragonite (Zeebe and Wolf-Gladrow, 2001). The impact of these more extreme conditions on marine calcifiers, such as coccolithophores, has not yet been sufficiently investigated in order to assess the effects of ocean OAE on marine primary production.
Emiliania huxleyi is the most abundant and widespread coccolithophore species in the modern ocean (Westbroek et al., 1993) and served as a model species in plenty of studies that investigated the impact of ocean acidification on coccolithophores (see Wheeler et al., 2023). Of the five coccolithophore species included in the meta-analysis of Bednaršek et al. (2024), E. huxleyi stood out as the sole species where calcification did not display a neutral effect of increased alkalinity on calcification.
In this study, we tested the response of E. huxleyi in growth and calcification under progressively increasing total alkalinity (TA) levels. The increase in alkalinity was achieved by adding a sodium hydroxide (NaOH) solution to the culture medium. The medium was not allowed to equilibrate with the atmosphere after NaOH addition, and the response of E. huxleyi to decreasing CO2 concentrations and increasing pH due to enhanced alkalinity was investigated.
The study aimed to test whether (i) the growth rate of E. huxleyi decreases under reduced CO2 concentrations due to enhanced alkalinity and (ii) the carbonate chemistry conditions under increasing TA (i.e., increasing [HCO], lower [H+]) that are conducive to calcification lead to physiological disbalances between calcification and growth.
2.1 Culture conditions and experimental design
Monospecific cultures of Emiliania huxleyi (B92/11; Plymouth Marine Laboratory) were grown in batch cultures at low biomass (< 60 000 cells mL−1; Zondervan et al., 2002) to avoid a significant impact of biological processes on the chemical conditions of the growth medium. Emiliania huxleyi was grown at 15 °C in a photoperiod of 16 : 8 h light to dark and a photon flux of 150 µmol m−2 s−1 (measured with a LI-COR LI-250A light meter). The growth medium was based on artificial seawater (Kester et al., 1967) with a salinity of 34, which was enriched with 64 µmol kg−1 NaNO3, 4 µmol kg−1 NaHPO4, and trace metals and vitamins according to the medium (Guillard and Ryther, 1962). In addition, 10 nmol kg−1 SeO2 was added (Danbara and Shiraiwa, 1999) along with 2 mL kg−1 of 0.2 µm filtered natural seawater to prevent the potential growth limitation of E. huxleyi due to other substances not included in the receipt (Bach et al., 2011). The medium was filtered (0.2 µm) into sterile 0.6 L polycarbonate bottles where different amounts of NaOH (1 M) solution were added to set up an alkalinity gradient among the replicates. The alkalinity gradient was set up across 16 distinct treatments, with intervals of 50–100 µmol kg−1 from ambient TA levels of 2350 up to ∼ 3500 µmol kg−1. After the addition of NaOH, the bottles were sealed gastight, and the headspace inside was kept as low as possible to minimize gas exchange. Small volumes of a pre-culture with exponentially growing E. huxleyi were pipetted into the bottles to meet target cell concentrations of around 100 cells mL−1. The cells were acclimated for seven to nine generations to the respective alkalinity conditions, and samples of 0.5 mL were taken at selected time points to monitor the concentration and growth of E. huxleyi. After the acclimation phase, a small volume of the cultures was transferred into a further set of polycarbonate bottles (2.7 L) filled with fresh NaOH-manipulated medium. Cells were homogenized by gentle rotation, and samples for TA, dissolved inorganic carbon (DIC), nutrients, and cell abundance were taken. Thereupon, the content of the bottles was gently transferred into smaller (2 L) polycarbonate bottles for the main experiment. Bottles were filled from bottom to top using a hose and a funnel to limit gas exchange and avoid bubble formation. The headspace inside the bottles was kept at < 5 mL. Bottles were regularly gently rotated to keep the cultures in suspension. The growth period during the main experiment was estimated from the respective cell concentrations determined during the acclimation phase. Cells were harvested at low biomass to keep the change in dissolved inorganic carbon (DIC) due to the buildup of biogenic carbon below 4 % over the growth period. Samples for cell concentrations, particulate organic carbon (POC) and particulate inorganic carbon (PIC) content, and nutrient concentrations were taken at the end of the experiment.
2.2 Determination of the carbonate system
Samples for TA and DIC measurements were 0.2 µm filtered with low pressure, poisoned with a saturated HgCl2 solution (0.5 ‰ final concentration) and stored at 4 °C until analysis. TA concentrations were determined by potentiometric titration with a Metrohm Compact 862 Titrosampler and corrected with certified reference material (A. Dickson, Scripps Institution of Oceanography, La Jolla, California; Dickson et al., 2003). DIC concentrations were measured with an Automated Infrared Inorganic Carbon Analyzer (AIRICA) equipped with a LI-COR detector (LI-7000 CO2 H2O analyzer) and corrected with the certified reference material. The carbonate system parameters were calculated from salinity, temperature, TA, and DIC using the program CO2SYS (Pierrot et al., 2006) with equilibrium constants from Mehrbach et al. (1973), refit by Dickson and Millero (1987).
2.3 Determination of E. huxleyi concentrations and growth rate
Samples of 0.5 mL were transferred into Eppendorf tubes, and the cell concentrations of E. huxleyi were measured with a flow cytometer (Accuri C6, Becton Dickinson) equipped with a 488 nm diode laser at a flow rate of 66 µL min−1. Cells were determined based on their red fluorescence signal (> 670 nm) and forward light scatter. Daily growth rates (μ, d−1) were determined using Eq. (1):
where N denotes the cell concentration of E. huxleyi and t represents the time in days.
2.4 Particulate inorganic carbon (PIC) and particulate organic carbon (POC)
Samples for total particulate carbon (TPC) and particulate organic carbon (POC) were obtained by gentle filtration of the cultures through combusted (500 °C for 5 h) glass fiber filters (0.7 µm), which were stored at −20 °C until analysis. POC filters were kept in a desiccator with fuming hydrochloric acid (37 %) for 2 h to remove all particulate inorganic carbon (PIC). After that, TPC and POC filters were dried overnight at 60 °C, and their carbon content was measured in an elemental analyzer (Euro EA, EuroVector). The amount of PIC was determined as the difference between TPC and POC. PIC and POC production rates were calculated for each sample by multiplying the μ with the cellular POC or PIC contents. The ratio of PIC to POC is indicative of the cellular degree of calcification.
2.5 Statistical analyses and data fitting
The data were analyzed using a nonlinear regression model. Numerous treatment levels were established without replication, aiming to provide quantitative insights for ecological modeling while preserving statistical power (Cottingham et al., 2005). The coefficients of determination (R2) and p-values for the regression model are presented in the Results section. The Monod equations were used to analyze the growth rate, chlorophyll a (Chl a), and calcification relating to resource concentration (e.g., Riebesell et al., 1993; Rost et al., 2003). The Monod function is defined as Eq. (2):
where V represents the growth rate (or POC or PIC); Vmax is the maximum growth rate (or POC or PIC) at high, non-limiting concentrations; R is the resource concentration in the environment; and k is the half-saturation constant, the resource concentration at which the growth rate is half of its maximum value. All statistics and curve-fitting were conducted in RStudio 2022.12.0 (R packages “drc” and “ggplot2”; R Core Team, 2023).
3.1 TA manipulation and carbonate chemistry
The addition of NaOH led to a steady TA increase among the culture bottles up to a concentration of Δ1200 µmol kg−1 (Fig. 1a). The measured TA values at the beginning of the experiment were slightly lower than the target concentrations (Fig. 1a; Table 1). The deviation from target concentrations increased with increasing concentrations of NaOH added. The TA values at the end of the experiment were slightly lower in most treatments. Also, the DIC concentrations show some minor fluctuations from the beginning to the end of the experiment (Fig. 1b).
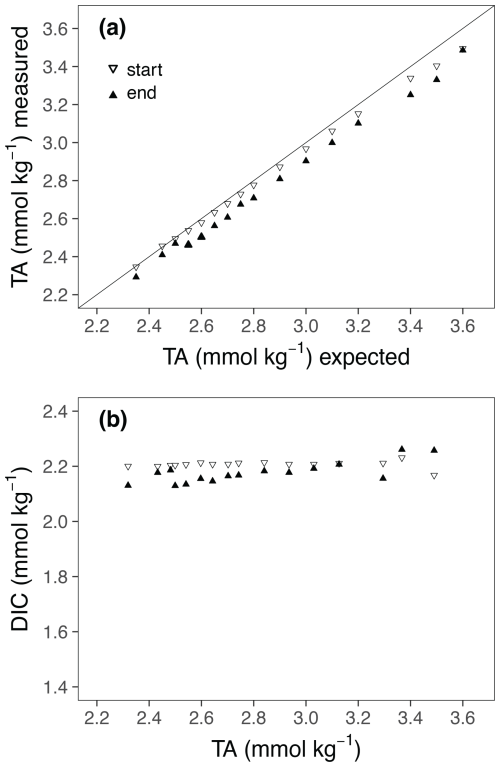
Figure 1(a) Comparison of targeted and measured TA values at the start and end of the experiment. (b) TA : DIC diagrams at the start and end of the experiments.
The effects of increasing TA on the carbonate chemistry parameters are shown in Fig. 2 and Table 1. The concentrations of CO2 [CO2] and bicarbonate ions [HCO] decreased with increasing TA (Fig. 2a, b), whereas the carbonate ion concentrations [CO] increased (Fig. 2c). The saturation state of calcite (Ωcalcite) increases with increasing TA from 2.7 in the lowest treatment to 24 in the highest treatment (Table 1).
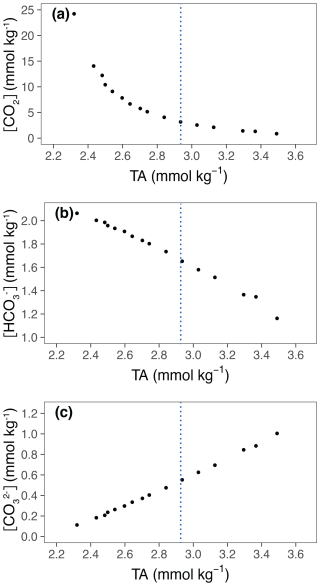
Figure 2Relevant carbonate chemistry parameters in relation to TA. TA is the average of the values at the start and at the end of the experiment. (a) [CO2], (b) [HCO], (c) [CO]. The blue lines represent ΔTA 600 µmol kg−1, which is the level of alkalinity beyond which E. huxleyi shows a decline in growth.
3.2 Physiological response of E. huxleyi
The growth rate (μ) of E. huxleyi, the production of POC and PIC, decreased with increasing TA (Table 2; Fig. 3a–c). There was no significant effect of TA on the ratio of PIC to POC (R2=0.003, p = 0.806) (Table 2; Fig. 3d). The growth rate declined with decreasing [CO2] and was analyzed using the Monod equation (Fig. 4a). The fit predicts a maximum growth rate of 1.24 d−1 (confidence interval (CI): 1.1–1.38 d−1) with a residual standard error of 0.09 d−1 (14 df) and a half-velocity constant μmax=2.04 µmol CO2 kg−1. POC production was a function of μ and likewise decreased with declining [CO2] (Table 2; Fig. 4b). The corresponding saturation function results in a maximum POC production rate of 21.10 (CI: 12.43–29.77) pg C cell−1 d−1 with a residual standard error of 3.48 pg C cell−1 d−1 and a half-saturation constant of 5.02 µmol kg−1 CO2. The PIC production rate generally decreased with decreasing [HCO] (Fig. 4c; R2=0.40, p < 0.01) and followed the same pattern of the saturation function plotted over the [CO2] (Fig. 4d). The Monod equation predicts a maximum PIC production rate of 9.7 pg C cell−1 d−1 (CI: 5.89–13.52) pg C cell−1 d−1 with a residual standard error of 2.58 pg C cell−1 d−1. The low PIC value (0.11 pg cell−1 d−1) obtained at TA 2499 µmol kg−1 is likely an outlier. We hypothesized that this discrepancy may be due to an error in reporting the filtration volume for TPC for this sample (Table 1). Despite this, the sample was retained for analysis, as no other anomalies were observed in either the carbonate chemistry parameters or the growth of E. huxleyi. Statistical analyses were conducted both with and without this sample, and no significant differences were observed in the final results.
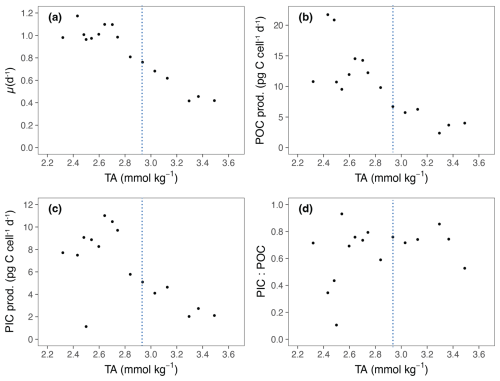
Figure 3Physiological response parameters for Emiliania huxleyi in relation to TA. TA is the average of the values at the start and at the end of the experiment. (a) Growth rates (μ). (b) Particulate organic carbon production (POC prod.). (c) Particulate inorganic carbon production (PIC prod.). (d) PIC : POC ratio. The blue lines represent ΔTA 600 µmol kg−1, which is the level of alkalinity beyond which E. huxleyi shows a decline in growth.
This experiment was conducted according to the guidelines of best practice in ocean alkalinity research (Oschlies et al., 2023), which emphasize the importance of small-scale laboratory experiments (Iglesias-Rodríguez et al., 2023) in contributing to the assessment of an environmental safety approach of OAE. Bottle experiments are considered essential to comprehending the responses of keystone species to various OAE application scenarios, enabling the understanding of the physiological performance of model species and the identification of potential tipping points in OAE.
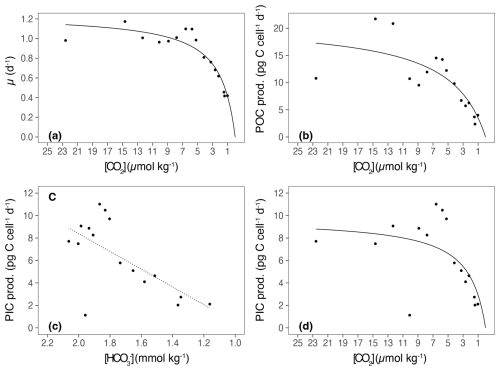
Figure 4Growth rates and cellular POC and PIC productions in relation to [CO2] and [HCO]. (a) Growth rate (μ) in relation to [CO2]. The solid line represents the Michaelis–Menten fit in the form of V= Vmax× fCO + fCO2, where V is the growth at a specific fCO2, Vmax is the theoretical maximum of growth or production, and Km is the fCO2 at which the maximum is half saturated (R2=0.87, p < 0.0001). (b) POC production (POC prod.) in relation to [CO2], using the Michaelis–Menten fit. In this case, V is the POC at a specific fCO2, Vmax is the theoretical maximum of production, and Km is the fCO2 at which the maximum is half saturated (R2=0.65, p < 0.05). (c) PIC production (PIC prod.) in relation to [HCO]. The fit according to the linear regression is given (R2=0.40, p < 0.01). (d) PIC production (PIC prod.) in relation to [CO2], using the Michaelis–Menten fit. In this case, V is the PIC at a specific fCO2, Vmax is the theoretical maximum of production, and Km is the fCO2 at which the maximum is half saturated (R2=0.40, p = 0.12). The biological response data are plotted against the means of the initial and final [CO2] and [HCO] values.
Among the different sources of alkalinity, the addition of NaOH in a non-equilibrated way increasingly gains attention due to its smaller environmental footprint and high dissolution rates (Hartmann et al., 2023; Iglesias-Rodríguez et al., 2023; Riebesell et al., 2023). For these reasons, NaOH is considered one of the most suitable feedstocks for OAE in pelagic environments (Eisaman et al., 2023; Iglesias-Rodríguez et al., 2023), with field trials already considering its use. The addition of alkalinity leads to a perturbation of the carbonate chemistry, the extent of which depends on the amount of alkalinity added. The delivery of alkalinizing substances from platforms, pipes, or ships to the ocean is expected to cause an initial, localized impact, potentially raising the pH above 9 (e.g., Bach et al., 2019; Suitner et al., 2024). These substances will dilute over years to decades (He and Tyka, 2023), lessening the disturbance. However, it is important to consider the biological impacts of the initial discharge. The localized, temporary increase in alkalinity and pH could create extreme conditions for marine organisms, potentially forming impact hotspots that affect phytoplankton species diversity and growth, with repercussions on trophic interactions higher up the food chain (Bach et al., 2019). The range tested in this study remains within the proposed alkalinity targets, reaching approximately 3000–4000 µmol kg−1 (Renforth and Henderson, 2017). In this experiment, the highest TA tested was below 4000 µmol kg−1.
In this study, the response of the biogeochemically important coccolithophore species, E. huxleyi, to increasing alkalinity was tested in a system where the carbonate chemistry was changed towards the decline of the [CO2] while the total concentration of DIC remained constant. Any gas exchange and equilibration with the atmosphere was excluded, simulating the pronounced carbonate chemistry perturbations that occur in the non-equilibrated transient state immediately after alkalinity addition.
Previous studies showed that the growth rate and POC production of coccolithophores are dependent on CO2 concentrations (e.g., Krug et al., 2011; Bach et al., 2011, 2013, 2015). Most of these studies provided limited data for conditions where the pH is higher than 8.4 and [CO2] < 6 µmol kg−1. These values are not unrealistic because, in natural environments, E. huxleyi can occasionally experience CO2 limitation in post-bloom phases when [CO2] falls below 7.5 µmol kg−1 (Bach et al., 2013). Similarly, some OAE scenarios predict even stronger CO2 limitation with values below 5 µmol kg−1 (Hartmann et al., 2023; Suitner et al., 2024).
In our study, E. huxleyi exhibited strong reductions in the growth and production of POC (below 7 pg cell−1 d−1) starting at ∼ΔTA600 µmol kg−1 (fCO2 < 86 µatm; [CO2] < 3.1 µmol kg−1), suggesting a threshold below which E. huxleyi primary production was limited by [CO2]. However, there was only a weak relationship between calcification rates and HCO concentration. Furthermore, the PIC : POC ratio remained stable, with values always lower than 1.
Our study does not have the purpose of conceptually understanding the acquisition of DIC and its subsequent use in photosynthesis and calcification under the perturbation induced by OAE. However, the knowledge gained from ocean acidification studies can help link some physiological mechanisms when E. huxleyi is subjected to low [CO2]. Under low [CO2], the phytoplankton, to overcome the constraints inflicted by an inefficient carbon uptake and to maintain higher photosynthetic efficiency, have developed the so-called carbon concentration mechanisms (CCMs) that function to enhance the HCO concentration inside the cell and promote its conversion into CO2 (Badger et al., 1998). The timing of CCM evolution may have been a response to declining atmospheric CO2 concentrations during the Neogene, thus enabling Noelaerhabdaceae, like E. huxleyi, to maintain competitive growth rates under lower CO2 levels (Bolton and Stoll, 2013; Bach et al., 2013).
Indeed, several studies indicate that CO2 is the primary source of growth and photosynthesis and that E. huxleyi up-regulates its CCMs when CO2 decreases to avoid a shortage in CO2 (Rost et al., 2003; Bach et al., 2013). HCO is an additional inorganic carbon source for growth and Chl a production of E. huxleyi (Paasche, 1964; Rost et al., 2003; Schulz et al., 2007) under low [CO2]. The system demonstrates constrained effectiveness, primarily due to elevated CO2 loss rates from leakage (Rost et al., 2006): observations indicate that, in coccolithophores, CO2 leakage escalates as [CO2] levels fall below 20 PA (Trimborn et al., 2007), concomitant with a pronounced decline in growth rates and POC production. This pattern could explain the reduction in growth and POC observed in our study when [CO2] is lower than 100 µmol kg−1 (ΔTA 600).
It is also possible that the insufficient CO2 supply for growth and photosynthesis indirectly limits calcification. In principle, the alkalinity addition increased Ωcalcite (Table 2), which should be a favorable condition that reduces the metabolic costs for calcification in coccolithophores (Bach et al., 2019). Our experiment shows a decrease in calcification with increasing alkalinity, but the data cannot prove whether calcification is indirectly limited by the insufficient CO2 and/or HCO supply for photosynthesis and growth. Since it is known that the formation of the coccosphere in E. huxleyi is closely related to the growth of the individual cell (Kottmeier et al., 2020), we speculate that the observed reduction in PIC production in our data can be explained by the reduction in growth rate under a [CO2]-limited scenario.
Gately et al. (2023) recently investigated the effect of “moderate” (ΔTA ∼ 700 µmol kg−1) and “high” (ΔTA ∼ 2700 µmol kg−1) alkalinity enhancement on the coccolithophore E. huxleyi in comparison to the diatom Chaetoceros sp. The manipulation allowed atmospheric equilibration of CO2 after TA manipulation, and only mild fluctuations in pH were tested. The study evidenced that an equilibrated limestone-inspired alkalinity enhancement has little effect on the physiological performance of E. huxleyi, suggesting that calcifiers may be relatively resilient to OAE when CO2 is not a limiting factor.
On the other hand, our study evidenced that the increase in alkalinity without CO2 equilibration or with incomplete CO2 equilibration affects the growth rate of E. huxleyi due to CO2 limitation beyond a ΔTA increase of 600 µmol kg−1 seawater.
CO2 equilibration would occur on the order of weeks to months (Ringham et al., 2024); thus, within the period after the addition of alkaline, E. huxleyi may experience a competitive disadvantage compared to other species or phytoplankton groups that have more effective CCM strategies. Since the cellular calcification (PIC : POC) remains stable under increasing TA, the overall calcite production will decrease along with decreasing growth rates in this scenario.
To conclude, to consider the applicability of OAE at a large scale, it is important to investigate the potential effects of enhanced alkalinity on both entire marine communities and relevant key taxa, since even small effects on single species could alter phytoplankton community composition, with significant impacts on their population size that translate into their ecological and biogeochemical relevance. Given that numerous studies on ocean acidification have demonstrated species- and even strain-specific reactions to changes in carbonate chemistry (e.g., Langer et al., 2006, 2009; Bach et al., 2015), the complexity of this pattern is further emphasized. Hence, further physiological data are urgently required to indicate the relevant processes that need to be investigated in community-level studies before OAE application can be considered.
The datasets presented in this study are available upon request.
MH designed the experiments with CAUT. CAUT carried out the experiments. GF analyzed the data and prepared the article with contributions from all co-authors.
Allanah J. Paul has been employed by the non-profit organization Bellona as a CDR Research and Technology Advisor since October 2023 but completed the research reported in this article prior to starting this role. Allanah is also an external scientific advisor to Seafields (https://www.seafields.eco/, last access: 1 July 2024), an aquaculture business for CDR using seaweed.
Publisher’s note: Copernicus Publications remains neutral with regard to jurisdictional claims made in the text, published maps, institutional affiliations, or any other geographical representation in this paper. While Copernicus Publications makes every effort to include appropriate place names, the final responsibility lies with the authors.
We thank Kerstin Nachtigall, Levka Hansen, and Jannes Hoffman for supporting particulate carbon measurements.
The article processing charges for this open-access publication were covered by the GEOMAR Helmholtz Centre for Ocean Research Kiel.
This paper was edited by Koji Suzuki and reviewed by two anonymous referees.
Bach, L. T., Riebesell, U., and Schulz, K. G.: Distinguishing between the effects of ocean acidification and ocean carbonation in the coccolithophore Emiliania huxleyi, Limnol. Oceanogr., 56, 2040–2050, https://doi.org/10.4319/lo.2011.56.6.2040, 2011.
Bach, L. T., Mackinder, L. C., Schulz, K. G., Wheeler, G., Schroeder, D. C., Brownlee, C., and Riebesell, U.: Dissecting the impact of CO2 and pH on the mechanisms of photosynthesis and calcification in the coccolithophore Emiliania huxleyi, New Phytol., 199, 121–134, 2013.
Bach, L. T., Riebesell, U., Gutowska, M. A., Federwisch, L., and Schulz, K. G.: A unifying concept of coccolithophore sensitivity to changing carbonate chemistry embedded in an ecological framework, Prog. Oceanogr., 135, 125–138, 2015.
Bach, L. T., Gill, S. J., Rickaby, R. E., Gore, S., and Renforth, P.: CO2 removal with enhanced weathering and ocean alkalinity enhancement: potential risks and co-benefits for marine pelagic ecosystems, Front. Clim., 1, 7, https://doi.org/10.3389/fclim.2019.00007, 2019.
Badger, M. R., Andrews, T. J., Whitney, S. M., Ludwig, M., Yellowlees, D. C., Leggat, W., and Price, G. D.: The diversity and coevolution of Rubisco, plastids, pyrenoids, and chloroplast-based CO 2-concentrating mechanisms in algae, Can. J. Bot., 76, 1052–1071, https://doi.org/10.1139/b98-074, 1998.
Beaufort, L., Probert, I., de Garidel-Thoron, T., Bendif, E. M., Ruiz-Pino, D., Metzl, N., Goyet, C., Buchet, N., Coupel, P., Grelaud, M., Rost, B., Rickaby, R. E. M., and de Vargas, C.: Sensitivity of coccolithophores to carbonate chemistry and ocean acidification, Nature, 476, 80–83, https://doi.org/10.1038/nature10295, 2011.
Bednaršek, N., Pelletier, G., van de Mortel, H., García-Reyes, M., Feely, R., and Dickson, A.: Unifying framework for assessing sensitivity for marine calcifiers to ocean alkalinity enhancement identifies winners, losers and biological thresholds – importance of caution with precautionary principle, EGUsphere [preprint], https://doi.org/10.5194/egusphere-2024-947, 2024.
Bolton, C. T. and Stoll, H. M.: Late Miocene threshold response of marine algae to carbon dioxide limitation, Nature, 500, 558–562, https://doi.org/10.1038/nature12448, 2013.
Broecker, W. and Clark, E.: Ratio of coccolith CaCO3 to foraminifera CaCO3 in late Holocene deep sea sediments, Paleoceanography, 24, PA3205, https://doi.org/10.1029/2009PA001731, 2009.
Cottingham, K. L., Lennon, J. T., and Brown, B. L.: Knowing when to draw the line: designing more informative ecological experiments, Front. Ecol. Environ., 3, 145–152, https://doi.org/10.1890/1540-9295(2005)003[0145:KWTDTL]2.0.CO;2, 2005.
Danbara, A. and Shiraiwa, Y.: The requirement of selenium for the growth of marine coccolithophorids, Emiliania huxleyi, Gephyrocapsa oceanica and Helladosphaera sp. (Prymnesiophyceae), Plant Cell Physiol., 40, 762–766, 1999.
Dickson, A. G. and Millero, F. J.: A comparison of the equilibrium constants for the dissociation of carbonic acid in seawater media, Deep-Sea Res. Pt. A, 34, 1733–1743, https://doi.org/10.1016/0198-0149(87)90021-5, 1987.
Dickson, A. G., Afghan, J. D., and Anderson, G. C.: Reference materials for oceanic CO2 analysis: a method for the certification of total alkalinity, Mar. Chem., 80, 185–197, https://doi.org/10.1016/S0304-4203(02)00133-0, 2003.
Eisaman, M. D., Geilert, S., Renforth, P., Bastianini, L., Campbell, J., Dale, A. W., Foteinis, S., Grasse, P., Hawrot, O., Löscher, C. R., Rau, G. H., and Rønning, J.: Assessing the technical aspects of ocean-alkalinity-enhancement approaches, in: Guide to Best Practices in Ocean Alkalinity Enhancement Research, edited by: Oschlies, A., Stevenson, A., Bach, L. T., Fennel, K., Rickaby, R. E. M., Satterfield, T., Webb, R., and Gattuso, J.-P., Copernicus Publications, State Planet, 2-oae2023, 3, https://doi.org/10.5194/sp-2-oae2023-3-2023, 2023.
Faucher, G., Riebesell, U., and Bach, L. T.: Can morphological features of coccolithophores serve as a reliable proxy to reconstruct environmental conditions of the past?, Clim. Past, 16, 1007–1025, https://doi.org/10.5194/cp-16-1007-2020, 2020.
Feng, E. Y., Koeve, W., Keller, D. P., and Oschlies, A.: Model-Based Assessment of the CO2 Sequestration Potential of Coastal Ocean Alkalinization, Earth's Future, 5, 1252–1266, https://doi.org/10.1002/2017EF000659, 2017.
Gately, J. A., Kim, S. M., Jin, B., Brzezinski, M. A., and Iglesias-Rodriguez, M. D.: Coccolithophores and diatoms resilient to ocean alkalinity enhancement: A glimpse of hope?, Sci. Adv., 9, eadg6066, https://doi.org/10.1126/sciadv.adg6066, 2023.
Gattuso, J.-P., Magnan, A., Billé, R., Cheung, W. W. L., Howes, E. L., Joos, F., Allemand, D., Bopp, L., Cooley, S. R., Eakin, C. M., Hoegh-Guldberg, O., Kelly, R. P., Pörtner, H.-O., Rogers, A. D., Baxter, J. M., Laffoley, D., Osborn, D., Rankovic, A., Rochette, J., Sumaila, U. R., Treyer, S., and Turley, C.: Contrasting futures for ocean and society from different anthropogenic CO2 emissions scenarios, Science, 349, aac4722, https://doi.org/10.1126/science.aac4722, 2015.
GESAMP: High level review of a wide range of proposed marine geoengineering techniques, 98th Edn., edited by: GESAMP, International Maritime Organization, London, https://doi.org/10.25607/OBP-1944, 2019.
Guillard, R. R. L. and Ryther, J. H.: Studies of marine planktonic diatoms: i. Cyclotella nana hustedt, and Detonula confervacea (cleve) gran., Can. J. Microbiol., 8, 229–239, https://doi.org/10.1139/m62-029, 1962.
Hartmann, J., West, A. J., Renforth, P., Köhler, P., De La Rocha, C. L., Wolf-Gladrow, D. A., Dürr, H. H., and Scheffran, J.: Enhanced chemical weathering as a geoengineering strategy to reduce atmospheric carbon dioxide, supply nutrients, and mitigate ocean acidification, Rev. Geophys., 51, 113–149, 2013.
Hartmann, J., Suitner, N., Lim, C., Schneider, J., Marín-Samper, L., Arístegui, J., Renforth, P., Taucher, J., and Riebesell, U.: Stability of alkalinity in ocean alkalinity enhancement (OAE) approaches – consequences for durability of CO2 storage, Biogeosciences, 20, 781–802, https://doi.org/10.5194/bg-20-781-2023, 2023.
He, J. and Tyka, M. D.: Limits and CO 2 equilibration of near-coast alkalinity enhancement, Biogeosciences, 20, 27–43, https://doi.org/10.5194/bg-20-27-2023, 2023.
Iglesias-Rodríguez, M. D., Rickaby, R. E. M., Singh, A., and Gately, J. A.: Laboratory experiments in ocean alkalinity enhancement research, in: Guide to Best Practices in Ocean Alkalinity Enhancement Research, edited by: Oschlies, A., Stevenson, A., Bach, L. T., Fennel, K., Rickaby, R. E. M., Satterfield, T., Webb, R., and Gattuso, J.-P., Copernicus Publications, State Planet, 2-oae2023, 5, https://doi.org/10.5194/sp-2-oae2023-5-2023, 2023.
IPCC: Summary for Policymakers, in: Climate Change 2021: The Physical Science Basis, Contribution of Working Group I to the Sixth Assessment Report of the Intergovernmental Panel on Climate Change, edited by: Masson-Delmotte, V., Zhai, P., Pirani, A., Connors, S. L., Péan, C., Berger, S., Caud, N., Chen, L., Goldfarb, M. I., Gomis, M., Huang, K., Leitzell, E., Lonnoy, J. B. R., Matthews, Y., Maycock, T. K., Waterfield, T., Yelekçi, O., Yu, R., and Zhou, B., Cambridge University Press, Cambridge University Press, Cambridge, United Kingdom and New York, NY, USA, https://doi.org/10.1017/9781009157896.001, 3–32, 2021.
Keller, D. P., Feng, E. Y., and Oschlies, A.: Potential climate engineering effectiveness and side effects during a high carbon dioxide-emission scenario, Nat. Commun., 5, 1–11, 2014.
Kester, D. R., Duedall, I. W., Connors, D. N., and Pytkowicz, R. M.: Preparation of artificial seawater, Limnol. Oceanogr., 12, 176–179, https://doi.org/10.4319/lo.1967.12.1.0176, 1967.
Kottmeier, D. M., Terbrüggen, A., Wolf-Gladrow, D. A., and Thoms, S.: Diel variations in cell division and biomass production of Emiliania huxleyi – Consequences for the calculation of physiological cell parameters, Limnol. Oceanogr., 65, 1781–1800, https://doi.org/10.1002/lno.11418, 2020.
Krug, S. A., Schulz, K. G., and Riebesell, U.: Effects of changes in carbonate chemistry speciation on Coccolithus braarudii: a discussion of coccolithophorid sensitivities, Biogeosciences, 8, 771–777, https://doi.org/10.5194/bg-8-771-2011, 2011.
Langer, G., Geisen, M., Baumann, K.-H., Kläs, J., Riebesell, U., Thoms, S., and Young, J. R.: Species-specific responses of calcifying algae to changing seawater carbonate chemistry, Geochem. Geophy. Geosy., 7, Q09006, https://doi.org/10.1029/2005GC001227, 2006.
Langer, G., Nehrke, G., Probert, I., Ly, J., and Ziveri, P.: Strain-specific responses of Emiliania huxleyi to changing seawater carbonate chemistry, Biogeosciences, 6, 2637–2646, https://doi.org/10.5194/bg-6-2637-2009, 2009.
Mehrbach, C., Culberson, C. H., Hawley, J. E., and Pytkowicx, R. M.: Measurement of the apparent dissociation constants of carbonic acid in seawater at atmospheric pressure, Limnol. Oceanogr., 18, 897–907, https://doi.org/10.4319/lo.1973.18.6.0897, 1973.
Oschlies, A., Stevenson, A., Bach, L. T., Fennel, K., Rickaby, R. E. M., Satterfield, T., Webb, R., and Gattuso, J.-P. (Eds.): Guide to Best Practices in Ocean Alkalinity Enhancement Research, Copernicus Publications, State Planet, 2-oae2023, https://doi.org/10.5194/sp-2-oae2023, 2023.
Paasche, E.: A tracer study of the inorganic carbon uptake during coccolith formation and photosynthesis in the coccolithophorid Coccolithus huxleyi, Physiol. Plant Suppl., 5–82, 1964.
Paul, A. J. and Bach, L. T.: Universal response pattern of phytoplankton growth rates to increasing CO2, New Phytol., 228, 1710–1716, https://doi.org/10.1111/nph.16806, 2020.
Pierrot, D., Lewis, E., and Wallace, D. W. R.: MS Excel program developed for CO2 system calculations, ORNL/CDIAC-105a, Carbon Dioxide Inf. Anal. Center, Oak Ridge Natl. Lab. US Dep, Energy, Oak Ridge, Tennessee, https://doi.org/10.3334/cdiac/otg.co2sys_xls_cdiac105a, 2006.
Poulton, A. J., Mark Moore, C., Seeyave, S., Lucas, M. I., Fielding, S., and Ward, P.: Phytoplankton community composition around the Crozet Plateau, with emphasis on diatoms and Phaeocystis, Deep-Sea Res. Pt. II, 54, 2085–2105, https://doi.org/10.1016/j.dsr2.2007.06.005, 2007.
R Core Team: R: A language and environment for statistical computing, R Foundation for Statistical Computing, Vienna, Austria [code], https://www.r-project.org/ (last access: 10 July 2024), 2023.
Renforth, P. and Henderson, G.: Assessing ocean alkalinity for carbon sequestration, Rev. Geophys., 55, 636–674, 2017.
Riebesell, U., Wolf-Gladrow, D. A., and Smetacek, V.: Carbon dioxide limitation of marine phytoplankton growth rates, Nature, 361, 249–251, 1993.
Riebesell, U., Zondervan, I., Rost, B., Tortell, P. D., Zeebe, R. E., and Morel, F. M.: Reduced calcification of marine plankton in response to increased atmospheric CO2, Nature, 407, 364–367, 2000.
Riebesell, U., Basso, D., Geilert, S., Dale, A. W., and Kreuzburg, M.: Mesocosm experiments in ocean alkalinity enhancement research, in: Guide to Best Practices in Ocean Alkalinity Enhancement Research, edited by: Oschlies, A., Stevenson, A., Bach, L. T., Fennel, K., Rickaby, R. E. M., Satterfield, T., Webb, R., and Gattuso, J.-P., Copernicus Publications, State Planet, 2-oae2023, 6, https://doi.org/10.5194/sp-2-oae2023-6-2023, 2023.
Ringham, M. C., Hirtle, N., Shaw, C., Lu, X., Herndon, J., Carter, B. R., and Eisaman, M. D.: An assessment of ocean alkalinity enhancement using aqueous hydroxides: kinetics, efficiency, and precipitation thresholds, Biogeosciences, 21, 3551–3570, https://doi.org/10.5194/bg-21-3551-2024, 2024.
Rost, B., Riebesell, U., Burkhardt, S., and Sültemeyer, D.: Carbon acquisition of bloom-forming marine phytoplankton, Limnol. Oceanogr., 48, 55–67, 2003.
Rost, B., Riebesell, U., and Sültemeyer, D.: Carbon acquisition of marine phytoplankton: Effect of photoperiod length, Limnol. Oceanogr., 51, 12–20, https://doi.org/10.4319/lo.2006.51.1.0012, 2006.
Schulz, K. G., Rost, B., Burkhardt, S., Riebesell, U., Thoms, S., and Wolf-Gladrow, D. A.: The effect of iron availability on the regulation of inorganic carbon acquisition in the coccolithophore Emiliania huxleyi and the significance of cellular compartmentation for stable carbon isotope fractionation, Geochim. Cosmochim. Ac., 71, 5301–5312, https://doi.org/10.1016/j.gca.2007.09.012, 2007.
Sett, S., Bach, L. T., Schulz, K. G., Koch-Klavsen, S., Lebrato, M., and Riebesell, U.: Temperature modulates coccolithophorid sensitivity of growth, photosynthesis and calcification to increasing seawater pCO2, PloS One, 9, e88308, https://doi.org/10.1371/journal.pone.0088308, 2014.
Suitner, N., Faucher, G., Lim, C., Schneider, J., Moras, C. A., Riebesell, U., and Hartmann, J.: Ocean alkalinity enhancement approaches and the predictability of runaway precipitation processes: results of an experimental study to determine critical alkalinity ranges for safe and sustainable application scenarios, Biogeosciences, 21, 4587–4604, https://doi.org/10.5194/bg-21-4587-2024, 2024.
Taylor, L. L., Quirk, J., Thorley, R., Kharecha, P. A., Hansen, J., Ridgwell, A., Lomas, M. R., Banwart, S. A., and Beerling, D. J.: Enhanced weathering strategies for stabilizing climate and averting ocean acidification, Nat. Clim. Change, 6, 402–406, 2016.
Trimborn, S., Langer, G., and Rost, B. R.: Effect of varying calcium concentrations and light intensities on calcification and photosynthesis in Emiliania huxleyi, Limnol. Oceanogr., 52, 2285–2293, https://doi.org/10.4319/lo.2007.52.5.2285, 2007.
United Nations Framework Convention on Climate Change: Adoption of the Paris Agreement. Report No. FCCC/CP/2015/L.9/Rev.1, https://unfccc.int/sites/default/files/resource/docs/2015/cop21/eng/l09r01.pdf (last access: 1 May 2024), 2015.
Westbroek, P., Brown, C. W., Bleijswijk, J. van, Brownlee, C., Brummer, G. J., Conte, M., Egge, J., Fernández, E., Jordan, R., Knappertsbusch, M., Stefels, J., Veldhuis, M., van der Wal, P., and Young, J.: A model system approach to biological climate forcing, The example of Emiliania huxleyi, Glob. Planet. Change, 8, 27–46, https://doi.org/10.1016/0921-8181(93)90061-R, 1993.
Wheeler, G. L., Sturm, D., and Langer, G.: Gephyrocapsa huxleyi (Emiliania huxleyi) as a model system for coccolithophore biology, J. Phycol., 59, 1123–1129 https://doi.org/10.1111/jpy.13404, 2023
Wolf-Gladrow, D. A., Zeebe, R. E., Klaas, C., Körtzinger, A., and Dickson, A. G.: Total alkalinity: The explicit conservative expression and its application to biogeochemical processes, Mar. Chem., 106, 287–300, 2007.
Zeebe, R. E. and Wolf-Gladrow, D.: CO2 in Seawater: Equilibrium, Kinetics, Isotopes, Gulf Professional Publishing, 382 pp., ISBN 9780444509468, 2001.
Zondervan, I., Rost, B., and Riebesell, U.: Effect of CO2 concentration on the PIC POC ratio in the coccolithophore Emiliania huxleyi grown under light-limiting conditions and different daylengths, J. Exp. Mar. Biol. Ecol., 272, 55–70, https://doi.org/10.1016/S0022-0981(02)00037-0, 2002.